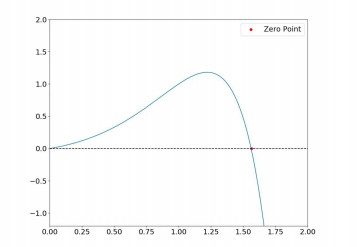
A novel treatment of fractional-time derivative using the incompressible smoothed particle hydrodynamics (ISPH) method is introduced to simulate the bioconvection flow of nano-enhanced phase change materials (NEPCM) in a porous hexagonal cavity. The fractional-time derivative is based on the Caputo style, which reflects the fractional order behavior in complex systems. In this work, the circular rotation of the embedded four-pointed star and the motion of oxytactic microorganisms in a hexagonal cavity are conducted. Due to the significance of fractional derivatives in handling real physical problems with more flexibility than conventional derivatives, the present scheme of the ISPH method is developed to solve the fractional-time derivative of the bioconvection flow in a porous hexagonal cavity. This study implicates the variations of a fractional-time derivative, a parametric of an inner four-pointed star, and the pertinent physical parameters on the behavior of a bioconvection flow of a nanofluid in a hexagonal-cavity containing oxytactic microorganisms. The presence of microorganisms has a significant role in many biological, engineering, and medical phenomena. From the present numerical investigation, it is well mentioned that the computational time of the transient processes can be reduced by applying a fractional-time derivative. The variable sizes of an inner four-pointed star enhance the bioconvection flow in a hexagonal cavity.
Citation: Abdelraheem M. Aly, Abd-Allah Hyder. Fractional-time derivative in ISPH method to simulate bioconvection flow of a rotated star in a hexagonal porous cavity[J]. AIMS Mathematics, 2023, 8(12): 31050-31069. doi: 10.3934/math.20231589
[1] | Chang Liu, Jianping Li . Sharp bounds on the zeroth-order general Randić index of trees in terms of domination number. AIMS Mathematics, 2022, 7(2): 2529-2542. doi: 10.3934/math.2022142 |
[2] | Swathi Shetty, B. R. Rakshith, N. V. Sayinath Udupa . Extremal graphs and bounds for general Gutman index. AIMS Mathematics, 2024, 9(11): 30454-30471. doi: 10.3934/math.20241470 |
[3] | Fan Wu, Xinhui An, Baoyindureng Wu . Sombor indices of cacti. AIMS Mathematics, 2023, 8(1): 1550-1565. doi: 10.3934/math.2023078 |
[4] | Edil D. Molina, José M. Rodríguez-García, José M. Sigarreta, Sergio J. Torralbas Fitz . On the Gutman-Milovanović index and chemical applications. AIMS Mathematics, 2025, 10(2): 1998-2020. doi: 10.3934/math.2025094 |
[5] | Damchaa Adiyanyam, Enkhbayar Azjargal, Lkhagva Buyantogtokh . Bond incident degree indices of stepwise irregular graphs. AIMS Mathematics, 2022, 7(5): 8685-8700. doi: 10.3934/math.2022485 |
[6] | Tariq A. Alraqad, Igor Ž. Milovanović, Hicham Saber, Akbar Ali, Jaya P. Mazorodze, Adel A. Attiya . Minimum atom-bond sum-connectivity index of trees with a fixed order and/or number of pendent vertices. AIMS Mathematics, 2024, 9(2): 3707-3721. doi: 10.3934/math.2024182 |
[7] | Zhenhua Su, Zikai Tang, Hanyuan Deng . Higher-order Randić index and isomorphism of double starlike trees. AIMS Mathematics, 2023, 8(12): 31186-31197. doi: 10.3934/math.20231596 |
[8] | Muhammad Kamran Jamil, Muhammad Imran, Aisha Javed, Roslan Hasni . On the first general Zagreb eccentricity index. AIMS Mathematics, 2021, 6(1): 532-542. doi: 10.3934/math.2021032 |
[9] | Zongrong Qin, Dingjun Lou . The k-subconnectedness of planar graphs. AIMS Mathematics, 2021, 6(6): 5762-5771. doi: 10.3934/math.2021340 |
[10] | Hongzhuan Wang, Xianhao Shi, Ber-Lin Yu . On the eccentric connectivity coindex in graphs. AIMS Mathematics, 2022, 7(1): 651-666. doi: 10.3934/math.2022041 |
A novel treatment of fractional-time derivative using the incompressible smoothed particle hydrodynamics (ISPH) method is introduced to simulate the bioconvection flow of nano-enhanced phase change materials (NEPCM) in a porous hexagonal cavity. The fractional-time derivative is based on the Caputo style, which reflects the fractional order behavior in complex systems. In this work, the circular rotation of the embedded four-pointed star and the motion of oxytactic microorganisms in a hexagonal cavity are conducted. Due to the significance of fractional derivatives in handling real physical problems with more flexibility than conventional derivatives, the present scheme of the ISPH method is developed to solve the fractional-time derivative of the bioconvection flow in a porous hexagonal cavity. This study implicates the variations of a fractional-time derivative, a parametric of an inner four-pointed star, and the pertinent physical parameters on the behavior of a bioconvection flow of a nanofluid in a hexagonal-cavity containing oxytactic microorganisms. The presence of microorganisms has a significant role in many biological, engineering, and medical phenomena. From the present numerical investigation, it is well mentioned that the computational time of the transient processes can be reduced by applying a fractional-time derivative. The variable sizes of an inner four-pointed star enhance the bioconvection flow in a hexagonal cavity.
We only consider finite and undirected graphs throughout this paper. Let G=(V(G),E(G)) be a graph with n=|V(G)| vertices and m=|E(G)| edges. For any vertex u∈V(G), we use dG(u) (or du when no confusion can arise) to denote the degree of u in G, which is the number of edges incident to u. Such a maximal number (resp. minimal number) is called the maximal degree Δ(G) (resp. minimal degree δ(G)). For any vertex u in G, we use NG(u) to denote the set of all vertices adjacent with u, and the elements of NG(u) are called neighbors of u. A sequence of positive integers π=(d1,d2,…,dn) is called the degree sequence of G if di=dvi for any vertex vi∈V(G), where i=1,2,…,n.
The join of two graphs G1 and G2, denoted by G1+G2, is the graph with the vertex set V(G1)∪V(G2) and edge set E(G1)∪E(G2)∪{xy|x∈V(G1),y∈V(G2)}. The cyclomatic number of G is the minimum number of edges in it whose removal makes it acyclic, denoted by γ=γ(G). Let Gγn be the set of n-vertex graphs with cyclomatic number γ. We use Kn and Pn to denote the complete graph and path of n vertices, respectively. As usual, we use the symbol ℓ(Pn) to denote the length of the path Pn, which equals to the number of edges in Pn. The cyclomatic number, denoted by γ, of a graph G is the minimum number of edges of G whose removal makes G acyclic. Let Gγn be the class of all connected graphs with order n and cyclomatic number γ. We use [4] for terminology and notation not defined here.
The topological index is a real number that can be used to characterize the properties of the molecule graph. Nowadays, hundreds of topological indices have been considered and used in quantitative structure-activity and quantitative structure-property relationships. One of the well-known topological indices is the general Randić index, which was defined by Bollobás and Erdös [5] and Amic [1] independently:
Rα(G)=∑uv∈E(G)[dudv]α, |
where α is a nonzero real number. This topological index has been extensively investigated. We encourage interested readers to consult [3,6,7,10,11,13] for more mathematical properties and their applications.
Even though the mathematical and chemical theory of the general Randić index has been well considered, some extremal graph-theoretical problems concerning this graph invariant are still open. In this paper, we focus on exploring the extremal graphs in Gγn with respect to the general Randić index.
It is interesting to explore the extremal graphs for some topological indices in the class of graphs with a given cyclomatic number. In this section, we focus on determining the extremal graphs in Gγn with the minimum general Randić index. Before proceeding, we shall prove or list several facts as preliminaries.
Lemma 2.1. The function P(x,α)=2αxα+1−(x−1)α[2α(x−2)+3α]+xα(2α−3α)−6α>0 for x≥4 and 1≤α≤3925.
Proof. It is routine to check that
P(x,α)=2αxα+1−(x−1)α[2α(x−2)+3α]+xα(2α−3α)−6α=2αxα+1−(x−1)α[2α(x−1)−2α+3α]+xα(2α−3α)−6α=2α[xα+1−(x−1)α+1]+(2α−3α)(x−1)α+(2α−3α)xα−6α=2α[xα+1−(x−1)α+1]+(2α−3α)[xα+(x−1)α]−6α=2α[xα+(x−1)α]+2αx(x−1)[xα−1−(x−1)α−1]+(2α−3α)[xα+(x−1)α]−6α=(2⋅2α−3α)[xα−(x−1)α]+2αx(x−1)[xα−1−(x−1)α−1]−6α. |
Note that ρ(t)=tα−(t−1)α is an increasing function for t∈[4,+∞), and 2⋅2α>3α if, and only if, α<ln2ln3−ln2≈1.709, then we have
P(x,α)=(2⋅2α−3α)[xα−(x−1)α]+2αx(x−1)[xα−1−(x−1)α−1]−6α≥(2⋅2α−3α)(4α+3α)+2α⋅12⋅(4α−1−3α−1)−6α=5⋅8α−3⋅6α−9α−12α. |
For simplicity, let H(α)=5⋅8α−3⋅6α−9α−12α. To continue our proof, we first verify the following fact.
Claim 1. The function ϱ(t)=k1at−k2bt−k3ct has a unique zero point in the interval [0,+∞) for any positive real numbers k1,k2,k3,a,b,c such that k1−k2−k3>0 and 1<a<b<c.
Proof of Claim 1. It is routine to check that ϱ′(t)=k1lna⋅at−k2lnb⋅bt−k3lnc⋅ct. Note that ϱ(0)=k1−k2−k3>0 and ϱ(M)=at[k1−k2(ba)t−k3(ca)t]t=M→−∞, and it follows that ϱ(t) has zero points in the interval [0,+∞). Without loss of generality, we assume that t1,t2=t1+h∈[0,+∞) are the two distinct zero points of ϱ(t) for h>0, which is equivalent to k1at1−k2bt1−k3ct1=0 and k1at2−k2bt2−k3ct2=0. Besides, we know that ϱ′(t)=k1lna⋅at−k2lnb⋅bt−k3lnc⋅ct, which implies that
ϱ′(t1)=k1lna⋅at1−k2lnb⋅bt1−k3lnc⋅ct1<lna(k1at1−k2bt1−k3ct1)=0. |
In addition, we have
ϱ(t2)=ϱ(t1+h)=k1at1ah−k2bt1bh−k3ct1ch=(k2bt1+k3ct1)ah−k2bt1bh−k3ct1ch<(k2bt1+k3ct1)ah−(k2bt1+k3ct1)bh=(k2bt1+k3ct1)(ah−bh)<0, |
which contradicts to the fact that ϱ(t2)=0. Hence, there must exist a unique number t0∈[0,+∞) such that ϱ(t0)=0. As desired, we have completed the proof of Claim 1.
Claim 2. The function H(α)=5⋅8α−3⋅6α−9α−12α has a unique zero point in the interval (1,2).
Proof of Claim 2. It is routine to check that H′(α)=5ln8⋅8α−3ln6⋅6α−ln9⋅9α−ln12⋅12α. Note that H(1)=1>0 and H(2)=−13<0, and it follows that H(α) has zero points in the interval (1,2). Without loss of generality, we assume that α0,α1,…,αl are the zero points of H(α) such that 1<α0<α1<…<αl. Hence, H(α0)=5⋅8α0−3⋅6α0−9α0−12α0=0. Furthermore,
H′(α0)=5ln8⋅8α0−3ln6⋅6α0−ln9⋅9α0−ln12⋅12α0=ln8(3⋅6α0+9α0+12α0)−3ln6⋅6α0−ln9⋅9α0−ln12⋅12α0=3(ln8−ln6)⏟k16α0−(ln9−ln8)⏟k29α0−(ln12−ln8)⏟k312α0. |
It follows from Claim 1 that ϱ(t)|a=6,b=9,c=12 has a unique zero point in the interval t0∈[0,+∞). Consequently, we know that the unique zero point of ϱ(t)|a=6,b=9,c=12 must lie in the interval (0,1) since ϱ(0)|a=6,b=9,c=12>0 and ϱ(1)|a=6,b=9,c=12=−0.7473<0. Hence, ϱ(t)|a=6,b=9,c=12<0 always holds for any real number t≥1. This implies that H′(αi)=ϱ(αi)|a=6,b=9,c=12<0 for αi>1 and i=0,1,…,l, which contradicts to the continuity of the function H(α). As desired, we have completed the proof of Claim 2.
Now, we continue to our proof. Note that H(3925)=5⋅83925−3⋅63925−93925−123925≈0.01857>0 and H(1.57)=5⋅81.57−3⋅61.57−91.57−121.57≈−0.07428<0. Hence, P(x,α)≥H(α)>0 for α∈[1,3925]. As desired, we have completed the proof of Lemma 2.1.
Lemma 2.2. The function Q(x,α)=3α(xα+1−(x−1)α+1)−9α+2xα(2α−3α)>0 for x≥4 and α≥1.
Proof. For simplicity, we distinguish the following two cases.
Case 1. α∈[1,3).
Note that h(t)=tα is an increasing function in the interval [1−1x,1] for α≥1, and it follows from Lagrange's mean value formula that h(1)−h(1−1x)=[1−(1−1x)]h′(ξ)=1xh′(ξ)=1xαξα−1>0, where ξ∈(1−1x,1). Hence, x[1−(1−1x)α]=x[h(1)−h(1−1x)]=αξα−1. Thus, we have
Qx(x,α)=3α(α+1)[xα−(x−1)α]+2αxα−1(2α−3α)=xα−1{3α(α+1)x[1−(1−1x)α]+2α(2α−3α)}=xα−1[3αα(α+1)ξα−1+2α(2α−3α)]=αxα−1[3α(α+1)ξα−1+2(2α−3α)]. |
By our initial hypothesis, it is routine to check that ξα−1>(1−1x)α−1, then we have
Qx(x,α)>αxα−1[3α(α+1)(1−1x)α−1+2(2α−3α)]>αxα−1[3α(α+1)(34)α−1+2(2α−3α)](because1−1x>34)=2α3αxα−1[12(α+1)(34)α−1+(23)α−1]>2α3αxα−1[932(α+1)+(23)α−1](because(34)α−1>(34)2). |
Let p(α)=932(α+1)+(23)α−1, then we have p′(α)=932+(23)αln23 and p″(α)=(23)α(ln23)2>0. Hence, p′(α)≥p′(1)=932+23ln(23)≥1100>0, which implies that p(α) is increasing in the interval [1,+∞). Hence, p(α)≥p(1)=1148>0. It immediately yields that Qx(x,α)>2α3αxα−1p(α)>0. Therefore, we have
Q(x,α)≥f(4,α)=3α(4α+1−3α+1)−9α+2⋅4α(2α−3α)=2⋅9α[(129)α+(89)α−2]>0, |
as desired, and we have completed the proof.
Case 2. α∈[3,+∞).
Note that
Qx(x,α)=3α(α+1)[xα−(x−1)α]+2αxα−1(2α−3α)=xα−1{3α(α+1)x[1−(1−1x)α]+2α(2α−3α)}. |
Let g(α)=1−2x−(1−1x)α be a function defined in the interval [3,+∞), then we have g′(α)=(1−1x)αln(1+1x−1)>0. Hence, g(α)≥g(3)=1−2x−(1−1x)3=x2−3x+1x3>0, implying that 1−(1−1x)α>2x. Thus, we have
Qx(x,α)=xα−1{3α(α+1)x[1−(1−1x)α]+2α(2α−3α)}>2αxα−1[3αα+1α−(3α−2α)](becauseα+1α>1)>0. |
Let l(α)=(129)α+(89)α−2. It is routine to check that l′(α)=(89)α[(128)αln(129)+ln(89)]>(89)α[ln(129)+ln(89)]=(89)αln15>0. Hence, l(α)≥l(3)=782729>0. It then follows that
Q(x,α)≥f(4,α)=3α(4α+1−3α+1)−9α+2⋅4α(2α−3α)=2⋅9α[(129)α+(89)α−2]>0, |
as desired, and we have completed the proof.
Proposition 2.3. Let G∈Gγn be a graph with γ≥3 and n≥2(γ−1), then there is no pendent vertex in G if it has a minimum general Randić index for α≥1.
Proof. Suppose to the contrary that there exists a pendent vertex in G. Let u be a vertex of degree at least three and NG(u)={u1,u2,…,uk}. In what follows, we use P=uu1^u2…^ur to denote a pendent path in G. Assume that u2≠u1 is another neighbor of u with du2≥2. We consider the graph ^G1=G−uu2+u2^ur (depicted in Figure 2), which is an element of Gγn. Let l−2 be the number of vertices in {u3,u4,…,uk}, whose degree is greater than or equal to two. Clearly, l≥2 and k∑i=3dαui≥2α(l−2). For simplicity, we distinguish the following two cases:
Case 1. ℓ(P)=1.
Direct calculations show that
Rα(G)−Rα(^G1)=dαudαu2+dαu−2α(du−1)α−2αdαu2+[dαu−(du−1)α]k∑i=3dαui≥dαudαu2+dαu−2α(du−1)α−2αdαu2+2α(l−2)[dαu−(du−1)α]=[(dαu2−2α+1)(dαu−2α)]⏟A1+2α{(l−1)[dαu−(du−1)α]+(1−2α)}⏟A2. |
It is not difficult to find the first term of the previous equality A1=[(dαu2−2α+1)(dαu−2α)]>0 for α≥1, du≥3 and du2≥2. To continue the proof, it remains to verify that A2>0. For simplicity, we let H(x)=(l−1)[xα−(x−1)α]−(2α−1) for α≥1 and x≥3. It is routine to check that H(x)>[(xα−(x−1)α)−(3α−2α)]+[(3α−2α)−(2α−1)] since l≥3. Note that f1(x)=xα−(x−1)α is increasing in the interval [3,△], then we have xα−(x−1)α≥3α−2α. In addition, we know that 3α−2α≥2α−1 always holds for α≥1. Hence, H(x)>0 and, consequently, we have A2>0. It then immediately deduces that Rα(G)−Rα(^G1)>0, a contradiction. This implies that there is no pendent vertex in G.
Case 2. ℓ(P)≥2.
Direct calculations show that
Rα(G)−Rα(^G1)=dαudαu2−(du−1)α2α+2αdαu−2α(du−1)α+2α(1−2α)−2αdαu2+[dαu−(du−1)α]k∑i=3dαui≥dαudαu2−(du−1)α2α+2αdαu−2α(du−1)α+2α(l−2)[dαu−(du−1)α]+2α(1−2α)−2αdαu2=dαu2(dαu−2α)⏟A3+2α(l−1)[dαu−(du−1)α]⏟A4+2α[dαu−(du−1)α+1−2α]⏟A5. |
Note that A3>0 and A4>0, and it is also not difficult to find that A5=1l−1A2 is positive under the initial assumptions. Hence, Rα(G)−Rα(^G1)>0. Again a contradiction. This implies that there is no pendent vertex in G.
As desired, we complete the proof of Proposition 2.3.
Proposition 2.4. Let G∈Gγn be a graph with γ≥3 and n≥2(γ−1), then the maximum vertex degree is three in G if it has minimum general Randić index for 1≤α≤3925.
Proof. It follows from Proposition 2.3 that G contains at least one cycle as its induced subgraph, and the n-vertex cycle is the only connected graph for the which minimum and maximum vertex degree is two. Hence, in conjunction with the assumption γ≥3, we have Δ=Δ(G)≥3. To complete the proof, it suffices to show that Δ=3. If Δ>3, then it is routine to check that
n=∑2≤i≤Δni≥2(γ−1)=2(m−n)=2(∑2≤i≤Δini2−∑2≤i≤Δni), |
which is equivalent to
n2≥∑4≤i≤Δ(i−3)ni>∑4≤i≤Δ(4−3)ni>0. |
Hence, there at least exists a vertex of degree two. For simplicity, we suppose that u is the vertex in G with maximum degree and NG(u)={u1,u2,…,uΔ}. We distinguish the following two cases.
Case 1. ∃i∈{1,2,…,Δ} such that dui=2.
For convenience, we suppose that u1 is the neighbor of u with degree two and du2≥du3≥…≥2.
Subcase 1.1. du2=2 and u1 is not adjacent to u2.
Let ^G2=G−uu2+u1u2∈Gγn. t is the neighbor of u1, different from u, depicted in Figure 3. Hence, we have
Rα(G)−Rα(^G2)=dαu2α+1−(du−1)α3α−6α+dαt(2α−3α)+[dαu−(du−1)α]Δ∑i=3dαui=dαu2α+1−(du−1)α3α−6α+dαt(2α−3α)+2α(du−2)[dαu−(du−1)α]=2αdα+1u−(du−1)α[2α(du−2)+3α]+dαt(2α−3α)−6α≥2αdα+1u−(du−1)α[2α(du−2)+3α]+dαu(2α−3α)−6α. |
For simplicity, we let f2(x)=2αxα+1−(x−1)α[2α(x−2)+3α]+xα(2α−3α)−6α. It follows from Lemma 2.1 that f2(x)=P(x,α)>0 for x≥4 and 1≤α≤3925. Hence, Rα(G)−Rα(^G2)>0, which contradicts to the choice of G. Hence, the maximum vertex degree of G is three.
Subcase 1.2. du2=2 and u1 is adjacent to u2.
Let ^G3=G−uu3+u1u3∈Gγn, depicted in Figure 4. Hence, we have
Rα(G)−Rα(^G3)=3×dαu2α+4α−(du−1)α3α−2×6α−2α(du−1)α+[dαu−(du−1)α]Δ∑i=4dαui=3×dαu2α+4α−(du−1)α3α−2×6α−2α(du−1)α+2α(du−3)[dαu−(du−1)α]=2αdα+1u+4α−(du−1)α[2α(du−2)+3α]−2×6α. |
Let f3(x)=2αxα+1+4α−(x−1)α[2α(x−2)+3α]−2×6α, and then we have f3(x)=f2(x)+(3α−2α)(xα−2α)>0. Hence, Rα(G)−Rα(^G3)>0 for x≥4 and 1≤α≤3925, which contradicts to the choice of G. Hence, the maximum vertex degree of G is three.
Subcase 1.3. du2>2 and u1 is adjacent to u2 and du3>2.
Let ^G4=G−uu3+u1u3∈Gγn, depicted in Figure 5. Hence, we have
Rα(G)−Rα(^G4)=dαu2α+2αdαu2+dαudαu2+dαudαu3−(du−1)α3α−3αdαu2−dαu2(du−1)α−3αdαu3+[dαu−(du−1)α]Δ∑i=4dαui≥dαu2α+dαu2(2α−3α)+dαu3(dαu−3α)−(du−1)α3α+dαu2(dαu3−3α)−(du−1)α3α+2α(du−3)[dαu−(du−1)α]>2αdα+1u−(du−1)α[2α(du−2)+3α]+dαu(2α−3α)−6α>0, |
and the last inequality holds because f2(x)>0 for x≥4 and 1≤α≤3925. Hence, Rα(G)−Rα(^G4)>0. Again, a contradiction. Hence, the maximum vertex degree of G is three.
Subcase 1.4. du2>2, u1 is adjacent to u2 and du3=2.
Let ^G5=G−uu3+u1u3∈Gγn, depicted in Figure 6. Hence, we have
Rα(G)−Rα(^G5)=dαu2α+1+2αdαu2+dαudαu2−6α−(du−1)α3α−dαu23α−(du−1)αdαu2+[dαu−(du−1)α]Δ∑i=4dαui≥dαu2α+1−6α−(du−1)α3α+dαu2[(2α−3α+dαu−(du−1)α)]+2α(du−3)[dαu−(du−1)α]>2αdα+1u−(du−1)α[2α(du−2)+3α]+4α−2×6α>0, |
and the last inequality holds because f3(x)>0 for x≥4 and 1≤α≤3925. Hence, Rα(G)−Rα(^G5)>0. Again, a contradiction. Thus, we have completed that the maximum vertex degree of G is three.
Subcase 1.5. du2>2, u1 is not adjacent to u2.
Let ^G6=G−uu2+u1u2∈Gγn, depicted in Figure 7. Hence, we have
Rα(G)−Rα(^G6)=dαu2α+2αdαt+dαudαu2−(du−1)α3α−3αdαt−3αdαu2+[dαu−(du−1)α]Δ∑i=3dαui≥dαu2α+dαu(2α−3α)+2α(dαu−3α)−(du−1)α3α+2α(du−2)[dαu−(du−1)α]=2αdα+1u−(du−1)α[2α(du−2)+3α]+dαu(2α−3α)−6α>0, |
and the last inequality holds because f2(x)>0 for x≥4 and 1≤α≤3925. Hence, Rα(G)−Rα(^G6)>0, a contradiction to the choice of G. Hence, the maximum vertex degree of G is three.
Case 2. ∀i∈{1,2,…,Δ} such that dui>2.
Note that there is a vertex u0∈V(G)∖NG(u) of degree two, which is not adjacent to at least one neighbor, say u1, of u. Let ^G7=G−uu1+u0u1∈Gγn (depicted in Figure 8). Hence,
Rα(G)−Rα(^G7)=dαu1(dαu−3α)+(2α−3α)∑z∈NG(u0)dαz+[dαu−(du−1)α]Δ∑i=2dαui≥3α(dαu−3α)+2×dαu(2α−3α)+3α(du−1)[dαu−(du−1)α]=3α[dα+1u−(du−1)α+1]−9α+2×dαu(2α−3α). |
For simplicity, we let f4(x)=3α[xα+1−(x−1)α+1]−9α+2xα(2α−3α)=Q(x,α), which is positive for x≥4 and α≥1 by Lemma 2.2. Hence, we have Rα(G)−Rα(^G7)>0. Thus, there would be a contradiction to the choice of G, and the maximum vertex degree of G is three.
This completes the proof of Proposition 2.4.
Let φij be the number of edges in G joining the vertices of degree i and j, and we use ni and nj to denote the number of vertices of degree i and j, respectively.
Proposition 2.5.. ([2]) Let G∈Gγn, γ≥3, be a graph such that it contains only vertices of degrees two and three, then the following holds:
(i) at least two vertices of degree two are adjacent if n>5(γ−1).
(ii) φ22=0 implies φ33=0 (or φ33=0 implies φ22=0) if n=5(γ−1).
(iii) at least two vertices of degree three are adjacent if 2(γ−1)≤n≤5(γ−1).
Proposition 2.6. Let G∈Gγn be a graph with γ≥3 and n>5(γ−1), then at least one of the vertices x and y for any edge e=xy has the degree two in G if it has a minimum general Randić index for 1≤α≤3925.
Proof. By Proposition 2.3 and Proposition 2.4, we know 2≤du≤3 holds for any vertex u in G. Simultaneously, it follows from Proposition 2.5 that there at least exist two vertices, say u1 and u2, such that φ22>0. Suppose to the contrary that there exists two adjacent vertices v1 and v2 of degree three (i.e., φ33>0). Let u0≠u1 be the vertex adjacent with u2, which may coincide with v1 or v2. For convenience, we distinguish the following two cases.
Case 1. NG(u1)∩NG(u2)=∅.
Let ^G8=G−{u1u2,u2u0,v1v2}+{u1u0,v1u2,v2u2} (depicted in Figure 9), which is an element in Gγn. By direct calculations, we have Rα(G)−Rα(^G8)=4α+9α−2×6α>0. This contradicts to the assumption of G. Hence, φ33=0. As desired, we have completed the proof.
Case 2. NG(u1)∩NG(u2)≠∅.
Without loss of generality, we let u0∈NG(u1)∩NG(u2)≠∅. In what follows, we consider the following three subcases.
If u0≠{v1,v2} and u0 is not adjacent to v1 and v2, we let ^G9=G−{u2u0,v1v2}+{u2v2,v1u0} (depicted in Figure 10), which is an element in Gγn. By direct calculations, we have Rα(G)−Rα(^G9)=0. Note that N^G9(u1)∩N^G9(u2)=∅, by the analogous method as in Case 1, and there exists a new graph ~G1 such that Rα(G)−Rα(~G1)=Rα(^G9)−Rα(~G1)>0. This contradicts to the assumption of G. As desired, we have completed the proof.
If u0≠{v1,v2} and u0 is adjacent to v1, we let ^G10=G−{u1u2,v1v2}+{u1v1,u2v2}, which is an element in Gγn. By direct calculations, we have Rα(G)−Rα(^G10)=4α+9α−2⋅6α>0. This contradicts to the assumption of G. As desired, we have completed the proof.
If u0=v2, then we consider a neighbor ˜v of v1 different from v2. Let ^G11=G−{u2v2,˜vv1}+{v1u2,˜vv2}∈Gγn, and again we obtain that Rα(G)−Rα(^G11)=0. Note that N^G11(u1)∩N^G11(u2)=∅, by the analogous method as in Case 1, and there exists a new graph ~G2 such that Rα(G)−Rα(~G2)=Rα(^G11)−Rα(~G2)>0. This contradicts to the assumption of G. We have completed the proof.
Proposition 2.7. Let G∈Gγn be a graph with γ≥3 and n=5(γ−1), then one of the vertices x and y for any edge e=xy has the degree two and the other has the degree three in G if it has the minimum general Randić index for 1≤α≤3925.
Proof. It follows from Propositions 2.3 and 2.4 that 2≤du≤3 holds for any vertex u in G. If φ22=0 and φ33≠0, then one can find that φ23=0 by Proposition 2.5, a contradiction. If φ22≠0 and φ33≠0, then from the proof of Proposition 2.6 we conclude that there exists a graph ^G12∈Gγn such that Rα(G)−Rα(^G12)>0. This contradicts to the initial assumption of G. Hence, φ22=φ33=0. As desired, we complete the proof of Proposition 2.7.
In a similar way, we obtain the following fact.
Proposition 2.8. Let G∈Gγn be a graph with γ≥3 and 2(γ−1)<n<5(γ−1), then G does not contain any edge connecting the vertices of degree two if it has a minimum general Randić index for 1≤α≤3925.
Denote by ¯Gij[φij≠0] that the graphs contain only vertices of degree i and j, such that for every edge in a one end-vertex has the degree i and the other end-vertex has the degree j, and we use ¯Gij[φii=0] (resp. ¯Gij[φjj=0]) to denote the graphs containing only vertices of degree i and j, such that no vertices of degree i (resp. j) are adjacent.
Theorem 2.9. Let G∈Gγn be a graph with γ≥3 and n vertices, then the following holds for 1≤α≤3925:
(i) Rα(G)≥9α(n+γ−1) for n=2(γ−1), with equality if, and only if, G is isomophic to cubic graphs.
(ii) Rα(G)≥6α(2n−4γ+4)−9α(n−5γ+5) for 2(γ−1)<n<5(γ−1), with equality if, and only if, G is isomophic to ¯G23[φ22=0].
(iii) Rα(G)≥6α(n+γ−1) for n=5(γ−1), with equality if, and only if, G is isomophic to ¯G23[φ23≠0].
(iv) Rα(G)≥4α(n−5γ+5)+6α(6γ−6) for n>5(γ−1), with equality if, and only if, G is isomophic to ¯G23[φ33=0].
Proof. Let ^G13∈Gγn be a graph that achieves the minimum general Randić index. We only give the proof of (ii); the rest could be proved in a similar way. It follows from Propositions 2.3 and 2.4 that 2≤du≤3 holds for any vertex u in ^G13. Hence, we have n2+n3=n and 2n2+3n3=2(n+γ−1) by the Handshaking Theorem. Besides, by Proposition 2.8, it is easily seen that φ22=0. Hence, φ23=2n2 and φ23+2φ33=3n3. Direct calculations show that φ23=2n−4γ+4 and φ33=5γ−n−5. Thus, Rα(G)≥Rα(^G13)=6α(2n−4γ+4)−9α(n−5γ+5). The corresponding extremal graph is ¯G23[φ22=0].
The second Zagreb index is another well-known vertex degree-based graph invariant in chemical graph theory, which was introduced in 1972 by Gutman and Trinajstić [8]. We encourage the interested reader to consult [9,12] for more information for this graph invariant. Undoubtedly, the second Zagreb index is the special case of the general Randić index when α=1. It is easily seen that Theorem 2.9 extends one of the main results proved by Ali et al. [2].
We begin with the following auxiliary result, which plays an important part in our proofs.
Proposition 3.1. Let G∈Gγn be a graph with a maximum general Randić index for α≥1, then Δ(G)=n−1.
Proof. Suppose to the contrary that there exists a vertex u in G with Δ(G)=du<n−1. Note that there exists v∈V(G) such that u≠v, du≥dv and NG(v)∖NG(u)={v1,v2,…,vp}≠∅. We can construct a new graph ^G14 the following way
^G14=G−{vv1,vv2,…,vvp}+{uv1,uv2,…,uvp}∈Gγn. |
It is routine to check that
Rα(^G14)−Rα(G)=∑x∈NG(u)∖NG(v)[(du+p)α−dαu]dαx+p∑i=1[(du+p)α−dαv]dαvi+∑y∈NG(u)∩NG(v)[(du+p)α+(dv−p)α−dαu−dαv]dαy. |
Note that H(t)=tα is an increasing function for α≥1, and the first and second terms of the previous equality are nonnegative. By the Lagrange mean value theorem, we have (du+p)α−dαu=αpξα−1 (resp. dαv−(dv−p)α=αpηα−1) for ξ∈(du,du+p) (resp. η∈(dv−p,dv)). Hence, A6=(du+p)α+(dv−p)α−dαu−dαv>0, and, consequently, we have Rα(^G14)>Rα(G), a contradiction. This completes the proof.
Proposition 3.2. ([14]) Let x1,x2,…,xn,p,t≥1 be integers, α be any real number such that α∉{0,1} and x1+x2+…+xn=p.
(1) The function f(x1,x2,…,xn)=∑ni=1xαi is the minimum for α<0 or α>1 (maximum for 0<α<1, respectively) if, and only if, x1,x2,…,xn are almost equal, or |xi−xj|≤1 for every i,j=1,2,…,n.
(2) If x1≥x2≥t, the maximum of the function f(x1,x2,…,xn) is reached for α<0 or α>1 (minimum for 0<α<1,respectively) only for x1=p−t−n+2,x2=t,x3=x4=…=xn=1. The second maximum (the second minimum, respectively) is attained only for x1=p−t−n+1,x2=t+1,x3=x4=…=xn=1.
Theorem 3.3. Let G∈Gγn be a graph with \gamma = {k-1\choose 2} and k\geq 4 , then for \alpha\geq 1 we have
\begin{equation*} \begin{split} R_{\alpha}(G)\leq {k-1\choose 2}(k^{2}-2k+3)^{2\alpha}+(n-1)^{\alpha}(k^{2}-2k+3)^{\alpha}+(n-2)(n-1)^{\alpha}, \end{split} \end{equation*} |
with equality if, and only if, G\cong(K_{1}^{\gamma}\cup (n-2)K_{1})+K_{1}\cong K_{n}^{\gamma} , depicted in Figure 11.
Proof. It follows from Proposition 3.1 that there at least exists one vertex with a maximum degree n-1 . Hence, we have G = \widehat{G_{15}}+K_{1} , which contains |V(\widehat{G_{15}})| = n-1 vertices and m(\widehat{G_{15}}) = {k-1\choose 2} edges. For simplicity, let \pi = (d_{1}, d_{2}, \ldots, d_{n}) and \widehat{\pi} = (\widehat{d_{1}}, \widehat{d_{2}}, \ldots, \widehat{d_{n-1}}) be the nonincreasing degree sequence of G and \widehat{G_{15}} , respectively. Hence, d_{i} = \widehat{d_{i-1}}+1 for i = 2, 3, \ldots, n and d_{1} = n-1. Thus, we have
\begin{equation*} \begin{split} R_{\alpha}(G) = &\sum\limits_{v_{1}v_{j}\in E(G)}\left[d_{1}d_{j}\right]^{\alpha}+\sum\limits_{v_{i}v_{j}\in E(G), 2\leq i < j\leq n}\left[d_{i}d_{j}\right]^{\alpha}\\ = &(n-1)^{\alpha}\sum\limits_{i = 2}^{n}d_{i}^{\alpha}+\sum\limits_{v_{i}v_{j}\in E(\widehat{G_{15}})}\left[\left(\widehat{d_{i}}+1\right)\left(\widehat{d_{j}}+1\right)\right]^{\alpha}. \end{split} \end{equation*} |
By Proposition 3.2 for \alpha\geq 1 , we have
\begin{equation*} \begin{split} \mathscr{A}_{7} = &\sum\limits_{i = 1}^{n}d_{i}^{\alpha}-d_{1}^{\alpha}\\ \leq&1\cdot(n-1)^{\alpha}+1\cdot t^{\alpha}+(n-2)\cdot 1^{\alpha}-1\cdot(n-1)^{\alpha}\\ = &(n-1)^{\alpha}+(k^{2}-3k+3)^{\alpha}+(n-2)-(n-1)^{\alpha}\\ = &(k^{2}-3k+3)^{\alpha}+(n-2), \end{split} \end{equation*} |
where d_{1} = n-1 = 2m-t-n+2, d_{2} = t = 2\gamma+1 and d_{3} = d_{4} = \ldots = d_{n} = 1 . In addition, we find the maximum value of
\begin{equation*} \begin{split} \mathscr{A}_{8} = &\sum\limits_{v_{i}v_{j}\in E(\widehat{G_{15}})}\left[\left(\widehat{d_{i}}+1\right)\left(\widehat{d_{j}}+1\right)\right]^{\alpha}\\ \leq& {k-1\choose 2}\left[\left(k^{2}-3k+3\right)\left(k^{2}-3k+3\right)\right]^{\alpha}, \end{split} \end{equation*} |
with equality if, and only if, \widehat{G_{15}}\cong K_{1}^{\gamma}\cup (n-2)K_{1} .
It follows from the previous that
\begin{equation*} \begin{split} R_{\alpha}(G) = &(n-1)^{\alpha}\mathscr{A}_{7}+\mathscr{A}_{8}\\ \leq &{k-1\choose 2}(k^{2}-2k+3)^{2\alpha}+(n-1)^{\alpha}(k^{2}-2k+3)^{\alpha}+(n-2)(n-1)^{\alpha}, \end{split} \end{equation*} |
Hence, G = (K_{1}^{\gamma}\cup (n-2)K_{1})+K_{1}\cong K_{n}^{\gamma} . This completes the proof of Theorem 3.3.
The authors declare they have not used Artificial Intelligence(AI) tools in the creation of this article.
We would like to show our great gratitude to the anonymous referees for carefully reading the manuscript and improving its presentation and accuracy. The first author is supported by the Natural Science Foundation of Beijing (No.1222012) and National Key Research and Development Project (No.2019YFB2006602).
The authors declare that they have no conflicts of interest.
[1] | S. U. S. Choi, J. A. Eastman, Enhancing thermal conductivity of fluids with nanoparticles, ASME International Mechanical Engineering Congress & Exposition, San Francisco, 1995. |
[2] | S. K. Das, S. U. Choi, W. Yu, T. Pradeep, Nanofluids: science and technology, New York: John Wiley & Sons, 2007. |
[3] |
J. A. Eastman, U. S. Choi, S. Li, L. J. Thompson, S. Lee, Enhanced thermal conductivity through the development of nanofluids, MRS Online Proceedings Library, 457 (1996), 3–11. https://doi.org/10.1557/PROC-457-3 doi: 10.1557/PROC-457-3
![]() |
[4] |
J. Buongiorno, L. W. Hu, Innovative technologies: two-phase heat transfer in water-based nanofluids for nuclear applications final report, Nuclear Engineering Education Research (NEER) Program, 2009. https://doi.org/10.2172/958216 doi: 10.2172/958216
![]() |
[5] |
N. Putra, Yanuar, F. N. Iskandar, Application of nanofluids to a heat pipe liquid-block and the thermoelectric cooling of electronic equipment, Exp. Therm. Fluid Sci., 35 (2011), 1274–1281. https://doi.org/10.1016/j.expthermflusci.2011.04.015 doi: 10.1016/j.expthermflusci.2011.04.015
![]() |
[6] |
O. Mahian, A. Kianifar, S. A. Kalogirou, I. Pop, S. Wongwises, A review of the applications of nanofluids in solar energy, Int. J. Heat Mass Tran., 57 (2013), 582–594. https://doi.org/10.1016/j.ijheatmasstransfer.2012.10.037 doi: 10.1016/j.ijheatmasstransfer.2012.10.037
![]() |
[7] |
S. Rashidi, O. Mahian, E. M. Languri, Applications of nanofluids in condensing and evaporating systems, J. Therm. Anal. Calorim., 131 (2018), 2027–2039. https://doi.org/10.1007/s10973-017-6773-7 doi: 10.1007/s10973-017-6773-7
![]() |
[8] |
M. A. Nazari, M. H. Ahmadi, M. Sadeghzadeh, M. B. Shafii, M. Goodarzi, A review on application of nanofluid in various types of heat pipes, J. Cent. South Univ., 26 (2019), 1021–1041. https://doi.org/10.1007/s11771-019-4068-9 doi: 10.1007/s11771-019-4068-9
![]() |
[9] |
J. Li, X. Zhang, B. Xu, M. Yuan, Nanofluid research and applications: a review, Int. J. Heat Mass Tran., 127 (2021), 105543. https://doi.org/10.1016/j.icheatmasstransfer doi: 10.1016/j.icheatmasstransfer
![]() |
[10] |
M. A. Sheremet, Applications of Nanofluids, Nanomaterials (Basel), 11 (2021), 1716. https://doi.org/10.3390/nano11071716 doi: 10.3390/nano11071716
![]() |
[11] |
A. V. Kuznetsov, The onset of thermo-bioconvection in a shallow fluid saturated porous layer heated from below in a suspension of oxytactic microorganisms, Eur. J. Mech. B-Fluid., 25 (2006), 223–233. https://doi.org/10.1016/j.euromechflu.2005.06.003 doi: 10.1016/j.euromechflu.2005.06.003
![]() |
[12] |
A. V. Kuznetsov, Nanofluid bioconvection in water-based suspensions containing nanoparticles and oxytactic microorganisms: oscillatory instability, Nanoscale Res. Lett., 6 (2011), 100. https://doi.org/10.1186/1556-276X-6-100 doi: 10.1186/1556-276X-6-100
![]() |
[13] |
A. J. Hillesdon, T. J. Pedley, Bioconvection in suspensions of oxytactic bacteria: linear theory, J. Fluid Mech., 324 (1996), 223–259. https://doi.org/10.1017/S0022112096007902 doi: 10.1017/S0022112096007902
![]() |
[14] |
T. J. Pedley, N. A. Hill, J. O. Kessler, The growth of bioconvection patterns in a uniform suspension of gyrotactic micro-organisms, J. Fluid Mech., 195 (1988), 223–237. https://doi.org/10.1017/S0022112088002393 doi: 10.1017/S0022112088002393
![]() |
[15] |
T. Yamamoto, Numerical simulation of the flows of phototactic microalgae suspensions in an illuminated circular channel, Nihon Reoroji Gakk., 43 (2015) 53–62. https://doi.org/10.1678/rheology.43.53 doi: 10.1678/rheology.43.53
![]() |
[16] |
M. A. Sheremet, I. Pop, Thermo-bioconvection in a square porous cavity filled by oxytactic microorganisms, Transp. Porous Med., 103 (2014), 191–205. https://doi.org/10.1007/s11242-014-0297-4 doi: 10.1007/s11242-014-0297-4
![]() |
[17] |
C. S. Balla, A. Ramesh, N. Kishan, A. M. Rashad, Z. M. A. Abdelrahman, Bioconvection in oxytactic microorganism-saturated porous square enclosure with thermal radiation impact, J. Therm. Anal. Calorim., 140 (2020), 2387–2395. https://doi.org/10.1007/s10973-019-09009-7 doi: 10.1007/s10973-019-09009-7
![]() |
[18] |
S. Ahmad, M. Ashraf, K. Ali, Bioconvection due to gyrotactic microbes in a nanofluid flow through a porous medium, Heliyon, 6 (2020), e05832. https://doi.org/10.1016/j.heliyon.2020.e05832 doi: 10.1016/j.heliyon.2020.e05832
![]() |
[19] |
D. K. Mandal, N. Biswas, N. K. Manna, R. S. R. Gorla, A. J. Chamkha, Role of surface undulation during mixed bioconvective nanofluid flow in porous media in presence of oxytactic bacteria and magnetic fields, Int. J. Mech. Sci., 211 (2021), 106778. https://doi.org/10.1016/j.ijmecsci.2021.106778 doi: 10.1016/j.ijmecsci.2021.106778
![]() |
[20] |
N. Biswas, D. K. Mandal, N. K. Manna, A. C. Benim, Magneto-hydrothermal triple-convection in a W-shaped porous cavity containing oxytactic bacteria, Sci. Rep., 12 (2022), 18053. https://doi.org/10.1038/s41598-022-18401-7 doi: 10.1038/s41598-022-18401-7
![]() |
[21] |
M. Habibishandiz, Z. Saghir, MHD mixed convection heat transfer of nanofluid containing oxytactic microorganisms inside a vertical annular porous cylinder, International Journal of Thermofluids, 14 (2022), 100151. https://doi.org/10.1016/j.ijft.2022.100151 doi: 10.1016/j.ijft.2022.100151
![]() |
[22] |
S. Hussain, A. M. Aly, H. F. Öztop, Magneto-bioconvection flow of hybrid nanofluid in the presence of oxytactic bacteria in a lid-driven cavity with a streamlined obstacle, Int. Commun. Heat Mass, 134 (2022), 106029. https://doi.org/10.1016/j.icheatmasstransfer.2022.106029 doi: 10.1016/j.icheatmasstransfer.2022.106029
![]() |
[23] |
A. M. Rashad, H. A. Nabwey, Gyrotactic mixed bioconvection flow of a nanofluid past a circular cylinder with convective boundary condition, J. Taiwan Inst. Chem. E., 99 (2019), 9–17. https://doi.org/10.1016/j.jtice.2019.02.035 doi: 10.1016/j.jtice.2019.02.035
![]() |
[24] |
B. P. Geridonmez, H. F. Oztop, Conjugate natural convection flow of a nanofluid with oxytactic bacteria under the effect of a periodic magnetic field, J. Magn. Magn. Mater., 564 (2022), 170135. https://doi.org/10.1016/j.jmmm.2022.170135 doi: 10.1016/j.jmmm.2022.170135
![]() |
[25] |
B. Chen, X. Wang, R. Zeng, Y. Zhang, X. Wang, J. Niu, et al., An experimental study of convective heat transfer with microencapsulated phase change material suspension: Laminar flow in a circular tube under constant heat flux, Exp. Therm. Fluid Sci., 32 (2008), 1638–1646. https://doi.org/10.1016/j.expthermflusci.2008.05.008 doi: 10.1016/j.expthermflusci.2008.05.008
![]() |
[26] |
W. Wu, H. Bostanci, L. C. Chow, Y. Hong, C. M. Wang, M. Su, et al., Heat transfer enhancement of PAO in microchannel heat exchanger using nano-encapsulated phase change indium particles, Int. J. Heat Mass Tran., 58 (2013), 348–355. https://doi.org/10.1016/j.ijheatmasstransfer.2012.11.032 doi: 10.1016/j.ijheatmasstransfer.2012.11.032
![]() |
[27] |
C. J. Smith, P. M. Forster, R. Crook, Global analysis of photovoltaic energy output enhanced by phase change material cooling, Appl. Energ., 126 (2014), 21–28. https://doi.org/10.1016/j.apenergy.2014.03.083 doi: 10.1016/j.apenergy.2014.03.083
![]() |
[28] |
W. Su, J. Darkwa, G. Kokogiannakis, Review of solid-liquid phase change materials and their encapsulation technologies, Renew. Sust. Energ. Rev., 48 (2015), 373–391. https://doi.org/10.1016/j.rser.2015.04.044 doi: 10.1016/j.rser.2015.04.044
![]() |
[29] |
Y. Pahamli, M. J. Hosseini, A. A. Ranjbar, R. Bahrampoury, Analysis of the effect of eccentricity and operational parameters in PCM-filled single-pass shell and tube heat exchangers, Renew. Energ., 97 (2016), 344–357. https://doi.org/10.1016/j.renene.2016.05.090 doi: 10.1016/j.renene.2016.05.090
![]() |
[30] |
C. Liu, Z. Rao, J. Zhao, Y. Huo, Y. Li, Review on nanoencapsulated phase change materials: Preparation, characterization and heat transfer enhancement, Nano Energy, 13 (2015), 814–826. https://doi.org/10.1016/j.nanoen.2015.02.016 doi: 10.1016/j.nanoen.2015.02.016
![]() |
[31] |
H. R. Seyf, Z. Zhou, H. B. Ma, Y. Zhang, Three dimensional numerical study of heat-transfer enhancement by nano-encapsulated phase change material slurry in microtube heat sinks with tangential impingement, Int. J. Heat Mass Tran., 56 (2013), 561–573. https://doi.org/10.1016/j.ijheatmasstransfer.2012.08.052 doi: 10.1016/j.ijheatmasstransfer.2012.08.052
![]() |
[32] |
M. Ghalambaz, S. A. M. Mehryan, I. Zahmatkesh, A. Chamkha, Free convection heat transfer analysis of a suspension of nano-encapsulated phase change materials (NEPCMs) in an inclined porous cavity, Int. J. Therm. Sci., 157 (2020), 106503. https://doi.org/10.1016/j.ijthermalsci.2020.106503 doi: 10.1016/j.ijthermalsci.2020.106503
![]() |
[33] |
S. A. M. Mehryan, M. Ismael, M. Ghalambaz, Local thermal nonequilibrium conjugate natural convection of nano-encapsulated phase change particles in a partially porous enclosure, Math. Method. Appl. Sci., 2020 (2020), 6338. https://doi.org/10.1002/mma.6338 doi: 10.1002/mma.6338
![]() |
[34] |
S. Hussain, N. Alsedias, A. M. Aly, Natural convection of a water-based suspension containing nano-encapsulated phase change material in a porous grooved cavity, J. Energy Storage, 51 (2022), 104589. https://doi.org/10.1016/j.est.2022.104589 doi: 10.1016/j.est.2022.104589
![]() |
[35] |
C. J. Ho, Y. C. Liu, T. F. Yang, M. Ghalambaz, W. M. Yan, Convective heat transfer of nano-encapsulated phase change material suspension in a divergent minichannel heatsink, Int. J. Heat Mass Tran., 165 (2021), 120717. https://doi.org/10.1016/j.ijheatmasstransfer.2020.120717 doi: 10.1016/j.ijheatmasstransfer.2020.120717
![]() |
[36] |
R. A. Gingold, J. J. Monaghan, Smoothed particle hydrodynamics: theory and application to non-spherical stars, Mon. Not. R. Astron. Soc., 181 (1977), 375–389. https://doi.org/10.1093/mnras/181.3.375 doi: 10.1093/mnras/181.3.375
![]() |
[37] |
L. B. Lucy, A numerical approach to the testing of the fission hypothesis, Astron. J., 82 (1977), 1013–1024. https://doi.org/10.1086/112164 doi: 10.1086/112164
![]() |
[38] |
S. J. Cummins, M. Rudman, An SPH projection method, J. Comput. Phys., 152 (1999), 584–607. https://doi.org/10.1006/jcph.1999.6246 doi: 10.1006/jcph.1999.6246
![]() |
[39] |
M. Asai, A. M. Aly, Y. Sonoda, Y. Sakai, A stabilized incompressible SPH method by relaxing the density invariance condition, J. Appl. Math., 2012 (2012), 139583. https://doi.org/10.1155/2012/139583 doi: 10.1155/2012/139583
![]() |
[40] |
F. Garoosi, A. Shakibaeinia, Numerical simulation of entropy generation due to natural convection heat transfer using Kernel Derivative-Free (KDF) Incompressible Smoothed Particle Hydrodynamics (ISPH) model, Int. J. Heat Mass Tran., 150 (2020), 119377. https://doi.org/10.1016/j.ijheatmasstransfer.2020.119377 doi: 10.1016/j.ijheatmasstransfer.2020.119377
![]() |
[41] |
A. M. Aly, Mixing between solid and fluid particles during natural convection flow of a nanofluid-filled H-shaped cavity with three center gates using ISPH method, Int. J. Heat Mass Tran., 157 (2020), 119803. https://doi.org/10.1016/j.ijheatmasstransfer.2020.119803 doi: 10.1016/j.ijheatmasstransfer.2020.119803
![]() |
[42] |
A. M. Aly, A. M. Yousef, N. Alsedais, MHD double diffusion of a nanofluid within a porous annulus using a time fractional derivative of the ISPH method, Int. J. Mod. Phys. C, 33 (2021), 2250056. https://doi.org/10.1142/S0129183122500565 doi: 10.1142/S0129183122500565
![]() |
[43] |
Y. Shimizu, H. Gotoh, A. Khayyer, K. Kita, Fundamental investigation on the applicability of higher-order consistent ISPH method, Int. J. Offshore Polar, 32 (22022), 275–284. https://doi.org/10.17736/ijope.2022.jc868 doi: 10.17736/ijope.2022.jc868
![]() |
[44] |
A. M. Salehizadeh, A. R. Shafiei, A coupled ISPH-TLSPH method for simulating fluid-elastic structure interaction problems, J. Marine. Sci. Appl., 21 (2022), 15–36. https://doi.org/10.1007/s11804-022-00260-3 doi: 10.1007/s11804-022-00260-3
![]() |
[45] |
N. Alsedais, A. Al-Hanaya, A. M. Aly, Magneto-bioconvection flow in a porous annulus between circular cylinders containing oxytactic microorganisms and NEPCM, Int. J. Numer. Method. H., 33 (2023), 3228–3254. https://doi.org/10.1108/HFF-02-2023-0095 doi: 10.1108/HFF-02-2023-0095
![]() |
[46] |
W. Alhejaili, A. M. Aly, Magneto-bioconvection flow in an annulus between circular cylinders containing oxytactic microorganisms, Int. Commun. Heat Mass, 146 (2023), 106893. https://doi.org/10.1016/j.icheatmasstransfer.2023.106893 doi: 10.1016/j.icheatmasstransfer.2023.106893
![]() |
[47] |
A. Alaria, A. M. Khan, D. L. Suthar, D. Kumar, Application of fractional operators in modelling for charge carrier transport in amorphous semiconductor with multiple trapping, Int. J. Appl. Comput. Math., 5 (2019), 167. https://doi.org/10.1007/s40819-019-0750-8 doi: 10.1007/s40819-019-0750-8
![]() |
[48] |
M. M. A. Khater, D. Baleanu, On abundant new solutions of two fractional complex models, Adv. Differ. Equ., 2020 (2020), 268. https://doi.org/10.1186/s13662-020-02705-x doi: 10.1186/s13662-020-02705-x
![]() |
[49] |
A. Hyder, A. H. Soliman, A new generalized θ-conformable calculus and its applications in mathematical physics, Phys. Scr., 96 (2020), 015208. https://doi.org/10.1088/1402-4896/abc6d9 doi: 10.1088/1402-4896/abc6d9
![]() |
[50] |
A. Hyder, M. A. Barakat, Novel improved fractional operators and their scientific applications, Adv. Differ. Equ., 2021 (2021), 389. https://doi.org/10.1186/s13662-021-03547-x doi: 10.1186/s13662-021-03547-x
![]() |
[51] |
A. Hyder, M. A. Barakat, A. Fathallah, C. Cesarano, Further integral inequalities through some generalized fractional integral operators, Fractal Fract., 5 (2021), 282. https://doi.org/10.3390/fractalfract5040282 doi: 10.3390/fractalfract5040282
![]() |
[52] |
T. Abdeljawad, On conformable fractional calculus, J. Comput. Appl. Math., 279 (2015), 57–66. https://doi.org/10.1016/j.cam.2014.10.016 doi: 10.1016/j.cam.2014.10.016
![]() |
[53] |
M. Ghalambaz, A. J. Chamkha, D. Wen, Natural convective flow and heat transfer of Nano-Encapsulated Phase Change Materials (NEPCMs) in a cavity, Int. J. Heat Mass Tran., 138 (2019), 738–749. https://doi.org/10.1016/j.ijheatmasstransfer.2019.04.037 doi: 10.1016/j.ijheatmasstransfer.2019.04.037
![]() |
[54] | I. Podlubny, Fractional differential equations: An introduction to fractional derivatives, fractional differential equations, to methods of their solution and some of their applications, San Diego: Academic Press, 1999. |
[55] |
Ş. Toprakseven, Numerical solutions of conformable fractional differential equations by Taylor and finite difference methods, Süleyman Demirel Üniversitesi Fen Bilimleri Enstitüsü Dergisi, 23 (2019), 850–863. https://doi.org/10.19113/sdufenbed.579361 doi: 10.19113/sdufenbed.579361
![]() |