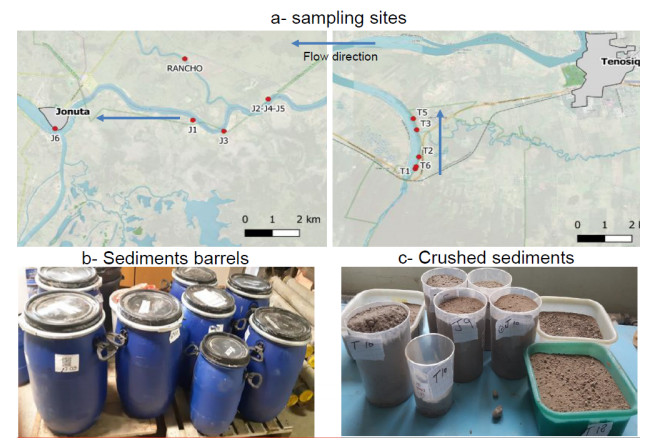
In the present paper, we establish the incomplete exponential type (IEF) of R-matrix functions and identify some properties of the incomplete exponential matrix functions including integral representation, some derivative formula and generating functions of the incomplete exponential of R-matrix functions. Finally, special cases of the presented results are pointed out.
Citation: Ahmed Bakhet, Mohra Zayed. Incomplete exponential type of R-matrix functions and their properties[J]. AIMS Mathematics, 2023, 8(11): 26081-26095. doi: 10.3934/math.20231329
[1] | Congying Wang, Fu Zhao, Carol Handwerker . Transforming and integrating informal sectors into formal e-waste management system: A case study in Guiyu, China. Clean Technologies and Recycling, 2022, 2(4): 225-246. doi: 10.3934/ctr.2022012 |
[2] | Chukwuebuka C. Okafor, Chinelo A. Nzekwe, Nixon N. Nduji, Charles C. Ajaero, Juliet C. Ibekwe . Energy and material recovery potential from municipal solid wastes (MSW) in Nigeria: Challenges and opportunities. Clean Technologies and Recycling, 2022, 2(4): 282-307. doi: 10.3934/ctr.2022015 |
[3] | Glenn Baxter . Towards sustainable airport waste management through the adoption of a "green" airport strategy: The case of Incheon International Airport. Clean Technologies and Recycling, 2022, 2(4): 247-278. doi: 10.3934/ctr.2022013 |
[4] | Kyle Pender, Liu Yang . Glass fibre composites recycling using the fluidised bed: A study into the economic viability in the UK. Clean Technologies and Recycling, 2023, 3(3): 221-240. doi: 10.3934/ctr.2023014 |
[5] | Wilson Uzochukwu Eze, Reginald Umunakwe, Michael Ifeanyichukwu Ugbaja, Mohammed Kabiru Yakubu, Narcillina Nkechi Adegboro, Amina Hassan Bayero, Maryann Ifeoma Uzochukwu . Utilization of commodity plastic wastes in flexible pavement: A review. Clean Technologies and Recycling, 2023, 3(1): 71-91. doi: 10.3934/ctr.2023005 |
[6] | Dmitry Baturkin, Ousmane A. Hisseine, Radhouane Masmoudi, Arezki Tagnit-Hamou, Slimane Metiche, Luc Massicotte . Compressive behavior of FRP-tube-confined concrete short columns using recycled FRP materials from wind turbine blades: Experimental investigation and analytical modelling. Clean Technologies and Recycling, 2022, 2(3): 136-164. doi: 10.3934/ctr.2022008 |
[7] | Ana Cram, Jose Espiritu, Heidi Taboada, Delia J. Valles-Rosales, Young Ho Park, Efren Delgado, Jianzhong Su . Multi-objective biofuel feedstock optimization considering different land-cover scenarios and watershed impacts. Clean Technologies and Recycling, 2022, 2(2): 103-118. doi: 10.3934/ctr.2022006 |
[8] | Yi Ji, Edwin E. Kpodzro, Chad T. Jafvert, Fu Zhao . Direct recycling technologies of cathode in spent lithium-ion batteries. Clean Technologies and Recycling, 2021, 1(2): 124-151. doi: 10.3934/ctr.2021007 |
[9] | Charles C. Ajaero, Chukwuebuka C. Okafor, Festus A. Otunomo, Nixon N. Nduji, John A. Adedapo . Energy production potential of organic fraction of municipal solid waste (OFMSW) and its implications for Nigeria. Clean Technologies and Recycling, 2023, 3(1): 44-65. doi: 10.3934/ctr.2023003 |
[10] | Wilson Uzochukwu Eze, Reginald Umunakwe, Henry Chinedu Obasi, Michael Ifeanyichukwu Ugbaja, Cosmas Chinedu Uche, Innocent Chimezie Madufor . Plastics waste management: A review of pyrolysis technology. Clean Technologies and Recycling, 2021, 1(1): 50-69. doi: 10.3934/ctr.2021003 |
In the present paper, we establish the incomplete exponential type (IEF) of R-matrix functions and identify some properties of the incomplete exponential matrix functions including integral representation, some derivative formula and generating functions of the incomplete exponential of R-matrix functions. Finally, special cases of the presented results are pointed out.
Usumacinta River basin (Tabasco, Mexico) is located in a tropical zone and it is vulnerable to climate changes and human activities. Concrete blocks and fired bricks are commonly used construction materials in this region and soil used in these comes from quarries. Higher energy consumption and use of nonrenewable soil resources adversely affect the socio-ecosystem of the region. The use of dredged sediments by partially or fully replacing soil can reduce the burden on nonrenewable clay resources [1]. Sediments are naturally accumulated in the ports, navigation channels, dams and lakes due to their transportation by water channels and erosion of coastal areas. These sediments are dredged to maintain the navigation, water flow and storage operations. A large quantity of dredged sediments is released into the sea. In the case of fluvial sediments, it is difficult to dump sediments in the sea due to remoteness. The strict environmental regulations have encouraged the reuse of sediments in different applications. The higher initial water content of dredged sediments is a problem for their reuse. The initial water content of dredged sediments sometimes ranges up to 300% [2]. Sediments consist of water, organic and inorganic materials and sometimes pollutants. Inorganic compounds include silicates (quartz, mica, feldspar, etc.) clay minerals (kaolinite, illite and montmorillonite), carbonates (calcium carbonate, dolomite), iron oxides, phosphates and sulfides [3]. Polluted sediments contain heavy metals such as Hg, Ni, Cr, Pb, Cu, etc. and organic compounds such as polycyclic aromatic hydrocarbons (PAH) and polychlorinated biphenyls (PCB) due to the leaching of heavy metals and chemicals from industrial and agricultural waste. Polluted sediments are stored at land sites and treated to remove contaminants. Magnetic separators are used to remove metals in sediments. Organic impurities can be decreased by burning the sediments in applications such as clinker and fired bricks as different experimental studies have demonstrated that organic impurities were volatilized during the firing operation of bricks. Furthermore, the leaching of sediments-based bricks shows a decrease in impurities as they are immobilized with the formation of new minerals by firing the bricks at high temperatures sulfides [4,5].
The limits of concentration of heavy metals in dredged marine and estuarine sediments are defined by the French Ministry of Ecology and sustainable development which classifies the sediments based on the quantity of heavy metals in sediments. The metals include the elements such as (Cd, Cr, Cu, Pb, Zn, As, Ni and Hg). Sediments with contaminants above the defined threshold need prior treatment before their reuse [6].
Sediment valorization in different sectors can be achieved by the sediment's nature and the demand of the local industry. As dredged sediments are heterogeneous, research on these sediments is concerned only with their specific use. Common sectors for sediments recycling are roads, cement, concrete, backfill operations, beach nourishments and ceramics etc. [7,8,9]. In the ceramic industry, sediments can be used to make bricks and tiles. Understanding the physical, chemical and mineralogical characteristics of dredged sediments is essential for their use in bricks as dehydration of sediments, deposit selection, presence of salts and organic substance, firing and mechanical behavior of sediments are deciding factors for sediments suitability for bricks [10]. Physical properties of sediments include density, Atterberg limits, grain size analysis, etc. The chemical analysis provides information about different oxides in the soil mass like MgO, SiO2, Cao, alkalis and alumina. Mineralogical analysis of sediments helps to see the percentage of minerals like quartz, kaolinite, muscovite, illite, montmorillonite etc. [11].
Physical and mechanical characteristics of fired bricks are influenced by the nature of the sediments, percentage of clay, silt, sand, organic matter and different minerals. Clay, silt and sand are the main constituents of sediments. The soil used for manufacturing fired bricks are usually composed of oxides such as silica, alumina, calcite and oxides of iron, magnesium, sodium etc. [12,13,14].
Along with raw material, manufacturing methods and firing temperature are also important for fired bricks [15]. Dredged sediments have higher natural moisture content which can be controlled with the addition of additives, such as quick lime [16] or drying the sediments before their reuse in bricks as compaction of bricks which removes the voids and increases the brick's strength is heavily influenced by molding moisture content [17]. The firing of bricks consumes a higher amount of energy and during the firing of bricks, different reactions such as evaporation of moisture, combustion of organic matter, decomposition of carbonates and vitrification take place [18]. The firing temperature of bricks ranges from 600 to 1100 ℃ and depends on the type of soil and the strength of bricks needed [19].
For sediment reuse in fired bricks, their characteristics play an important role. Studies on the characterization and valorization of Usumacinta River sediments are limited. Therefore, this research is primarily focused on the characterization of sediments from the Usumacinta River and their reuse in manufacturing fired bricks at moderate temperatures to preserve non-renewable soil resources. Additionally, brick manufacturing at low temperature allows the reuse of local agro-industry waste (wooden and plant waste) to burn bricks which decrease cost and eliminate waste. As river sediments are heterogenous, it is difficult to develop a universal approach. However, the approach adopted for Usumacinta River sediments can be helpful for other cases as the use of renewable river dredged sediments and local agro-waste for firing bricks can be replicated to preserve the resources and produce low-cost bricks by optimizing the use of energy at a local scale [20].
Usumacinta River sediments are dredged from Tenosique (T) and Jonuta (J) towns in the Tabasco state of Mexico and are named T1, T2, T5, T6, J3 and J4. Sediment sampling locations near Tenosique and Jonuta are shown in Figure 1a. Figure 1b shows hermetic barrels filled with dredged sediments while Figure 1c shows the dried sediments.
The coordinates of dredging sites are shown in Table 1.
Sediments | Latitude | Longitude |
T1 | 17.43126 | −91.4875 |
T2 | 17.44998 | −91.4882 |
T5 | 17.44998 | −91.4882 |
T6 | 17.43205 | −91.4872 |
J3 | 18.08497 | −92.0798 |
J4 | 18.09736 | −92.0642 |
Sediment's physical, chemical, and geotechnical characteristics were investigated to observe their suitability for fired bricks. Sediment characteristics include mineralogy, chemical composition, Atterberg limits, thermogravimetry, shear strength, carbonate content, organic matter, Methylene blue value etc. [21]. These characteristics have a significant influence on the mechanical characteristics of fired bricks. Granulometry of sediments helps to observe sediment's suitability for ceramic applications with the Winkler diagram. Chemical composition is useful to see the percentage of different oxides. The presence of pollutants is also detected with chemical analysis. The oxide content of sediments is used to observe sediment's suitability for ceramic application in the Augustinik diagram. Atterberg limits of sediments help to find molding moisture content through Sembenelli interpretation and the sediment's suitability for molding is observed with a clay workability chart.
Grain size analysis of sediments was performed with laser granulometry. Sediments were classified according to French standards [22]. Carbonate content (CaCO3) of sediments was found with the Bernard calcimeter method by sediments reaction with acid [23]. Organic matter (OM) of sediments was found by burning the sediments at 550 ℃ [24]. Atterberg limits of sediments were determined by French standards [25]. Methylene blue value (MBV) was found with the French standard [26]. The pH of sediments was measured with a pH meter according to French standards [27]. The optimum water content (Wopt) of sediments was determined with the Proctor test [28].
The chemical composition of sediments is important for the strength and quality of bricks. The elemental composition of Usumacinta sediments was determined with SEM analysis while oxide and mineralogical composition were found with XRD by Yamaguchi [29]. Thermogravimetric analysis (TGA) of Usumacinta sediments was done with TGA 295 F1 Libra thermogravimetric analyzer (Netzsch) to examine the sediment's thermal stability at a temperature range of 25 to 850 ℃.
The manufacturing process of fired bricks consists of material preparation, molding, drying and firing. Usumacinta River sediments were dried in an oven at 40 ℃ which is similar to the summer temperatures in the zones where sediments have been dredged, as the envisaged approach is air drying of sediments. Dried sediments were crushed and passed through a 2 mm sieve and mixed with molding moisture content from tap water to make sediment paste. The quantity of molding moisture varies with soil type and manufacturing technique. Sembenelli interpretation is widely used in industry to find the molding moisture content of sediments. Sembenelli's interpretation describes the water content necessary to make fired bricks. In Figure 2, the water content suitable for fired bricks ranges between points C and D which are the plasticity and liquidity limits of soil [19].
The higher molding moisture content leads to shrinkage and crack growth. Low water content makes compaction difficult and increases the pores in the sediment mixture. Therefore, the midpoint between the plasticity limit (point C) and liquidity limit (point D) was considered as molding moisture content for Usumacinta sediments. Table 2 shows the water content used to mix sediments derived from the Sembenelli diagram.
Sediments | T1 | T2 | T5 | T6 | J3 | J4 |
W (%) | 29.8 | 23.4 | 26.4 | 25.1 | 29.6 | 42.9 |
The sediment mixture was molded into laboratory-scale cubic and prismatic specimens of size 20 × 20 × 20 mm3 and 15 × 15 × 60 mm3 for compressive and tensile strength testing of bricks. Manual molding was done with bottomless wooden molds. Molds were filled with sediments, leveled, and manually compacted, and excessive clay on the top of the molds was removed. After molding, bricks were oven-dried at 60 ℃ for 4–12 hours. Drying of bricks prevents the bricks from swelling at a high temperature which occurs due to moisture entrapped in the bricks. Moreover, it preserves the shape of bricks and facilitates their transportation. Usumacinta sediments bricks were fired at a temperature range of 700 to 1100 ℃. This is a common temperature range in brick kilns and research studies [18,30,31] and is achievable by burning agro-waste. The manufacturing steps of fired bricks are shown in Figure 3.
ATG analysis of Usumacinta River sediments shows that during the firing of bricks, breakdown of carbonates occurs around 700 ℃ which releases CaO that reacts with free silica and alumina. Decomposition and disappearing phases in fired bricks contribute to the formation of the vitreous phase [32] which is important for the strength of bricks. Therefore, the firing process was kept simplified and bricks were burnt at a temperature range of 700 to 1100 ℃. The firing duration of bricks ranges from 4–12 hours in different industrial and research works [13]. Usumacinta sediments bricks were fired for 6 hours and left to cool in the oven.
The compressive and tensile strength of Usumacinta bricks was found with the Shimadzu AGS-X model machine by using 200 N and 50 KN sensors at a displacement rate of 0.5 mm/min. Compressive strength testing of Usumacinta bricks is shown in Figure 4a and the typical compressive load-deflection curve for Usumacinta River sediments bricks is shown in Figure 4b. Indirect tensile strength of fired bricks was found with a three-point bending test according to ASTM standard [33] as shown in Figure 4c. Typical flexural load-deflection curve for fired bricks is presented in Figure 4d. Flexural load-deflection curve shows that fired bricks have brittle behavior as the post-peak load-bearing capacity of bricks is zero.
The physical and chemical characteristics of Usumacinta sediments are summarized in Table 3.
Sediments | OM (%) | MBV (g/100g) | CaCO3 (%) | Wopt (%) | ρsed (g/cm3) | pH (-) | LL (%) | Clay (%) | Silt (%) | Sand (%) |
T1 | 3.77 | 2.53 | 8.21 | 17.30 | 2.70 | 8.04 | 39.01 | 6.90 | 40.20 | 52.90 |
T2 | 3.50 | 2.00 | 7.19 | 15.50 | 2.71 | 8.21 | 28.75 | 3.57 | 31.08 | 65.35 |
T5 | 3.46 | 5.90 | 1.90 | 20.80 | 2.61 | 8.17 | 36.75 | 8.75 | 45.90 | 45.00 |
T6 | 4.90 | 1.93 | 8.73 | 17.90 | 2.68 | 8.61 | 30.71 | 6.09 | 40.70 | 53.20 |
J3 | 4.73 | 3.61 | 8.19 | 18.80 | 2.70 | 8.01 | 38.91 | 4.66 | 36.30 | 59.00 |
J4 | 5.72 | > 8.00 | 8.49 | 21.00 | 2.53 | 8.51 | 61.98 | 13.40 | 62.50 | 24.10 |
Table 3 shows that the calcite content of T6 sediments is higher and for T5 sediments it is lowest. The calcite content of sediments is the cause of efflorescence in fired bricks. Calcareous sediments exhibit good compressive strength at low temperatures, but their resistance against salt crystallization is poor due to bad pore size distribution. The organic matter of most of the sediments is below 5% which is low and good for bricks as higher organic matter increases the pores and water absorption of bricks and decreases the strength of bricks [18,34]. On the other hand, the creation of pores decreases by combustion of organic matter and decreases the density of bricks and improves their thermal characteristics by controlling the temperature and humidity by absorbing the moisture. pH value of sediments shows that most of the sediments are slightly alkaline. The liquidity value of J4 sediments is very high as these sediments have high clay content. The clay content of most of the sediments is low, except J4 and T5. An excessive amount of clay results in the shrinkage of bricks and crack development.
The elemental composition of Usumacinta sediments was found with SEM analysis and the results are shown in Table 4. The oxide composition of sediments is important and helps to determine the sediment's suitability for fired bricks. The oxide composition of Usumacinta sediments is shown in Table 4.
Sediments | Elemental composition | Oxide composition | ||||||||||||||
O (%) | Al (%) | Si (%) | Ca (%) | Mg (%) | Fe (%) | K (%) | Na (%) | P (%) | SiO2 (%) | Al2O3 (%) | TiO2 (%) | Fe2O3 (%) | CaO (%) | K2O (%) | ||
T1 | 51.98 | 7.51 | 38.47 | 1.43 | 0.61 | - | - | - | - | 55.4 | 12.2 | 1.2 | 10.9 | 16.0 | 3.6 | |
T2 | 57.77 | 9.04 | 22.28 | 2.01 | 2.57 | 3.89 | 1.31 | 1.12 | 0.00 | 55.4 | 11.7 | 1.2 | 8.5 | 11.2 | 3.7 | |
T5 | 59.74 | 9.60 | 30.36 | 0.00 | 0.29 | - | - | - | - | 62.8 | 14.7 | 1.0 | 9.1 | 8.3 | 3.2 | |
T6 | 50.69 | 8.54 | 36.99 | 3.25 | 0.52 | - | - | - | - | 59.6 | 12.2 | 1.1 | 9.8 | 12.1 | 4.2 | |
J3 | 54.88 | 8.66 | 22.42 | 3.75 | 1.52 | 4.66 | 2.88 | 0.34 | 0.90 | 59.3 | 14.6 | 1.0 | 9.7 | 11.2 | 3.5 | |
J4 | 57.06 | 12.11 | 26.18 | 5.27 | 0.30 | - | - | 0.18 | - | 56.3 | 16.1 | 1.9 | 16.1 | 6.4 | 2.6 |
O, Si, and Al are major elements in the Usumacinta River sediments. Similarly, oxides of Al, Si and Ca are the main oxides in Usumacinta sediments. Most of the oxide values in Usumacinta River sediments are within the range of traditional oxide range of soils used for ceramics in France as described in Table 5.
Range | LOI | SiO2 | Al2O3 | TiO2 | Fe2O3 | CaO | MgO | Na2O | K2O | S | F |
Minimum % | 3 | 35 | 8 | 0.3 | 2 | 0.5 | 0 | 0.1 | 0.1 | 0 | 0 |
Maximum % | 18 | 80 | 30 | 2 | 10 | 18 | 5 | 1.5 | 4.5 | 0.5 | 0.15 |
The mineralogical composition of sediments was determined with XRD and the results are shown in Table 6.
Mnt (%) | Ilt (%) | Vrm (%) | Bt (%) | Qz (%) | Cal (%) | Dol (%) | Crs (%) | Or (%) | Ano (%) | Ab (%) | NIM (%) | |
T1 | 2.6 | 5.1 | 3.1 | 3.1 | 43.7 | 7.1 | 18.4 | 2.5 | 4.0 | 5.3 | 3.2 | 1.9 |
T2 | 4.0 | 2.2 | 2.2 | 2.7 | 52.0 | 4.8 | 14.1 | 3.2 | 3.8 | 5.9 | 3.9 | 1.2 |
T5 | 5.1 | 5.4 | 3.0 | 2.6 | 50.5 | 3.0 | 9.7 | 1.7 | 3.1 | 5.4 | 3.2 | 7.3 |
T6 | 3.4 | 2.3 | 3.1 | 2.8 | 48.7 | 3.9 | 14.7 | 2.6 | 4.0 | 9.2 | 3.8 | 1.5 |
J3 | 3.6 | 2.6 | 2.9 | 2.9 | 48.4 | 5.6 | 16.5 | 2.9 | 4.2 | 5.9 | 3.0 | 1.5 |
J4 | 10.0 | 6.4 | 17.1 | 7.0 | 21.4 | 2.2 | 10.1 | 1.6 | 5.3 | 9.6 | 4.3 | 5 |
Mnt = montmorillonite, Ilt = illite, Vrm = vermiculite, Bt = biotite, Qz = quartz, Cal = calcite, Dol = dolomite, Crs = cristobalite, Or = orthoclase, Ano = anorthoclase, Ab =albite, NIM = non-identified minerals. |
XRD analysis of sediments shows that quartz is an important mineral in Usumacinta sediments. J4 sediments have higher clay minerals such as montmorillonite, illite, and vermiculite minerals contrary to kaolinite which absorbs a higher amount of water and is susceptible to significant volume variations [35]. TGA analysis of Usumacinta sediments was performed at a temperature range of 25 to 850 ℃. The degradation of Usumacinta sediments with temperature is illustrated in Table 7.
Sediments | 200 (%) | 300–550 (%) | 550–800 (%) | Total loss (%) |
T1 | 1.65 | 2.14 | 7.08 | 10.86 |
T2 | 1.21 | 1.82 | 6.95 | 10.60 |
T5 | 3.65 | 2.53 | 1.87 | 8.04 |
T6 | 1.93 | 2.25 | 7.68 | 11.86 |
J3 | 2.38 | 2.54 | 7.78 | 12.69 |
J4 | 4.18 | 2.15 | 9.28 | 15.60 |
TGA analysis shows the initial drying of sediments by removal of pore water at a temperature of 200 ℃ takes place with a mass reduction of 1.21% to 4.18%. This is followed by the removal of water in the molecular structure of clay at 200 to 500 ℃ with mass loss of 1.82% to 2.5%. Around 500 ℃, mass loss takes place as kaolinite is transformed into metakaolin after decomposition and inherent water is evaporated [13]. Dehydroxylation of clay happens between 500 to 800 ℃ and mass loss is highest in this zone [36]. Overall mass loss is highest in J4 sediments and minimum in T5 sediments. This is because organic matter is highest in J4 sediments and lowest in T5 sediments. Calcium carbonate is another factor as it transforms into CaO and releases CO2. CaCO3 is very low in T5 sediments.
To manufacture fired bricks from Usumacinta sediments, their suitability for bricks was observed with industrial approaches such as the Winkler diagram [37], Augustinik [18], clay workability chart [38] and Sembenelli graph.
Winkler diagram [36] describes the suitability of sediments for bricks and tiles on the base of their clay, silt and sand content. Different zones suitable for ceramics are highlighted in the soil texture ternary diagram in Figure 5a. Usumacinta River sediment's suitability for fired bricks is also described in the Winkler diagram in Figure 5a. Usumacinta sediments lie outside the recommended zones of bricks. However, this diagram mainly deals with soil mined from quarries and it is possible to shift the sediments inside the recommended zones by mixing sediments with higher clay content.
Clay workability chart helps to observe the sediment's suitability for molding on the base of Atterberg limits of sediments. Clay workability chart is shown in Figure 5b. Sediments that lie inside zone C have optimum molding characteristics. Sediments in zone D have good molding characteristics. Sediments outside zone C and D do not possess good molding properties. It can be observed in Figure 5b that T5, J4 and T6 exhibit ideal molding qualities while T1 and T2 sediments have good molding characteristics. J3 sediments are outside the zone suitable for molding. Molding characteristics of sediments vary with the consistency limits of sediments. The plasticity limit of T2 and J3 sediments is low due to high sand and coarse silt content which affects their position in the clay workability chart.
The Augustinik diagram describes the sediment's suitability for fired bricks on the base of oxide content. Sediment suitability for bricks and tiles is defined with oxide percentages as shown by separate zones in Figure 5c. Al2O3/SiO2 ratio is usually below 0.55 for the fired bricks and below 0.3 for tiles [18]. Figure 5d shows the ternary diagram proposed by Taha [39] in which a circular zone is suitable for fired bricks based on the chemical composition of the soil. Usumacinta sediments were positioned in Figure 5d with their respective oxide content. It can be observed that Usumacinta sediment's chemical composition is appropriate for its use in fired bricks.
Analysis of environmental, physico-chemical and mineralogical shows that sediment characteristics vary with the site, and especially the presence of fine particles and the percentage of clay minerals. However, each sampling location has a significant sediment volume to satisfy the local demands. The presence of pollutants such as heavy metals, PAHs and PCBs is negligible in Usumacinta River sediments. Usumacinta sediments have higher montmorillonite, illite and vermiculite minerals contrary to Kaolinite which absorbs a higher amount of water and is susceptible to significant volume variations [36].
Augustinik diagram shows that the oxide content of sediments is suitable for bricks. Furthermore, the clay workability chart shows that most of the sediments have good molding characteristics. Winkler diagram shows that the clay content of sediments is lower than the recommended percentage of fine particles. However, two sites Jonuta (J4) and Tenosique (T5) have a higher percentage of clay minerals and fine particles with good plasticity. Furthermore, mixing sediments to deal with the missing component can improve their granulometry and suitability for bricks.
The strength optimization of fired bricks with temperature was done by firing bricks at 700 to 1100 ℃. Chemical reactions occur at high temperatures in bricks and sediments are transformed into a liquid state due to which pores are filled and isolated [36]. Calcium carbonate at a temperature close to 800 ℃, changes into CaO. CaO reacts with moisture and transforms into Ca (OH)2. The transition of Cao into Ca (OH)2 during firing is followed by an increase in volume, and if Ca (OH)2 catches CO2 from the air, CaCO3 is formed. By formation of CaCO3, volume is increased three-times and causes the popping and damage of products [40,41]. Damaged Usumacinta river sediment bricks during firing are shown in Figure 6a. This phenomenon was observed in J4 sediments bricks. Insufficient molding moisture content, high clay content and lower compaction are some other reasons behind the presence of cracks and pores. The water absorption of bricks increases with increasing pores and cracks. At high firing temperatures, porosity of bricks decreases. The length and thickness of bricks also decrease at high temperatures.
Bricks offer different colors, which depend on different minerals present inside the sediments. The presence of iron oxide, calcium oxide, and magnesium oxide and the occurrence of chemical reactions during firing give bricks a different appearance. At high temperatures, iron minerals transform into hematite which gives bricks a reddish and reddish-brown color. The color of bricks after firing in the case of kaolinite clays depends on Fe2O3 content and impurities. The yellow color of bricks indicates higher quartz and kaolinite and less CaO content. In CaO-rich sediments, pyroxene is formed and a light brown color in bricks appears [41]. Yellow color bricks are dominant due to minerals like quartz, diopside and feldspar while red color bricks are dominant in quartz, hematite and feldspar minerals [42]. The color variation of J3 (J3-9C) sediments bricks is shown in Figure 6b.
Physical characteristics of Usumacinta bricks such as linear shrinkage, absolute density and water absorption were observed through testing and measurement. Linear shrinkage and density of bricks at different firing temperatures are shown in Table 8.
Sediments | Linear shrinkage | Density | |||||||||
LS (%) 700 ℃ | LS (%) 800 ℃ | LS (%) 900 ℃ | LS (%) 1000 ℃ | LS (%) 1100 ℃ | ρ (kg/m3) 700 ℃ | ρ (kg/m3) 800 ℃ | ρ (kg/m3) 900 ℃ | ρ (kg/m3) 1000 ℃ | ρ (kg/m3) 1100 ℃ | ||
T1 | 0.6 | 0.9 | 1.6 | 0.7 | 1.7 | 994 | 1268 | 1303 | 1188 | 1244 | |
T2 | - | - | - | - | - | 1146 | 1155 | 1148 | - | 1320 | |
T5 | 10.7 | 10.2 | 12.8 | 11.1 | 13.2 | 1683 | 1606 | 1724 | 1672 | 1818 | |
T6 | 1.2 | 2.1 | 2.3 | 3.4 | 3.4 | 1321 | 1337 | 1277 | 1358 | 1432 | |
J3 | 4.9 | 4.6 | 4.6 | 5.7 | 6.9 | 1331 | 1281 | 1226 | 1302 | 1401 | |
J4 | 12.7 | 13.9 | 12.9 | 12.1 | 13.5 | 1702 | 1588 | 1554 | 1666 | 1637 |
Linear shrinkage is highest in T5 and J4 sediments. Linear shrinkage varies with sediments properties and in bricks made from dredged sediments, it ranges from 2.5% to 12.53% [10]. Higher linear shrinkage is usually associated with higher molding moisture content, higher organic matter and higher clay content. Similarly, with increasing temperatures, linear shrinkage in bricks increases. At high temperature around 1100 ℃, pores in bricks are filled due to vitrification which decreases the density of bricks and increases the linear shrinkage [43]. Table 8 shows that the density of bricks increases with increasing temperature as pores are reduced with increasing temperature due to the amorphous phase. Furthermore, clay sediments have higher shrinkage on drying which increases their density. The water absorption of bricks was determined by immersing the bricks in water for 24 hours. Table 9 shows the water absorption of bricks at 850 ℃.
Sediments | T1 | T2 | T5 | T6 | J3 | J4 |
WA (%) | 22.4 | 17.6 | 10.7 | 19.3 | 21.5 | 16.2 |
Most of the water absorption in Usumacinta bricks occurs during the first hour of immersion and decreases with increasing temperature. At 850 ℃, T5 and J4 sediments have comparatively lower water absorption associated with a higher percentage of fine particles and clay minerals. Good quality bricks usually absorb 15–22% water [44]. However, sediments-based bricks have a significant variation in water absorption as shown in Table 11. Mechanical characteristics of fired bricks were determined with compressive and flexural strength tests. The approximate estimation of error is around ±0.2 MPa. Strength results are summarized in Table 10.
Sediments | 700 ℃ | 800 ℃ | 850 ℃ | 900 ℃ | 1000 ℃ | 1100 ℃ | |
Compressive strength (MPa) | T1 | 0.40 | 0.57 | 0.53 | 1.84 | 0.68 | 2.69 |
T2 | 0.10 | 0.12 | 0.94 | 0.83 | 0.67 | 2.00 | |
T5 | 15.51 | 7.59 | 4.92 | 13.15 | 9.04 | 16.67 | |
T6 | 1.33 | 2.18 | 2.04 | 2.93 | 1.93 | 6.75 | |
J3 | 2.46 | 3.50 | 3.00 | 6.25 | 3.76 | 9.05 | |
J4 | 17.04 | 19.00 | 19.15 | 15.57 | 15.65 | 19.38 | |
Flexural strength (MPa) | T1 | 0.17 | 0.28 | 0.45 | 0.43 | 0.64 | 1.23 |
T2 | 0.20 | - | 0.63 | - | 0.31 | 0.90 | |
T5 | 3.55 | 2.31 | 2.23 | 4.57 | 4.17 | 6.60 | |
T6 | 0.35 | 0.99 | 0.96 | 1.36 | 1.78 | 3.00 | |
J3 | 0.92 | 1.37 | 1.92 | 3.84 | 2.15 | 4.86 | |
J4 | 6.35 | 9.01 | 7.66 | 7.30 | 12.82 | 8.68 |
References | Sediment origin | Sediment (%) | Others (%) | Firing temp. (℃) | UCS (MPa) | WSC (%) | Porosity (%) | Density (g/cm3) |
Anger (2014) | Dam sed | 80–100 | Kaolinite | 800–1150 | 7.5–10 | 20–31 | 41–51 | 1.36–1.52 |
Ben Allal et al. (2011) | Port sed | 0–70 | Clay | 920 | 6.8–33 | 10–33 | 18–45 | 1.81–1.37 |
Benkadja et al. (2013) | Dam sed | 0–65 | sand | 800 | 55 | |||
Boulingui et al. (2015) | Mined clay | 900–1150 | ||||||
Chiang et al. (2008) | Dam sed | 80–100 | Clay | 1050–1150 | ||||
Haurine (2015) | Dam sed | 70–100 | sand | 950–1100 | 5–50 | |||
Labiod et al. (2004) | Dam sed | 100 | - | 5–9 | ||||
Liang & Li (2015) | Dam sed | Gypsum | 1100 | |||||
Marouf et al. (2018) | Dam sed | 850–1050 | 21 | 30–40 | ||||
Nedloussi et al. (2019) | Dam sed | 80–100 | Sand | 600–900 | 28–46 | |||
Remini (2006) | Dam sed | 0–100 | clay | 900 | 10–40 | 6–20 | 12–24 | 1.4–1.9 |
Romero et al. (2009) | Harbour sed | 100 | - | 900–1200 | 34 | 4-22 | 12–38 | 1.60–2.45 |
Samara et al. (2009) | River sed | 100 | - | 1000 | 36 | 7.5 | 15.4 | - |
Tangprasert et al. (2015) | River sed | 80–100 | Husks | 700 | 1.9–9 | 17–29 | - | 1.13–1.57 |
Torres et al. (2009) | River sed | 5–10 | Clay | 950–1100 | 7.5–35 | 3.5–15 | - | - |
Wei et al. (2014a) | Harbour sed | Slag | 950–1100 | |||||
Xu et al. (2014) | River sed | 50–80 | 1100 | |||||
Note: sed = sediments; sediment rate: sediment proportion in mixture; others: other components in mixture; UCS: unconfined compressive strength; WSC: water sorption capacity (24h); PI: plasticity index; firing temp.: firing temperature; 880–1100: optimal values of temperature. |
Bricks with sediments T5 and J4 have good compressive strengths which are 16.7 and 19.38 MPa at 1100 ℃. These sediments have good strength even at a moderate temperature of 700 ℃. Furthermore, no additive was used to increase the strength, which is important for the cost and environmental aspects. The compressive strength of sandy sediments such as T1 and T2 is significantly low. T6 and J3 sediments have good strength at 1100 ℃. The compressive strength of bricks increases with increasing temperature. Similar trends have been observed in literature studies [45,46]. However, high firing temperature increases the cost and decreases the eco-friendly nature of bricks. Recommended compressive strength of bricks varies in different standards and depends on the use of bricks also. In Indian standards, the minimum recommended compressive strength is around 3MPa while in ASTM standards this value is around 15MPa. Generally, good-quality bricks have compressive strength between 5–15 MPA [19]. The flexural strength (indirect tensile strength) of fired bricks is directly proportional to the compressive strength of bricks. In Table 10, it can be observed that T1 and T2 sediments have low tensile strength ranging from 0.17 to 1.23 MPa while T5 and J4 sediments have high tensile strength ranging from 2.31 to 12.82 MPa. The trend is similar to the compressive strength.
The modulus of elasticity of bricks of Usumacinta sediments was determined with a compressive strength test. The modulus of elasticity of Usumacinta bricks ranges from 2 to 1018 MPa. The modulus of elasticity increases with increasing compressive strength of bricks. Therefore, J4 and T5 sediments have a higher modulus of elasticity while T2 sediments have a low modulus of elasticity as their compressive strength is very low. For most of the Usumacinta bricks, the elasticity modulus is below 400 MPa. The relationship between compressive strength and modulus of elasticity for Usumacinta sediments is shown in Figure 7a. The relationship between flexural strength and compressive strength for fired bricks from Usumacinta river sediments is shown in Figure 7b. Compressive strength is around 2.14 times flexural strength. The typical ratio value for concrete is around 10 but for bricks, this value is low as the compressive and flexural strength of fired bricks is low when compared with concrete.
Mechanical characteristics of fired bricks show that the strength of fired bricks is heavily influenced by grain size and mineralogy. The percentage of fine particles and clay minerals are some important factors that influence the strength of bricks. T2 sediments which have a high amount of sand and low MBV values have very low compressive and tensile strength, and bricks from these sediments are fragile. T6 and J3 sediments bricks have a good compressive and tensile strength at high temperatures. A higher strength of bricks is obtained at a moderate temperature with T5 and J4 sediments which have a comparatively lower percentage of carbonates (i.e. calcite and dolomite). Quartz content in J4 sediments is considerably lower than other sediments while the presence of clay minerals in J4 sediments i.e. montmorillonite (17%), illite (6.4%), vermiculite (17.1%) is significantly higher than other sediments. Clay minerals are responsible for plasticity in sediments which is essential for the strength of bricks. The effect of the oxide content of sediments on fired bricks is unclear as all the sediments have suitable oxide content but the strength of bricks has significant variation. Winkler's approach of fired bricks seems to better address the sediment's suitability for fired bricks as sediments (T5, J4) close to the zone suitable for bricks show good strength.
The influence of size on the strength of Usumacinta bricks was observed by manufacturing fired bricks of size 4 × 4 × 4 cm3 with Usumacinta River sediments (T5) fired at 850 ℃. The strength of fired bricks from Usumacinta River sediments were compared to bricks made from traditional materials, fiber-reinforced Usumacinta sediments-based earth bricks and lime-stabilized earth bricks from Usumacinta sediments [47]. The compressive strength and physical characteristics of some sediments-based bricks in literature are shown in Table 11.
Table 10 shows that there is a variation in the strength of bricks manufactured with sediments. The strength of Usumacinta River sediments-based fired bricks is lower than the bricks made with traditional soils. Appropriate production methods (extrusion instead of molding), the addition of industrial byproducts such as slag or ash and higher firing temperature are a few important elements that can be considered to increase the strength of fired bricks [48,49]. In addition, low-strength Usumacinta River sediments-based bricks can be used for local sustainable construction including walls for agriculture and habitats for the population (structural and non-load-bearing applications such as walls, pavements, etc.) according to the strength of the bricks.
In this research, Usumacinta River sediment characteristics were investigated for their use in fired bricks. Sediment texture shows that most of the sediments are sandy loam except T5 and J4 which have high clay content and T2 which are sandy sediments. Usumacinta sediments have a low organic matter which is below 5% for most of the sediments. The carbonate content of sediments is also low and varies from 1.9% to 8.5%. Sediment chemical composition shows SiO2 and Al2O3 are major oxides in Usumacinta sediments. The presence of pollutants in Usumacinta sediments is negligible.
Sediment suitability for fired bricks was observed with industrial approaches including the Winkler diagram, clay workability chart and Augustinik diagram which suggest that sediments can be used for fired bricks. Usumacinta sediments were directly used for fired bricks without using any additive. Bricks were manufactured at a firing temperature of 700 to 1100 ℃. T5 and J4 sediments have high compressive and tensile strength. The maximum compressive strength for T5 and J4 sediment at 1100 ℃ is 16.67 and 19.38 MPa respectively. T2 sediments have the lowest compressive strength which is around 2 MPa at 1100 ℃. J4 sediments have compressive strength above 15 MPa at all temperature range from 700 to 1100 ℃ and satisfy the European and ASTM standards. The compressive strength of both T5 and J4 sediments is considerably high even at a moderate temperature of 850 ℃. Overall, Usumacinta sediment bricks exhibit good compressive strength and satisfy the strength recommendations of fired bricks, especially at 1100 ℃.
The authors declare they have not used Artificial Intelligence (AI) tools in the creation of this article.
This work has been funded by the project "From traditional uses to an integrated valorization of sediments in the Usumacinta River basin (VAL-USES)" from the Agence Nationale de la Recherche of France (ANR-17-CE03-0012-01) and the Consejo Nacional de Ciencia y Tecnología of Mexico (FONCICYT-290792).
Conceptualization, D.L. and M. H.; Experimental work, M.H. and A.H.; Analysis, M.H.; Writing, M. H. and D.L.; Supervision, D.L, N.L. and H.Z.; Review and editing, M.H.; and D.L.; Project administration, D.L., I.D.-M., A.R. and N.L.; Funding acquisition, D.L., N.L., I.D.-M. All authors have read and agreed to the published version of the manuscript.
All authors declare no conflicts of interest in this paper.
[1] |
M. A. Chaudhry, A. Qadir, Incomplete exponential and hypergeometric functions with applications to the non central χ2 -distribution, Commun. Stat. Theor. M., 34 (2005), 525–535. https://doi.org/10.1081/STA-200052154 doi: 10.1081/STA-200052154
![]() |
[2] |
H. M. Srivastava, M. A. Chaudhry, R. P. Agarwal, The incomplete Pochhammer symbols and their applications tohypergeometric and related functions, Integr. Trans. Spec. Funct., 23 (2012), 659–683. https://doi.org/10.1080/10652469.2011.623350 doi: 10.1080/10652469.2011.623350
![]() |
[3] |
R. Desai, A. K. Shukla, Some results on function pRq(α,β;z), J. Math. Anal. Appl., 448 (2017), 187–197. https://doi.org/10.1016/j.jmaa.2016.10.048 doi: 10.1016/j.jmaa.2016.10.048
![]() |
[4] |
L. Jódar, J. C. Cortés, Some properties of Gamma and Beta matrix functions, Appl. Math. Lett., 11 (1998), 89–93. https://doi.org/10.1016/S0893-9659(97)00139-0 doi: 10.1016/S0893-9659(97)00139-0
![]() |
[5] |
L. Jódar, J. C. Cortés, On the hypergeometric matrix function, J. Comput. Appl. Math., 99 (1998), 205–217. https://doi.org/10.1016/S0377-0427(98)00158-7 doi: 10.1016/S0377-0427(98)00158-7
![]() |
[6] |
L. Jódar, J. C. Cortés, Closed form general solution of the hypergeometric matrix differential equation, Math. Comput. Model., 32 (2000), 1017–1028. https://doi.org/10.1016/S0895-7177(00)00187-4 doi: 10.1016/S0895-7177(00)00187-4
![]() |
[7] |
F. He, A. Bakhet, M. Hidan, M. Abdalla, Two variables Shivley's matrix polynomials, Symmetry, 11 (2019), 151. https://doi.org/10.3390/sym11020151 doi: 10.3390/sym11020151
![]() |
[8] |
S. Khan, N. A. M. Hassan, 2-variables Laguerre matrix polynomials and Lie-algebraic techniques, J. Phys. A Math. Theor., 43 (2010), 235204. https://doi.org/10.1088/1751-8113/43/23/235204 doi: 10.1088/1751-8113/43/23/235204
![]() |
[9] |
R. S. Batahan, A new extension of Hermite matrix polynomials and its applications, Linear Algebra Appl., 419 (2006), 82–92. https://doi.org/10.1016/j.laa.2006.04.006 doi: 10.1016/j.laa.2006.04.006
![]() |
[10] |
H. M. Srivastava, W. A. Khan, H. Haroon, Some expansions for a class of generalized Humbert matrix polynomials, RACSAM, 113 (2019), 3619–3634. https://doi.org/10.1007/s13398-019-00720-6 doi: 10.1007/s13398-019-00720-6
![]() |
[11] |
S. Khan, N. Raza, 2-variable generalized Hermite matrix polynomials and Lie algebra representation, Rep. Math. Phys., 66 (2010), 159–174. https://doi.org/10.1016/S0034-4877(10)00024-8 doi: 10.1016/S0034-4877(10)00024-8
![]() |
[12] |
S. Khan, A. Al-Gonah, Multi-variable Hermite matrix polynomials: Properties and applications, J. Math. Anal. Appl., 412 (2014), 222–235. https://doi.org/10.1016/j.jmaa.2013.10.037 doi: 10.1016/j.jmaa.2013.10.037
![]() |
[13] | G. S. Kahmmash, A study of a two variables Gegenbauer matrix polynomials and second order matrix partial differential equations, Int. J. Math. Anal., 2 (2008), 807–821. |
[14] |
L. Kargin, V. Kurt, Chebyshev-type matrix polynomials and integral transforms, Hacet. J. Math. Stat., 44 (2015), 341–350. https://doi.org/10.15672/HJMS.2015449102 doi: 10.15672/HJMS.2015449102
![]() |
[15] |
J. Sastre, L. Jódar, Asymptotics of the modified Bessel and incomplete gamma matrix functions, Appl. Math. Lett., 16 (2003), 815–820. https://doi.org/10.1016/S0893-9659(03)90001-2 doi: 10.1016/S0893-9659(03)90001-2
![]() |
[16] | L. Jódar, R. Company, E. Navarro, Solving explicitly the Bessel matrix differential equation, without increasing problem dimension, Congr. Numer., 92 (1993), 261–276. |
[17] |
R. Dwivedi, R. Sanjhira, On the matrix function pRq(A,B;z) and its fractional calculus properties, Commun. Math., 31 (2023), 43–56. https://doi.org/10.46298/cm.10205 doi: 10.46298/cm.10205
![]() |
[18] |
A. Bakhet, F. He, On the matrix version of extended Struve function and its application on fractional calculus, Filomat, 36 (2022), 3381–3392. https://doi.org/10.2298/FIL2210381B doi: 10.2298/FIL2210381B
![]() |
[19] |
T. Cuchta, D. Growb, N. Wintz, Discrete matrix hypergeometric functions, J. Math. Anal. Appl., 518 (2023), 126716. https://doi.org/10.1016/j.jmaa.2022.126716 doi: 10.1016/j.jmaa.2022.126716
![]() |
1. | Madiha Salhi, Ridha Abdeljabar, Khaled Kharrati, Improvement of Tozeur’s fired bricks properties: an experimental approach, 2024, 2365-6433, 10.1007/s41207-024-00701-1 |
Sediments | Latitude | Longitude |
T1 | 17.43126 | −91.4875 |
T2 | 17.44998 | −91.4882 |
T5 | 17.44998 | −91.4882 |
T6 | 17.43205 | −91.4872 |
J3 | 18.08497 | −92.0798 |
J4 | 18.09736 | −92.0642 |
Sediments | T1 | T2 | T5 | T6 | J3 | J4 |
W (%) | 29.8 | 23.4 | 26.4 | 25.1 | 29.6 | 42.9 |
Sediments | OM (%) | MBV (g/100g) | CaCO3 (%) | Wopt (%) | ρsed (g/cm3) | pH (-) | LL (%) | Clay (%) | Silt (%) | Sand (%) |
T1 | 3.77 | 2.53 | 8.21 | 17.30 | 2.70 | 8.04 | 39.01 | 6.90 | 40.20 | 52.90 |
T2 | 3.50 | 2.00 | 7.19 | 15.50 | 2.71 | 8.21 | 28.75 | 3.57 | 31.08 | 65.35 |
T5 | 3.46 | 5.90 | 1.90 | 20.80 | 2.61 | 8.17 | 36.75 | 8.75 | 45.90 | 45.00 |
T6 | 4.90 | 1.93 | 8.73 | 17.90 | 2.68 | 8.61 | 30.71 | 6.09 | 40.70 | 53.20 |
J3 | 4.73 | 3.61 | 8.19 | 18.80 | 2.70 | 8.01 | 38.91 | 4.66 | 36.30 | 59.00 |
J4 | 5.72 | > 8.00 | 8.49 | 21.00 | 2.53 | 8.51 | 61.98 | 13.40 | 62.50 | 24.10 |
Sediments | Elemental composition | Oxide composition | ||||||||||||||
O (%) | Al (%) | Si (%) | Ca (%) | Mg (%) | Fe (%) | K (%) | Na (%) | P (%) | SiO2 (%) | Al2O3 (%) | TiO2 (%) | Fe2O3 (%) | CaO (%) | K2O (%) | ||
T1 | 51.98 | 7.51 | 38.47 | 1.43 | 0.61 | - | - | - | - | 55.4 | 12.2 | 1.2 | 10.9 | 16.0 | 3.6 | |
T2 | 57.77 | 9.04 | 22.28 | 2.01 | 2.57 | 3.89 | 1.31 | 1.12 | 0.00 | 55.4 | 11.7 | 1.2 | 8.5 | 11.2 | 3.7 | |
T5 | 59.74 | 9.60 | 30.36 | 0.00 | 0.29 | - | - | - | - | 62.8 | 14.7 | 1.0 | 9.1 | 8.3 | 3.2 | |
T6 | 50.69 | 8.54 | 36.99 | 3.25 | 0.52 | - | - | - | - | 59.6 | 12.2 | 1.1 | 9.8 | 12.1 | 4.2 | |
J3 | 54.88 | 8.66 | 22.42 | 3.75 | 1.52 | 4.66 | 2.88 | 0.34 | 0.90 | 59.3 | 14.6 | 1.0 | 9.7 | 11.2 | 3.5 | |
J4 | 57.06 | 12.11 | 26.18 | 5.27 | 0.30 | - | - | 0.18 | - | 56.3 | 16.1 | 1.9 | 16.1 | 6.4 | 2.6 |
Range | LOI | SiO2 | Al2O3 | TiO2 | Fe2O3 | CaO | MgO | Na2O | K2O | S | F |
Minimum % | 3 | 35 | 8 | 0.3 | 2 | 0.5 | 0 | 0.1 | 0.1 | 0 | 0 |
Maximum % | 18 | 80 | 30 | 2 | 10 | 18 | 5 | 1.5 | 4.5 | 0.5 | 0.15 |
Mnt (%) | Ilt (%) | Vrm (%) | Bt (%) | Qz (%) | Cal (%) | Dol (%) | Crs (%) | Or (%) | Ano (%) | Ab (%) | NIM (%) | |
T1 | 2.6 | 5.1 | 3.1 | 3.1 | 43.7 | 7.1 | 18.4 | 2.5 | 4.0 | 5.3 | 3.2 | 1.9 |
T2 | 4.0 | 2.2 | 2.2 | 2.7 | 52.0 | 4.8 | 14.1 | 3.2 | 3.8 | 5.9 | 3.9 | 1.2 |
T5 | 5.1 | 5.4 | 3.0 | 2.6 | 50.5 | 3.0 | 9.7 | 1.7 | 3.1 | 5.4 | 3.2 | 7.3 |
T6 | 3.4 | 2.3 | 3.1 | 2.8 | 48.7 | 3.9 | 14.7 | 2.6 | 4.0 | 9.2 | 3.8 | 1.5 |
J3 | 3.6 | 2.6 | 2.9 | 2.9 | 48.4 | 5.6 | 16.5 | 2.9 | 4.2 | 5.9 | 3.0 | 1.5 |
J4 | 10.0 | 6.4 | 17.1 | 7.0 | 21.4 | 2.2 | 10.1 | 1.6 | 5.3 | 9.6 | 4.3 | 5 |
Mnt = montmorillonite, Ilt = illite, Vrm = vermiculite, Bt = biotite, Qz = quartz, Cal = calcite, Dol = dolomite, Crs = cristobalite, Or = orthoclase, Ano = anorthoclase, Ab =albite, NIM = non-identified minerals. |
Sediments | 200 (%) | 300–550 (%) | 550–800 (%) | Total loss (%) |
T1 | 1.65 | 2.14 | 7.08 | 10.86 |
T2 | 1.21 | 1.82 | 6.95 | 10.60 |
T5 | 3.65 | 2.53 | 1.87 | 8.04 |
T6 | 1.93 | 2.25 | 7.68 | 11.86 |
J3 | 2.38 | 2.54 | 7.78 | 12.69 |
J4 | 4.18 | 2.15 | 9.28 | 15.60 |
Sediments | Linear shrinkage | Density | |||||||||
LS (%) 700 ℃ | LS (%) 800 ℃ | LS (%) 900 ℃ | LS (%) 1000 ℃ | LS (%) 1100 ℃ | ρ (kg/m3) 700 ℃ | ρ (kg/m3) 800 ℃ | ρ (kg/m3) 900 ℃ | ρ (kg/m3) 1000 ℃ | ρ (kg/m3) 1100 ℃ | ||
T1 | 0.6 | 0.9 | 1.6 | 0.7 | 1.7 | 994 | 1268 | 1303 | 1188 | 1244 | |
T2 | - | - | - | - | - | 1146 | 1155 | 1148 | - | 1320 | |
T5 | 10.7 | 10.2 | 12.8 | 11.1 | 13.2 | 1683 | 1606 | 1724 | 1672 | 1818 | |
T6 | 1.2 | 2.1 | 2.3 | 3.4 | 3.4 | 1321 | 1337 | 1277 | 1358 | 1432 | |
J3 | 4.9 | 4.6 | 4.6 | 5.7 | 6.9 | 1331 | 1281 | 1226 | 1302 | 1401 | |
J4 | 12.7 | 13.9 | 12.9 | 12.1 | 13.5 | 1702 | 1588 | 1554 | 1666 | 1637 |
Sediments | T1 | T2 | T5 | T6 | J3 | J4 |
WA (%) | 22.4 | 17.6 | 10.7 | 19.3 | 21.5 | 16.2 |
Sediments | 700 ℃ | 800 ℃ | 850 ℃ | 900 ℃ | 1000 ℃ | 1100 ℃ | |
Compressive strength (MPa) | T1 | 0.40 | 0.57 | 0.53 | 1.84 | 0.68 | 2.69 |
T2 | 0.10 | 0.12 | 0.94 | 0.83 | 0.67 | 2.00 | |
T5 | 15.51 | 7.59 | 4.92 | 13.15 | 9.04 | 16.67 | |
T6 | 1.33 | 2.18 | 2.04 | 2.93 | 1.93 | 6.75 | |
J3 | 2.46 | 3.50 | 3.00 | 6.25 | 3.76 | 9.05 | |
J4 | 17.04 | 19.00 | 19.15 | 15.57 | 15.65 | 19.38 | |
Flexural strength (MPa) | T1 | 0.17 | 0.28 | 0.45 | 0.43 | 0.64 | 1.23 |
T2 | 0.20 | - | 0.63 | - | 0.31 | 0.90 | |
T5 | 3.55 | 2.31 | 2.23 | 4.57 | 4.17 | 6.60 | |
T6 | 0.35 | 0.99 | 0.96 | 1.36 | 1.78 | 3.00 | |
J3 | 0.92 | 1.37 | 1.92 | 3.84 | 2.15 | 4.86 | |
J4 | 6.35 | 9.01 | 7.66 | 7.30 | 12.82 | 8.68 |
References | Sediment origin | Sediment (%) | Others (%) | Firing temp. (℃) | UCS (MPa) | WSC (%) | Porosity (%) | Density (g/cm3) |
Anger (2014) | Dam sed | 80–100 | Kaolinite | 800–1150 | 7.5–10 | 20–31 | 41–51 | 1.36–1.52 |
Ben Allal et al. (2011) | Port sed | 0–70 | Clay | 920 | 6.8–33 | 10–33 | 18–45 | 1.81–1.37 |
Benkadja et al. (2013) | Dam sed | 0–65 | sand | 800 | 55 | |||
Boulingui et al. (2015) | Mined clay | 900–1150 | ||||||
Chiang et al. (2008) | Dam sed | 80–100 | Clay | 1050–1150 | ||||
Haurine (2015) | Dam sed | 70–100 | sand | 950–1100 | 5–50 | |||
Labiod et al. (2004) | Dam sed | 100 | - | 5–9 | ||||
Liang & Li (2015) | Dam sed | Gypsum | 1100 | |||||
Marouf et al. (2018) | Dam sed | 850–1050 | 21 | 30–40 | ||||
Nedloussi et al. (2019) | Dam sed | 80–100 | Sand | 600–900 | 28–46 | |||
Remini (2006) | Dam sed | 0–100 | clay | 900 | 10–40 | 6–20 | 12–24 | 1.4–1.9 |
Romero et al. (2009) | Harbour sed | 100 | - | 900–1200 | 34 | 4-22 | 12–38 | 1.60–2.45 |
Samara et al. (2009) | River sed | 100 | - | 1000 | 36 | 7.5 | 15.4 | - |
Tangprasert et al. (2015) | River sed | 80–100 | Husks | 700 | 1.9–9 | 17–29 | - | 1.13–1.57 |
Torres et al. (2009) | River sed | 5–10 | Clay | 950–1100 | 7.5–35 | 3.5–15 | - | - |
Wei et al. (2014a) | Harbour sed | Slag | 950–1100 | |||||
Xu et al. (2014) | River sed | 50–80 | 1100 | |||||
Note: sed = sediments; sediment rate: sediment proportion in mixture; others: other components in mixture; UCS: unconfined compressive strength; WSC: water sorption capacity (24h); PI: plasticity index; firing temp.: firing temperature; 880–1100: optimal values of temperature. |
Sediments | Latitude | Longitude |
T1 | 17.43126 | −91.4875 |
T2 | 17.44998 | −91.4882 |
T5 | 17.44998 | −91.4882 |
T6 | 17.43205 | −91.4872 |
J3 | 18.08497 | −92.0798 |
J4 | 18.09736 | −92.0642 |
Sediments | T1 | T2 | T5 | T6 | J3 | J4 |
W (%) | 29.8 | 23.4 | 26.4 | 25.1 | 29.6 | 42.9 |
Sediments | OM (%) | MBV (g/100g) | CaCO3 (%) | Wopt (%) | ρsed (g/cm3) | pH (-) | LL (%) | Clay (%) | Silt (%) | Sand (%) |
T1 | 3.77 | 2.53 | 8.21 | 17.30 | 2.70 | 8.04 | 39.01 | 6.90 | 40.20 | 52.90 |
T2 | 3.50 | 2.00 | 7.19 | 15.50 | 2.71 | 8.21 | 28.75 | 3.57 | 31.08 | 65.35 |
T5 | 3.46 | 5.90 | 1.90 | 20.80 | 2.61 | 8.17 | 36.75 | 8.75 | 45.90 | 45.00 |
T6 | 4.90 | 1.93 | 8.73 | 17.90 | 2.68 | 8.61 | 30.71 | 6.09 | 40.70 | 53.20 |
J3 | 4.73 | 3.61 | 8.19 | 18.80 | 2.70 | 8.01 | 38.91 | 4.66 | 36.30 | 59.00 |
J4 | 5.72 | > 8.00 | 8.49 | 21.00 | 2.53 | 8.51 | 61.98 | 13.40 | 62.50 | 24.10 |
Sediments | Elemental composition | Oxide composition | ||||||||||||||
O (%) | Al (%) | Si (%) | Ca (%) | Mg (%) | Fe (%) | K (%) | Na (%) | P (%) | SiO2 (%) | Al2O3 (%) | TiO2 (%) | Fe2O3 (%) | CaO (%) | K2O (%) | ||
T1 | 51.98 | 7.51 | 38.47 | 1.43 | 0.61 | - | - | - | - | 55.4 | 12.2 | 1.2 | 10.9 | 16.0 | 3.6 | |
T2 | 57.77 | 9.04 | 22.28 | 2.01 | 2.57 | 3.89 | 1.31 | 1.12 | 0.00 | 55.4 | 11.7 | 1.2 | 8.5 | 11.2 | 3.7 | |
T5 | 59.74 | 9.60 | 30.36 | 0.00 | 0.29 | - | - | - | - | 62.8 | 14.7 | 1.0 | 9.1 | 8.3 | 3.2 | |
T6 | 50.69 | 8.54 | 36.99 | 3.25 | 0.52 | - | - | - | - | 59.6 | 12.2 | 1.1 | 9.8 | 12.1 | 4.2 | |
J3 | 54.88 | 8.66 | 22.42 | 3.75 | 1.52 | 4.66 | 2.88 | 0.34 | 0.90 | 59.3 | 14.6 | 1.0 | 9.7 | 11.2 | 3.5 | |
J4 | 57.06 | 12.11 | 26.18 | 5.27 | 0.30 | - | - | 0.18 | - | 56.3 | 16.1 | 1.9 | 16.1 | 6.4 | 2.6 |
Range | LOI | SiO2 | Al2O3 | TiO2 | Fe2O3 | CaO | MgO | Na2O | K2O | S | F |
Minimum % | 3 | 35 | 8 | 0.3 | 2 | 0.5 | 0 | 0.1 | 0.1 | 0 | 0 |
Maximum % | 18 | 80 | 30 | 2 | 10 | 18 | 5 | 1.5 | 4.5 | 0.5 | 0.15 |
Mnt (%) | Ilt (%) | Vrm (%) | Bt (%) | Qz (%) | Cal (%) | Dol (%) | Crs (%) | Or (%) | Ano (%) | Ab (%) | NIM (%) | |
T1 | 2.6 | 5.1 | 3.1 | 3.1 | 43.7 | 7.1 | 18.4 | 2.5 | 4.0 | 5.3 | 3.2 | 1.9 |
T2 | 4.0 | 2.2 | 2.2 | 2.7 | 52.0 | 4.8 | 14.1 | 3.2 | 3.8 | 5.9 | 3.9 | 1.2 |
T5 | 5.1 | 5.4 | 3.0 | 2.6 | 50.5 | 3.0 | 9.7 | 1.7 | 3.1 | 5.4 | 3.2 | 7.3 |
T6 | 3.4 | 2.3 | 3.1 | 2.8 | 48.7 | 3.9 | 14.7 | 2.6 | 4.0 | 9.2 | 3.8 | 1.5 |
J3 | 3.6 | 2.6 | 2.9 | 2.9 | 48.4 | 5.6 | 16.5 | 2.9 | 4.2 | 5.9 | 3.0 | 1.5 |
J4 | 10.0 | 6.4 | 17.1 | 7.0 | 21.4 | 2.2 | 10.1 | 1.6 | 5.3 | 9.6 | 4.3 | 5 |
Mnt = montmorillonite, Ilt = illite, Vrm = vermiculite, Bt = biotite, Qz = quartz, Cal = calcite, Dol = dolomite, Crs = cristobalite, Or = orthoclase, Ano = anorthoclase, Ab =albite, NIM = non-identified minerals. |
Sediments | 200 (%) | 300–550 (%) | 550–800 (%) | Total loss (%) |
T1 | 1.65 | 2.14 | 7.08 | 10.86 |
T2 | 1.21 | 1.82 | 6.95 | 10.60 |
T5 | 3.65 | 2.53 | 1.87 | 8.04 |
T6 | 1.93 | 2.25 | 7.68 | 11.86 |
J3 | 2.38 | 2.54 | 7.78 | 12.69 |
J4 | 4.18 | 2.15 | 9.28 | 15.60 |
Sediments | Linear shrinkage | Density | |||||||||
LS (%) 700 ℃ | LS (%) 800 ℃ | LS (%) 900 ℃ | LS (%) 1000 ℃ | LS (%) 1100 ℃ | ρ (kg/m3) 700 ℃ | ρ (kg/m3) 800 ℃ | ρ (kg/m3) 900 ℃ | ρ (kg/m3) 1000 ℃ | ρ (kg/m3) 1100 ℃ | ||
T1 | 0.6 | 0.9 | 1.6 | 0.7 | 1.7 | 994 | 1268 | 1303 | 1188 | 1244 | |
T2 | - | - | - | - | - | 1146 | 1155 | 1148 | - | 1320 | |
T5 | 10.7 | 10.2 | 12.8 | 11.1 | 13.2 | 1683 | 1606 | 1724 | 1672 | 1818 | |
T6 | 1.2 | 2.1 | 2.3 | 3.4 | 3.4 | 1321 | 1337 | 1277 | 1358 | 1432 | |
J3 | 4.9 | 4.6 | 4.6 | 5.7 | 6.9 | 1331 | 1281 | 1226 | 1302 | 1401 | |
J4 | 12.7 | 13.9 | 12.9 | 12.1 | 13.5 | 1702 | 1588 | 1554 | 1666 | 1637 |
Sediments | T1 | T2 | T5 | T6 | J3 | J4 |
WA (%) | 22.4 | 17.6 | 10.7 | 19.3 | 21.5 | 16.2 |
Sediments | 700 ℃ | 800 ℃ | 850 ℃ | 900 ℃ | 1000 ℃ | 1100 ℃ | |
Compressive strength (MPa) | T1 | 0.40 | 0.57 | 0.53 | 1.84 | 0.68 | 2.69 |
T2 | 0.10 | 0.12 | 0.94 | 0.83 | 0.67 | 2.00 | |
T5 | 15.51 | 7.59 | 4.92 | 13.15 | 9.04 | 16.67 | |
T6 | 1.33 | 2.18 | 2.04 | 2.93 | 1.93 | 6.75 | |
J3 | 2.46 | 3.50 | 3.00 | 6.25 | 3.76 | 9.05 | |
J4 | 17.04 | 19.00 | 19.15 | 15.57 | 15.65 | 19.38 | |
Flexural strength (MPa) | T1 | 0.17 | 0.28 | 0.45 | 0.43 | 0.64 | 1.23 |
T2 | 0.20 | - | 0.63 | - | 0.31 | 0.90 | |
T5 | 3.55 | 2.31 | 2.23 | 4.57 | 4.17 | 6.60 | |
T6 | 0.35 | 0.99 | 0.96 | 1.36 | 1.78 | 3.00 | |
J3 | 0.92 | 1.37 | 1.92 | 3.84 | 2.15 | 4.86 | |
J4 | 6.35 | 9.01 | 7.66 | 7.30 | 12.82 | 8.68 |
References | Sediment origin | Sediment (%) | Others (%) | Firing temp. (℃) | UCS (MPa) | WSC (%) | Porosity (%) | Density (g/cm3) |
Anger (2014) | Dam sed | 80–100 | Kaolinite | 800–1150 | 7.5–10 | 20–31 | 41–51 | 1.36–1.52 |
Ben Allal et al. (2011) | Port sed | 0–70 | Clay | 920 | 6.8–33 | 10–33 | 18–45 | 1.81–1.37 |
Benkadja et al. (2013) | Dam sed | 0–65 | sand | 800 | 55 | |||
Boulingui et al. (2015) | Mined clay | 900–1150 | ||||||
Chiang et al. (2008) | Dam sed | 80–100 | Clay | 1050–1150 | ||||
Haurine (2015) | Dam sed | 70–100 | sand | 950–1100 | 5–50 | |||
Labiod et al. (2004) | Dam sed | 100 | - | 5–9 | ||||
Liang & Li (2015) | Dam sed | Gypsum | 1100 | |||||
Marouf et al. (2018) | Dam sed | 850–1050 | 21 | 30–40 | ||||
Nedloussi et al. (2019) | Dam sed | 80–100 | Sand | 600–900 | 28–46 | |||
Remini (2006) | Dam sed | 0–100 | clay | 900 | 10–40 | 6–20 | 12–24 | 1.4–1.9 |
Romero et al. (2009) | Harbour sed | 100 | - | 900–1200 | 34 | 4-22 | 12–38 | 1.60–2.45 |
Samara et al. (2009) | River sed | 100 | - | 1000 | 36 | 7.5 | 15.4 | - |
Tangprasert et al. (2015) | River sed | 80–100 | Husks | 700 | 1.9–9 | 17–29 | - | 1.13–1.57 |
Torres et al. (2009) | River sed | 5–10 | Clay | 950–1100 | 7.5–35 | 3.5–15 | - | - |
Wei et al. (2014a) | Harbour sed | Slag | 950–1100 | |||||
Xu et al. (2014) | River sed | 50–80 | 1100 | |||||
Note: sed = sediments; sediment rate: sediment proportion in mixture; others: other components in mixture; UCS: unconfined compressive strength; WSC: water sorption capacity (24h); PI: plasticity index; firing temp.: firing temperature; 880–1100: optimal values of temperature. |