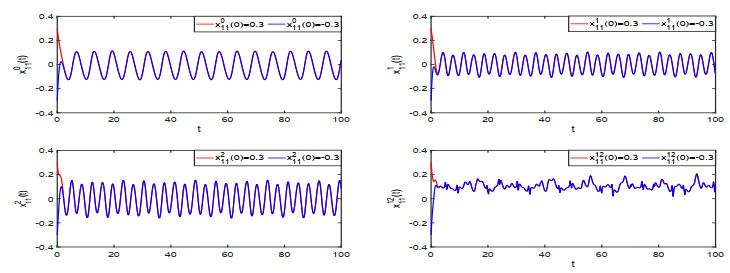
The genesis models of the iron-ores hosted in banded iron formations (BIFs) in the Hamersley Province of Western Australia have been debated since the iron-ore deposits were discovered in the 1960s. The existing models considered the few physicochemical conditions for the iron-ore enrichment from BIFs. This study incorporates the latest research outcomes in conversions among the major magnetic minerals under different physicochemical conditions with the thermal magnetic analysis for BIFs and iron-ores collected from the Hamersley Province to fill the gap in knowledge highlighted by existing studies of the iron ores and BIFs. The results indicate that the high-grade hematite ores might have been undergone a physicochemical process under hydrothermal conditions between 120 ℃ and 220 ℃ during the major stage of enrichment from the original BIFs in the Brockman Iron Formation. Such physicochemical conditions would require either that the BIF units were buried 4000–5000 m underground with tilted broad channels formed by large-scale deformation in the region that facilitates hydrothermal reactions and leaching by the fluids flowing down deep to 4000–5000 m, somehow similar to the deep-seated supergene model proposed in previous works, or that the BIF units were still buried but the hydrothermal fluids coming up from deeper sources spread widely over the broad channels to ensure the high-grade hematite ores are consistently uniform over the entire deposit. The large-scale martite-goethite deposits in the Marra Mamba Iron Formation might be derived from multiple supergene phases from hematite-martite ores below 100 ℃ in the natural process of oxidization near surface, somewhat similar to the existing model for the channel iron deposits. Magnetite contained within current BIFs and iron ores was least likely derived from primary hematite in BIFs.
Citation: William Guo. Thermal magnetic analysis on iron ores and banded iron formations (BIFs) in the Hamersley Province: Implications of origins of magnetic minerals and iron ores[J]. AIMS Geosciences, 2023, 9(2): 311-329. doi: 10.3934/geosci.2023017
[1] | Nina Huo, Bing Li, Yongkun Li . Global exponential stability and existence of almost periodic solutions in distribution for Clifford-valued stochastic high-order Hopfield neural networks with time-varying delays. AIMS Mathematics, 2022, 7(3): 3653-3679. doi: 10.3934/math.2022202 |
[2] | Yongkun Li, Xiaoli Huang, Xiaohui Wang . Weyl almost periodic solutions for quaternion-valued shunting inhibitory cellular neural networks with time-varying delays. AIMS Mathematics, 2022, 7(4): 4861-4886. doi: 10.3934/math.2022271 |
[3] | Ardak Kashkynbayev, Moldir Koptileuova, Alfarabi Issakhanov, Jinde Cao . Almost periodic solutions of fuzzy shunting inhibitory CNNs with delays. AIMS Mathematics, 2022, 7(7): 11813-11828. doi: 10.3934/math.2022659 |
[4] | Yuwei Cao, Bing Li . Existence and global exponential stability of compact almost automorphic solutions for Clifford-valued high-order Hopfield neutral neural networks with D operator. AIMS Mathematics, 2022, 7(4): 6182-6203. doi: 10.3934/math.2022344 |
[5] | Jin Gao, Lihua Dai . Weighted pseudo almost periodic solutions of octonion-valued neural networks with mixed time-varying delays and leakage delays. AIMS Mathematics, 2023, 8(6): 14867-14893. doi: 10.3934/math.2023760 |
[6] | Hedi Yang . Weighted pseudo almost periodicity on neutral type CNNs involving multi-proportional delays and D operator. AIMS Mathematics, 2021, 6(2): 1865-1879. doi: 10.3934/math.2021113 |
[7] | Yanshou Dong, Junfang Zhao, Xu Miao, Ming Kang . Piecewise pseudo almost periodic solutions of interval general BAM neural networks with mixed time-varying delays and impulsive perturbations. AIMS Mathematics, 2023, 8(9): 21828-21855. doi: 10.3934/math.20231113 |
[8] | Zhangir Nuriyev, Alfarabi Issakhanov, Jürgen Kurths, Ardak Kashkynbayev . Finite-time synchronization for fuzzy shunting inhibitory cellular neural networks. AIMS Mathematics, 2024, 9(5): 12751-12777. doi: 10.3934/math.2024623 |
[9] | Abdulaziz M. Alanazi, R. Sriraman, R. Gurusamy, S. Athithan, P. Vignesh, Zaid Bassfar, Adel R. Alharbi, Amer Aljaedi . System decomposition method-based global stability criteria for T-S fuzzy Clifford-valued delayed neural networks with impulses and leakage term. AIMS Mathematics, 2023, 8(7): 15166-15188. doi: 10.3934/math.2023774 |
[10] | Xiaofang Meng, Yongkun Li . Pseudo almost periodic solutions for quaternion-valued high-order Hopfield neural networks with time-varying delays and leakage delays on time scales. AIMS Mathematics, 2021, 6(9): 10070-10091. doi: 10.3934/math.2021585 |
The genesis models of the iron-ores hosted in banded iron formations (BIFs) in the Hamersley Province of Western Australia have been debated since the iron-ore deposits were discovered in the 1960s. The existing models considered the few physicochemical conditions for the iron-ore enrichment from BIFs. This study incorporates the latest research outcomes in conversions among the major magnetic minerals under different physicochemical conditions with the thermal magnetic analysis for BIFs and iron-ores collected from the Hamersley Province to fill the gap in knowledge highlighted by existing studies of the iron ores and BIFs. The results indicate that the high-grade hematite ores might have been undergone a physicochemical process under hydrothermal conditions between 120 ℃ and 220 ℃ during the major stage of enrichment from the original BIFs in the Brockman Iron Formation. Such physicochemical conditions would require either that the BIF units were buried 4000–5000 m underground with tilted broad channels formed by large-scale deformation in the region that facilitates hydrothermal reactions and leaching by the fluids flowing down deep to 4000–5000 m, somehow similar to the deep-seated supergene model proposed in previous works, or that the BIF units were still buried but the hydrothermal fluids coming up from deeper sources spread widely over the broad channels to ensure the high-grade hematite ores are consistently uniform over the entire deposit. The large-scale martite-goethite deposits in the Marra Mamba Iron Formation might be derived from multiple supergene phases from hematite-martite ores below 100 ℃ in the natural process of oxidization near surface, somewhat similar to the existing model for the channel iron deposits. Magnetite contained within current BIFs and iron ores was least likely derived from primary hematite in BIFs.
As stated in [1], a nervous system in the real world, synaptic transmission is a noisy process caused by random fluctuations in neurotransmitter release and other probabilistic factors. Therefore, it is necessary to consider stochastic neural networks (NNs) because random inputs may change the dynamics of the (NN) [2,3,4,5].
SICNNs, which were proposed in [6], have attracted the interest of many scholars since their introduction due to their special roles in psychophysics, robotics, adaptive pattern recognition, vision, and image processing. In the above applications, their dynamics play an important role. Thereupon, their various dynamics have been extensively studied (see [7,8,9,10,11,12,13] and references therein). However, there is limited research on the dynamics of stochastic SICNNs. Therefore, it is necessary to further study the dynamics of such NNs.
On the one hand, research on the dynamics of NNs that take values from a non commutative algebra, such as quaternion-valued NNs [14,15,16], octonion-valued NNs [17,18,19,20], and Clifford-valued NNs [21,22,23], has gained the interest of many researchers because such neural networks can include typical real-valued NNs as their special cases, and they have superior multi-dimensional signal processing and data storage capabilities compared to real-valued NNs. It is worth mentioning that in recent years, many authors have conducted extensive research on various dynamics of Clifford-valued NNs, such as the existence, multiplicity and stability of equilibrium points, and the existence, multiplicity and stability of almost periodic solutions as well as the synchronization problems [22,23,24,25,26,27,28,29,30]. However, most of the existing results for the dynamics of Clifford-valued NNs has been obtained through decomposition methods [24,25,26,27]. However, the results obtained by decomposition methods are generally not convenient for direct application, and there is little research on Clifford-valued NNs using non decomposition methods [28,29,30]. Therefore, further exploration of using non decomposition methods to study the dynamics of Clifford-valued NNs has important theoretical significance and application value.
On the other hand, Bohr's almost periodicity is a special case of Stepanov's almost periodicity, but there is little research on the Stepanov periodic oscillations of NNs [19,31,32,33], especially the results of Stepanov's almost periodic solutions of stochastic SICNNs with discrete and infinitely distributed delays have not been published yet.
Motivated by the discussion above, our purpose of this article is to establish the existence and global exponential stability of Stepanov almost periodic solutions in the distribution sense for a stochastic Clifford-valued SICNN with mixed delays via non decomposition methods.
The subsequent sections of this article are organized as follows. Section 2 introduces some concepts, notations, and basic lemmas and gives a model description. Section 3 discusses the existence and stability of Stepanov almost periodic solutions in the distribution sense of the NN under consideration. An example is provided in Section 4. Finally, Section 5 provides a brief conclusion.
Let A={∑ϑ∈Pxϑeϑ,xϑ∈R} be a real Clifford-algebra with N generators e∅=e0=1, and eh,h=1,2,⋯,N, where P={∅,0,1,2,⋯,ϑ,⋯,12⋯N}, e2i=1,i=1,2,⋯,r,e2i=−1,i=r+1,r+2,⋯,m,eiej+ejei=0,i≠j and i,j=1,2,⋯,N. For x=∑ϑ∈Pxϑeϑ∈A, we indicate ‖x‖♭=maxϑ∈P{|xϑ|},xc=∑ϑ≠∅xϑeϑ,x0=x−xc, and for x=(x11,x12,…,x1n,x21,x22,…,x2n,…,xmn)T∈Am×n, we denote ‖x‖0=max{‖xij‖♭,1≤i≤m,1≤j≤n}. The derivative of x(t)=∑ϑ∈Pxϑ(t)eϑ is defined by ˙x(t)=∑ϑ∈P˙xϑ(t)eϑ and the integral of x(t)=∑ϑ∈Pxϑ(t)eϑ over the interval [a,b] is defined by ∫bax(t)dt=∑ϑ∈P(∫baxϑ(t)dt)eϑ.
Let (Y,ρ) be a separable metric space and P(Y) the collection of all probability measures defined on Borel σ-algebra of Y. Denote by Cb(Y) the set of continuous functions f:Y→R with ‖g‖∞:=supx∈Y{|g(x)|}<∞.
For g∈Cb(Y), μ,ν∈P(Y), let us define
‖g‖L=supx≠y|g(x)−g(y)|ρ(x,y),‖g‖BL=max{‖g‖∞,‖g‖L}, |
ρBL(μ,ν):=sup‖g‖BL≤1|∫Ygd(μ−ν)|. |
According to [34], (Y,ρBL(⋅,⋅)) is a Polish space.
Definition 2.1. [35] A continuous function g:R→Y is called almost periodic if for every ε>0, there is an ℓ(ε)>0 such that each interval with length ℓ has a point τ meeting
ρ(g(t+τ),g(t))<ε,forallt∈R. |
We indicate by AP(R,Y) the set of all such functions.
Let (X,‖⋅‖) signify a separable Banach space. Denote by μ(X):=P∘X−1 and E(X) the distribution and the expectation of X:(Ω,F,P)→X, respectively.
Let Lp(Ω,X) indicate the family of all X-valued random variables satisfying E(‖X‖p)=∫Ω‖X‖pdP<∞.
Definition 2.2. [21] A process Z:R→Lp(Ω,X) is called Lp-continuous if for any t0∈R,
limt→t0E‖Z(t)−Z(t0)‖p=0. |
It is Lp-bounded if supt∈RE‖Z(t)‖p<∞.
For 1<p<∞, we denote by Lploc(R,X) the space of all functions from R to X which are locally p-integrable. For g∈Lploc(R,X), we consider the following Stepanov norm:
‖g‖Sp=supt∈R(∫t+1t‖g(s)‖pds)1p. |
Definition 2.3. [35] A function g∈Lploc(R,X) is called p-th Stepanov almost periodic if for any ε>0, it is possible to find a number ℓ>0 such that every interval with length ℓ has a number τ such that
‖g(t+τ)−g(t)‖Sp<ε. |
Definition 2.4. [9] A stochastic process Z∈Lploc(R,Lp(Ω,X)) is said to be Sp-bounded if
‖Z‖Sps:=supt∈R(∫t+1tE‖Z(s)‖pds)1p<∞. |
Definition 2.5. [9] A stochastic process Z∈Lloc(R,Lp(Ω,H)) is called Stepanov almost periodic in p-th mean if for any ε>0, it is possible to find a number ℓ>0 such that every interval with length ℓ has a number τ such that
‖Z(t+τ)−Z(t)‖Sps<ε. |
Definition 2.6. [9] A stochastic process Z:R→Lp(Ω,X)) is said to be p-th Stepanov almost periodic in the distribution sense if for each ε>0, it is possible to find a number ℓ>0 such that any interval with length ℓ has a number τ such that
supa∈R(∫a+1adpBL(P∘[Z(t+τ)]−1,P∘[Z(t)]−1)dt)1p<ε. |
Lemma 2.1. [36] (Burkholder-Davis-Gundy inequality) If f∈L2(J,R), p>2, B(t) is Brownian motion, then
E[supt∈J|∫tt0f(s)dB(s)|p]≤CpE[∫Tt0|f(s)|2ds]p2, |
where cp=(pp+12(p−1)p−1)p2.
The model that we consider in this paper is the following stochastic Clifford-valued SICNN with mixed delays:
dxij(t)=[−aij(t)xij(t)+∑Ckl∈Nh1(i,j)Cklij(t)f(xkl(t−τkl(t)))xij(t)+∑Ckl∈Nh2(i,j)Bklij(t)∫∞0Kij(u)g(xkl(t−u))duxij(t)+Lij(t)]dt+∑Ckl∈Nh3(i,j)Eklij(t)δij(xij(t−σij(t)))dωij(t), | (2.1) |
where i=1,2,⋯,m,j=1,2,⋯,n, Cij(t) represents the cell at the (i,j) position, the h1-neighborhood Nh1(i,j) of Cij is given as:
Nh1(i,j)={Ckl:max(|k−i|,|l−j|)≤h1,1≤k≤m,1≤l≤n}, |
Nh2(i,j),Nh3(i,j) are similarly defined, xij denotes the activity of the cell Cij, Lij(t):R→A corresponds to the external input to Cij, the function aij(t):R→A represents the decay rate of the cell activity, Cklij(t):R→A,Bklij(t):R→A and Eklij(t):R→A signify the connection or coupling strength of postsynaptic activity of the cell transmitted to the cell Cij, and the activity functions f(⋅):A→A, and g(⋅):A→A are continuous functions representing the output or firing rate of the cell Ckl, and τkl(t),σij(t):R→R+ are the transmission delay, the kernel Kij(t):R→R is an integrable function, ωij(t) represents the Brownian motion defined on a complete probability space, δij(⋅):A→A is a Borel measurable function.
Let (Ω, F, {Ft}t⩾, P ) be a complete probability space in which \{\mathscr{F}_t\}_{t\geqslant 0} is a natural filtration meeting the usual conditions. Denote by B_{\mathscr{F}_0}([-\theta, 0], \mathscr{A}^n) the family of bounded, \mathscr{F}_0 -measurable and \mathscr{A}^n -valued random variables from [-\theta, 0]\rightarrow \mathscr{A}^n . The initial values of system (2.1) are depicted as
x_i(s) = \phi_i(s),\; \; \; s\in[-\theta,0], |
where \phi_i\in B_{\mathscr{F}_0} ([-\theta, 0], \mathscr{A}), \theta = \max\limits_{1\leq i, j\leq n}\{ \sup\limits_{t\in \mathbb{R}}\tau_{ij}(t), \sup\limits_{t\in \mathbb{R}}\sigma_{ij}(t)\} .
For convenience, we introduce the following notations:
\begin{align*} &\underline{a}^0 = \min\limits_{ij\in\Lambda} \underline{a}_{ij}^0 = \min\limits_{ij\in\Lambda}\inf\limits_{t\in\mathbb{R}} a_{ij}^0(t),\; \; \bar{a}^0 = \max\limits_{ij\in\Lambda} \bar{a}_{ij}^0 = \max\limits_{ij\in\Lambda}\sup\limits_{t\in\mathbb{R}} a_{ij}^0(t),\; \; {C_{ij}^{kl}}^+ = \sup\limits_{t\in\mathbb{R}}\|C_{ij}^{kl}(t) \|_{\flat},\\ &\bar{a^c} = \max\limits_{ij\in\Lambda} \bar{a}_{ij}^c = \max\limits_{ij\in\Lambda}\sup\limits_{t\in\mathbb{R}} \|a_{ij}^c(t)\|_{\flat}, \; \; {B_{ij}^{kl}}^+ = \sup\limits_{t\in\mathbb{R}} \|B_{ij}^{kl}(t)\|_{\flat},\; \; {E_{ij}^{kl}}^+ = \sup\limits_{t\in\mathbb{R}}\|E_{ij}^{kl}(t)\|_{\flat}, \\ & K_{ij}^+ = \sup\limits_{t\in\mathbb{R}}K_{ij}(t),\; \; \tau_{kl}^+ = \sup\limits_{t\in\mathbb{R}}\tau_{kl}(t),\; \; \dot{\tau}_{kl}^+ = \sup\limits_{t\in\mathbb{R}}\dot{\tau}_{kl}(t), \sigma_{ij}^+ = \sup\limits_{t\in \mathbb{R}}\sigma_{ij}(t),\; \; \dot{\sigma}_{ij}^+ = \sup\limits_{t\in\mathbb{R}}\dot{\sigma}_{ij}(t), \\ & M_L = \max\limits_{ij\in\Lambda} L_{ij}^+ = \max\limits_{ij\in\Lambda}\sup\limits_{t\in\mathbb{R}}\|L_{ij}(t)\|_{\flat},\; \; \theta = \max\limits_{ij\in\Lambda}\{\tau_{ij}^+,\sigma_{ij}^+\},\; \; \Lambda = \{11,12,\cdots,1n,\cdots,mn\}. \end{align*} |
Throughout this paper, we make the following assumptions:
(A1) For ij\in\Lambda , f, g, \delta_{ij}\in C(\mathscr{A}, \mathscr{A}) satisfy the Lipschitz condition, and f, g are bounded, that is, there exist constants L_f > 0, L_g > 0, L_{ij}^{\delta} > 0, M_f > 0, M_g > 0 such that for all x, y\in \mathscr{A} ,
\begin{align*} ||f(x)-f(y)||_{\flat}\leq L_f||x-y||_{\flat},\; \; ||g(x)-g(y)||_{\flat}\leq L_g||x-y||_{\flat},\\ ||\delta_{ij}(x)-\delta_{ij}(y)||_{\flat}\leq L_{ij}^{\delta}||x-y||_{\flat} , ||f(x)||_{\flat}\leq M_f ,\; \; ||g(x)||_{\flat}\leq M_g; \end{align*} |
furthermore, f(0) = g(0) = \delta_{ij}(0) = 0 .
(A2) For ij\in\Lambda , {a}_{ij}^0\in AP(\mathbb{R}, \mathbb{R}^+), {a}_{ij}^c\in AP(\mathbb{R}, \mathscr{A}), \tau_{ij}, \sigma_{ij}\in AP(\mathbb{R}, \mathbb{R}^+)\cap C^1(\mathbb{R}, \mathbb{R}) satisfying 1-\dot{\tau}_{ij}^+, 1-\dot{\sigma}_{ij}^+ > 0 , C_{ij}^{kl}, B_{ij}^{kl}, E_{ij}^{kl}\in AP(\mathbb{R}, \mathscr{A}) , L = (L_{11}, L_{12}, \cdots, L_{mn})\in\mathscr{L}_{loc}^p(\mathbb{R}, L^p(\Omega, \mathscr{A}^{m\times n})) is almost periodic in the sense of Stepanov.
(A3) For p > 2, \frac{1}{p}+\frac{1}{q} = 1 ,
\begin{align*} 0 < r^1: = & \frac{8^p}{4}\max\limits_{ij\in\Lambda}\bigg\{ \bigg(\frac{p}{q\underline{a}_{ij}^0}\bigg)^{\frac{p}{q}}\frac{q}{p\underline{a}_{ij}^0}\bigg[ (\bar{a}_{ij}^c)^p + \bigg(\sum\limits_{C_{kl}\in N_{h_1}(i,j)}({C_{ij}^{kl}}^+)^q\bigg)^{\frac{p}{q}}(2\kappa L_f+M_f)^p \\ & + \bigg(\sum\limits_{C_{kl}\in N_{h_2}(i,j)}({B_{ij}^{kl}}^+)^q\bigg)^{\frac{p}{q}} \bigg( (2\kappa L_g+M_g) \int_0^{\infty}|K_{ij}(u)|du\bigg)^{p}\bigg] \\ & +C_p\bigg(\frac{p-2}{2\underline{a}_{ij}^0}\bigg)^{\frac{p-2}{2}}\frac{q}{p\underline{a}_{ij}^0}\bigg(\sum\limits_{C_{kl}\in N_{h_3}(i,j)}({E_{ij}^{kl}}^+)^q\bigg)^{\frac{p}{q}} (L_{ij}^{\delta})^p\bigg\} < 1, \end{align*} |
and for p = 2 ,
\begin{align*} 0 < r^2: = & 16\max\limits_{ij\in\Lambda}\bigg\{\frac{1}{(\underline{a}_{ij}^0)^2} \bigg[ (\bar{a}_{ij}^c)^2 + \sum\limits_{C_{kl}\in N_{h_1}(i,j)}({C_{ij}^{kl}}^+)^2 (2\kappa L_f+M_f)^2+ \sum\limits_{C_{kl}\in N_{h_2}(i,j)}({B_{ij}^{kl}}^+)^2 \\ & \times\bigg((2\kappa L_g+M_g)\int_0^{\infty}|K_{ij}(u)|du\bigg)^2\bigg] + \frac{1}{2\underline{a}_{ij}^0} \sum\limits_{C_{kl}\in N_{h_3}(i,j)}({E_{ij}^{kl}}^+)^2 (L_{ij}^{\delta})^2\bigg\} < 1. \end{align*} |
(A4) For \frac{1}{p}+\frac{1}{q} = 1 ,
\begin{align*} 0 < \frac{q}{p\underline{a}^0}\rho^1 : = & 16^{p-1} \frac{q}{p\underline{a}^0} \max\limits_{ij\in\Lambda} \bigg\{ \bigg( \frac{p}{q\underline{a}_{ij}^0 }\bigg)^{\frac{p}{q}} \bigg[ (\bar{a}_{ij}^c )^p + \bigg( \sum\limits_{C_{kl}\in N_{h_1}(i,j)} ({C_{ij}^{kl}}^+)^q \bigg)^{\frac{p}{q}} \bigg[ 2^{p-1} (L_f )^p \notag\\ & \times \sum\limits_{C_{kl}\in N_{h_1}(i,j)} \frac{e^{\frac{p}{q}\underline{a}_{ij}^0 {\tau_{kl}}^+} (2\kappa)^p } {1-{\dot{\tau}}_{kl}^+ } +(M_f )^p \bigg] +\bigg( \sum\limits_{C_{kl}\in N_{h_2}(i,j) } \big( {B_{ij}^{kl}}^+ \big)^q \bigg)^{\frac{p}{q}} \bigg[ \bigg( 2\kappa L_g \\ & \times \int_0^{\infty} |K_{ij}(u)|du \bigg)^{p} + \bigg( M_g \int_0^\infty |K_{ij}(u)|du \bigg)^p \bigg] \bigg] + 2^{p-1} C_p \big(\frac{p-2}{2\underline{a}_{ij}^0} \big)^{\frac{p-2} {2} } \\ & \times \bigg( \sum\limits_{C_{kl}\in N_{h_3}(i,j)} ({E_{ij}^{kl}}^+ )^q \bigg)^{\frac{p}{q}} ( L_{ij}^{\delta})^p \frac{e^{\frac{p}{q}\underline{a}_{ij}^0 \sigma_{ij}^+ } } {1-\dot{\sigma}_{ij}^+ } \bigg\} < 1, \,\, (p > 2), \end{align*} |
\begin{align*} 0 < \frac{\rho^2}{\underline{a}^0}: = & \frac{32}{\underline{a}^0} \max\limits_{ij\in\Lambda} \bigg\{ \bigg(\frac{1}{\underline{a}_{ij}^0}\bigg) \sum\limits_{C_{kl}\in N_{h_1}(i,j)} ({C_{ij}^{kl}}^+)^2 \bigg[ (L_f )^2 \sum\limits_{C_{kl}\in N_{h_1}(i,j)}\frac{e^{\underline{a}_{ij}^0 {\tau_{kl}}^+} (2\kappa)^2 } {1-{\dot{\tau}}_{kl}^+ } +\frac{(M_f)^2}{2} \bigg] \\ & + \sum\limits_{C_{kl}\in N_{h_3}(i,j)} ({E_{ij}^{kl}}^+ )^2 ( L_{ij}^{\delta} )^2 \frac{e^{2\underline{a}_{ij}^0 \sigma_{ij}^+ } } {1-\dot{\sigma}_{ij}^+ } + \frac{1}{2\underline{a}_{ij}^0 } \sum\limits_{C_{kl}\in N_{h_2}(i,j) } \big( {B_{ij}^{kl}}^+ \big)^2 (4\kappa^2 L_g^2+M_g^2) \\ & \times \bigg( \int_0^{\infty} |K_{ij}(u)| du \bigg)^2 +\frac{(\bar{a}_{ij}^c)^2}{2\underline{a}_{ij}^0} \bigg\} < 1,\,\, (p = 2). \end{align*} |
(A5) The kernel K_{ij} is almost periodic and there exist constants M > 0 and u > 0 such that |K_{ij}(t)|\leq Me^{-ut} for all t\in \mathbb{R} .
Let \mathbb{X} indicate the space of all \mathcal{L}^p -bounded and \mathcal{L}^p -uniformly continuous stochastic processes from \mathbb{R} to \mathcal{L}^p(\Omega, \mathscr{A}^{m\times n}) , then with the norm \|\phi\|_{\mathbb{X}} = \sup\limits_{t\in\mathbb{R}}\big\{E\|\phi(t)\|_0^p\big\}^{\frac{1}{p}}, where \phi = (\phi_{11}, \phi_{12}, \ldots, \phi_{mn})\in \mathbb{X} , it is a Banach space.
Set \phi^0 = (\phi_{11}^0, \phi_{12}^0, \ldots, \phi_{mn}^0)^T , where \phi_{ij}^0(t) = \int_{-\infty}^te^{-\int_s^ta_{ij}^0(u)du}L_{ij}(s)ds, t\in\mathbb{R}, ij\in\Lambda . Then, \phi^0 is well defined under assumption (A2) . Consequently, we can take a constant \kappa such that \kappa\geq \|\phi^0\|_{\mathbb{X}} .
Definition 3.1. [37] An \mathscr{F}_t -progressively measurable stochastic process x(t) = (x_{11}(t), x_{12}(t), \ldots, x_{mn}(t))^T is called a solution of system (2.1), if x(t) solves the following integral equation:
\begin{align} x_{ij}(t) = & x_{ij}(t_0)e^{-\int_{t_0}^ta_{ij}^0(u)du}+\int_{t_0}^{t}e^{-\int_{s}^ta_{ij}^0(u)du}\bigg[ -a_{ij}^c(s)x_{ij}(s)+\sum\limits_{C_{kl}\in N_{h_1}(i,j)}C_{ij}^{kl}(s) \\ & \times f(x_{kl}(s-\tau_{kl}(s)))x_{ij}(s)+ \sum\limits_{C_{kl}\in N_{h_2}(i,j)}B_{ij}^{kl}(s)\int_{0}^{\infty}K_{ij}(u)g(x(s-u))dux_{ij}(s) \\ &+L_{ij}(s) \bigg]ds+\int_{t_0}^te^{-\int_{s}^ta_{ij}^0(u)du} \sum\limits_{C_{kl}\in N_{h_3}(i,j)}E_{ij}^{kl}(s)\delta_{ij}(x_{ij}(s-\sigma_{ij}(s)))dw_{ij}(s). \end{align} | (3.1) |
In (3.1), let t_0\rightarrow -\infty , then one gets
\begin{align} x_{ij}(t) = & \int_{-\infty}^{t}e^{-\int_{s}^ta_{ij}^0(u)du}\bigg[ -a_{ij}^c(s)x_{ij}(s)+\sum\limits_{C_{kl}\in N_{h_1}(i,j)}C_{ij}^{kl}(s)f(x_{kl}(s-\tau_{kl}(s)))x_{ij}(s) \\ & + \sum\limits_{C_{kl}\in N_{h_2}(i,j)}B_{ij}^{kl}(s)\int_{0}^{\infty}K_{ij}(u)g(x(s-u))dux_{ij}(s)+L_{ij}(s) \bigg]ds+\int_{-\infty}^te^{-\int_{s}^ta_{ij}^0(u)du} \\ & \times \sum\limits_{C_{kl}\in N_{h_3}(i,j)}E_{ij}^{kl}(s)\delta_{ij}(x_{ij}(s-\sigma_{ij}(s)))dw_{ij}(s), t\geq t_0,ij\in\Lambda. \end{align} | (3.2) |
It is easy to see that if x(t) solves (3.2), then it also solves (2.1).
Theorem 3.1. Assume that (A1) – (A4) hold. Then the system (2.1) has a unique \mathcal{L}^p -bounded and \mathcal{L}^p -uniformly continuous solution in \mathbb{X}^* = \{\phi\in\mathbb{X}:\|\phi-\phi^0\|_{\mathbb{X}}\leq \kappa\} , where \kappa is a constant satisfying \kappa\geq \|\phi^0\|_{\mathbb{X}} .
Proof. Define an operator \phi:\mathbb{X}^*\to \mathbb{X} as follows:
(\Psi\phi)(t) = ( (\Psi_{11}\phi)(t),(\Psi_{12}\phi)(t),\ldots,(\Psi_{mn}\phi)(t) )^T, |
where (\phi_{11}, \phi_{12}, \ldots, \phi_{mn})^T\in\mathbb{X} , t\in\mathbb{R} and
\begin{align} (\Psi_{ij}\phi)(t) = &\int_{-\infty}^{t}e^{-\int_{s}^ta_{ij}^0(u)du}\bigg[ -a_{ij}^c(s)\phi_{ij}(s)+ \sum\limits_{C_{kl}\in N_{h_1}(i,j)}C_{ij}^{kl}(s)f(\phi_{kl}(s-\tau_{kl}(s)))\phi_{ij}(s) \\ & + \sum\limits_{C_{kl}\in N_{h_2}(i,j)}B_{ij}^{kl}(s)\int_{0}^{\infty}K_{ij}(u)g(\phi_{kl}(s-u))du\phi_{ij}(s)+L_{ij}(s) \bigg]ds\\ &+\int_{-\infty}^te^{-\int_{s}^ta_{ij}^0(u)du} \sum\limits_{C_{kl}\in N_{h_3}(i,j)}E_{ij}^{kl}(s)\delta_{ij}(\phi_{ij}(s-\sigma_{ij}(s)))d\omega_{ij}(s), ij\in\Lambda. \end{align} | (3.3) |
First of all, let us show that E\|\Psi \phi(t)-\phi^0(t)\|_{0}^p\leq \kappa for all \phi\in \mathbb{X}^* .
Noticing that for any \phi\in\mathbb{X}^* , it holds
\|\phi\|_{\mathbb{X}}\leq \|\phi^0 \|_{\mathbb{X}}+\|\phi-\phi^0 \|_{\mathbb{X}}\leq 2\kappa. |
Then, we deduce that
\begin{align} & E\|\Psi \phi(t)-\phi^0(t)\|_{0}^p \\ \leq & 4^{p-1}\max\limits_{ij\in\Lambda} \bigg\{ E\bigg\|\int_{-\infty}^{t} -e^{-\int_{s}^ta_{ij}^0(u)du} a_{ij}^c(s)\phi_{ij}(s) \bigg\|_{\flat}^p \bigg\} +4^{p-1}\max\limits_{ij\in\Lambda}\bigg\{E\bigg\|\int_{-\infty}^{t} e^{-\int_{s}^ta_{ij}^0(u)du} \\ &\times \sum\limits_{C_{kl}\in N_{h_1}(i,j)}C_{ij}^{kl}(s) f(\phi_{kl}(s-\tau_{kl}(s)))\phi_{ij}(s)ds\bigg\|_{\flat}^p\bigg\} + 4^{p-1}\max\limits_{ij\in\Lambda} \bigg\{E\bigg\|\int_{-\infty}^{t} e^{-\int_{s}^ta_{ij}^0(u)du} \\ &\times \sum\limits_{C_{kl}\in N_{h_2}(i,j)}B_{ij}^{kl}(s)\int_{0}^{\infty}K_{ij}(u)g(\phi_{kl}(s-u))du\phi_{ij}(s)ds\bigg\|_{\flat}^p\bigg\}\\ & + 4^{p-1}\max\limits_{ij\in\Lambda} \bigg\{E\bigg\|\int_{-\infty}^{t} e^{-\int_{s}^ta_{ij}^0(u)du} \sum\limits_{C_{kl}\in N_{h_3}(i,j)}E_{ij}^{kl}(s)\delta_{ij}(\phi_{ij}(s-\sigma_{ij}(s)))d\omega_{ij}(s)\bigg\|_{\flat}^p\bigg\}\\ : = &F_1+F_2+F_3+F_4 . \end{align} | (3.4) |
By the Hölder inequality, we have
\begin{align} F_2\leq & 4^{p-1}\max\limits_{ij\in\Lambda}\bigg\{E\bigg\|\bigg[ \int_{-\infty}^te^{-\frac{q}{p}\int_s^ta_{ij}^0(u)du}ds \bigg]^{\frac{p}{q}} \bigg[\int_{-\infty}^te^{-\frac{p}{q}\int_s^ta_{ij}^0(u)du} \\ & \times\bigg(\sum\limits_{C_{kl}\in N_{h_1}(i,j)}C_{ij}^{kl}(s)f(\phi_{kl}(s-\tau_{kl}(s)))\phi_{ij}(s)\bigg)^pds\bigg]\bigg\|_{\flat}\bigg\} \\ \leq & 4^{p-1} \max\limits_{ij\in\Lambda}\bigg\{ \bigg(\frac{p}{q\underline{a}_{ij}^0}\bigg)^{\frac{p}{q}} E\bigg[ \int_{-\infty}^t e^{-\frac{p}{q}\int_{s}^ta_{ij}^0(u)du}\bigg(\sum\limits_{C_{kl}\in N_{h_1}(i,j)}(\|C_{ij}^{kl}(s)\|_{\flat})^q\bigg)^{\frac{p}{q}} \\ &\times\sum\limits_{ij\in\Lambda}(2\kappa L_f)^p\|\phi_{ij}(s)\|_{\flat}^pds \bigg] \bigg\} \\ \leq & 4^{p-1}\max\limits_{ij\in\Lambda}\bigg\{ \bigg(\frac{p}{q\underline{a}_{ij}^0}\bigg)^{\frac{p}{q}} \frac{q}{p\underline{a}_{ij}^0} \bigg(\sum\limits_{C_{kl}\in N_{h_1}(i,j)} ({C_{ij}^{kl}}^+)^q \bigg) ^{\frac{p}{q}} (2\kappa L_f )^p \bigg\}\|\phi\|_{\mathbb{X}}^{p}. \end{align} | (3.5) |
Similarly, one has
\begin{align} F_1 \leq & 4^{p-1}\max\limits_{ij\in\Lambda}\bigg\{\bigg(\frac{p}{q\underline{a}_{ij}^0}\bigg)^{\frac{p}{q}} \frac{ q}{p\underline{a}_{ij}^0} (\bar{a}_{ij}^c)^p \bigg\}\|\phi\|_{\mathbb{X}}^{p}, \end{align} | (3.6) |
\begin{align} F_3 \leq & 4^{p-1}\max\limits_{ij\in\Lambda}\bigg\{ \bigg(\frac{p}{q\underline{a}_{ij}^0}\bigg)^{\frac{p}{q}} \frac{ q}{p\underline{a}_{ij}^0} \bigg (\sum\limits_{C_{kl}\in N_{h_2}(i,j)} ({B_{ij}^{kl}}^+)^q \bigg ) ^{\frac{p}{q}} \bigg( 2\kappa L_g \int_0^\infty |K_{ij}(u)|du \bigg )^{p} \bigg \} \|\phi\|_{\mathbb{X}}^{p}. \end{align} | (3.7) |
By the Burkolder-Davis-Gundy inequality and the Hölder inequality, when p > 2 , we infer that
\begin{align} F_4\leq&4^{p-1}C_p\max\limits_{ij\in\Lambda} \bigg\{E\bigg[\int_{-\infty}^{t}\bigg\| e^{-\int_{s}^ta_{ij}^0(u)du}\sum\limits_{C_{kl}\in N_{h_3}(i,j)}E_{ij}^{kl}(s)\delta_{ij}(\phi_{ij}(s-\sigma_{ij}(s)))\bigg\|_\flat^2ds\bigg]^{\frac{p}{2}}\bigg\}\\ \leq & 4^{p-1} C_p \max\limits_{ij\in\Lambda}\bigg\{ E\bigg[ e^{-2\int_s^ta_{ij}^0(u)du} \bigg\|\sum\limits_{C_{kl}\in N_{h_3}(i,j)}E_{ij}^{kl}\delta_{ij}(\phi_{ij}(s-\sigma_{ij}(s)))\bigg\|_{\flat}^2ds \bigg]^{\frac{p}{2}} \bigg\} \\ \leq & 4^{p-1} C_p \max\limits_{ij\in\Lambda}\bigg \{ E\bigg [ \int_{-\infty}^t (e^{-2\int_s^ta_{ij}^0(u)du})^{\frac{p}{p-2}\times\frac{1}{p}}ds \bigg ]^{\frac{p-2}{p}\times\frac{p}{2}} \\ &\times E\bigg[ \int_{-\infty}^t (e^{-2\int_s^ta_{ij}^0(u)du})^{\frac{1}{q}\times\frac{p}{2}} \bigg( \bigg\|\sum\limits_{C_{kl}\in N_{h_3}(i,j)}E_{ij}^{kl}(s)\delta_{ij}\phi_{ij}(s-\sigma_{ij}(s))\bigg\|_{\flat}^2\bigg)^{\frac{p}{2}}ds \bigg] \bigg\} \\ \leq& 4^{p-1} C_p \max\limits_{ij\in\Lambda}\bigg\{ \bigg( \frac{p-2}{2\underline{a}_{ij}^0} \bigg)^{\frac{p-2}{2}} \frac{q}{p\underline{a}_{ij}^0} E\bigg\|\sum\limits_{C_{kl}\in N_{h_3}(i,j)}E_{ij}^{kl}(s)\delta_{ij}(\phi_{ij}(s-\sigma_{ij}(s)))\bigg\|_{\flat}^p\bigg\} \\ \leq & 4^{p-1} C_p \max\limits_{ij\in\Lambda}\bigg\{ \bigg( \frac{p-2}{2\underline{a}_{ij}^0} \bigg)^{\frac{p-2}{2}} \frac{q}{p\underline{a}_{ij}^0}\bigg( \sum\limits_{C_{kl}\in N_{h_3}(i,j)}({E_{ij}^{kl}}^+)^q \bigg)^{\frac{p}{q}} (L_{ij}^{\delta})^p \bigg\} \|\phi\|_{\mathbb{X}}^p. \end{align} | (3.8) |
When p = 2 , by the It \rm \hat{o} isometry, it follows that
\begin{align} F_4\leq & 4 \max\limits_{ij\in\Lambda}\bigg\{ E\bigg[ \int_{-\infty}^t e^{-2\int_s^ta_{ij}^0(u)du} \bigg\| \sum\limits_{C_{kl}\in N_{h_3}(i,j)}E_{ij}^{kl}(s)\delta_{ij}(\phi_{ij}(s-\sigma_{ij}(s)))\bigg\|_{\mathscr{A} }^2ds \bigg] \bigg\} \\ \leq & 4 \max\limits_{ij\in\Lambda}\bigg\{ \frac{1}{2\underline{a}_{ij}^0} \sum\limits_{C_{kl}\in N_{h_3}(i,j)}({E_{ij}^{kl}}^+)^2 (L_{ij}^{\delta})^2 \bigg\} \|\phi\|_{\mathbb{X}}^2. \end{align} | (3.9) |
Putting (3.5)–(3.9) into (3.4), we obtain that
\begin{align} \|\Psi\phi-\phi^0\|_{\mathbb{X}}^p \leq & 4^{p-1} \max\limits_{ij\in\Lambda}\bigg\{ \bigg(\frac{p}{q\underline{a}_{ij}^0}\bigg)^{\frac{p}{q}} \frac{q}{p\underline{a}_{ij}^0} \bigg[ (\bar{a}_{ij}^c)^p +\bigg(\sum\limits_{C_{kl}\in N_{h_1}(i,j)} ({C_{ij}^{kl}}^+)^q \bigg)^{\frac{p}{q}} (2\kappa L_f)^p \\ & + \bigg(\sum\limits_{C_{kl}\in N_{h_2}(i,j)} ({B_{ij}^{kl}}^+)^q \bigg) ^{\frac{p}{q}} \bigg(2\kappa L_g\int_0^\infty |K_{ij}(u)|du\bigg)^{p} \bigg] \\ & + C_p \bigg( \frac{p-2}{2\underline{a}_{ij}^0} \bigg)^{\frac{p-2}{2}} \frac{q}{p\underline{a}_{ij}^0} \bigg( \sum\limits_{C_{kl}\in N_{h_3}(i,j)}({E_{ij}^{kl}}^+)^q \bigg)^{\frac{p}{q}} (L_{ij}^{\delta})^p \bigg\}\|\phi\|_{\mathbb{X}}^{p} \leq \kappa^{p}, \,\,(p > 2), \end{align} | (3.10) |
and
\begin{align} \|\Psi\phi-\phi^0\|_{\mathbb{X}}^2 \leq & 4 \max\limits_{ij\in\Lambda}\bigg\{ \frac{1}{(a_{ij}^-)^2} \bigg[ (\bar{a}_{ij}^c)^2 + \sum\limits_{C_{kl}\in N_{h_1}(i,j)} ({C_{ij}^{kl}}^+)^2 (2\kappa L_f)^2 + \sum\limits_{C_{kl}\in N_{h_2}(i,j)} ({B_{ij}^{kl}}^+)^2 \bigg(2\kappa L_g \\ & \times\int_0^\infty |K_{ij}(u)|du\bigg)^2 \bigg] + \frac{1}{2\underline{a}_{ij}^0} \sum\limits_{C_{kl}\in N_{h_3}(i,j)}({E_{ij}^{kl}}^+)^2 (L_{ij}^{\delta})^2 \bigg\}\|\phi\|_{\mathbb{X}}^2 \leq \kappa^2, \,\, (p = 2). \end{align} | (3.11) |
It follows from (3.10), (3.11) and (A3) that \|\Psi\phi-\phi^0\|_{\mathbb{X}} \leq \kappa .
Then, using the same method as that in the proof of Theorem 3.2 in [21], we can show that \Psi\phi is \mathcal{L}^p -uniformly continuous. Therefore, we have \Psi({\mathbb{X}}^*) \subset \mathbb{X}^* .
Last, we will show that \Psi is a contraction mapping. Indeed, for any \psi, \varphi \in \mathbb{X}^* , when p > 2 , we have
\begin{align} & E\|(\Phi \varphi)(t)-(\Phi \psi)(t)\|_{0}^{p} \\ \leq & 4^{p-1} \max _{ij\in\Lambda} \bigg\{ E \bigg\| \int_{-\infty}^{t} e^{-\int_{s}^{t} a_{ij}^0(u) d u} (-a_{ij}^c(s)\varphi_{ij}(s)+a_{ij}^c(s)\psi_{ij}(s)) d s \bigg\|_{\flat}^{p} \bigg\} \\ &+4^{p-1} \max _{ij\in\Lambda} \bigg\{ E \bigg\| \int_{-\infty}^{t} e^{-\int_{s}^{t} a_{ij}^0(u) d u} \sum\limits_{C_{kl}\in N_{h_1}(i,j)} C_{i j}^{kl}(s) \bigg[f\big(\varphi_{kl}(s-\tau_{kl}(s)) \big)\varphi_{ij}(s) \\ & -f\big( \psi_{kl}(s-\tau_{kl}(s)) \big)\psi_{ij}(s) \bigg] d s \bigg\|_{\flat}^{p} \bigg\} +4^{p-1} \max _{ij\in\Lambda} \bigg\{E \bigg\| \int_{-\infty}^{t} e^{-\int_{s}^{t} a_{ij}^0(u) d u} \sum\limits_{C_{kl}\in N_{h_2}(i,j)} B_{i j}^{kl}(s) \\ & \times \bigg[ \int_0^{\infty} K_{ij}(u)g\big(\varphi_{kl}(s-u)\big) du\varphi_{ij}(s) -\int_0^{\infty} K_{ij}(u)g\big(\psi_{kl}(s-u)\big) du\psi_{ij}(u) \bigg] d s \bigg\|_{\flat}^{p}\bigg\} \\ & + 4^{p-1} \max _{ij\in\Lambda} \bigg\{E \bigg\| \int_{-\infty}^{t} e^{-\int_{s}^{t} a_{ij}^0(u) d u} \sum\limits_{C_{kl}\in N_{h_3}(i,j)} E_{i j}^{kl}(s)\bigg[\delta_{ij}\big(\varphi_{ij}(s-\sigma_{i j}(s))\big) \\ & -\delta_{ij}\big(\psi_{ij}(s-\sigma_{i j}(s))\big)\bigg] d \omega_{ij}(s) \bigg\|_{\flat}^{p}\bigg\} \\ \leq & 4^{p-1} \max\limits_{ij\in\Lambda} \bigg\{ \bigg( \frac{p}{q\underline{a}_{ij}^0} \bigg)^{\frac{p}{q}} \frac{q}{p\underline{a}_{ij}^0} \bigg[ (\bar{a}_{ij}^c)^p + \bigg( \sum\limits_{C_{kl}\in N_{h_1}(i,j)} ({C_{i j}^{kl}}^+)^q \bigg)^{\frac{p}{q}} (2\kappa L_f+M_f)^p \\ & + \bigg( \sum\limits_{C_{kl}\in N_{h_2}(i,j)} ({B_{i j}^{kl}}^+)^q \bigg)^{\frac{p}{q}} \bigg( (2\kappa L_g+M_g)\int_0^{\infty}|K_{ij}(u)|du \bigg)^p \bigg] + C_p \bigg( \frac{p-2}{2\underline{a}_{ij}^0} \bigg)^{\frac{p-2}{2}} \frac{q}{p\underline{a}_{ij}^0} \\ & \times \bigg(\sum\limits_{C_{kl}\in N_{h_3}(i,j)} ({E_{ij}^{kl}}^+)^q \bigg)^{\frac{p}{q}} (L_{ij}^{\delta})^p \bigg\} \| \varphi-\psi \|_{\mathbb{X}}^p. \end{align} | (3.12) |
Similarly, for p = 2 , we can get
\begin{align} & E\|(\Phi \varphi)(t)-(\Phi \psi)(t)\|_{0}^{2} \\ \leq & 4 \max\limits_{ij\in\Lambda} \bigg\{ \frac{1}{(\underline{a}_{ij}^0)^2} \bigg[ (\bar{a}_{ij}^c)^2 + \sum\limits_{C_{kl}\in N_{h_1}(i,j)} ({C_{i j}^{kl}}^+)^2 (2\kappa L_f+M_f)^2 + \sum\limits_{C_{kl}\in N_{h_2}(i,j)} ({B_{i j}^{kl}}^+)^2 \\ & \times \bigg( (2\kappa L_g+M_g)\int_0^{\infty}|K_{ij}(u)|du \bigg)^2 \bigg] + \frac{1}{2\underline{a}_{ij}^0} \sum\limits_{C_{kl}\in N_{h_3}(i,j)} ({E_{ij}^{kl}}^+)^2 (L_{ij}^{\delta})^2 \bigg\} \| \varphi-\psi \|_{\mathbb{X}}^2. \end{align} | (3.13) |
From (3.12) and (3.13) it follows that
\begin{align*} \|(\Phi \varphi)(t)-(\Phi \psi)(t)\|_{\mathbb{X}} \leq \sqrt[p]{r^1}\| \varphi- \psi \|_{\mathbb{X}}, (p > 2),\\ \|(\Phi \varphi)(t)-(\Phi \psi)(t)\|_{\mathbb{X}} \leq \sqrt{r^2}\| \varphi- \psi \|_{\mathbb{X}}, (p = 2). \end{align*} |
Hence, by virtue of (A3) , \Psi is a contraction mapping. So, \Psi has a unique fixed point x in \mathbb{X}^* , i.e., (2.1) has a unique solution x in \mathbb{X}^* .
Theorem 3.2. Assume that (A1) – (A5) hold. Then the system (2.1) has a unique p -th Stepanov-like almost periodic solution in the distribution sense in \mathbb{X}^* = \{\phi\in\mathbb{X}:\|\phi-\phi^0\|_{\mathbb{X}}\leq \kappa\} , where \kappa is a constant satisfying \kappa\geq \|\phi^0\|_{\mathbb{X}} .
Proof. From Theorem 3.1, we know that (2.1) has a unique solution x in \mathbb{X}^* . Now, let us show that x is Stepanov-like almost periodic in distribution. Since x\in \mathbb{X}^* , it is \mathcal{L}^p -uniformly continuous and satisfies \|x\|\leq 2\kappa . So, for any \varepsilon > 0 , there exists \delta\in (0, \varepsilon) , when |h| < \delta , we have \sup_{t\in\mathbb{R}} E\| x(t+h)-x(t) \|_0^p < \varepsilon . Hence, we derive that
\begin{align} \sup\limits_{\xi\in\mathbb{R}} \int_\xi^{\xi+1} E\| x(t+h)-x(t) \|_0^p dt < \varepsilon. \end{align} | (3.14) |
For the \delta above, according to (A2) , we have, for ij\in\Lambda ,
\begin{align*} & |a_{ij}^0(t+\tau)-a_{ij}^0(t)| < \delta,\; \; \|a_{ij}^c(t+\tau)-a_{ij}^c(t)\|_{\flat}^p < \delta, \; \; \|C_{ij}^{kl}(t+\tau)-C_{ij}^{kl}(t)\|_{\flat}^p < \delta,\; \; \\ &|\tau_{ij}(t+\tau)-\tau_{ij}(t)| < \delta,\; \; \|B_{ij}^{kl}(t+\tau)-B_{ij}^{kl}(t)\|_{\flat}^p < \delta, \; \; \|E_{ij}^{kl}(t+\tau)-E_{ij}^{kl}(t)\|_{\flat}^p < \delta,\; \; \\ & |\sigma_{ij}(t+\tau)-\sigma_{ij}(t)| < \delta,\; \; \sup\limits_{\xi\in\mathbb{R}}\int_\xi^{\xi+1} \| L_{ij}(t+\tau)-L_{ij}(t) \|_{\flat}^p dt < \delta. \end{align*} |
As |\tau_{ij}(t+\tau)-\tau_{ij}(t)| < \delta , by (3.14), there holds
\begin{align*} \sup\limits_{\xi\in\mathbb{R}}\int_\xi^{\xi+1} E\| x(s-\tau_{ij}(s+\tau))-x(s-\tau_{ij}(s)) \|_{0}^p ds < \varepsilon. \end{align*} |
Based on (3.2), we can infer that
\begin{align*} x_{ij}(t+\tau) = & \int_{-\infty}^{t}e^{-\int_{s}^ta_{ij}^0(u+\tau)du}\bigg[ -a_{ij}^c(s+\tau)x_{ij}(s+\tau) +\sum\limits_{C_{kl}\in N_{h_1}(i,j)}C_{ij}^{kl}(s+\tau) \notag \\ & \times f(x_{kl}(s+\tau-\tau_{kl}(s+\tau))) x_{ij}(s+\tau)+ \sum\limits_{C_{kl}\in N_{h_2}(i,j)}B_{ij}^{kl}(s+\tau)\int_{0}^{\infty}K_{ij}(u) \notag\\ & \times g(x_{kl}(s+\tau-u))dux_{ij}(s+\tau) +L_{ij}(s+\tau) \bigg]ds +\int_{-\infty}^te^{-\int_{s}^ta_{ij}(u+\tau)du} \notag\\ & \times \sum\limits_{C_{kl}\in N_{h_3}(i,j)}E_{ij}^{kl}(s+\tau)\delta_{ij}(x_{ij}(s+\tau-\sigma_{ij}(s+\tau)))d[\omega_{ij}(s+\tau) -\omega_{ij}(\tau) ], \end{align*} |
in which ij\in\Lambda, \omega_{ij}(s+\tau)-\omega_{ij}(\tau) is a Brownian motion having the same distribution as \omega_{ij}(s) .
Let us consider the process
\begin{align} x_{ij}(t+\tau) = & \int_{-\infty}^{t}e^{-\int_{s}^ta_{ij}^0(u+\tau)du}\bigg[ -a_{ij}^c(s+\tau)x_{ij}(s+\tau) +\sum\limits_{C_{kl}\in N_{h_1}(i,j)}C_{ij}^{kl}(s+\tau) \\ & \times f(x_{kl}(s+\tau-\tau_{kl}(s+\tau))) x_{ij}(s+\tau)+ \sum\limits_{C_{kl}\in N_{h_2}(i,j)}B_{ij}^{kl}(s+\tau)\int_{0}^{\infty}K_{ij}(u) \\ & \times g(x_{kl}(s+\tau-u))dux_{ij}(s+\tau) +L_{ij}(s+\tau) \bigg]ds +\int_{-\infty}^te^{-\int_{s}^ta_{ij}(u+\tau)du} \\ & \times \sum\limits_{C_{kl}\in N_{h_3}(i,j)}E_{ij}^{kl}(s+\tau)\delta_{ij}(x_{ij}(s+\tau-\sigma_{ij}(s+\tau)))d\omega_{ij}(s). \end{align} | (3.15) |
From (3.2) and (3.15), we deduce that
\begin{align} & \int_\xi^{\xi+1} E\| x(t+\tau)-x(t) \|_0^p dt \\ \leq & 16^{p-1} \max\limits_{ij\in\Lambda} \bigg\{ \int_\xi^{\xi+1} E \bigg\| \int_{-\infty}^t e^{-\int_s^t a_{ij}^0(u+\tau)du} \sum\limits_{C_{kl}\in N_{h_1}(i,j)} C_{ij}^{kl}(s+\tau) \\ & \times \bigg[f(x_{kl}(s+\tau-\tau_{kl}(s+\tau)))x_{ij}(s+\tau)-f(x_{kl}(s-\tau_{kl}(s)))x_{ij}(s+\tau)\bigg] ds\bigg\|_{\flat}^p dt \bigg\} \\ &+ 16^{p-1} \max\limits_{ij\in\Lambda} \bigg\{ \int_\xi^{\xi+1} E \bigg\| \int_{-\infty}^t e^{-\int_s^t a_{ij}^0(u+\tau)du} \sum\limits_{C_{kl}\in N_{h_1}(i,j)} \big(C_{ij}^{kl}(s+\tau) - C_{ij}^{kl}(s) \big) \\ & \times f(x_{kl}(s-\tau_{kl}(s)))x_{ij}(s+\tau) ds\bigg\|_{\mathscr{A} }^p dt \bigg\} + 16^{p-1} \max\limits_{ij\in\Lambda} \bigg\{ \int_\xi^{\xi+1} E \bigg\| \int_{-\infty}^t e^{-\int_s^t a_{ij}^0(u+\tau)du} \\ &\sum\limits_{C_{kl}\in N_{h_1}(i,j)} C_{ij}^{kl}(s) \times f(x_{kl}(s-\tau_{kl}(s)))\big( x_{ij}(s+\tau) - x_{ij}(s) \big)ds\bigg\|_{\flat}^p dt \bigg\} \\ & + 16^{p-1} \max\limits_{ij\in\Lambda} \bigg\{ \int_\xi^{\xi+1} E \bigg\| \int_{-\infty}^t \big(e^{-\int_s^t a_{ij}^0(u+\tau)du} - e^{-\int_s^t a_{ij}^0(u)du} \big) \sum\limits_{C_{kl}\in N_{h_1}(i,j)} C_{ij}^{kl}(t) \\ & \times f(x_{kl}(s-\tau_{kl}(s)))x_{ij}(s) ds\bigg\|_{\flat}^p dt \bigg\} + 16^{p-1} \max\limits_{ij\in\Lambda} \bigg\{ \int_\xi^{\xi+1} E \bigg\| \int_{-\infty}^t e^{-\int_s^t a_{ij}^0(u+\tau)du} \\ &\sum\limits_{C_{kl}\in N_{h_2}(i,j)} B_{ij}^{kl}(s+\tau) \bigg[\int_0^{\infty}K_{ij}(u) g(x_{kl}(s+\tau-u)) du x_{ij}(s+\tau) - \int_0^{\infty}K_{ij}(u) \\ & \times g(x_{kl}(s-u)) du x_{ij}(s+\tau)\bigg] ds\bigg\|_{\flat}^p dt \bigg\} + 16^{p-1} \max\limits_{ij\in\Lambda} \bigg\{ \int_\xi^{\xi+1} E \bigg\| \int_{-\infty}^t e^{-\int_s^t a_{ij}^0(u+\tau)du} \\ & \sum\limits_{C_{kl}\in N_{h_2}(i,j)} \big(B_{ij}^{kl}(s+\tau) - B_{ij}^{kl}(s) \big) \int_0^{\infty}K_{ij}(u) g(x_{kl}(s-u)) du x_{ij}(s+\tau) ds\bigg\|_{\flat}^p dt \bigg\} \\ &+ 16^{p-1} \max\limits_{ij\in\Lambda} \bigg\{ \int_\xi^{\xi+1} E \bigg\| \int_{-\infty}^t e^{-\int_s^t a_{ij}^0(u+\tau)du} \sum\limits_{C_{kl}\in N_{h_2}(i,j)} B_{ij}^{kl}(s) \int_0^{\infty}K_{ij}(u) g(x_{kl}(s-u)) du \\ & \times \big( x_{ij}(s+\tau) - x_{ij}(s) \big)ds\bigg\|_{\flat}^p dt \bigg\} + 16^{p-1} \max\limits_{ij\in\Lambda} \bigg\{ \int_\xi^{\xi+1} E \bigg\| \int_{-\infty}^t \big(e^{-\int_s^t a_{ij}^0(u+\tau)du} \\ & - e^{-\int_s^t a_{ij}^0(u)du} \big) \sum\limits_{C_{kl}\in N_{h_2}(i,j)} B_{ij}^{kl}(s) \int_0^{\infty}K_{ij}(u) g(x_{kl}(s-u)) du x_{ij}(s) ds\bigg\|_{\flat}^p dt \bigg\} \\ & + 16^{p-1} \max\limits_{ij\in\Lambda} \bigg\{ \int_\xi^{\xi+1} E \bigg\| \int_{-\infty}^t e^{-\int_s^t a_{ij}^0(u+\tau)du} (L_{ij}(s+\tau) - L_{ij}(s)) ds \bigg\|_{\flat}^p dt \bigg\} \\ & + 16^{p-1} \max\limits_{ij\in\Lambda} \bigg\{ \int_\xi^{\xi+1} E \bigg\| \int_{-\infty}^t \big(e^{-\int_s^t a_{ij}^0(u+\tau)du} - e^{-\int_s^t a_{ij}^0(u)du} \big) L_{ij}(s) ds \bigg\|_{\flat}^p dt \bigg\} \\ & + 16^{p-1} \max\limits_{ij\in\Lambda} \bigg\{ \int_\xi^{\xi+1} E \bigg\| \int_{-\infty}^t e^{-\int_s^t a_{ij}(u+\tau)du} \sum\limits_{C_{kl}\in N_{h_3}(i,j)} E_{ij}^{kl}(s+\tau) \\ & \times \bigg[ \delta_{ij}(x_{ij}(s+\tau-\sigma_{ij}(s+\tau)))- \delta_{ij}(x_{ij}(s-\sigma_{ij}(s))) \bigg] d\omega_{ij}(s) \bigg\|_{\mathscr{A} }^p dt \bigg\} \\ & + 16^{p-1} \max\limits_{ij\in\Lambda} \bigg\{ \int_\xi^{\xi+1} E \bigg\| \int_{-\infty}^t e^{-\int_s^t a_{ij}^0(u+\tau)du} \sum\limits_{C_{kl}\in N_{h_3}(i,j)} \big(E_{ij}^{kl}(s+\tau) - E_{ij}^{kl}(s) \big) \\ & \times \delta_{ij}(x_{ij}(s-\sigma_{ij}(s))) d\omega_{ij}(s) \bigg\|_{\flat}^p dt \bigg\} + 16^{p-1} \max\limits_{ij\in\Lambda} \bigg\{ \int_\xi^{\xi+1} E \bigg\| \int_{-\infty}^t \big(e^{-\int_s^t a_{ij}^0(u+\tau)du} \\ & - e^{-\int_s^t a_{ij}^0(u)du} \big) \sum\limits_{C_{kl}\in N_{h_3}(i,j)} E_{ij}^{kl}(t) \delta_{ij}(x_{ij}(s-\sigma_{ij}(s))) d\omega_{ij}(s) \bigg\|_{\flat}^p dt \bigg\} \\ & + 16^{p-1} \max\limits_{ij\in\Lambda} \bigg\{ \int_\xi^{\xi+1} E \bigg\| \int_{-\infty}^t e^{-\int_s^t a_{ij}^0(u+\tau)du} \big( a_{ij}^c(s)-a_{ij}^c(s+\tau) \big)x_{ij}(s+\tau) ds \bigg\|_{\flat}^p dt \bigg\} \\ & + 16^{p-1} \max\limits_{ij\in\Lambda} \bigg\{ \int_\xi^{\xi+1} E \bigg\| \int_{-\infty}^t e^{-\int_s^t a_{ij}(u+\tau)du} a_{ij}^c(s)\big( x_{ij}(s)-x_{ij}(s+\tau) \big) ds \bigg\|_{\flat}^p dt \bigg\} \\ & + 16^{p-1} \max\limits_{ij\in\Lambda} \bigg\{ \int_\xi^{\xi+1} E \bigg\| \int_{-\infty}^t \big(e^{-\int_s^t a_{ij}(u+\tau)du} - e^{-\int_s^t a_{ij}(u)du} \big) (-a_{ij}^c(s))x_{ij}(s) ds \bigg\|_{\flat}^p dt \bigg\} \\ : = & \sum\limits_{i = 1}^{16} H_i. \end{align} | (3.16) |
Employing the Hölder inequality, we can obtain
\begin{align*} H_1 \leq & 32^{p-1} \max\limits_{ij\in\Lambda} \bigg\{ \bigg( \frac{p}{q\underline{a}_{ij}^0} \bigg)^{\frac{p}{q}} \bigg( \sum\limits_{C_{kl}\in N_{h_1}(i,j)} ({C_{ij}^{kl}}^+)^q \bigg)^{\frac{p}{q}} (L_f )^p \sum\limits_{C_{kl}\in N_{h_1}(i,j)} \int_\xi^{\xi+1} \bigg[ \int_{-\infty}^t e^{-\frac{p}{q}(t-s)\underline{a}_{ij}^0} \notag \\ & \times E \big\| \big [x(s+\tau-\tau_{kl}(s+\tau) ) - x(s-\tau_{kl}(s+\tau) ) \big] x(t+\tau) \big\|_0^p ds \notag\\ & + \int_{-\infty}^t e^{-\frac{p}{q}(t-s)\underline{a}_{ij}^0} E \big\| \big[ x(s-\tau_{kl}(s+\tau) ) - x(s-\tau_{kl}(s) ) \big] x(t+\tau) \big\|_0^p ds \bigg] dt \bigg\}. \end{align*} |
By a change of variables and Fubini's theorem, we infer that
\begin{align} H_1 \leq & 32^{p-1} \max\limits_{ij\in\Lambda} \bigg\{ \bigg( \frac{p}{q\underline{a}_{ij}^0} \bigg)^{\frac{p}{q}} \bigg( \sum\limits_{C_{kl}\in N_{h_1}(i,j)} ({C_{ij}^{kl}}^+)^q \bigg)^{\frac{p}{q}} (L_f )^p \\ & \times \sum\limits_{C_{kl}\in N_{h_1}(i,j)} \int_\xi^{\xi+1} \bigg[ \int_{-\infty}^{t-\tau_{kl}(t+\tau)} \frac{1 }{1-{\dot{\tau}}_{kl}(s+\tau) } e^{-\frac{p}{q}}\underline{a}_{ij}^0(t-u-\tau_{kl}(s+\tau) ) \\ & \times E \big\| \big [x(u+\tau) - x(u) \big] x(t+\tau) \big\|_0^p du + \frac{q{\varepsilon}^p (2\kappa)^p } {p\underline{a}_{ij}^0 } \bigg] dt \bigg\} \\ \leq & 32^{p-1} \max\limits_{ij\in\Lambda} \bigg\{ \bigg( \frac{p}{q\underline{a}_{ij}^0} \bigg)^{\frac{p}{q}} \bigg( \sum\limits_{C_{kl}\in N_{h_1}(i,j)} ({C_{ij}^{kl}}^+)^q \bigg)^{\frac{p}{q}} (L_f )^p \sum\limits_{C_{kl}\in N_{h_1}(i,j)}\frac{e^{\frac{p}{q}}\underline{a}_{ij}^0 {\tau_{kl}}^+ (2\kappa)^p } {1-{\dot{\tau}}_{kl}^+ } \\ & \times \int_{-\infty}^{\xi} e^{-\frac{p}{q}(\xi-s)\underline{a}_{ij}^0} \bigg( \int_{s}^{s+1} E \big\| x(t+\tau) - x(t) \big\|_0^p dt \bigg) ds \bigg\} + \bigtriangleup_{H_1} \varepsilon , \end{align} | (3.17) |
where
\begin{align*} \bigtriangleup_{H_1} = 32^{p-1} \max\limits_{ij\in\Lambda} \bigg\{ \frac{q (2\kappa)^p } {p\underline{a}_{ij}^0 } \bigg( \frac{p}{q\underline{a}_{ij}^0} \bigg)^{\frac{p}{q}} \bigg( \sum\limits_{C_{kl}\in N_{h_1}(i,j)} ({C_{ij}^{kl}}^+)^q \bigg)^{\frac{p}{q}} (L_f )^p {\varepsilon}^{p-1} \bigg\} . \end{align*} |
Similarly, when p > 2 , one can obtain
\begin{align} H_{11} \leq & 16^{p-1} C_p \max\limits_{ij\in\Lambda} \bigg\{ \int_\xi^{\xi+1} E \bigg[ \int_{-\infty}^t e^{-2\int_s^t a_{ij}^0(u+\tau)du} E \bigg\| \sum\limits_{C_{kl}\in N_{h_2}(i,j)} E_{ij}^{kl}(s+\tau) \\ & \times \big( \delta_{ij}(x_{ij}(s+\tau-\sigma_{ij}(s+\tau)))- \delta_{ij}(x_{ij}(s-\sigma_{ij}(s))) \big) \bigg\|_{\flat}^2 ds \bigg]^{\frac{p}{2}} dt \bigg\} \\ \leq & 32^{p-1} C_p \max\limits_{ij\in\Lambda} \bigg\{ \big(\frac{p-2}{2\underline{a}_{ij}^0} \big)^{\frac{p-2} {2} } \big( \sum\limits_{C_{kl}\in N_{h_2}(i,j)} ({E_{ij}^{kl}}^+ )^q \big)^{\frac{p}{q}} ( L_{ij}^{\delta})^p \frac{e^{\frac{p}{q}\underline{a}_{ij}^0 \sigma_{ij}^+ } } {1-\dot{\sigma}_{ij}^+ } \int_{-\infty}^{\xi} e^{-\frac{p}{q}(\xi-s)\underline{a}_{ij}^0 } \\ & \times \bigg( \int_s^{s+1} E \big\| x(t+\tau) - x(t) \big\|_0^p dt \bigg) ds\bigg\} +\bigtriangleup_{H_{11}^1 } \varepsilon, \end{align} | (3.18) |
where
\begin{align*} \bigtriangleup_{H_{11}^1} = 32^{p-1} C_p \max\limits_{ij\in\Lambda} \bigg\{ \big(\frac{p-2}{2\underline{a}_{ij}^0} \big)^{\frac{p-2} {2} } \big( \sum\limits_{C_{kl}\in N_{h_3}(i,j)} ({E_{ij}^{kl}}^+ )^q \big)^{\frac{p}{q}} \sum\limits_{C_{kl}\in N_{h_3}(i,j)}( L_{ij}^{\delta})^p \frac{q}{p\underline{a}_{ij}^0} {\varepsilon}^{p-1} \bigg\} , \end{align*} |
\begin{align} H_{12} \leq & 16^{p-1} C_p \max\limits_{ij\in\Lambda} \bigg\{ \int_\xi^{\xi+1} E \bigg[ \int_{-\infty}^t e^{-2\int_s^t a_{ij}^0(u+\tau)du} E \bigg\| \sum\limits_{C_{kl}\in N_{h_3}(i,j)} \big( E_{ij}^{kl}(s+\tau) - E_{ij}^{kl}(s) \big) \\ & \times \delta_{ij}(x_{ij}(s-\sigma_{ij}(s))) \bigg\|_{\mathscr{A} }^2 ds \bigg]^{\frac{p}{2}} dt \bigg\} \\ \leq & 16^{p-1} C_p \max\limits_{ij\in\Lambda} \bigg\{ \big(\frac{p}{q\underline{a}_{ij}^0} \big)^{\frac{p} {q} } \frac{q}{p\underline{a}_{ij}^0} ( L_{ij}^{\delta})^p \frac{e^{\frac{p}{q}\underline{a}_{ij}^0 \sigma_{ij}^+ } } {1-\dot{\sigma}_{ij}^+ } (2\kappa)^p \bigg\} \varepsilon^{\frac{p}{q}} : = \bigtriangleup_{H_{12}^1} \varepsilon , \end{align} | (3.19) |
and when p = 2 , we have
\begin{align} H_{11} \leq & 16 \max\limits_{ij\in\Lambda} \bigg\{ \int_\xi^{\xi+1} E \bigg[ \int_{-\infty}^t e^{-2\int_s^t a_{ij}^0(u+\tau)du} \sum\limits_{C_{kl}\in N_{h_3}(i,j)} ({E_{ij}^{kl}}^+)^2 \\ & \times \sum\limits_{C_{kl}\in N_{h_3}(i,j)} ( L_{ij}^{\delta} )^2 \| x_{ij}(s+\tau-\sigma_{ij}(s+\tau) ) - x_{ij}(s-\sigma_{ij}(s) ) \|_{\flat}^2 ds \bigg] dt \bigg\} \\ \leq & 32 \max\limits_{ij\in\Lambda} \bigg\{ \sum\limits_{C_{kl}\in N_{h_3}(i,j)} ({E_{ij}^{kl}}^+)^2 ( L_{ij}^{\delta} )^2 \frac{e^{2\underline{a}_{ij}^0 \sigma_{ij}^+} }{1-\dot{\sigma}_{ij}^+ } \int_{-\infty}^{\xi} e^{-2(\xi-s)\underline{a}_{ij}^0 } \\ & \times \bigg( \int_{s}^{s+1} E \| x(t+\tau) - x(t) \|_0^2 dt \bigg) ds \bigg\} + \bigtriangleup_{H_{11}^2} \varepsilon, \end{align} | (3.20) |
where
\begin{align*} \bigtriangleup_{H_{11}^2} = \frac{32}{\underline{a}_{ij}^0} \max\limits_{ij\in\Lambda} \bigg\{ \sum\limits_{C_{kl}\in N_{h_3}(i,j)} ({E_{ij}^{kl}}^+)^2 \sum\limits_{C_{kl}\in N_{h_3}(i,j)} ( L_{ij}^{\delta} )^2 {\varepsilon} \bigg\} , \end{align*} |
\begin{align} H_{12} \leq & 16 \max\limits_{ij\in\Lambda} \bigg\{ \frac{1}{(\underline{a}_{ij}^0)^2} ( L_{ij}^{\delta})^2 \frac{e^{\underline{a}_{ij}^0 \sigma_{ij}^+ } } {1-\dot{\sigma}_{ij}^+ } 4\kappa^2 \bigg\} \varepsilon : = \bigtriangleup_{H_{12}^2} \varepsilon. \end{align} | (3.21) |
In the same way, we can get
\begin{align} H_2 \leq & 16^{p-1} \max\limits_{ij\in\Lambda} \bigg\{ ( \frac{mnp}{q\underline{a}_{ij}^0 })^{\frac{p}{q}} \frac{q}{p \underline{a}_{ij}^0 } (2\kappa M_f )^p \bigg\} \varepsilon^{\frac{p}{q} } : = \bigtriangleup_{H_{2}} \varepsilon, \end{align} | (3.22) |
\begin{align} H_3 \leq & 16^{p-1} \max\limits_{ij\in\Lambda} \bigg\{ ( \frac{p}{q\underline{a}_{ij}^0 })^{\frac{p}{q}} \bigg ( \sum\limits_{C_{kl}\in N_{h_1}(i,j) } \big( {C_{ij}^{kl}}^+ \big)^q \bigg)^{\frac{p}{q}} (M_f )^p \int_{-\infty}^\xi e^{-\frac{p}{q} (\xi-s) \underline{a}_{ij}^0} \\ & \times \bigg(\int_s^{s+1} E \| x(t+\tau) - x(t) \|_0^p dt \bigg) ds \bigg\}, \end{align} | (3.23) |
\begin{align} H_5 \leq & 16^{p-1} \max\limits_{ij\in\Lambda} \bigg\{ \bigg( \frac{p}{q\underline{a}_{ij}^0 } \bigg)^{\frac{p}{q}} \bigg( \sum\limits_{C_{kl}\in N_{h_2}(i,j) } \big( {B_{ij}^{kl}}^+ \big)^q \bigg)^{\frac{p}{q}} \bigg( 2\kappa L_g\int_0^{\infty} |K_{ij}(u)| du \bigg)^p \int_{-\infty}^\xi e^{-\frac{p}{q} (\xi-s) \underline{a}_{ij}^0} \\ & \times \bigg(\int_s^{s+1} E \| x(t+\tau) - x(t) \|_0^p dt \bigg) ds\bigg\}, \end{align} | (3.24) |
\begin{align} H_6 \leq & 16^{p-1} \max\limits_{ij\in\Lambda} \bigg\{ ( \frac{mnp}{q\underline{a}_{ij}^0 })^{\frac{p}{q}} \frac{q}{p \underline{a}_{ij}^0 } \bigg( (2\kappa M_g )\int_0^{\infty}|K_{ij}(u)|du \bigg)^p \bigg\} \varepsilon^{\frac{p}{q} } : = \bigtriangleup_{H_{6}} \varepsilon, \end{align} | (3.25) |
\begin{align} H_7 \leq & 16^{p-1} \max\limits_{ij\in\Lambda} \bigg\{ ( \frac{p}{q\underline{a}_{ij}^0 })^{\frac{p}{q}} \bigg( \sum\limits_{C_{kl}\in N_{h_2}(i,j) } \big( {B_{ij}^{kl}}^+ \big)^q \bigg)^{\frac{p}{q}} \big( M_g\int_0^\infty |K_{ij}(u)|du \big)^p \int_{-\infty}^\xi e^{-\frac{p}{q} (\xi-s) \underline{a}_{ij}^0} \\ & \times \bigg(\int_s^{s+1} E \| x(t+\tau) - x(t) \|_0^p dt \bigg) ds \bigg\}, \end{align} | (3.26) |
\begin{align} H_9 \leq & 16^{p-1} \max\limits_{ij\in\Lambda} \bigg\{ \big( \frac{p}{q \underline{a}_{ij}^0}\big)^{\frac{p}{q}} \frac{q}{p \underline{a}_{ij}^0} \bigg\} \varepsilon^{p } : = \bigtriangleup_{H_{9}} \varepsilon, \end{align} | (3.27) |
\begin{align} H_{14} \leq & 16^{p-1} \max\limits_{ij\in\Lambda} \bigg\{ \big( \frac{p}{q \underline{a}_{ij}^0}\big)^{\frac{p}{q}} \frac{q}{p \underline{a}_{ij}^0} (2\kappa)^p \bigg\} \varepsilon^{p } : = \bigtriangleup_{H_{14}} \varepsilon, \end{align} | (3.28) |
\begin{align} H_{15} \leq & 16^{p-1} \max\limits_{ij\in\Lambda} \bigg\{ ( \frac{p}{q\underline{a}_{ij}^0 })^{\frac{p}{q}} (\bar{a}_{ij}^c )^p \int_{-\infty}^\xi e^{-\frac{p}{q} (\xi-s) \underline{a}_{ij}^0} \bigg(\int_s^{s+1} E \| x(t+\tau) - x(t) \|_0^p dt \bigg) ds \bigg\}. \end{align} | (3.29) |
Noting that
\begin{align} &\bigg[ \int_{-\infty}^t \big| e^{-\int_s^t a_{ij}^0(u+\tau)du } - e^{-\int_s^t a_{ij}^0(u)du} \big|^{\frac{q}{p}} ds \bigg]^{\frac{p}{q}} \\ \leq & \bigg[ \int_{-\infty}^t e^{-\frac{q}{p}\underline{a}_{ij}^0 (t-s) } \bigg( \int_s^t | a_{ij}^0(u+\tau) -a_{ij}^0(u) | du \bigg)^{\frac{q}{p}} ds \bigg]^{\frac{p}{q}} \leq \bigg( \Gamma\big(\frac{q+p}{p}\big) \bigg)^{\frac{p}{q}} \bigg( \frac{p}{q\underline{a}_{ij}^0} \bigg)^{\frac{p+q}{q}} \varepsilon . \end{align} | (3.30) |
We can gain
\begin{align} H_4 \leq & 16^{p-1} \max\limits_{ij\in\Lambda} \bigg\{ \bigg( \Gamma\big(\frac{q+p}{p} \big) \bigg)^{\frac{p}{q}} \Gamma\big( \frac{q+p}{p} \big) \big(\frac{1}{\underline{a}_{ij}^0}\big)^{\frac{2(p+q)}{q}} \\ & \times \bigg( \sum\limits_{C_{kl}\in N_{h_1}(i,j)} ({C_{ij}^{kl}}^+)^q \bigg)^{\frac{p}{q}} (2\kappa M_f )^{p} \bigg\} \varepsilon^{\frac{p}{q}+1} : = \bigtriangleup_{H_{4}} \varepsilon, \end{align} | (3.31) |
\begin{align} H_8 \leq & 16^{p-1} \max\limits_{ij\in\Lambda} \bigg\{ \bigg( \Gamma\big(\frac{q+p}{p} \big) \bigg)^{\frac{p}{q}} \Gamma\big( \frac{q+p}{p} \big) \big(\frac{1}{\underline{a}_{ij}^0}\big)^{\frac{2(p+q)}{q}} \\ & \times \bigg( \sum\limits_{C_{kl}\in N_{h_2}(i,j)} ({B_{ij}^{kl}}^+)^q \bigg)^{\frac{p}{q}} (2\kappa M_g\int_0^{\infty} |K_{ij}(u)|du )^p \bigg\} \varepsilon^{\frac{p}{q}+1} : = \bigtriangleup_{H_{8}} \varepsilon, \end{align} | (3.32) |
\begin{align} H_{10} \leq & 16^{p-1} \max\limits_{ij\in\Lambda} \bigg\{ \bigg( \Gamma\big(\frac{q+p}{p} \big) \bigg)^{\frac{p}{q}} \Gamma\big( \frac{q+p}{p} \big) \big(\frac{1}{\underline{a}_{ij}^0}\big)^{\frac{2(p+q)}{q}} (M_L)^p \bigg\} \varepsilon^{\frac{p}{q}+1} : = \bigtriangleup_{H_{10}} \varepsilon, \end{align} | (3.33) |
\begin{align} H_{16} \leq & 16^{p-1} \max\limits_{ij\in\Lambda} \bigg\{ \bigg( \Gamma\big(\frac{q+p}{p} \big) \bigg)^{\frac{p}{q}} \Gamma\big( \frac{q+p}{p} \big) \big(\frac{1}{\underline{a}_{ij}^0}\big)^{\frac{2(p+q)}{q}} (2\kappa \bar{a}_{ij}^c)^p \bigg\} \varepsilon^{\frac{p}{q}+1} : = \bigtriangleup_{H_{16}} \varepsilon, \end{align} | (3.34) |
when p > 2 , we have
\begin{align} H_{13} \leq & 16^{p-1} C_p \max\limits_{ij\in\Lambda} \bigg\{ \int_\xi^{\xi+1} E \bigg[ \int_{-\infty}^t \bigg( e^{-\int_s^t a_{ij}^0(u+\tau)du } - e^{-\int_s^t a_{ij}^0(u)du} \bigg)^2 \\ & \times \sum\limits_{C_{kl}\in N_{h_3}(i,j)} E_{ij}^{kl}(s) \| \delta_{ij}(x_{ij}(s-\sigma_{ij}(s))) \|_{\flat}^2 ds \bigg]^{\frac{p}{2}} dt \bigg\} \\ \leq & 16^{p-1} C_p \max\limits_{ij\in\Lambda} \bigg\{ \bigg( \Gamma\big(\frac{p}{p-2} \big) \bigg)^{\frac{p-2}{2}} \big( \frac{p-2}{2\underline{a}_{ij}^0} \big)^{\frac{p}{2}} \\ & \times \frac{q}{p\underline{a}_{ij}^0} \bigg( \sum\limits_{C_{kl}\in N_{h_3}(i,j)} ({E_{ij}^{kl}}^+)^q \bigg)^{\frac{p}{q}} (L_{ij}^{\delta})^p \frac{e^{\frac{p}{q}\underline{a}_{ij}^0 \sigma_{ij}^+} } {1-{\dot{\sigma}}_{ij}^+} (2\kappa)^{p} \bigg\} \varepsilon : = \bigtriangleup_{H_{13}^1} \varepsilon, \end{align} | (3.35) |
for p = 2 , we get
\begin{align} H_{13} \leq & 16 \max\limits_{ij\in\Lambda} \bigg\{ \sum\limits_{C_{kl}\in N_{h_3}(i,j)} ({E_{ij}^{kl}}^+)^2 (L_{ij}^{\delta})^2 \frac{e^{2\underline{a}_{ij}^0 \sigma_{ij}^+} } {1-{\dot{\sigma}}_{ij}^+} \frac{ \Gamma(3) }{8(\underline{a}_{ij}^0)^3} (2\kappa)^{2} \bigg\} \varepsilon : = \bigtriangleup_{H_{13}^2} \varepsilon . \end{align} | (3.36) |
Substituting (3.17)–(3.36) into (3.16), we have the following two cases:
Case 1. When p > 2 , we have
\begin{align*} & \int_\xi^{\xi+1} E\| x(t+\tau) -x(t) \|_0^p dt \\ \leq & H^1 \varepsilon + \rho^1 \int_{-\infty}^\xi e^{-(\xi-s)\frac{p}{q}\underline{a}^0}\bigg( \int_s^{s+1} E\| x(t+\tau)-x(t) \|_0^p dt \bigg) ds\\ \leq & H^1 \varepsilon + \rho^1\sup\limits_{s\in \mathbb{R}}\bigg( \int_s^{s+1} E\| x(t+\tau)-x(t) \|_0^p dt \bigg) \int_{-\infty}^\xi e^{-(\xi-s)\frac{p}{q}\underline{a}^0} ds\\ \leq & H^1 \varepsilon + \rho^1\frac{q}{p\underline{a}^0}\sup\limits_{s\in \mathbb{R}}\bigg( \int_s^{s+1} E\| x(t+\tau)-x(t) \|_0^p dt \bigg), \end{align*} |
where \rho^1 is the same as that in (A3) and H^1 = \bigtriangleup_{H_1} + \bigtriangleup_{H_2} +\bigtriangleup_{H_4} +\bigtriangleup_{H_6} +\bigtriangleup_{H_8} +\bigtriangleup_{H_9} +\bigtriangleup_{H_{10}} +\bigtriangleup_{H_{14}} +\bigtriangleup_{H_{16}} +\bigtriangleup_{H_{11}^1} +\bigtriangleup_{H_{12}^1} +\bigtriangleup_{H_{13}^1}.
By (A4) , we know \rho^1 < \frac{p\underline{a}^0}{q} . Hence, we derive that
\begin{align} \sup\limits_{\xi\in \mathbb{R}}\int_\xi^{\xi+1} E\| x(t+\tau)-x(t) \|_0^p dt \; < \; \frac{p\underline{a}^0 H^1}{p\underline{a}^0 - \rho^1 q} \varepsilon. \end{align} | (3.37) |
Case 2. When p = 2 , we can obtain
\begin{align*} & \int_\xi^{\xi+1} E\| x(t+\tau)-x(t) \|_0^2 dt \\ \leq & H^2 \varepsilon + \rho^2 \int_{-\infty}^\xi e^{-(\xi-s)\frac{p}{q}\underline{a}^0 }\bigg( \int_s^{s+1} E\| x(t+\tau)-x(t) \|_0^2 dt \bigg) ds, \end{align*} |
where \rho^2 is defined in (A4) and
\begin{align*} H^2 = & 32 \max\limits_{ij\in\Lambda} \bigg\{ \bigg(\frac{2\kappa} {\underline{a}_{ij}^0 } \bigg)^{2} \sum\limits_{C_{kl}\in N_{h_1}(i,j)} ({C_{ij}^{kl}}^+)^2 (L_f )^2 \bigg\} {\varepsilon} + 16 \max\limits_{ij\in\Lambda} \bigg\{ \frac{m^2n^2}{(\underline{a}_{ij}^0)^2 } \bigg[ (2\kappa M_f )^2 +\bigg(2\kappa M_g \\ & \times \int_0^{\infty}|K_{ij}(u)|du \bigg)^2 \bigg]+ (\Gamma(2))^2 \bigg(\frac{1}{\underline{a}_{ij}^0}\bigg)^{4} \bigg[ \sum\limits_{C_{kl}\in N_{h_1}(i,j)} ({C_{ij}^{kl}}^+)^2 (2\kappa M_f)^2 + \sum\limits_{C_{kl}\in N_{h_2}(i,j)} ({B_{ij}^{kl}}^+)^2 \\ & \times \bigg(2\kappa M_g \int_0^{\infty} |K_{ij}(u)|du \bigg)^2 +(M_L)^2 + (2\kappa \bar{a}_{ij}^c)^2\bigg] + \frac{1}{(\underline{a}_{ij}^0 )^2} \bigg[ \sum\limits_{C_{kl}\in N_{h_2}(i,j) }\big( {B_{ij}^{kl}}^+ \big)^2 \\ & \times \bigg(2\kappa M_g \int_0^{\infty} |K_{ij}(u)| du \bigg)^2 + (2\kappa)^2+1 \bigg] \bigg\} \varepsilon +\bigtriangleup_{H_{11}^2} +\bigtriangleup_{H_{12}^2} +\bigtriangleup_{H_{13}^2}. \end{align*} |
Similar to the previous case, by (A4) , we know \rho^2 < \underline{a}^0 and hence, we can get that
\begin{align} \int_\xi^{\xi+1} E\| x(t+\tau)-x(t) \|_0^2 dt \; < \; \frac{\underline{a}^0 H^2}{\underline{a}^0 - \rho^2} \varepsilon. \end{align} | (3.38) |
Noting that
\begin{align*} &d_{BL}(P\circ[x(t+\tau)]^{-1},P\circ[x(t)]^{-1})\bigg)\\ \leq &\sup\limits_{\|f\|_{BL}\leq 1}\bigg|\int_{\mathscr{A}^{m\times n}}fd(P\circ[x(t+\tau)]^{-1}-P\circ[x(t)]^{-1})\bigg|\\ \leq&\sup\limits_{\|f\|_{BL}\leq 1}\bigg|\int_{\Omega}f(x(t+\tau))-f(x(t))\bigg|dP\\ \leq&\int_{\Omega}\|x(t+\tau)-x(t)\|_0dP\\ \leq&(E\|x(t+\tau)-x(t)\|_0^p)^{\frac{1}{p}}. \end{align*} |
Hence, we have
\begin{align} &\sup\limits_{\xi\in\mathbb{R}}\bigg(\int_\xi^{\xi+1}d_{BL}^p(P\circ[x(t+\tau)]^{-1},P\circ[x(t)]^{-1})dt\bigg)^{\frac{1}{p}}\\ \leq &\bigg(\sup\limits_{\xi\in\mathbb{R}}\int_\xi^{\xi+1}E\|x(t+\tau)-x(t)\|_0^pd t\bigg)^{\frac{1}{p}}. \end{align} | (3.39) |
Combining (3.37)–(3.39), we can conclude that x(t) is p -th Stepanov almost periodic in the distribution sense. The proof is complete.
Similar to the proof of Theorem 3.7 in [21], one can easily show that.
Theorem 3.3. Suppose that (A1) – (A5) are fulfilled and let x be the Stepanov almost periodic solution in the distribution sense of system (2.1) with initial value \varphi . Then there exist constants \lambda > 0 and M > 0 such that for an arbitrary solution y with initial value \psi satisfies
\begin{align*} E\| y(t)-x(t) \|_0^p \leq M \| \varphi -\psi \|_1 e^{-\frac{p}{q}\lambda t}, \; \; t > 0, \end{align*} |
where \| \varphi -\psi \|_1 = \sup_{a\in[-\theta, 0]} E\| \varphi(s) -\psi(s) \|_0^p , i.e., the solution x is globally exponentially stable.
The purpose of this section is to demonstrate the effectiveness of the results obtained in this paper through a numerical example.
In neural network (2.1), choose f(x) = \frac{1}{25} \sin x^0 e_0+\frac{1}{30} \sin (x^2+x^0) e_1+\frac{1}{35}\sin^2x^{12} e_2+\frac{1}{40}\tanh^2x^2 e_{12}, g(x) = \frac{1}{40} \sin x^0 e_0+\frac{1}{35} \sin (x^2+x^1) e_1+\frac{1}{30}\sin^2x^{12} e_2+\frac{1}{25}\tanh^2x^1 e_{12} , K_{ij}(t) = \frac{1}{e^{t}} and
\begin{align*} \begin{bmatrix} a_{11}(t) \\ a_{12}(t) \\ a_{21}(t) \\ a_{22}(t) \\ \end{bmatrix} = \begin{bmatrix} (7+2\text{sin}t) \; e_0 +\text{cos}t \; e_1 +\text{cos}\sqrt5t \; e_2+\text{sin}\sqrt3t \; e_{12} \\ (6-\text{cos}t) e_0+\text{sin}\sqrt3t e_2+\text{cos}\sqrt3t e_{12} \\ (8+\text{cos}\sqrt3t) \; e_0+\text{sin}\sqrt5t \; e_1+\text{sin}t \; e_{12} \\ (10-2\text{sin}\sqrt5t) \; e_0+\text{cos}\sqrt3t \; e_1+\text{cos}t \; e_2+\text{tanh}t \; e_{12} \\ \end{bmatrix}, \end{align*} |
\begin{align*} \begin{bmatrix} C_{11}(t) & C_{12}(t) \\ C_{21}(t) & C_{22}(t) \\ \end{bmatrix} = \begin{bmatrix} B_{11}(t) & B_{12}(t) \\ B_{21}(t) & B_{22}(t) \\ \end{bmatrix} = \begin{bmatrix} E_{11}(t) & E_{12}(t) \\ E_{21}(t) & E_{22}(t) \\ \end{bmatrix} \\ = \begin{bmatrix} 0.02+0.01\text{cost} & 0.03+0.2\sin \sqrt3 \text{t} \\ 0.05+0.07\sin \sqrt5 \text{t} & 0.02+0.05\cos \sqrt3 \text{t}\\ \end{bmatrix}e_0, \end{align*} |
\begin{align*} \begin{bmatrix} L_{11}(t) \\ L_{12}(t) \\ L_{21}(t) \\ L_{22}(t) \\ \end{bmatrix} = \begin{bmatrix} (0.2|\cos|t) \; e_0+(0.2\text{cos}\sqrt3t) \; e_1 + 0.3\text{sin}(\sqrt{3} t) \; e_2 + (0.08\sin t+0.04 e^{-t}) \; e_{12}\\ (0.3\sin(\sqrt{2}t)+e^{-t}) \; e_0+(0.1\cos \sqrt{5} \text{t}+0.04e^{-t}) \; e_1 +0.2e^{-t} \; e_2 + 0.2\text{sin}t \; e_{12} \\ 0.02\sin t\; e_0 + 0.05\text{sin}\sqrt5t \; e_1 + (0.03\cos t+0.01e^{-t}) \; e_2 + 0.02\text{cos}\sqrt{3}t \; e_{12} \\ 0.08\text{sin}t \; e_0+ (0.04\text{cos}\sqrt5t +0.04e^{-t} ) \; e_1 +0.03\text{sin}\sqrt5t \; e_{12} \\ \end{bmatrix}, \end{align*} |
\begin{align*} \begin{bmatrix} \tau_{11}(t) & \tau_{12}(t) \\ \tau_{21}(t) & \tau_{22}(t) \\ \end{bmatrix} = \begin{bmatrix} \sigma_{11}(t) & \sigma_{12}(t) \\ \sigma_{21}(t) & \sigma_{22}(t) \\ \end{bmatrix} = \begin{bmatrix} 0.03+0.009\text{sin0.6t} & 0.05+0.05\text{cos1.2t}\\ 0.02-0.008\text{sin1.1t} & 0.09+0.04\text{sin1.7t} \\ \end{bmatrix}, \end{align*} |
\begin{align*} \begin{bmatrix} \delta_{11}(x) \\ \delta_{12}(x) \\ \delta_{21}(x) \\ \delta_{22}(x) \\ \end{bmatrix} = \begin{bmatrix} \frac{1}{15} \sin \sqrt{3}x^0 e_0+\frac{1}{20} \sin x^2 e_1 +\frac{1}{30}\tanh^2x^1 e_{12} \\ 0.04 \sin x^0 e_1+0.03\sin^2x^{12} e_2+0.05\sin^2x^2 e_{12}\\ 0.02 \tanh x^2 e_0+0.06 \sin x^1 e_1+0.015\sin^2x^{0} e_2 \\ \frac{1}{20} \sin x^1 e_0+\frac{1}{15} \tanh x^2 e_1+\frac{1}{25}\sin x^{12} e_2+\frac{1}{40}\sin x^0 e_{12} \\ \end{bmatrix}, \end{align*} |
and let h_1 = h_2 = 1, h_3 = 0, m = n = 2 . Then we get
\begin{align*} & L_f = L_g = M_g = M_f = 0.04,\; \underline{a}^0 = 5,\; \bar{a}^c = 1,\; M_L = 1, \; M = u = 1,\; L_{11}^{\delta} = \frac{1}{15} ,\; L_{12}^{\delta} = 0.05,\; \\ & L_{21}^{\delta} = 0.06 ,\; L_{22}^{\delta} = \frac{1}{15},\; \tau_{11}^{+} = \sigma_{11}^+ = 0.039 ,\; \tau_{12}^{+} = \sigma_{12}^+ = 0.1,\; \tau_{21}^{+} = \sigma_{21}^+ = 0.028 ,\; \tau_{22}^{+} = \sigma_{22}^+\\ & = 0.13,\; {\dot{\tau}}_{11}^{+} = {\dot{\sigma}}_{11}^+ = 0.0054 ,\; {\dot{\tau}}_{12}^{+} = {\dot{\sigma}}_{12}^+ = 0.006,\; {\dot{\tau}}_{21}^{+} = {\dot{\sigma}}_{21}^+ = 0.0088 ,\; {\dot{\tau}}_{22}^{+} = {\dot{\sigma}}_{22}^+ = 0.068,\\ & \sum\limits_{C_{kl}\in N_1(1,1)}{C_{11}^{kl}}^+ = \sum\limits_{C_{kl}\in N_1(1,1)}{B_{11}^{kl}}^+ = \sum\limits_{C_{kl}\in N_1(1,2)}{C_{12}^{kl}}^+ = \sum\limits_{C_{kl}\in N_1(1,2)}{B_{12}^{kl}}^+ = \sum\limits_{C_{kl}\in N_1(2,1)}{C_{21}^{kl}}^+ \\ & = \sum\limits_{C_{kl}\in N_1(2,1)}{B_{21}^{kl}}^+ = \sum\limits_{C_{kl}\in N_1(2,2)}{C_{22}^{kl}}^+ \; = \; \sum\limits_{C_{kl}\in N_1(2,2)}{B_{22}^{kl}}^+ = 0.45, \; \sum\limits_{C_{kl}\in N_0(1,1)}{E_{11}^{kl}}^+ = 0.03\\ & \sum\limits_{C_{kl}\in N_0(1,2)}{E_{12}^{kl}}^+ = 0.23,\; \sum\limits_{C_{kl}\in N_0(2,1)}{E_{21}^{kl}}^+ = 0.12,\; \sum\limits_{C_{kl}\in N_0(2,2)}{E_{22}^{kl}}^+ = \; 0.07. \end{align*} |
Take \kappa = 1 , p = \frac{21}{10}, q = \frac{21}{11} , then we have
\begin{align*} r^1 < 0.6812 < 1, \; \; \; \frac{q}{p\underline{a}^0}\rho^1 < 0.6259 < 1. \end{align*} |
And when p = 2 , we have
\begin{align*} r^2 < 0.6408 < 1, \; \; \frac{\rho^2}{\underline{a}^0} < 0.6786 < 1. \end{align*} |
Thus, all assumptions in Theorems 3.2 and 3.3 are fulfilled. So we can conclude that the system (2.1) has a unique S^p -almost periodic solution in the distribution sense which is globally exponentially stable.
The results are also verified by the numerical simulations in Figures 1–4.
From these figures, we can observe that when the four primitive components of each solution of this system take different initial values, they eventually tend to stabilize. It can be seen that these solutions that meet the above conditions do exist and are exponentially stable.
In this article, we establish the existence and global exponential stability of Stepanov almost periodic solutions in the distribution sense for a class of stochastic Clifford-valued SICNNs with mixed delays. Even when network (2.1) degenerates into a real-valued NN, the results of this paper are new. In fact, uncertainty, namely fuzziness, is also a problem that needs to be considered in real system modeling. However, we consider only the disturbance of random factors and do not consider the issue of fuzziness. In a NN, considering the effects of both random perturbations and fuzziness is our future direction of effort.
The authors declare that they have not used Artificial Intelligence (AI) tools in the creation of this article.
This work is supported by the National Natural Science Foundation of China under Grant No. 12261098.
The authors declare that they have no conflicts of interest.
[1] | Blockley JG (1969) The stratigraphy of the Mount Tom Price orebody and its implication in the genesis of iron ore. Western Australia Geological Survey Annual Report 1968. Perth, Australia: Western Australia Geological Survey. |
[2] | Ayers DE (1971) The hematite enrichment ores of Mount Tom Price and Mount Whaleback. Aust Inst Min Metall Proc 238: 47–58. |
[3] | Trendall AF, Pepper RS (1977) Chemical composition of the Brockman Iron Formation. Perth, Australia: Western Australia Geological Survey. |
[4] |
Morris RC (1980) A textural and mineralogical study of the relationship of iron ore to banded iron-formation in the Hamersley iron province of Western Australia. Econ Geol 75: 184–209. https://doi.org/10.2113/gsecongeo.75.2.184 doi: 10.2113/gsecongeo.75.2.184
![]() |
[5] | Kneeshaw M (1984) Pilbara iron ore classification—a proposal for a common classification for BIF-derived supergene iron ore. Australas Inst Min Metall 289: 157–162. |
[6] | Guo WW (1999) Magnetic petrophysics and density investigations of the Hamersley Province, Western Australia: implications for magnetic and gravity interpretation, Australia: The University of Western Australia. |
[7] | Li ZX, Guo W, Powell CMA (2000) Timing and genesis of Hamersley BIF-hosted iron deposits: a new palaeomagnetic interpretation, Perth, Australia: Minerals & Energy Research Institute of Western Australia. |
[8] |
Thorne WS, Hagemann SG, Barley M (2004). Petrographic and geochemical evidence for hydrothermal evolution of the North Deposit, Mt Tom Price, Western Australia. Miner Deposita 39: 766–783. https://doi.org/10.1007/s00126-004-0444-x doi: 10.1007/s00126-004-0444-x
![]() |
[9] |
Bodycoat FM (2010) Stratigraphic and structural setting of iron mineralization at E Deposit (East), Area C, Hamersley Province, Western Australia. Appl Earth Sci 119: 49–55. https://doi.org/10.1179/037174510X12853354810543 doi: 10.1179/037174510X12853354810543
![]() |
[10] | Clark DA, Foss CA, Austin J, et al. (2015) Three-dimensional mapping of magnetite and hematite concentrations from reprocessing of detailed aeromagnetic data. Proceedings of the Iron Ore Conference, Perth, Australia. |
[11] | Morris RC (1985) Genesis of iron ore in banded iron–formation by supergene and supergene–metamorphic processes—a conceptual model, In: Wolf KH, Eds., Handbook of strata–bound and stratiform ore deposits, Elsevier, 73–235. |
[12] | Harmsworth RA, Kneeshaw M, Morris RC, et al. (1990) BIF-derived iron ores of the Hamersley Province. Australas Inst Min Metall Mono 14: 617–642. |
[13] |
Barley M, Pickard AL, Hagemann S, et al. (1999). Hydrothermal origin for the 2 billion year old Mount Tom Price giant iron ore deposit, Hamersley Province, Western Australia. Miner Deposita 34: 784–789. https://doi.org/10.1007/s001260050238 doi: 10.1007/s001260050238
![]() |
[14] |
Powell CMcA, Oliver NHS, Li ZX, et al. (1999) Synorogenic hydrothermal origin for giant Hamersley iron oxide ore bodies. Geology 27: 175–178. https://doi.org/10.1130/0091-7613(1999)027<0175:SHOFGH>2.3.CO;2 doi: 10.1130/0091-7613(1999)027<0175:SHOFGH>2.3.CO;2
![]() |
[15] |
Brocks JJ, Summons RE, Buick R, et al. (2003) Origin and significance of aromatic hydrocarbons in giant iron ore deposits of the late Archean Hamersley Basin, Western Australia. Org Geochem 34: 1161–1175. https://doi.org/10.1016/S0146-6380(03)00065-2 doi: 10.1016/S0146-6380(03)00065-2
![]() |
[16] |
Clout JMF (2006) Iron formation-hosted iron ores in the Hamersley Province of Western Australia. Appl Earth Sci 115: 115–125. https://doi.org/10.1179/174327506X138931 doi: 10.1179/174327506X138931
![]() |
[17] |
Morris RC, Kneeshaw M (2011) Genesis modelling for the Hamersley BIF-hosted iron ores of Western Australia: a critical review. Aust J Earth Sci 58: 417–451. https://doi.org/10.1080/08120099.2011.566937 doi: 10.1080/08120099.2011.566937
![]() |
[18] |
White AJR, Legras M, Smith RE, et al. (2014) Deformation-driven, regional-scale metasomatism in the Hamersley Basin, Western Australia. J Metamorphic Geol 32: 417–433. https://doi.org/10.1111/jmg.12078 doi: 10.1111/jmg.12078
![]() |
[19] |
Perring C, Crowe M, Hronsky J (2020). A new fluid-flow model for the genesis of banded iron formation-hosted martite–goethite mineralization, with special reference to the North and South Flank deposits of the Hamersley Province, Western Australia. Econ Geol 115: 627–659. https://doi.org/10.5382/econgeo.4734 doi: 10.5382/econgeo.4734
![]() |
[20] |
Perring CS (2021) Petrography of martite–goethite ore and implications for ore genesis, South Flank, Hamersley Province, Western Australia. Aust J Earth Sci 68: 782–798. https://doi.org/10.1080/08120099.2021.1863860 doi: 10.1080/08120099.2021.1863860
![]() |
[21] |
Perring CS, Hronsky JMA, Crowe M (2022) Phanerozoic history of the Pilbara region: implications for iron mineralization. Aust J Earth Sci 69: 757–775. https://doi.org/10.1080/08120099.2022.2048888 doi: 10.1080/08120099.2022.2048888
![]() |
[22] |
Tompkins LA, Cowan DR (2001) Opaque mineralogy and magnetic properties of selected banded iron‐formations, Hamersley Basin, Western Australia. Aust J Earth Sci 48: 427–437. https://doi.org/10.1046/j.1440-0952.2001.00869.x doi: 10.1046/j.1440-0952.2001.00869.x
![]() |
[23] |
Rasmussen B, Muhling JR (2018) Making magnetite late again: Evidence for widespread magnetite growth by thermal decomposition of siderite in Hamersley banded iron formations. Precambrian Res 306: 64–93. https://doi.org/10.1016/j.precamres.2017.12.017 doi: 10.1016/j.precamres.2017.12.017
![]() |
[24] |
Warchola T, Lalonde SV, Pecoits E, et al. (2018) Petrology and geochemistry of the Boolgeeda Iron Formation, Hamersley Basin, Western Australia. Precambrian Res 316: 155–173. https://doi.org/10.1016/j.precamres.2018.07.015 doi: 10.1016/j.precamres.2018.07.015
![]() |
[25] | Guo WW (2015) Magnetic mineralogical characteristics of Hamersley iron ores in Western Australia. J App Math Phys 3: 150–155. http://dx.doi.org/10.4236/jamp.2015.32023 |
[26] | Blockley JG, Tehnas IJ, Mandyczewsky A, et al. (1993) Proposed stratigraphic subdivision of the Marra Mamba Iron formation and the lower Wittenoom Dolomite, Geological Survey of Western Australia Report 34. Perth, Australia: Western Australia Geological Survey. |
[27] |
Guo W (2023) Density investigation and implications for exploring iron-ore deposits using gravity method in the Hamersley Province, Western Australia. AIMS Geosci 9: 34–48. https://doi.org/10.3934/geosci.2023003 doi: 10.3934/geosci.2023003
![]() |
[28] | Tarling DH, Hrouda F (1993) The Magnetic Anisotropy of Rocks, London, UK: Chapman & Hall. |
[29] | Dunlop DJ, Ozdemir O (1997) Rock Magnetism, Cambridge University Press, Cambridge, UK: http://dx.doi.org/10.1017/CBO9780511612794 |
[30] | Thompson R, Oldfield E (1986) Environmental Magnetism, London, UK: Allen and Unwin. http://dx.doi.org/10.1007/978-94-011-8036-8 |
[31] | Hunt, CP, Moskowitz BM, Banerjee SK (1995) Magnetic properties of rocks and minerals, In Ahrens TJ, Eds., Rock Physics and Phase Relations: A Handbook of Physical Constants, Vol. 3. Washington, DC: American Geophysical Union, 189–204. |
[32] |
Wang L, Pan Y, Li J, et al. (2008) Magnetic properties related to thermal treatment of pyrite. Sci China Ser D-Earth Sci 51: 1144–1153. https://doi.org/10.1007/s11430-008-0083-7 doi: 10.1007/s11430-008-0083-7
![]() |
[33] |
Pan Y, Zhu R, Liu Q, et al. (1999) Magnetic susceptibility variation and AMS exchange related to thermal treatment of siderite. Chin Sci Bull 44: 1135–1139. https://doi.org/10.1007/BF02886143 doi: 10.1007/BF02886143
![]() |
[34] |
Till JL, Nowaczyk N (2018) Authigenic magnetite formation from goethite and hematite and chemical remanent magnetization acquisition. Geophys J Int 213: 1818–1831. https://doi.org/10.1093/gji/ggy083 doi: 10.1093/gji/ggy083
![]() |
[35] |
Ouyang T, Appel E, Jia G et al. (2013) Magnetic mineralogy and its implication of contemporary coastal sediments from South China. Environ Earth Sci 68: 1609–1617. https://doi.org/10.1007/s12665-012-1854-1 doi: 10.1007/s12665-012-1854-1
![]() |
[36] |
Ter Maat GW, McEnroe SA, Church NS, et al. (2019). Magnetic mineralogy and petrophysical properties of ultramafic rocks: Consequences for crustal magnetism. Geochem Geophys Geosyst 20: 1794–1817. https://doi.org/10.1029/2018GC008132 doi: 10.1029/2018GC008132
![]() |
[37] |
Zhao XY, Liu QS (2010) Effects of the grain size distribution on the temperature-dependent magnetic susceptibility of magnetite nanoparticles. Sci China Earth Sci 53: 1071–1078. https://doi.org/10.1007/s11430-010-4015-y doi: 10.1007/s11430-010-4015-y
![]() |
[38] |
Carlut J, Isambert A, Bouquerel H, et al. (2015) Low temperature magnetic properties of the Late Archean Boolgeeda iron formation (Hamersley Group, Western Australia): environmental implications. Front Earth Sci 3. https://doi.org/10.3389/feart.2015.00018 doi: 10.3389/feart.2015.00018
![]() |
[39] |
Henry B, Jordanova D, Jordanova N, et al. (2005) Transformations of magnetic mineralogy in rocks revealed by difference of hysteresis loops measured after stepwise heating: theory and case studies, Geophys J Int 162: 64–78. https://doi.org/10.1111/j.1365-246X.2005.02644.x doi: 10.1111/j.1365-246X.2005.02644.x
![]() |
[40] |
Li Z, Chanéac C, Berger G, et al. (2019) Mechanism and kinetics of magnetite oxidation under hydrothermal conditions. RSC Adv 58: 33633–33642. https://doi.org/10.1039/c9ra03234g doi: 10.1039/c9ra03234g
![]() |
[41] |
Zhao J, Brugger J, Pring A (2019) Mechanism and kinetics of hydrothermal replacement of magnetite by hematite. Geosci Front 10: 29–41. https://doi.org/10.1016/j.gsf.2018.05.015 doi: 10.1016/j.gsf.2018.05.015
![]() |
[42] |
Yu J, Han Y, Li Y, et al. (2017) Mechanism and kinetics of the reduction of hematite to magnetite with CO–CO2 in a micro-fluidized bed. Minerals 7: 209. https://doi.org/10.3390/min7110209 doi: 10.3390/min7110209
![]() |
[43] |
Du W, Yang S, Pan F, et al. (2017) Hydrogen reduction of hematite ore fines to magnetite ore fines at low temperatures. J Chem. https://doi.org/10.1155/2017/1919720 doi: 10.1155/2017/1919720
![]() |