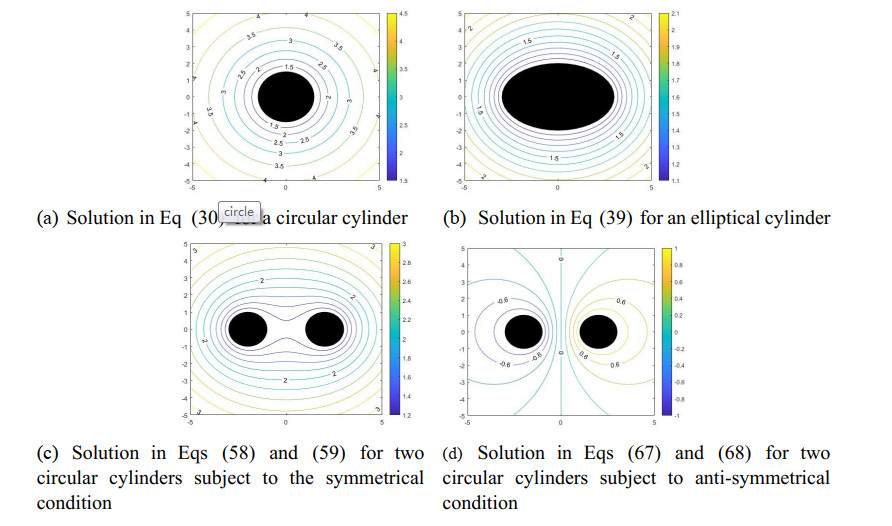
Trash mulches are remarkably effective in preventing soil erosion, reducing runoff-sediment transport-erosion, and increasing infiltration. The study was carried out to observe the sediment outflow from sugar cane leaf (trash) mulch treatments at selected land slopes under simulated rainfall conditions using a rainfall simulator of size 10 m × 1.2 m × 0.5 m with the locally available soil material collected from Pantnagar. In the present study, trash mulches with different quantities were selected to observe the effect of mulching on soil loss reduction. The number of mulches was taken as 6, 8 and 10 t/ha, three rainfall intensities viz. 11, 13 and 14.65 cm/h at 0, 2 and 4% land slopes were selected. The rainfall duration was fixed (10 minutes) for every mulch treatment. The total runoff volume varied with mulch rates for constant rainfall input and land slope. The average sediment concentration (SC) and sediment outflow rate (SOR) increased with the increasing land slope. However, SC and outflow decreased with the increasing mulch rate for a fixed land slope and rainfall intensity. The SOR for no mulch-treated land was higher than trash mulch-treated lands. Mathematical relationships were developed for relating SOR, SC, land slope, and rainfall intensity for a particular mulch treatment. It was observed that SOR and average SC values correlated with rainfall intensity and land slope for each mulch treatment. The developed models' correlation coefficients were more than 90%.
Citation: Sachin Kumar Singh, Dinesh Kumar Vishwakarma, Salwan Ali Abed, Nadhir Al-Ansari, P. S. Kashyap, Akhilesh Kumar, Pankaj Kumar, Rohitashw Kumar, Rajkumar Jat, Anuj Saraswat, Alban Kuriqi, Ahmed Elbeltagi, Salim Heddam, Sungwon Kim. Soil erosion control from trash residues at varying land slopes under simulated rainfall conditions[J]. Mathematical Biosciences and Engineering, 2023, 20(6): 11403-11428. doi: 10.3934/mbe.2023506
[1] | Xiao-Min Huang, Xiang-ShengWang . Traveling waves of di usive disease models with time delay and degeneracy. Mathematical Biosciences and Engineering, 2019, 16(4): 2391-2410. doi: 10.3934/mbe.2019120 |
[2] | Kang Wu, Yibin Lu . Numerical computation of preimage domains for spiral slit regions and simulation of flow around bodies. Mathematical Biosciences and Engineering, 2023, 20(1): 720-736. doi: 10.3934/mbe.2023033 |
[3] | Bing Hu, Minbo Xu, Zhizhi Wang, Jiahui Lin, Luyao Zhu, Dingjiang Wang . Existence of solutions of an impulsive integro-differential equation with a general boundary value condition. Mathematical Biosciences and Engineering, 2022, 19(4): 4166-4177. doi: 10.3934/mbe.2022192 |
[4] | Meng Zhao, Wan-Tong Li, Yang Zhang . Dynamics of an epidemic model with advection and free boundaries. Mathematical Biosciences and Engineering, 2019, 16(5): 5991-6014. doi: 10.3934/mbe.2019300 |
[5] | Huy Tuan Nguyen, Nguyen Van Tien, Chao Yang . On an initial boundary value problem for fractional pseudo-parabolic equation with conformable derivative. Mathematical Biosciences and Engineering, 2022, 19(11): 11232-11259. doi: 10.3934/mbe.2022524 |
[6] | Sai Zhang, Li Tang, Yan-Jun Liu . Formation deployment control of multi-agent systems modeled with PDE. Mathematical Biosciences and Engineering, 2022, 19(12): 13541-13559. doi: 10.3934/mbe.2022632 |
[7] | P. Vafeas, A. Skarlatos, P. K. Papadopoulos, P. Svarnas, N. Sarmas . A boundary value problem of heat transfer within DBD-based plasma jet setups. Mathematical Biosciences and Engineering, 2023, 20(10): 18345-18367. doi: 10.3934/mbe.2023815 |
[8] | Zhenwu Xiang, Qi Mao, Jintao Wang, Yi Tian, Yan Zhang, Wenfeng Wang . Dmbg-Net: Dilated multiresidual boundary guidance network for COVID-19 infection segmentation. Mathematical Biosciences and Engineering, 2023, 20(11): 20135-20154. doi: 10.3934/mbe.2023892 |
[9] | Fugeng Zeng, Yao Huang, Peng Shi . Initial boundary value problem for a class of p-Laplacian equations with logarithmic nonlinearity. Mathematical Biosciences and Engineering, 2021, 18(4): 3957-3976. doi: 10.3934/mbe.2021198 |
[10] | M. B. A. Mansour . Computation of traveling wave fronts for a nonlinear diffusion-advection model. Mathematical Biosciences and Engineering, 2009, 6(1): 83-91. doi: 10.3934/mbe.2009.6.83 |
Trash mulches are remarkably effective in preventing soil erosion, reducing runoff-sediment transport-erosion, and increasing infiltration. The study was carried out to observe the sediment outflow from sugar cane leaf (trash) mulch treatments at selected land slopes under simulated rainfall conditions using a rainfall simulator of size 10 m × 1.2 m × 0.5 m with the locally available soil material collected from Pantnagar. In the present study, trash mulches with different quantities were selected to observe the effect of mulching on soil loss reduction. The number of mulches was taken as 6, 8 and 10 t/ha, three rainfall intensities viz. 11, 13 and 14.65 cm/h at 0, 2 and 4% land slopes were selected. The rainfall duration was fixed (10 minutes) for every mulch treatment. The total runoff volume varied with mulch rates for constant rainfall input and land slope. The average sediment concentration (SC) and sediment outflow rate (SOR) increased with the increasing land slope. However, SC and outflow decreased with the increasing mulch rate for a fixed land slope and rainfall intensity. The SOR for no mulch-treated land was higher than trash mulch-treated lands. Mathematical relationships were developed for relating SOR, SC, land slope, and rainfall intensity for a particular mulch treatment. It was observed that SOR and average SC values correlated with rainfall intensity and land slope for each mulch treatment. The developed models' correlation coefficients were more than 90%.
Five basic quantities (voltage, charge, current, capacitance, and resistance) in electrostatics are involved in almost all applications. Electrostatics generally plays an important role in improving the performance of microelectro/mechanical systems (MEMS) and electron devices in the design stage. Many numerical methods (e.g., the finite difference method, the variational method, the moment method, the finite element method, and the boundary element method) were popularly used for engineering problems. Among diverse numerical techniques, the finite element method (FEM) and the boundary integral equation method (BIEM), as well as the boundary element method (BEM) become acceptable tools for engineers due to the increasing development of digital computing power. Here, we may focus on the mathematical study of the BIEM for electrostatics of two identical cylinders. Researchers have paid attention to the dual BEM paper of IEEE in 2003 [1], which has received nearly 5000 views in the Research Gate.
For a pair of two conducting cylinders, there is a large amount of literature on charged cylinders [2,3,4]. Different solutions existed to the electrostatic problem of two identical parallel cylinders held at the same (symmetric) potential [2,3]. A note was given to show their equivalence, and the identities were confirmed [4]. Four distinct solutions for the potential distribution around two equal circular parallel conducting cylinders by [2,3,5,6] were demonstrated to be equivalent by Lekner [7] by ways of several identities. Here, we may try an alternative way of BIEM using degenerate kernels to revisit this problem. A degenerate kernel is based on the method of separation variables, but it separates the variables in the two-point kernel function. Although the BIE in conjunction with the available degenerate kernel can only solve simple geometries and the results may be obtained more directly by using the method of separation variables for the solution instead of the fundamental solution, the tool can explain the rank-deficiency mechanism in the BIE/BEM such as degenerate scale, degenerate boundary, spurious eigenvalues and fictitious frequency, which is meaningful to the BEM community. Besides, symmetric and anti-symmetric cases are both considered. Regarding the anti-symmetric electrostatic potential, Lebedev et al. [8] have provided a closed-form solution by using the bipolar coordinates. The solution is interestingly found to be the simplest method of fundamental solution (MFS) of two opposite strengths of sources at the two foci. It is not trivial to check the asymptotic behavior at infinity of the two cases, symmetric and anti-symmetric. Besides, whether the equilibrium of the boundary flux along the two cylinders is satisfied or not is also our main concern.
Regarding the potential problem of a two-dimensional plane containing two circular boundaries, Chen and Shen [9] studied the multiply-connected Laplace problem. They found that a degenerate scale depends on the outer boundary. Chen et al. [10] solved the Laplace problem by using the BIEM in conjunction with the degenerate kernel to derive an analytical solution. It is found that a degenerate scale may occur due to the introduction of the logarithmic kernel for the two-dimensional case. Efficient techniques for the rank-deficiency of the BEM in electrostatic problems were proposed by Chyuan et al. [11]. Later, it was found that the special (degenerate) geometry happened to be the shape of unit logarithmic capacity. Kuo et al. [12] studied the degenerate scale for regular N-gon domains by using complex variables. Numerical implementation was also done by using the BEM. Kuo et al. [13] revisited the degenerate scale for an infinite plane problem containing two circular holes using the conformal mapping. Chen et al. [14] linked the logarithmic capacity in the potential theory and the degenerate scale in the BEM for two tangent discs. The logarithmic capacity of the line segment as well as the double degeneracy in the BIEM/BEM was studied by Chen et al. [15]. Due to the use of the two-dimensional fundamental solution in the BIEM, the solution space is expanded, and sometimes the corresponding matrix is rank deficient in the BEM. In other words, the integral operator of the logarithmic kernel is range deficient. A corresponding chart to show the rank deficiency and the null space of the integral operator of single, double layer potentials and their derivatives was given in [16,17,18], while the original one was provided in the face cover of the Strang book [19]. Fikioris et al. [20] solved rectangularly shielded lines by using the Carleman-Vekua method. In the mentioned paper [20], it is interesting to find that its formulation also needs a constraint [21] to ensure a unique solution. This outcome is similar to the paper of Chen et al. [22] using the Fichera's approach, where an additional constraint is also required.
In this paper, we revisit two cylinders of electrostatics by using the BIE with the degenerate kernel of the bipolar coordinates. Both the symmetric and anti-symmetric specified potentials are considered. Besides, the logarithmic capacity is also discussed. The boundary potential and flux are expanded by using the Fourier series, while the fundamental solution is represented by using the degenerate kernel. The equilibrium of boundary flux and the asymptotic behavior at infinity are also examined. The solution space expanded using the BIEM is compared with the true solution space. After summarizing the single (circle and ellipse) and two cylinders, a conclusion for constructing the solution space can be made.
First, we consider a conducting cylinder. The governing equation and the Dirichlet boundary condition are shown below:
∇2u(x)=0, x∈D,u(x)=ˉu(x), x∈B,u(x)= ln |x|+O(1), x→∞, | (1) |
where ∇2, D and B are the Laplace operator, the domain of interest and the boundary, respectively. Furthermore, x is the position vector of a field point and ˉu(x) is the specified B.C. The integral formulation for the Laplace problem is derived from Green's third identity. The representation of the conventional integral equation for the domain point is written as
2πu(x)=∫BT(s,x)ˉu(s)dB(s)−∫BU(s,x)t(s)dB(s),x∈D, | (2) |
where s is the position vector of a source point, U(s,x)= ln |x−s| is the fundamental solution, T(s,x)=∂U(s,x)∂ns, and t(x) is the unknown boundary flux. By moving the field point to the smooth boundary, Eq (2) becomes:
πˉu(x)=C.P.V.∫BT(s,x)ˉu(s)dB(s)−∫BU(s,x)t(s)dB(s),x∈B, | (3) |
where the C.P.V. denotes the Cauchy principal value, and T(s,x)=∂U(s,x)/∂ns is the closed-form kernel. Once the field point x locates outside the domain, we obtain the null-field integral equation as shown below:
0=∫BT(s,x)ˉu(s)dB(s)−∫BU(s,x)t(s)dB(s),x∈Dc. | (4) |
where Dc is the complementary domain. By employing the proper degenerate kernel (U(s,x)) to represent the closed-form fundamental solution, the collocation point can be exactly located on the real boundary free of facing the singular integral. Equations (2) and (4) can be rewritten as:
2πu(x)=∫BTdk(s,x)ˉu(s)dB(s)−∫BUdk(s,x)t(s)dB(s),x∈D∪B | (5) |
and
0=∫BTdk(s,x)ˉu(s)dB(s)−∫BUdk(s,x)t(s)dB(s),x∈Dc∪B. | (6) |
where Tdk(s,x) and Udk(s,x) are the corresponding degenerate kernels to represent T(s,x) and U(s,x), respectively. By setting the field point x=(ρ,ϕ) and the source point s=(R,θ) in the polar coordinates for a circular domain, the closed-form fundamental solution in Eqs (5) and (6) can be expressed by using the degenerate kernel form as shown below:
Udk(s,x)={Ui(R,θ;ρ,ϕ)= ln R−∑∞m=11m(ρR)m cos m(θ−ϕ), R≥ρ, (a)Ue(R,θ;ρ,ϕ)= ln ρ−∑∞m=11m(Rρ)m cos m(θ−ϕ), ρ>R, (b). | (7) |
and
Tdk(s,x)={Ti(R,θ;ρ,ϕ)=−(1R+∑∞m=1(ρmRm+1) cos m(θ−ϕ)), R>ρ, (a)Te(R,θ;ρ,ϕ)=∑∞m=1(Rm−1ρm) cos m(θ−ϕ), ρ>R. (b). | (8) |
The unknown boundary flux t(s) is expanded in terms of Fourier series as shown below:
t(s)=1Js(a0+∑∞n=1an cos (nθ)+∑∞n=1bn sin (nθ)), 0≤θ≤2π, | (9) |
where Js=1 is the Jacobian term, a0, an and bn are unknown coefficients. The given boundary condition is
ˉu(x)=v. | (10) |
where v is a constant. By considering R=a in Eqs (6)–(8), the coefficient of the Fourier constant base is
−a ln a a0=v, | (11) |
where a is the radius of the circular cylinder. Equation (11) indicates that the occurring mechanism of a degenerate scale is
ln a=0. | (12) |
When a=1, the coefficient of a0 cannot be determined. It results in a non-unique solution. This critical size is called a degenerate scale. In Rumely's book [23], the logarithmic capacity, cL, of a circle is equal to its radius. It is easily found that the special (degenerate) geometry happens to be the shape of unit logarithmic capacity. The discriminant Dp(a) of the degenerate scale in the BEM/BIEM for a circular boundary is written as
Dp(a)= ln a. | (13) |
If Dp(a)≠0, this size is an ordinary scale and there exists a unique solution. Otherwise, according to the Fredholm alternative theorem, there is no solution or infinite solutions. For an ordinary scale, the boundary flux, of the electrostatic field along the boundary, is obtained as
t(x)=−va ln a,x∈B. | (14) |
The unique solution of electrostatic potential is obtained by
u(x)=v ln ρ ln a, | (15) |
as shown in Figure 1(a). Even though the electrostatic field along the boundary in Eq (14) is not in equilibrium, i.e. ∫Bt(x)dB(x)≠0, the electrostatic field at infinity, Γ∞, would exist and satisfy the equilibrium condition together in total, ∫B+Γ∞t(x)dB(x)=0. If we normalize the potential on the cylinder to the unity, and let λ be the dimensionless ratio, the potential becomes
u(x)=v+λud(x), | (16) |
where
ud(x)= ln ρ− ln a. | (17) |
The solution by using the direct BIE of Eq (15) is the special case of Eq (16) by setting λ=vDp(a). When the size of the boundary is a degenerate scale, i.e., a=1 and Dp(a)= ln a=0, it has no solution if v≠0. If v=0, then the constant term in Eq (9), a0, is a free constant. The electrostatic potential yields
u(x)=a0 ln ρ, | (18) |
and Eq (16) would reduce to
u(x)=λud(x), | (19) |
and ud(x) in Eq (19) reduces to ln ρ since ln a=0. It is easy to find that a0 and λ are equivalent.
For an elliptical case, we naturally utilize the elliptic coordinates to solve the problem in the BIE. The relation between the Cartesian coordinates and the elliptic coordinates is given below:
x=c cosh ξ cos η,y=c sinh ξ sin η. | (20) |
where c is the focal length. By separating the source point and the field point in the elliptic coordinates [24] to represent the closed-form fundamental solution, we have
Udk(s,x)= ln |x−s|={Ui(ξs,ηs;ξx,ηx)=ξs+ ln c2−∑∞m=12me−mξs cosh m ξx cos m ηx cos m ηs −∑∞m=12me−mξs sinh m ξx sin m ηx sin m ηs, ξs≥ξx, (a)Ue(ξs,ηs;ξx,ηx)=ξx+ ln c2−∑∞m=12me−mξx cosh m ξs cos m ηx cos m ηs −∑∞m=12me−mξx sinh m ξs sin m ηx sin m ηs, ξs<ξx, (b) | (21) |
Tdk(s,x)=∂U(s,x)∂ns={Ti(ξs,ηs;ξx,ηx)=−1Js(1+2∑∞m=1e−mξs cosh m ξx cos m ηx cos m ηs +2∑∞m=1e−mξs sinh m ξx sin m ηx sin m ηs), ξs>ξx, (a)Te(ξs,ηs;ξx,ηx)=1Js(2∑∞m=1e−mξx sinh m ξs cos m ηx cos m ξs+2∑∞m=1e−mξx cosh m ξs sin m ηx sin m ηs), ξs<ξx. (b) | (22) |
where Js=c√ cosh 2ξs sin 2ηs+ sinh 2ξs cos 2ηs. The unknown boundary flux t(s) is expanded in terms of generalized Fourier series. We have
t(s)=1Js(a0+∑∞n=1an cos (nηs)+∑∞n=1bn sin (nηs)), 0≤ηs≤2π, | (23) |
where a0, an and bn are unknown coefficients. The given boundary condition is
ˉu(x)=v. | (24) |
By substituting Eqs (21a), (22a), (23) and (24) into Eq (6), the coefficient of the Fourier constant base is
−(ξ0+ ln c2)a0=v, | (25) |
Equation (25) indicates that the occurring mechanism of a degenerate scale is
ξ0+ ln c2=0. | (26) |
Equation (26) yields the degenerate scale of a+b2=1, where a and b are the semi-major and semi-minor axes of an ellipse, respectively. According to Eq (25), the discriminant of a degenerate scale in the BEM/BIEM is obtained
De(c,ξ0)=ξ0+ ln c2= ln (a+b2). | (27) |
In Rumely's book [23], the logarithmic capacity of an ellipse is equal to a+b2. According to Eqs (13) and (27), the logarithmic capacity, cL, and the discriminant, De(⋅), satisfy the relation,
cL=eDe(⋅). | (28) |
The relationship of the discriminant, logarithmic capacity, and degenerate scale are summarized in Table 1. If De(c,ξ0) is not equal to zero, this size is an ordinary scale with a unique solution. Otherwise, according to the Fredholm alternative theorem, it has no solution or infinite solution. For an ordinary scale, the boundary flux is obtained by
t(x)=−vDe(c,ξ0),x∈B. | (29) |
![]() |
The unique solution of electrostatic potential is
u(x)=(ξx+ ln c2)(vDe(c,ξ0)), | (30) |
as shown in Figure 1(b). Even though the boundary flux in Eq (29) is not in equilibrium, i.e., ∫Bt(x)dB(x)≠0, the electrostatic field at infinity, Γ∞, would exist and satisfy the equilibrium condition together in total, i.e., ∫B+Γ∞t(x)dB(x)=0. If we normalize the potential on the cylinder to the unity, and let λ be the dimensionless ratio, the potential becomes
u(x)=v+λud(x), | (31) |
where
ud(x)=(ξx+ ln c2)−(ξ0+ ln c2)=ξx−ξ0. | (32) |
A neat formula of ud(x) could be defined as ud(x)=De(ξx)−De(ξ0). The solution by using the direct BIE of Eq (30) is the special case of Eq (31), if λ=vDe(c,ξ0).
When the size of the boundary is a degenerate scale, De(c,ξ0)=ξ0+lnc2=0, it is no solution if v≠0. If v=0, the constant term in Eq (23), a0, is a free constant. The electrostatic potential yields
u(x)=(ξx+ ln c2)a0, | (33) |
and Eq (16) reduces to
u(x)=λud(x), | (34) |
where ud(x) in Eq (32) reduces to ξx+ ln c2 since De(ξ0) is equal to zero. It is easy to find that a0 and λ are equivalent. In addition, the degenerate scale in the BEM/BIEM is due to the logarithmic kernel.
For the single elliptical cylinder, the degenerate kernel is expanded in terms of the generalized form as
Udk(s,x)= ln |x−s|={Ui(ξs,ηs;ξx,ηx)=De(ξs)−∑∞m=1αm(ξs,ηs;ξx,ηx), ξs≥ξx, (a)Ue(ξs,ηs;ξx,ηx)=De(ξx)−∑∞m=1αm(ξx,ηx;ξs,ηs), ξs<ξx, (b) | (35) |
Tdk(s,x)=∂U(s,x)∂ns={Ti(ξs,ηs;ξx,ηx)=−1Js(D'eξs)−∑∞m=1βm(ξs,ηs;ξx,ηx)), ξs>ξx, (a)Te(ξs,ηs;ξx,ηx)=1Js∑∞m=1βm(ξs,ηs;ξx,ηx), ξs<ξx, (b) | (36) |
where ξ and η are the radial and angular directions, respectively, De(⋅) is the constant function for ηs and other term is α(⋅). The unknown boundary flux t(s) is expanded in terms of the generalized Fourier series as shown below:
t(s)=1Js(a0+∑∞n=1an cos (nηs)+∑∞n=1bn sin (nηs)), 0≤ηs≤2π, | (37) |
where a0, an and bn are unknown coefficients. By substituting Eqs (35a), (36a), (37) and the boundary condition (Eq (10)) into Eq (6), the coefficient of the Fourier constant base is
De(ξ0)a0=−vD'e(ξ0), | (38) |
If D(ξ0)≠0, then the unique solution of electrostatic potential is
u(x)=vDe(ξ0)De(ξx), | (39) |
and
ud(x)=De(ξx)−De(ξ0). | (40) |
When the size of the boundary is a degenerate scale, De(ξ0)=0, there is no solution if v≠0. If v=0, the constant term in Eq (37), a0, is a free constant. The electrostatic potential yields
u(x)=(De(ξx)−De(ξ0))a0, | (41) |
and Eq (40) reduces to
u(x)=λud(x), | (42) |
where ud(x) in Eq (40) is reduced to De(ξx), since De(ξ0) is equal to zero. By using the generalized form of Eqs (35) and (36), the analytical and neat form of ud(x) for the single elliptical cylinder is derived. It is easy to find that a0 and λ are equivalent. The generalized potential and the solution by using the BIEM are compared in Table 2.
![]() |
In this section, we consider two circular cylinders of electrostatics. The Dirichlet boundary conditions of two circular cylinders are given by
ul(x)=v1 and ur(x)=v2,x∈B, | (43) |
where ul(x) and ur(x) are potentials of the left and right circular boundaries, respectively, x is the position vector of the field point, B is the boundary, and v1 and v2 are specified constant potentials. The original problem can be decomposed into a symmetric problem and an anti-symmetric problem as shown below:
ul(x)=ur(x)=v,x∈B, symmetry BC, | (44) |
and
ul(x)=−ur(x)=v,x∈B. anti-symmetry BC. | (45) |
Since the problem contains two circular boundaries, we naturally employ the bipolar coordinates to express the closed-form fundamental solution. The relation between the Cartesian coordinates and the bipolar coordinates is shown below:
x=c sinh η cosh η− cos ξ,y=c sin ξ cosh η− cos ξ. | (46) |
where η and ξ are the radial and angular coordinates, respectively, c is the half distance between the two foci of the bipolar coordinates. By separating the source point and the field point in the bipolar coordinates [9] for the closed-form fundamental solution, we have
Udk(s,x)= ln |x−s|= |
{ ln (2c)+ηs−∑∞m=11m{e−m(ηs−ηx) cos [m(ξx−ξs)]−emηx cos (mξx)−emηs cos (mξs)},0>ηs≥ηx ln (2c)+ηx−∑∞m=11m{e−m(ηx−ηs) cos [m(ξx−ξs)]−emηx cos (mξx)−emηs cos (mξs)},0>ηx>ηs ln (2c)−∑∞m=11m{e−m(ηx−ηs) cos [m(ξx−ξs)]−e−mηx cos (mξx)−emηs cos (mξs)},ηx>0>ηs ln (2c)−ηs−∑∞m=11m{e−m(ηx−ηs) cos [m(ξx−ξs)]−e−mηx cos (mξx)−e−mηs cos (mξs)},ηx≥ηs>0 ln (2c)−ηx−∑∞m=11m{e−m(ηs−ηx) cos [m(ξx−ξs)]−e−mηx cos (mξx)−e−mηs cos (mξs)},ηs>ηx>0 ln (2c)−∑∞m=11m{e−m(ηs−ηx) cos [m(ξx−ξs)]−emηx cos (mξx)−e−mηs cos (mξs)},ηs>0>ηx | (47) |
Tdk(s,x)=∂U(s,x)∂ns= |
{1Js{−1+∑∞m=1[−e−m(ηs−ηx) cos [m(ξx−ξs)]−emηs cos (mξs)]},0>ηs≥ηx1Js∑∞m=1{e−m(ηx−ηs) cos [m(ξx−ξs)]−emηs cos (mξs)},0>ηx>ηs1Js∑∞m=1{e−m(ηx−ηs) cos [m(ξx−ξs)]−emηs cos (mξs)},ηx>0>ηs1Js{−1+∑∞m=1[e−m(ηx−ηs) cos [m(ξx−ξs)]−e−mηs cos (mξs)]},ηx≥ηs>01Js∑∞m=1{e−m(ηs−ηx) cos [m(ξx−ξs)]−e−mηs cos (mξs)},ηs>ηx>0 1Js∑∞m=1{e−m(ηs−ηx) cos [m(ξx−ξs)]−e−mηs cos (mξs)},ηs>0>ηx | (48) |
where x=(ηx,ξx), s=(ηs,ξs) and Js=c/[cosh(ηs)−cos(ξs)].
The boundary condition of the symmetry problem is shown in Eq (44). The unknown boundary densities on the two circular cylinders can be expanded by using the generalized Fourier series as shown below:
tM(s)={1Js(al0+∑∞n=1aln cos n ξs+∑∞n=1bln sin n ξs), ηs < 0,s∈Bl,1Js(ar0+∑∞n=1arn cos n ξs+∑∞n=1brn sin n ξs), ηs≥0,s∈Br, | (49) |
where al0,aln,bln,ar0,arn and brn are unknown coefficients of the generalized Fourier series. By substituting Eqs (47a), (47f), (48a), (48f), (44) and (49) into Eq (6), and collocating the null-field point on the left boundary, Bl, we have
−2πv−π{2( ln (2c)−η0)al0+∑∞n=11ne−nη0aln−∑∞n=11n(−2e−nη0al0+aln) cos n ξx−∑∞n=11nbln sin n ξx}−π{2 ln (2c)ar0+∑∞n=11ne−nη0arn+∑∞n=11n[(2e−nη0ar0−e−2nη0arn) cos n ξx−e−2nη0brn sin n ξx]}=0. | (50) |
Similarly, substituting Eqs (47c), (47d), (48c), (48d), (44) and (49) into Eq (6), and collocating the null-field point on the right boundary, Br, we have
−π{2 ln (2c)al0+∑∞n=11ne−nη0aln+∑∞n=11n[(−e−2nη0aln+2e−nη0al0) cos n ξx−e−2nη0bln sin n ξx]}−2πv−π{2( ln (2c)−η0)ar0+∑∞n=11ne−nη0arn+∑∞n=11n[(2e−nη0ar0−arn) cos n ξx−brn sin n ξx]}=0. | (51) |
By adding Eqs (50) and (51) together, we obtain
(4 ln (2c)−2η0)(al0+ar0)+2∑∞n=11ne−nη0(aln+arn)+∑∞n=11n[−(1+e−2nη0)(aln+arn)+4e−nη0(al0+ar0)] cos n ξx−∑∞n=11n[(1+e−2nη0)(bln+brn)] sin n ξx=−4v | (52) |
After comparing the coefficient of generalized Fourier bases, we have
{(4ln(2c)−2η0)(al0+ar0)+2∑∞n=11ne−nη0(aln+arn)=−4v,n=1, 2, 3...1n(1+e−2nη0)(aln+arn)+4e−nη0(al0+ar0)=0,n=1, 2, 3...1n(1+e−2nη0)(bln+brn)=0,n=1, 2, 3... | (53) |
By similarly subtracting Eq (50) from Eq (51), we have
π{−2η0al0+∑∞n=11n[(−1+e−2nη0)aln cos n ξx+(−1+e−2nη0)bln sin n ξx]}+π{2η0ar0+∑∞n=11n[(1−e−2nη0)arn cos n ξx+(1−e−2nη0)brn sin n ξx]}=0 | (54) |
After comparing the coefficient of generalized Fourier bases, we have
{2η0(al0−ar0)=0,n=1, 2, 3...1n(−1+e−2nη0)(aln−arn)=0, n=1, 2, 3...1n(−1+e−2nη0)(bln−brn)=0, n=1, 2, 3... | (55) |
In order to solve the coefficients al0 and ar0, we need to define a discriminant as shown below:
Db(c,η0)=2 ln (2c)−η0+∑∞n=11n4e−2nη0(1+e−2nη0). | (56) |
For the case of two cylinders, Rumely [23] employed the complex variable to derive the logarithmic capacity, as shown in Table 1. Since the logarithmic capacity is not a closed-form or an exact formula, the postulate in Eq (28) for the case of two cylinders could not be analytically verified at present. If Db≠0, the geometry of the problem is an ordinary scale, Eqs (53) and (55) yield the coefficients as shown below:
al0=ar0=vDb(c,η0)aln=arn=2e−nη0(1+e−2nη0)al0,n=1,2,3...bln=brn=0,n=1,2,3... | (57) |
Substituting Eqs (47b), (47f), (48b), (48f), (44) and the obtained unknown boundary densities into Eq (5) for the field solution of ηx<0, we have the unique solution
u(x)=vDb(c,η0)((2 ln (2c)+ηx+∑∞n=14ne−2nη01+e−2nη0)−∑∞n=12n(e−nη0enηx+e−nηxenη0+e−nη0−enηx) cos (nξx)),−η0≤ηx<0. | (58) |
Similar substitution of Eqs (47c), (47e), (48c), (48e), (44) and the obtained unknown densities into Eq (5), the field solution for ηx≥0 yields
u(x)=vDb(c,η0)((2 ln (2c)−ηx+∑∞n=14ne−2nη01+e−2nη0)−∑∞n=12n(e−nη0enηx+e−nηxenη0+e−nη0−e−nηx) cos (nξx)),η0≥ηx≥0. | (59) |
It is found that Eqs (58) and (59) show the symmetry solution. All potentials are shown in Figure 1(c). This solution will be compared and discussed with that of Darevski [2] later.
If Db(c,η0)=0, a degenerate scale occurs. When the constant potential v≠0, it yields no solution. When the constant potential v=0, it yields infinite solutions. Equations (53) and (55) yield the coefficients as shown below:
ar0=al0=k,aln=arn=4e−nη0(1+e−2nη0)k,n=1,2,3...bln=brn=0,n=1,2,3... | (60) |
where k is an arbitrary constant. In case of a degenerate scale, η0 becomes
η0=2 ln (2c)+∞∑n=11n4e−2nη0(1+e−2nη0) | (61) |
Substituting Eqs (47b), (47f), (48b), (48f), (44) and the obtained boundary unknown densities into Eq (5) for the field solution of ηx<0, we have the infinite solution,
u(x)=((η0+ ηx)−∞∑n=12n(e−nη0enηx+e−nηxenη0+e−nη0−enηx) cos (nξx))k, −η0≤ηx<0. | (62) |
Similar substitution of Eqs (47c), (47e), (48c), (48e), (44) and the obtained boundary unknown densities into Eq (5), the field solution for ηx≥0 yields the infinite solution,
u(x)=((η0−ηx)−∞∑n=12n(e−nη0enηx+e−nηxenη0+e−nη0−e−nηx) cos (nξx))k, η0≥ηx≥0. | (63) |
Equations (62) and (63) also indicate symmetry.
Similarly, we consider the anti-symmetry problem. The coefficient of generalized Fourier bases in Eqs (45) and (49) satisfy
{−(2 ln (2c)−η0+4∑∞n=1e−2nη0n(1+e−2nη0))(al0+ar0)=0,1n(1+e−2nη0)(aln+arn)+4e−nη0(al0+ar0)=0, n=1, 2, 3...1n(1+e−2nη0)(bln+brn)=0, n=1, 2, 3... | (64) |
and
{2η0(al0−ar0)=4v,1n(−1+e−2nη0)(aln−arn)=0, n=1, 2, 3...,1n(−1+e−2nη0)(bln−brn)=0, n=1, 2, 3... | (65) |
We also find the discriminant, Db(c,η0) in Eq (64). If Db(c,η0)≠0, the geometry of the problem is an ordinary scale. Equations (64) and (65) yield the coefficients as shown below:
al0=−ar0=vη0aln=arn=0,n=1,2,3...bln=brn=0,n=1,2,3... | (66) |
Substituting Eqs (47b), (47f), (48b), (48f), (45) and the obtained boundary unknown densities into Eq (5) for the field solution of ηx<0, we have
u(x)=vηxη0, ηx < 0. | (67) |
Substituting Eqs (47c), (47e), (48c), (48e), (45) and the obtained unknown densities into Eq (5) for the field solution of ηx≥0, we also have
u(x)=vηxη0, ηx≥0. | (68) |
All potentials are shown in Figure 1(d). The solution in Eq (68) matches well with that of Lebedev et al. [8]. From the viewpoint of the MFS, this solution is the simplest one since only two sources with opposite strengths are required to locate the two foci.
If Db(c,η0)=0, a degenerate scale occurs. Fortunately, it doesn't result in no solution whether v is equal to zero or not as shown in the boundary condition of Eq (45). Equations (64) and (65) yield the coefficients as shown below:
al0+ar0=2k,aln=arn=4e−nη0(1+e−2nη0)k,n=1,2,3...bln=brn=0,n=1,2,3... | (69) |
where k is an arbitrary constant. For a degenerate scale case, η0 satisfies Db(c,η0)=0, i.e.
η0=2 ln (2c)+∑∞n=11n4e−2nη0(1+e−2nη0) | (70) |
Substituting Eqs (47b), (47f), (48b), (48f), (45) and the obtained boundary unknown densities into Eq (5) for the field solution of ηx<0, we have
u(x)=vη0ηx+k( ln (2 cosh ηx−2 cos ξx)−η0+2∑∞n=11ne−nη0 cosh (nηx) cosh (nη0) cos (nξx)), −η0≤ηx≤0. | (71) |
Similarly substituting Eqs (47c), (47e), (48c), (48e), (45) and the obtained unknown densities into Eq (5), we obtain the field solution
u(x)=vη0ηx+k(ln(2 cosh ηx−2 cos ξx)−η0+2∑∞n=11ne−nη0 cosh (nη0) cosh (nηx) cos (nξx)), η0≥ηx > 0. | (72) |
Equations (71) and (72) destroy the anti-symmetry due to the second part of k. To obey the anti-symmetry solution, k should be zero. In other words, this k part in the solution of Eqs (71) and (72) also disobey the bounded potential at infinity. This solution for a free constant, k, will be compared with that of Lekner [4] later.
According to the solution of Lekner [4], the general solution space of the symmetry problem in Eq (44) is expressed as follows:
u(x)=v+λud(x) | (73) |
where
ud(x)= ln (2 cosh ηx−2 cos ξx)−η0+∑∞n=12ne−nη0 cosh (nηx) cosh (nη0) cos (nξx). | (74) |
By using the identity equation,
ln ( cosh ηx− cos ξx)=ηx−∑∞m=12me−mηx cos m ξx− ln 2, | (75) |
the solution by using the direct BIE of Eq (59) is rewritten as
u(x)=vDb(c,η0)( ln (2 cosh ηx−2 cos ξx)+∑∞n=12ne−nη0 cosh (nηx) cosh (nη0) cos (nξx)−(2 ln (2c)+∑∞n=11n4e−2nη01+e−2nη0)),η0≥ηx > 0. | (76) |
Equation (59) is the special case of Eq (73), if λ=−vDb(c,η0). When the size of the boundary is a degenerate scale, i.e., Db(c,η0)=0, the BIE solution does not exist if v≠0. If v=0, the constant term in Eq (57), ar0, is a free constant. The electrostatic potential is obtained by
u(x) =−( ln (2 cosh ηx−2 cos ξx)−η0+∑∞n=12ne−nη0 cosh (nηx) cosh (nη0) cos (nξx))ar0, η0≥ηx > 0, | (77) |
and Eq (73) can be reduced to
u(x)=λud(x), | (78) |
since v is zero. It is easy to find that ar0 and λ are equivalent.
Similarly, the general solution space of the anti-symmetry problem in Eq (45) is expressed as follows:
u(x)=vηxη0+λud(x) | (79) |
where
ud(x)= ln (2 cosh ηx−2 cos ξx)−η0+∑∞n=12ne−nη0 cosh (nηx) cosh (nη0) cos (nξx). | (80) |
Lebedev et al. [8] considered the condition at the infinity, u(x)=0,x→∞, the solution of Eq (79) would reduce to only
u(x)=vηxη0. | (81) |
It is the reason why the solution of Eq (68) by using the BIEM is a special case of Eq (79) for λ=0. When the size of the boundary is a degenerate scale, i.e., Db(c,η0)=0, it yields infinite solutions. Since the sum of constant terms, ar0 and al0, in Eq (69) is a free constant, k, the electrostatic potential yields
u(x)=vηxη0−( ln (2 cosh ηx−2 cos ξx)−η0+2∑∞n=11ne−nη0 cosh (nη0) cosh (nηx) cos (nξx))k, η0≥ηx > 0. | (82) |
It is easy to find that k and λ are equivalent. To sum up, the free constant, λ and ud(x) in the general solution by Lekner [4] are similar to the constant term in the boundary potential and the obtained BIE solution for the degenerate scale by Chen et al. [9], respectively. The obtained BIE solution for the degenerate case yields nontrivial boundary flux even though the boundary potential is trivial. The generalized potential and the available solutions by using the BIEM for the problem containing two cylinders are compared with each other in Table 3.
![]() |
This paper investigates the solution space for the electrostatics of two cylinders using the BIEM. Both the symmetric and anti-symmetric cases are considered. Flux equilibrium on the cylindrical boundaries and the asymptotic behavior at infinity is also examined. Moreover, on the base of the Fredholm alternative theorem, the relation of unique solution and the degenerate scale in the BIEM is linked. The logarithmic capacity and the discriminant are also linked by using an exponential relation. Besides, the degenerate scale is also related. Not only two cylinders but also a single one (circle or ellipse) are considered. Finally, the results are compared with those derived by other researchers. Linkage and agreement are made.
The authors wish to thank the financial supports from the National Science and Technology Council, Taiwan under Grant No. MOST 111-2221-E-019-009-MY3 for National Taiwan Ocean University.
The authors declare there is no conflict of interest.
[1] |
A. Kumawat, D. Yadav, P. Srivastava, D. Kumar, Restoration of agroecosystems with conservation agriculture for food security to achieve sustainable development goals, Land Degrad. Dev., 2023 (2023), 1–9. https://doi.org/10.1002/ldr.4677 doi: 10.1002/ldr.4677
![]() |
[2] |
S. Ullah, A. Ali, M. Iqbal, Z. A. Kazmi, M. Sodangi, R. F. Tufail, et al., Geospatial assessment of soil erosion intensity and sediment yield: a case study of Potohar Region, Environ. Earth Sci., 77 (2018), 705. https://doi.org/10.1007/s12665-018-7867-7 doi: 10.1007/s12665-018-7867-7
![]() |
[3] |
D. Pimentel, Soil erosion: A food and environmental threat, Environ. Dev. Sustain., 8 (2006), 119–137. https://doi.org/10.1007/s10668-005-1262-8 doi: 10.1007/s10668-005-1262-8
![]() |
[4] | M. Ashraf, K. Fayyaz-ul-Hussan, Sustainable environment management: impact of Agriculture, Sci. Technol. Dev., 19 (2000), 51–57. |
[5] |
P. R. Hobbs, Conservation agriculture: what is it and why is it important for future sustainable food production?, J. Agric. Sci., 145 (2007), 127. https://doi.org/10.1017/S0021859607006892 doi: 10.1017/S0021859607006892
![]() |
[6] | Z. Shah, M. Arshad, Land degradation in Pakistan: a serious threat to environments and economic sustainability, 2006 (2006). |
[7] |
S. A. Khresat, Z. Rawajfih, M. Mohammad, Land degradation in north-western Jordan: causes and processes, J. Arid. Environ., 39 (1998), 623–629. https://doi.org/10.1006/jare.1998.0385 doi: 10.1006/jare.1998.0385
![]() |
[8] | O. A. Abdi, E. K. Glover, O. Luukkanen, Causes and impacts of land degradation and desertification: Case study of the Sudan, Int. J. A gricul., 3 (2013), 40–51. |
[9] |
H. Eswaran, R. Lal, P. F. Reich, Land degradation: an overview, Resp. Degrad., 2001 (2001), 20–35. https://doi.org/10.1201/9780429187957-4 doi: 10.1201/9780429187957-4
![]() |
[10] |
R. Lal, Restoring soil quality to mitigate soil degradation, Sustainability, 7 (2015), 5875–5895. https://doi.org/10.3390/su7055875 doi: 10.3390/su7055875
![]() |
[11] |
R. Lal, Soil erosion and the global carbon budget, Environ. Int., 29 (2003), 437–450. https://doi.org/10.1016/S0160-4120(02)00192-7 doi: 10.1016/S0160-4120(02)00192-7
![]() |
[12] | M. Ashraf, F. U. Hassan, A. Saleem, M. M. Iqbal, Soil conservation and management: A prerequisite for sustainable agriculture in pothwar, Sci. Technol. Dev., 21 (2002), 25–31. |
[13] |
D. Pimentel, M. Burgess, Soil erosion threatens food production, Agriculture, 3 (2013), 443–463. https://doi.org/10.3390/agriculture3030443 doi: 10.3390/agriculture3030443
![]() |
[14] |
H. Aytop, S. Şenol, The effect of different land use planning scenarios on the amount of total soil losses in the Mikail Stream Micro-Basin, Environ. Monit. Assess, 194 (2022), 321. https://doi.org/10.1007/s10661-022-09937-2 doi: 10.1007/s10661-022-09937-2
![]() |
[15] | L. Tsegaye, R. Bharti, Assessment of the effects of agricultural management practices on soil erosion and sediment yield in Rib watershed, Ethiopia, Int. J. Environ. Sci. Technol., 2022 (2022). https://doi.org/10.1007/s13762-022-04018-w |
[16] | D. Mandal, V. N. Sharda, Assessment of permissible soil loss in India employing a quantitative bio-physical model, Curr. Sci., 100 (2011), 383–390. |
[17] | S. Kumar, J. G. Kalambukattu, Modeling and Monitoring Soil Erosion by Water Using Remote Sensing Satellite Data and GIS BT-Anthropogeomorphology: A Geospatial Technology Based Approach, Cham, Springer International Publishing, 2022. https://doi.org/10.1007/978-3-030-77572-8_14 |
[18] | T. Svoray, The Case of Agricultural Catchments BT-A Geoinformatics Approach to Water Erosion: Soil Loss and Beyond, Cham, Springer International Publishing, 2022. https://doi.org/10.1007/978-3-030-91536-0 |
[19] |
Y. Su, Y. Zhang, H. Wang, N. Lei, P. Li, J. Wang, Interactive effects of rainfall intensity and initial thaw depth on slope erosion, Sustainability, 14 (2022), 3172. https://doi.org/10.3390/su14063172 doi: 10.3390/su14063172
![]() |
[20] | Y. Li, X. Lu, R. A. Washington-Allen, Y. Li, Microtopographic controls on erosion and deposition of a rilled hillslope in eastern tennessee, USA, Remote Sens., 14 (2022), 1315. https://doi.org/10.3390/rs14061315 |
[21] | W. H. Wischmeier, D. D. Smith, Predicting rainfall-erosion losses from cropland east of the Rocky Mountains: Guide for selection of practices for soil and water conservation, in Agricultural Research Service, US Department of Agriculture, (1965). |
[22] | R. Cruse, D. Flanagan, J. Frankenberger, B. Gelder, D. Herzmann, D. James, et al., Daily estimates of rainfall, water runoff, and soil erosion in Iowa, J. Soil Water Conserv., 61 (2006), 191–199. |
[23] | V. Prasannakumar, H. Vijith, S. Abinod, N. Geetha, Estimation of soil erosion risk within a small mountainous sub-watershed in Kerala, India, using Revised Universal Soil Loss Equation (RUSLE) and geo-information technology, Geosci. Front., 3 (2012), 209–215. https://doi.org/10.1016/j.gsf.2011.11.003 |
[24] |
N. Kayet, K. Pathak, A. Chakrabarty, S. Sahoo, Evaluation of soil loss estimation using the RUSLE model and SCS-CN method in hillslope mining areas, Int. Soil Water Conserv. Res., 6 (2018), 31–42. https://doi.org/10.1016/j.iswcr.2017.11.002 doi: 10.1016/j.iswcr.2017.11.002
![]() |
[25] |
R. B. Bryan, Soil erodibility and processes of water erosion on hillslope, Geomorphology, 32 (2000), 385–415. https://doi.org/10.1016/S0169-555X(99)00105-1 doi: 10.1016/S0169-555X(99)00105-1
![]() |
[26] |
H. Aksoy, M. L. Kavvas, A review of hillslope and watershed scale erosion and sediment transport models. CATENA, 64 (2005), 247–271. https://doi.org/10.1016/j.catena.2005.08.008 doi: 10.1016/j.catena.2005.08.008
![]() |
[27] |
M. Mahmoodabadi, S. A. Sajjadi, Effects of rain intensity, slope gradient and particle size distribution on the relative contributions of splash and wash loads to rain-induced erosion, Geomorphology, 253 (2016), 159–167. https://doi.org/10.1016/j.geomorph.2015.10.010 doi: 10.1016/j.geomorph.2015.10.010
![]() |
[28] |
J. Lu, F. Zheng, G. Li, F. Bian, J. An, The effects of raindrop impact and runoff detachment on hillslope soil erosion and soil aggregate loss in the Mollisol region of Northeast China, Soil Tillage Res., 161 (2016), 79–85. https://doi.org/10.1016/j.still.2016.04.002 doi: 10.1016/j.still.2016.04.002
![]() |
[29] |
L. D. Meyer, W. H. Wischmeier, Mathematical simulation of the process of soil erosion by water, Trans. ASAE, 12 (1969), 754–758. https://doi.org/10.13031/2013.38945 doi: 10.13031/2013.38945
![]() |
[30] |
G. R. Foster, R. A. Young, M. J. M. Römkens, C. A. Onstad, Processes of soil erosion by water. Soil Eros Crop. Prod., 1985 (1985), 137–162. https://doi.org/10.2134/1985.soilerosionandcrop.c9 doi: 10.2134/1985.soilerosionandcrop.c9
![]() |
[31] |
T. Smets, J. Poesen, R. Bhattacharyya, M. A. Fullen, M. Subedi, C. A. Booth, et al., Evaluation of biological geotextiles for reducing runoff and soil loss under various environmental conditions using laboratory and field plot data, Land Degrad. Dev., 22 (2011), 480–494. https://doi.org/10.1002/ldr.1095 doi: 10.1002/ldr.1095
![]() |
[32] | L. Ferreras, E. Gomez, S. Toresani, I. Firpo, R. Rotondo, Effect of organic amendments on some physical, chemical and biological properties in a horticultural soil, Bioresour. Technol., 97 (2006), 635–640. https://doi.org/10.1016/j.biortech.2005.03.018 |
[33] |
K. S. Rawat, S. K. Singh, Appraisal of soil conservation capacity using NDVI Model-based c factor of RUSLE Model for a semi arid ungauged watershed: a case study, Water Conserv. Sci. Eng., 3 (2018), 47–58. https://doi.org/10.1007/s41101-018-0042-x doi: 10.1007/s41101-018-0042-x
![]() |
[34] |
P. Zhou, O. Luukkanen, T. Tokola, J. Nieminen, Effect of vegetation cover on soil erosion in a mountainous watershed, CATENA, 75 (2008), 319–325. https://doi.org/10.1016/j.catena.2008.07.010 doi: 10.1016/j.catena.2008.07.010
![]() |
[35] | J. D. R. Sinoga, A. R. Diaz, F. E. Bueno, J. F. M. Murillo, The role of soil surface conditions in regulating runoff and erosion processes on a metamorphic hillslope (Southern Spain): Soil surface conditions, runoff and erosion in Southern Spain, CATENA, 80 (2010), 131–139. https://doi.org/10.1016/j.catena.2009.09.007 |
[36] | L. Gholami, S. H. Sadeghi, M. Homaee, Straw mulching effect on splash erosion, runoff, and sediment yield from eroded plots, Soil Sci. Soc. Am. J., 77 (2013), 268–278. https://doi.org/10.2136/sssaj2012.0271 |
[37] |
S. H. R. Sadeghi, L. Gholami, E. Sharifi, A. K. Darvishan, M. Homaee, Scale effect on runoff and soil loss control using rice straw mulch under laboratory conditions, Solid. Earth, 6 (2015), 1–8. https://doi.org/10.5194/se-6-1-2015 doi: 10.5194/se-6-1-2015
![]() |
[38] |
T. Smets, J. Poesen, A. Knapen, Spatial scale effects on the effectiveness of organic mulches in reducing soil erosion by water, Earth Sci. Rev., 89 (2008), 1–12. https://doi.org/10.1016/j.earscirev.2008.04.001 doi: 10.1016/j.earscirev.2008.04.001
![]() |
[39] |
N. K. Fageria, Role of soil organic matter in maintaining sustainability of cropping systems, Commun. Soil Sci. Plant Anal., 43 (2012), 2063–2113. https://doi.org/10.1080/00103624.2012.697234 doi: 10.1080/00103624.2012.697234
![]() |
[40] |
S. E. Obalum, G. U. Chibuike, S. Y. Peth, Ouyang, Soil organic matter as sole indicator of soil degradation, Environ. Monit. Assess., 189 (2017), 176. https://doi.org/10.1007/s10661-017-5881-y doi: 10.1007/s10661-017-5881-y
![]() |
[41] |
Z. H. Shi, B. J. Yue, L. Wang, N. F. Fang, D. Wang, F. Z. Wu, Effects of mulch cover rate on interrill erosion processes and the size selectivity of eroded sediment on steep slopes, Soil Sci. Soc. Am. J., 77 (2013), 257–267. https://doi.org/10.2136/sssaj2012.0273 doi: 10.2136/sssaj2012.0273
![]() |
[42] |
T. Guo, Q. Wang, D. Li, J. Zhuang, Effect of surface stone cover on sediment and solute transport on the slope of fallow land in the semi-arid loess region of northwestern China, J. Soils Sediments, 10 (2010), 1200–1208. https://doi.org/10.1007/s11368-010-0257-8 doi: 10.1007/s11368-010-0257-8
![]() |
[43] |
M. Prosdocimi, A. Jordán, P. Tarolli, S. Keesstra, A. Novara, A. Cerdà, The immediate effectiveness of barley straw mulch in reducing soil erodibility and surface runoff generation in Mediterranean vineyards, Sci. Total Environ., 547 (2016), 323–330. https://doi.org/10.1016/j.scitotenv.2015.12.076 doi: 10.1016/j.scitotenv.2015.12.076
![]() |
[44] |
A. A. A. Montenegro, J. R. C. B. Abrantes, J. L. M. P. de Lima, V. P. Singh, T. E. M. Santos, Impact of mulching on soil and water dynamics under intermittent simulated rainfall, CATENA., 109 (2013), 139–149. https://doi.org/10.1016/j.catena.2013.03.018 doi: 10.1016/j.catena.2013.03.018
![]() |
[45] | A. D. Abrahams, A. J. Parsons, Relation between infiltration and stone cover on a semiarid hillslope, southern Arizona, J. Hydrol., 122 (1991), 49–59. https://doi.org/10.1016/0022-1694(91)90171-D |
[46] |
C. Valentin, A. Casenave, Infiltration into sealed soils as influenced by gravel cover, Soil Sci. Soc. Am. J., 56 (1992), 1667–1673. https://doi.org/10.2136/sssaj1992.03615995005600060002x doi: 10.2136/sssaj1992.03615995005600060002x
![]() |
[47] |
J. W. Poesen, D. Torri, K. Bunte, Effects of rock fragments on soil erosion by water at different spatial scales: a review, CATENA, 23 (1994), 141–166. https://doi.org/10.1016/0341-8162(94)90058-2 doi: 10.1016/0341-8162(94)90058-2
![]() |
[48] | A. Cerdà, Effects of rock fragment cover on soil infiltration, interrill runoff and erosion, Eur. J. Soil Sci., 52 (2001), 59–68. https://doi.org/10.1046/j.1365-2389.2001.00354.x |
[49] |
L. M. Zavala, A. Jordán, N. Bellinfante, J. Gill, Relationships between rock fragment cover and soil hydrological response in a Mediterranean environment, Soil Sci. Plant Nutr., 56 (2010), 95–104. https://doi.org/10.1111/j.1747-0765.2009.00429.x doi: 10.1111/j.1747-0765.2009.00429.x
![]() |
[50] |
R. Akis, R. Lal, Evaluation of seasonal effects of tillage and drainage management practices on soil physical properties and infiltration characteristics in a silt-loam soil, Eur. J. Sci. Technol., 2022 (2022), 1011–1023. https://doi.org/10.31590/ejosat.1050860 doi: 10.31590/ejosat.1050860
![]() |
[51] |
Molla A, Desta G, Molla GA, Soil management and crop practice effect on soil water infiltration and soil water storage in the humid Lowlands of Beles Sub-Basin, Ethiopia, Hydrology, 10 (2022), 1–11. https://doi.org/10.11648/j.hyd.20221001.11 doi: 10.11648/j.hyd.20221001.11
![]() |
[52] |
K. O. Adekalu, I. A. Olorunfemi, J. A. Osunbitan, Grass mulching effect on infiltration, surface runoff and soil loss of three agricultural soils in Nigeria, Bioresour. Technol., 98 (2007), 912–917. https://doi.org/10.1016/j.biortech.2006.02.044 doi: 10.1016/j.biortech.2006.02.044
![]() |
[53] |
C. Pan, Z. Shangguan, Runoff hydraulic characteristics and sediment generation in sloped grassplots under simulated rainfall conditions, J. Hydrol., 331 (2006), 178–185. https://doi.org/10.1016/j.jhydrol.2006.05.011 doi: 10.1016/j.jhydrol.2006.05.011
![]() |
[54] |
C. Pan, L. Ma, Z. Shangguan, Effectiveness of grass strips in trapping suspended sediments from runoff, Earth Surf. Process Landforms, 35 (2010), 1006–1013. https://doi.org/10.1002/esp.1997 doi: 10.1002/esp.1997
![]() |
[55] |
R. Bhattacharyya, T. Smets, M. A. Fullen, J. Poesen, C.A. Booth, Effectiveness of geotextiles in reducing runoff and soil loss: A synthesis, CATENA, 81 (2010), 184–195. https://doi.org/10.1016/j.catena.2010.03.003 doi: 10.1016/j.catena.2010.03.003
![]() |
[56] |
A. Cerdà, S. H. Doerr. The effect of ash and needle cover on surface runoff and erosion in the immediate post-fire period, CATENA., 74 (2008), 256–263. https://doi.org/10.1016/j.catena.2008.03.010 doi: 10.1016/j.catena.2008.03.010
![]() |
[57] |
P. R. Robichaud, S. A. Lewis, J. W. Wagenbrenner, L. E. Ashmun, R. E. Brown, Post-fire mulching for runoff and erosion mitigation: Part I: Effectiveness at reducing hillslope erosion rates, CATENA, 105 (2013), 75–92. https://doi.org/10.1016/j.catena.2012.11.015 doi: 10.1016/j.catena.2012.11.015
![]() |
[58] |
P. R. Robichaud, J. W. Wagenbrenner, S. A. Lewis, L. E. Ashmun, R. E. Brown, P. M. Wohlgemuth, Post-fire mulching for runoff and erosion mitigation Part Ⅱ: Effectiveness in reducing runoff and sediment yields from small catchments, CATENA, 105 (2013), 93–111. https://doi.org/10.1016/j.catena.2012.11.016 doi: 10.1016/j.catena.2012.11.016
![]() |
[59] |
T. H. Dao, Tillage and winter wheat residue management effects on water infiltration and storage, Soil Sci. Soc. Am. J., 57 (1993), 1586–1595. https://doi.org/10.2136/sssaj1993.03615995005700060032x doi: 10.2136/sssaj1993.03615995005700060032x
![]() |
[60] |
P. Govindasamy, J. Mowrer, N. Rajan, T. Provin, F. Hons, M. Bagavathiannan, Influence of long-term (36 years) tillage practices on soil physical properties in a grain sorghum experiment in Southeast Texas, Arch. Agron. Soil Sci., 67 (2021), 234–244. https://doi.org/10.1080/03650340.2020.1720914 doi: 10.1080/03650340.2020.1720914
![]() |
[61] |
Y. Wang, J. Qiao, W. Ji, J. Sun, D. Huo, Y. Liu, et al., Effects of crop residue managements and tillage practices on variations of soil penetration resistance in sloping farmland of Mollisols, Int. J. Agric. Biol. Eng., 14 (2021), 164–171. https://doi.org/10.25165/j.ijabe.20221501.6526 doi: 10.25165/j.ijabe.20221501.6526
![]() |
[62] |
L. Benkobi, M. J. Trlica, J. L. Smith, Soil loss as affected by different combinations of surface litter and rock, J. Environ. Qual., 22 (1993), 657–661. https://doi.org/10.2134/jeq1993.00472425002200040003x doi: 10.2134/jeq1993.00472425002200040003x
![]() |
[63] |
H. J. Schmalz, R. V. Taylor, T. N. Johnson, P. L. Kennedy, S. J. DeBano, B. A. Newingham, et al., Soil morphologic properties and cattle stocking rate affect dynamic soil properties, Rangel. Ecol. Manage., 66 (2013), 445–453. https://doi.org/10.2111/REM-D-12-00040.1 doi: 10.2111/REM-D-12-00040.1
![]() |
[64] |
X. Li, J. Niu, B. Xie, The effect of leaf litter cover on surface runoff and soil erosion in Northern China, PLoS One, 9 (2014), e107789. https://doi.org/10.1371/journal.pone.0107789 doi: 10.1371/journal.pone.0107789
![]() |
[65] |
M. A. Weltz, M. R. Kidwell, H. D. Fox, Influence of abiotic and biotic factors in measuring and modeling soil erosion on rangelands: State of knowledge, J. Range Manage., 51 (1998), 482–495. https://doi.org/10.2307/4003363 doi: 10.2307/4003363
![]() |
[66] |
Y. Xin, Y. Xie, Y. Liu, X. Ren, Residue cover effects on soil erosion and the infiltration in black soil under simulated rainfall experiments, J. Hydrol., 543 (2016), 651–658. https://doi.org/10.1016/j.jhydrol.2016.10.036 doi: 10.1016/j.jhydrol.2016.10.036
![]() |
[67] | F. L. Engel, I. Bertol, S. R. Ritter, A. P. González, J. P. Ferreiro, E. Vidal Vázquez, Soil erosion under simulated rainfall in relation to phenological stages of soybeans and tillage methods in Lages, SC, Brazil, Soil Tillage Res., 103 (2009), 216–221. https://doi.org/10.1016/j.still.2008.05.017 |
[68] |
J. R. C. B. Abrantes, S. A. Prats, J. J. Keizer, J. L. M. P. de Lima, Effectiveness of the application of rice straw mulching strips in reducing runoff and soil loss: Laboratory soil flume experiments under simulated rainfall, Soil Tillage Res., 180 (2018), 238–249. https://doi.org/10.1016/j.still.2018.03.015 doi: 10.1016/j.still.2018.03.015
![]() |
[69] |
D. R. Mailapalli, M. Burger, W. R. Horwath, W. W. Wallender, (2013) Crop residue biomass effects on agricultural runoff, Appl. Environ. Soil Sci., 2013 (2013), 1–8. https://doi.org/10.1155/2013/805206 doi: 10.1155/2013/805206
![]() |
[70] |
X. Liu, S. Zhang, X. Zhang, G. Ding, R. M. Cruse, Soil erosion control practices in Northeast China: A mini-review, Soil Tillage Res., 117 (2011), 44–48. https://doi.org/10.1016/j.still.2011.08.005 doi: 10.1016/j.still.2011.08.005
![]() |
[71] |
J. H. Zonta, M. A. Martinez, F. F. Pruski, D. D. Silva, M. R. Santos, Effect of successive rainfall with different patterns on soil water infiltration rate, Rev. Bras Ciência Solo, 36 (2012), 377–388. https://doi.org/10.1590/S0100-06832012000200007 doi: 10.1590/S0100-06832012000200007
![]() |
[72] |
S. A. Prats, J. R. Abrantes, I. P. Crema, J. J. Keizer, J. L. M. P. Lima, Runoff and soil erosion mitigation with sieved forest residue mulch strips under controlled laboratory conditions, Forest Ecol. Manage., 396 (2017), 102–112. https://doi.org/10.1016/j.foreco.2017.04.019 doi: 10.1016/j.foreco.2017.04.019
![]() |
[73] | S. A. Prats, M. A. Martins, M. C. Malvar, M. Ben-Hur, J. J. Keizer, Polyacrylamide application versus forest residue mulching for reducing post-fire runoff and soil erosion, Sci. Total Environ., 468–469 (2014), 464–474. https://doi.org/10.1016/j.scitotenv.2013.08.066 |
[74] |
S. A. Prats, M. C. Malvar, D. C. S. Vieira, L. MacDonald, J. J. Keizer, Effectiveness of hydromulching to reduce runoff and erosion in a recently burnt pine plantation in central portugal, Land Degrad. Dev., 27 (2013), 1319–1333. https://doi.org/10.1002/ldr.2236 doi: 10.1002/ldr.2236
![]() |
[75] |
S. A. Prats, L. H. MacDonald, M. Monteiro, A. J. D. Ferreira, C. O. A. Coelho, J. J. Keizer, Effectiveness of forest residue mulching in reducing post-fire runoff and erosion in a pine and a eucalypt plantation in north-central Portugal, Geoderma, 191 (2012), 115–124. https://doi.org/10.1016/j.geoderma.2012.02.009 doi: 10.1016/j.geoderma.2012.02.009
![]() |
1. | Jeng-Tzong Chen, Wei-Chen Tai, Ying-Te Lee, Shing-Kai Kao, An analytical Green’s function for Laplace operator in an infinite plane with two circular holes using degenerate kernels, 2023, 146, 08939659, 108774, 10.1016/j.aml.2023.108774 |
![]() |
![]() |
![]() |