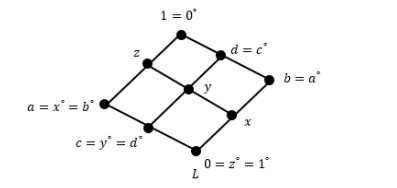
The finite-time H∞ performance of the interval type-2 Takagi-Sugeno fuzzy system (IT2 T-S) in presence of immeasurable states and input saturation is investigated. At first, an observer associated with IT2 T-S states is considered to address the problem of immeasurable states. After that, the input saturation is described based on the polyhedron model, and accordingly, a robust H∞ observer-based finite-time controller is proposed via non-PDC algorithm. Then, on the basis of the Lyapunov function method and LMIs theory, the sufficient conditions for the finite time stability of fuzzy systems are derived. At last, the feasibility of the designed algorithm is verified by two examples of the nonlinear mass-spring-damping system and tunnel diode circuit system, respectively.
Citation: Chuang Liu, Jinxia Wu, Weidong Yang. Robust H∞ output feedback finite-time control for interval type-2 fuzzy systems with actuator saturation[J]. AIMS Mathematics, 2022, 7(3): 4614-4635. doi: 10.3934/math.2022257
[1] | Ilyasse Lamrani, Imad El Harraki, M. A. Aziz-Alaoui, Fatima-Zahrae El Alaoui . Feedback stabilization for prey predator general model with diffusion via multiplicative controls. AIMS Mathematics, 2023, 8(1): 2360-2385. doi: 10.3934/math.2023122 |
[2] | Shanshan Yu, Jiang Liu, Xiaojie Lin . Multiple positive periodic solutions of a Gause-type predator-prey model with Allee effect and functional responses. AIMS Mathematics, 2020, 5(6): 6135-6148. doi: 10.3934/math.2020394 |
[3] | Heping Jiang . Complex dynamics induced by harvesting rate and delay in a diffusive Leslie-Gower predator-prey model. AIMS Mathematics, 2023, 8(9): 20718-20730. doi: 10.3934/math.20231056 |
[4] | Binfeng Xie, Na Zhang . Influence of fear effect on a Holling type III prey-predator system with the prey refuge. AIMS Mathematics, 2022, 7(2): 1811-1830. doi: 10.3934/math.2022104 |
[5] | Jagdev Singh, Behzad Ghanbari, Ved Prakash Dubey, Devendra Kumar, Kottakkaran Sooppy Nisar . Fractional dynamics and computational analysis of food chain model with disease in intermediate predator. AIMS Mathematics, 2024, 9(7): 17089-17121. doi: 10.3934/math.2024830 |
[6] | Chuanfu Chai, Yuanfu Shao, Yaping Wang . Analysis of a Holling-type IV stochastic prey-predator system with anti-predatory behavior and Lévy noise. AIMS Mathematics, 2023, 8(9): 21033-21054. doi: 10.3934/math.20231071 |
[7] | Anel Esquivel-Navarrete, Jorge Sanchez-Ortiz, Gabriel Catalan-Angeles, Martin P. Arciga-Alejandre . Tritrophic fractional model with Holling III functional response. AIMS Mathematics, 2024, 9(6): 15937-15948. doi: 10.3934/math.2024771 |
[8] | Mianjian Ruan, Chang Li, Xianyi Li . Codimension two 1:1 strong resonance bifurcation in a discrete predator-prey model with Holling Ⅳ functional response. AIMS Mathematics, 2022, 7(2): 3150-3168. doi: 10.3934/math.2022174 |
[9] | Chengchong Lu, Xinxin Liu, Zhicheng Li . The dynamics and harvesting strategies of a predator-prey system with Allee effect on prey. AIMS Mathematics, 2023, 8(12): 28897-28925. doi: 10.3934/math.20231481 |
[10] | Chuangliang Qin, Jinji Du, Yuanxian Hui . Dynamical behavior of a stochastic predator-prey model with Holling-type III functional response and infectious predator. AIMS Mathematics, 2022, 7(5): 7403-7418. doi: 10.3934/math.2022413 |
The finite-time H∞ performance of the interval type-2 Takagi-Sugeno fuzzy system (IT2 T-S) in presence of immeasurable states and input saturation is investigated. At first, an observer associated with IT2 T-S states is considered to address the problem of immeasurable states. After that, the input saturation is described based on the polyhedron model, and accordingly, a robust H∞ observer-based finite-time controller is proposed via non-PDC algorithm. Then, on the basis of the Lyapunov function method and LMIs theory, the sufficient conditions for the finite time stability of fuzzy systems are derived. At last, the feasibility of the designed algorithm is verified by two examples of the nonlinear mass-spring-damping system and tunnel diode circuit system, respectively.
MS-algebras were considered by T. S. Blyth and J. C. Varlet[15] as a common properties of de Morgan algebras and Stone algebras. T. S. Blyth and J. C. Varlet[16] described the lattice Λ(MS) of all subclasses of the class MS of all MS-algebras by identities.
A. Badawy, D. Guffovˊa and M. Haviar[10] introduced and characterized the class of principal MS-algebras and the class of decomposable MS-algebras by means of principal MS-triples and decomposable MS-triples, respectively. They obtained a one-to-one correspondence between principal MS-algebras (decomposable MS-algebras) and principal MS-triples (decomposable MS-triples). Moreover, they proved that the class of principal MS-algebras is a subclass of the class of decomposable MS-algebras.
A. Badawy[6] established the relationship between de Morgan filters and congruences of a decomposable MS-algebra. A. Badawy[3] introduced the notion of dL-filters of a principal MS-algebra and characterized certain congruences in terms of dL-filters. Recently, A. Badawy, M. Haviar and M. Ploˇsˇcica[11] introduced and described the notions of congruence pairs and perfect extensions of principal MS-algebras. They characterized the lattice of congruences of a principal MS-algebra in terms of congruence pairs. In 2021, S. El-Assar and A. Badawy[19] characterized permutability of congruences and strong extensions of decomposable MS-algebras by means of congruence pairs.
We review in Section 2 many basic concepts and results that we are using throughout this article. We give an example (Example 2.1) to determine the principsl MS-triple (L∘∘,D(L),φL) assosiated with a certain principal MS-algebra L. In Section 3, we give equivalent conditions for a pair of congruence (θ1,θ2)∈Con(L∘∘)×Conlat(D(L)) to become an MS-congruence pair of a principal MS-algebra L, where θ1 is a congruence on a de Morgan algebra L∘∘ and θ2 is a lattice congruence on the lattice D(L). Also, we characterize the lattice of all MS- congruence pairs which are induced by the Boolean elements of a principal MS-algebra. In Section 4, we discuss many properties of the congruence relation Ψ which defined on a principal MS-algebra L by
(a,b)∈Ψ⇔a∘=b∘⇔a∘∘=b∘∘. |
We prove that Ψ permutes with each congruence relation θ on L. Also, we characterize 2-permutability of congruences (briefly permutability) by means of MS-congruence pairs. We illustrate Examples 3.1 and 4.1 to clarify Theorems 3.1 and 4.4, respectively. Also, Example 4.2 introduces certain principal MS-algebra L, which has not 2-permutable congruences as well as D(L) has not also 2-permutable congruences. In Section 5, A characterization of n-permutability of congruences of a principal MS-algebra is given, which is a generalization of the characterization of 2-permutability of congruences of such algebras.
In this section, we recall some specific definitions and remarkable results which are discussed in previous articles [10,14,15,16,17,19].
Definition 2.1. [16] A de Morgan algebra is an algebra (L;∨,∧,−,0,1) of type (2, 2, 1, 0, 0), where (L;∨,∧,0,1) is a bounded distributive lattice and the unary operation − satisfying:
(1)¯¯a=a,(2)¯(a∨b)=¯a∧¯b,(3)¯1=0. |
Definition 2.2. [15] A Stone algebra is an algebra (L;∨,∧,∗,0,1) of type (2,2,1,0,0), where (L;∨,∧,0,1) is a bounded distributive lattice and the unary operation ∗ satisfying:
(1)(a∧b)∗=a∗∨b∗,(2)a∗∨a∗∗=1,(3)1∗=0. |
Definition 2.3. [15] An MS-algebra is an algebra (L;∨,∧,∘,0,1) of type (2, 2, 1, 0, 0), where (L;∨,∧,0,1) is a bounded distributive lattice and a unary operation ∘ satisfying:
(1)a≤a∘∘,(2)(x∧y)∘=x∘∨y∘,(3)1∘=0. |
The class MS of all MS-algebras is equational. A de Morgan algebra is an MS-algebra satisfying the identity, a=a∘∘. The class S of a Stone algebra is a subclass of MS satisfying a∧a∘=0.
The basic properties of MS-algebras which were shown in[15] are given in the following theorem.
Theorem 2.1. Let a,b be any two elements of an MS-algebra L. Then
(1) 0∘∘=0 and 1∘∘=1,
(2) a≤b⇒b∘≤a∘,
(3) a∘∘∘=a∘,
(4) a∘∘∘∘=a∘∘,
(5) (a∨b)∘=a∘∧b∘,
(6) (a∨b)∘∘=a∘∘∨b∘∘,
(7) (a∧b)∘∘=a∘∘∧b∘∘.
An element a of an MS-algebra L is called a closed element of L if a=a∘∘ and an element d∈L is called a dense element of L if d∘=0.
Theorem 2.2. Let L be an MS-algebra. Then
(1) the set L∘∘={a∈L:a=a∘∘} of all closed elements of L is a de Morgan subalgebra of L, see [17],
(2) the set D(L)={a∈L:a∘=0} of all dense elements of L is a principal filter of L, see [10].
Definition 2.4. [10] An MS-algebra (L;∨,∧,∘,0,1) is called a principal MS-algebra if it satisfies the following conditions:
(1) the filter D(L) is principal, that is, there exists an element dL∈L such that D(L)=[dL),
(2) a=a∘∘∧(a∨dL), for all a∈L.
A principal MS-algebra L is a principal Stone algebra if x∘∨x∘∘=1, for all x∈L.
Definition 2.5. [8] A principal MS-triple is (M,D,φ), where
(1) M is a de Morgan algebra,
(2) D is a bounded distributive lattice,
(3) φ is a (0,1)-lattice homomorphism from M into D.
Lemma 2.1. [10] Let L be a principal MS-algebra. Define a map φL:L∘∘→[dL) by the following rule
φL(a)=a∨dL,for all a∈L∘∘. |
Then φL is a (0,1)-lattice homomorphism.
Example 2.1. Consider the MS-algebra L in Figure 1.
It is clear that L is a principal MS-algebra with the smallest dense element z. Then the principal triple (L∘∘,D(L)=[z),φL) which associated with L is given in Figure 2.
Definition 2.6. [22] An equivalence relation θ on a lattice L is called a lattice congruence if (a,b)∈θ and (c,d)∈θ imply (a∨c,b∨d)∈θ and (a∧c,b∧d)∈θ.
Definition 2.7. An equivalence relation θ on an MS-algebra L is called a congruence on L if
(1) θ is a lattice congruence,
(2) (a,b)∈θ implies (a∘,b∘)∈θ.
We use Conlat(L) for the lattice of all lattice congruences of a lattice (L;∨,∧) and Con(L) for the lattice of all congruences of an MS-algebra (L;∨,∧,∘,0,1).
Definition 2.8. Let L be a lattice and θ∈Conlat(L). Then we define the principal congruence generated by (a,b) which denoted by θ(a,b) as follows:
θ(a,b)=⋀{θ∈Conlat(L):(a,b)∈θ}. |
If L is an MS-algebra and θ∈Con(L), then
θ(a,b)=⋀{θ∈Con(L):(a,b)∈θ}. |
Let L be an MS-algebra. Then (θL∘∘,θD(L))∈Con(L∘∘)×Conlat(D(L)), where θL∘∘ and θD(L) are the restrictions of θ∈Con(L) to L∘∘ and D(L), respectively. It is clear that θL∘∘ is a congruence relation on a de Morgan algebra L∘∘ and θD(L) is a lattice congruence on a lattice D(L).
The symbols ▽L and △L will be used for the universal congruence L×L and the equality congruence on L, respectively.
The concept of MS-congruence pairs is given as follows:
Definition 2.9. [11] Let L be a principal MS-algebra with a smallest dense element dL. A pair of congruences (θ1,θ2)∈Con(L∘∘)×Conlat(D(L)) will be called an MS-congruence pairs if
(a,b)∈θ1 implies (a∨dL,b∨dL)∈θ2. |
A characterization of congruences on a principal MS-algebra via MS-congruence pairs is given in the following theorem.
Theorem 2.3. [11] Let L be a principal MS-algebra with the smallest dense element dL. For every congruence θ on L, the restrictions of θ to L∘∘ and D(L) determine the MS-congruence pair (θL∘∘,θD(L)).
Conversely, every MS-congruence pair (θ1,θ2) uniquely determines a congruence θ on L satisfying θL∘∘=θ1 and θD(L)=θ2. Such congruence can be defined by the rule
(x,y)∈θ if and only if (x∘,y∘)∈θ1 and (x∨dL,y∨dL)∈θ2. |
Throughout this paper, dL denotes to the smallest dense element of a principal MS-algebra L.
For extra information of MS-algebras, principal MS-algebras and decomposable MS-algebras, we refer the reader to[1,2,3,4,5,6,7,8,9,12,13,14,15,16,17,18,20,21,22,23,24].
In this section, we characterize MS-congruence pairs of a principal MS-algebra and a principal Stone algebra. Also, we describe the lattice of all MS-congruence pairs which induced by the Boolean elements of a principal MS-algebra, in fact such lattice forms a Boolean algebra on it is own.
Lemma 3.1. Let L be a principal MS-algebra and (θ1,θ2) be an MS-congruence pair. Then
(a,b)∈θ1 and (x,y)∈θ2 imply (a∨x,b∨y)∈θ2. |
Proof. Let (a,b)∈θ1 and (x,y)∈θ2. Then (a∨dL,b∨dL)∈θ2 (by Definition 2.9) and (x,y)∈θ2 imply that (a∨x∨dL,b∨y∨dL)∈θ2. Therefore (a∨x,b∨y)∈θ2 as x,y≥dL.
Now, we give an important characterization of MS-congruence pairs of a principal MS-algebra.
Theorem 3.1. Let L be a principal MS-algebra with the smallest dense element dL. Then the following statements are equivalent:
(1) (θ1,θ2) is an MS-congruence pair of L,
(2) ConφL(L)⊆θ2, where
ConφL(θ1)={(φL(a),φL(b)):(a,b)∈θ1} and φL(a)=a∨dL,∀a∈L∘∘. |
Proof. (1⇒2) Let (θ1,θ2) be an MS-congruence pair of L and (x,y)∈ConφL(θ1). Then (x,y)=(φL(a),φL(b))=(a∨dL,b∨dL), where (a,b)∈θ1. Since (a,b)∈θ1, then (a∨dL,b∨dL)∈θ2, by (1). Thus (x,y)∈θ2. So ConφL(θ1)⊆θ2.
(2⇒1) Let Con(θ1)⊆θ2. We prove that (θ1,θ2) is an MS-congruence pair of L. Let (a,b)∈θ1. Then by (2), (a∨dL,b∨dL)=(φL(a),φL(b))∈θ2.
A characterization of congruence pairs of a principal Stone algebra is given in the following.
Theorem 3.2. Let L be a principal Stone algebra. Let (θ1,θ2)∈Con(L∘∘)×Conlat(D(L)). Then the following statements are equivalent:
(1) (θ1,θ2) is an MS-congruence pair of L,
(2) ConφL(θ1)⊆θ2,
(3) (a,1)∈θ1 and u≥a,u∈D(L) imply (u,1)∈θ2.
Proof. In Theorem 3.1, we proved that (1) and (2) are equivalent.
(2⇒3) Let ConφL(θ1)⊆θ2. Let (a,1)∈θ1 and u≥a,u∈D(L). Then (φL(a),φL(1))∈θ2 by (2). Thus (a∨dL,1)∈θ2.
Now (a∨dL,1)∈θ2 and (u,u)∈θ2,u≥a,u∈D(L) imply (a∨u∨dL,1∨u)∈θ2. Thus (u,1)∈θ2 as u≥a,dL.
(3⇒1) Since L is a Stone algebra then L∘∘ is a Boolean subalgebra of L and hence a∨a∘=1 for each a∈L∘∘. Suppose (3) holds and (a,b)∈θ1. Then (b∘,b∘)∈θ1 implies (a∨b∘,b∨b∘)=(a∨b∘,1)∈θ1 and (a∨a∘,b∨a∘)=(b∨a∘,1)∈θ1. Therefore (β,1)∈θ1, where β=(a∨b∘)∧(a∘∨b). It is clear that β∈L∘∘ and β∧a=β∧b=a∧b. Since β≤β∨dL∈D(L) and (β,1)∈θ1 then (β∨dL,1)∈θ2 by (3). Thus (a∨dL,a∨dL)∧(1,β∨dL)∈θ2 implies (a∨dL,(a∧β)∨dL)=(a∨dL,(a∧b)∨dL)∈θ2. Similarly, we can get (b∨dL,(b∧β)∨dL)=(b∨dL,(a∧b)∨dL)∈θ2. Then (b∨dL,b∨dL)∈θ2.
Example 3.1. Consider the principal MS-algebra L in Example 2.1 (Figure 1). The lattices Con(L) and A(L) of all congruences of L and all MS-congruence pairs of L are given in Figure 3, respectively.
Where,
△L={(x,x):x∈L},α={{0,c,a},{x,y,z},{b,d,1}},β={{0,c,a},{x,b,y,d,z,1}},γ={{0,x,b},{c,y,d},{a,z,1}},δ={{0},{c},{a},{x,b},{y,d},{z,1}},▽L=L×L. |
And
A(L)={(△L∘∘,△D(L)),(αL∘∘,αD(L)),(βL∘∘,βD(L)),(γL∘∘,γD(L)),(δL∘∘,δD(L)),(▽L∘∘,▽D(L))}={(△L∘∘,△D(L)),({{0,c,a},{b,d,1}},△D(L)),({{0,c,a},{b,d,1}},▽D(L)),({{a,1},{c,d},{0,b}},▽D(L)),(△L∘∘,▽D(L)),(▽L∘∘,▽D(L))}. |
It is clear that Con(L) isomorphic to A(L) under the isomorphism θ⟼(θL∘∘,θD(L)).
A subset I of a lattice L with 0 is called an ideal of L if
(1)0∈I,(2)a∨b∈I,∀a,b∈I,(3)I is an down-set, that is, ifx≤y,y∈Iandx∈L,thenx∈I. |
A principal ideal of L generated by a∈L is defined by
(a]={x∈L:x≤a}. |
A subset F of a lattice L with 1 is called a filter of L if
(1)1∈F,(2)a∧b∈F,∀a,b∈F,(3)F is an up-set, that is, ifx≥y,y∈Fandx∈L,thenx∈F. |
A principal filter of L generated by a∈L is defined by
[a)={x∈L:x≥a}. |
Definition 3.1. Let θ be a lattice congruence on a bounded lattice L. Then we have the following important subsets
(i) The Kernel of θ (Kerθ) is the set {x∈L:(x,0)∈θ}, which is an ideal of L,
(ii) The Cokernel of θ (Cokerθ) is the set {x∈L:(x,1)∈θ}, which is a filter of L.
Definition 3.2. [7] An element c of an MS-algebra L is called a Boolean element of L if c∨c∘=1.
It is ready seen that the set B(L)={c:c∨c∘=1} of all Boolean elements of L forms a Boolean subalgebra of L∘∘.
Lemma 3.2. Let c be a Boolean element of a principal MS-algebra L. Then
(1) θ(0,c) is a principal congruence relation on L∘∘ with Kerθ(0,c)=(c], where
(a,b)∈θ(0,c)⇔a∧c∘=b∧c∘, |
(2) θ(φL(c),1) is a principal congruence relation on D(L) with Cokerθ(φL(c),1)=[φL(c)), where
(x,y)∈θ(φL(c),1)⇔x∧(c∨dL)=y∧(c∨dL). |
Proof.
(1) It is easy to check that θ(0,c) is a principal congruence relation on L∘∘ with Kerθ(0,c)=(c].
(2) It is easy to show that θ(φL(c),1) is an equivalence relation on D(L). Let (x,y),(m,n)∈θ(φL(c),1). Thus x∧(c∨dL)=y∧(c∨dL) and m∧(c∨dL)=n∧(c∨dL). Then, we get (x∧m,y∧n)∈θ(φL(c),1) and (x∨m,y∨n)∈θ(φL(c),1). Therefore θ(φL(c),1) is a principal congruence on the lattice D(L). Also, we have
Coker(θ(φL(c),1))={x∈D(L):(x,1)∈θ(φL(c),1)}={x∈D(L):x∧(c∨dL)=1∧(c∨dL)}={x∈D(L):x∧(c∨dL)=c∨dL}={x∈D(L):x≥c∨dL}=[c∨dL)=[φL(c)). |
Now, we observe that every Boolean element c of a principal MS-algebra L associated with the MS-congruence pair of the form (θ(0,c),θ(φL(c∘),1)).
Theorem 3.3. Let L be a principal MS-algebra and c∈L∘∘. Then c is a Boolean element of L if and only if (θ(0,c),θ(φL(c∘),1)) is an MS-congruence pair of L.
Proof. Let c be a Boolean element of L. We proved that θ(0,c) and θ(φL(c∘),1) are MS-congruence on L∘∘ and lattice congruence on D(L), respectively (Lemma 3.2). To show that (θ(0,c),θ(φL(c∘),1)) is an MS-congruence pair, let (x,y)∈θ(0,c). Then
(x,y)∈θ(0,c)⇒x∧c∘=y∧c∘⇒(x∧c∘)∨dL=(y∧c∘)∨dL⇒(x∨dL)∧(c∘∨dL)=(y∨dL)∧(c∘∨dL)⇒(x∨dL,y∨dL)∈θ(φL(c∘),1). |
Conversely, let (θ(0,c),θ(φL(c∘),1)) be an MS-congruence pair. Since (0,c)∈θ(0,c) then c∧c∘ = 0∧c∘=0. Now, c∨c∘=(c∘∧c)∘=1. Therefore c is a Boolean element.
The basic properties of principal congruence relations θ(0,a) and θ(φL(a),1),∀a∈B(L) are given in the following:
Lemma 3.3. Let a,b be Boolean elements of a principal MS-algebra L. Then
(1) a≤b if and only if θ(0,a)⊆θ(0,b),
(2) a=b if and only if θ(0,a)=θ(0,b),
(3) θ(0,0)=△L∘∘ and θ(0,1)=▽L∘∘,
(4) θ(0,a)∨θ(0,b)=θ(0,a∨b),
(5) θ(0,a)∩θ(0,b)=θ(0,a∧b).
Lemma 3.4. Let L be a principal MS-algebra. Then for every a,b∈B(L), we have
(1) a≤b implies θ(φL(a∘),1)⊆θ(φL(b∘),1),
(2) θ(φL(a∘),1)∨θ(φL(b∘),1)=θ(φL(a∨b)∘,1),
(3) θ(φL(a∘),1)∧θ(φL(b∘),1)=θ(φL(a∧b)∘,1),
(4) θ(φL(0),1)=▽D(L) and θ(φL(1),1)=△D(L).
Proof.
(1) Let a≤b. Then a∘≥b∘. Let (x,y)∈θ(φL(a∘),1) then x∧(a∘∨dL)=y∧(a∘∨dL). Now
x∧(b∘∨dL)=x∧((a∘∧b∘)∨dL)=x∧{(a∘∨dL)∧(b∘∨dL)}=x∧(a∘∨dL)∧(b∘∨dL)=y∧(a∘∨dL)∧(b∘∨dL)=y∧{(a∘∧b∘)∨dL}=y∧(b∘∨dL). |
Then (x,y)∈θ(φL(b∘),1). Therefore θ(φL(a∘),1)⊆θ(φL(b∘),1).
(2) Since a,b≤a∨b then by (1), we have
θ(φL(a∘),1),θ(φL(b∘),1)⊆θ(φL(a∨b)∘,1). |
Then θ(φL(a∨b)∘,1) is an upper bound of θ(φL(a∘),1) and θ(φL(b∘),1). Let θ(φL(c∘),1) be an upper bound of θ(φL(a∘),1) and θ(φL(b∘),1).
Then θ(φL(a∘),1),θ(φL(b∘),1)⊆θ(φL(c∘),1) implies φL(c∘)≤φL(a∘), φL(b∘). Thus φL(c∘)≤φL(a∘∧b∘)=φL(a∨b)∘ and hence θ(φL(a∨b)∘,1)⊆θ(φL(c∘),1). Therefore θ(φL(a∨b)∘,1) is the least upper bound of θ(φL(a∘),1) and θ(φL(b∘),1).
(3) Since a∧b≤a,b then by (1) θ(φL(a∧b)∘,1)⊆θ(φL(a∘),1),θ(φL(b∘),1). Then θ(φL(a∧b)∘,1) is a lower bound of θ(φL(a∘),1) and θ(φL(b∘),1). Let θ(φL(c∘),1) be a lower bound of θ(φL(a∘),1) and θ(φL(b∘),1). Then θ(φL(c∘),1)⊆θ(φL(a∘),1),θ(φL(b∘),1) implies φL(a∘),φL(b∘)≤φL(c∘). Thus φL(a∧b)∘=φL(a∘)∨φL(b∘)≤φL(c∘) and hence θ(φL(c∘),1)⊆θ(φ(a∧b)∘,1). Therefore θ(φL(a∧b)∘,1) is the greatest lower bound of θ(φL(a∘),1) and θ(φL(b∘),1).
(4) Since dL,1∈θ(dL,1), then θ(dL,1)=D(L)×D(L)=▽D(L). Also, let (x,y)∈θ(φL(1),1) then x∧(1∨dL)=y∧(1∨dL). It follows, x=y and hence θ(φL(1),1)=△D(L).
Theorem 3.4. Let L be a principal MS-algebra. Consider the subsets H and G of Con(L∘∘) and Con(D(L)), respectively as follows:
H={θ(0,a):a∈B(L)},G={θ(φL(a),1):a∈B(L)}. |
Then we have
(1) (H;∨,∧,′,△L∘∘,▽L∘∘) is a Boolean algebra, where (θ(0,a))′=θ(a∘,0),
(2) (G;∨,∧,′,△D(L),▽DL) is a Boolean algebra, where (θ(φL(a),1))′=θ(φL(a∘),1).
Proof.
(1) Since θ(0,0)=△L∘∘ and θ(0,1)=▽L∘∘. Then ▽L∘∘,△L∘∘∈H. Let θ(0,a),θ(0,b)∈H. Then we get
θ(0,a)∨θ(0,b)=θ(0,a∨b), |
and
θ(0,a)∧θ(0,b)=θ(0,a∧b). |
Therefore H is a bounded lattice. Since B(L) is a Boolean algebra, then a∘∈B(L) for all a∈B(L). Thus θ(0,a∘)∈H. Then we have
θ(0,a)∨[θ(0,a)]′=θ(0,a)∨θ(0,a∘)=θ(0,a∨a∘)=θ(0,1)=▽L∘∘, |
and
θ(0,a)∧[(0,a)]′=θ(0,a)∧θ(0,a∘)=θ(φL(0,a∧a∘)=θ(0,0)=△L∘∘. |
Therefore (H;∨,∧,′,△L∘∘,▽L∘∘) is a Boolean algebra.
(2) We have △D(L)=θ(φL(1),1)∈G and ▽D(L)=θ(φL(0),1)=▽D(L)∈G. Let θ(φL(a),1),θ(φL(b),1)∈G. Then we get
θ(φL(a),1)∨θ(φL(b),1)=θ(φL(a∧b),1), |
and
θ(φL(a),1)∧θ(φL(b),1)=θ(φL(a∨b),1). |
Therefore G is a bounded lattice. Since B(L) is a Boolean algebra, then a∘∈B(L) for all a∈B(L). Thus θ(φL(a∘),1)∈G. Then we have
θ(φL(a),1)∨[θ(φL(a),1)]′=θ(φL(a),1)∨θ(φL(a∘),1)=θ(φL(a∧a∘),1)=θ(φL(0),1)=▽D(L), |
and
θ(φL(a),1)∧[θ(φL(a),1)]′=θ(φL(a),1)∧θ(φL(a∘),1)=θ(φL(a∨a∘),1)=θ(φL(1),1)=△D(L). |
Therefore (G;∨,∧,′,△DL,▽DL) is a Boolean algebra.
Let L be a principal MS-algebra. Let A(L) be the lattice of all MS-congruence pairs of L. We consider a subset A′(L) of A(L) as follows:
A′(L)={(θ(0,a),θ(φL(a∘),1)):a∈B(L)}. |
From the above results, we observe that the set A′(L) of all MS-congruence pairs induced by the Boolean elements of a principal MS-algebra forms bounded sublattice of the lattice A(L). Moreover A′(L) is a Boolean algebra on its own.
Theorem 3.5. Let L be a principal MS-algebra. Then (A′(L);∨,∧,′,0A′(L),1A′(L)) is a Boolean algebra, where
(θ(0,a),θ(φL(a∘),1))∨(θ(0,b),θ(φL(b∘),1))=(θ(0,a∨b),θ(φL(a∨b)∘,1)),(θ(0,a),θ(φL(a∘),1)∧(θ(0,b),θ(φL(b∘),1))=(θ(0,a∧b),θ(φL(a∧b)∘,1)),[(θ(0,a),θ(φL(a∘),1))]′=(θ(0,a∘),θ(φL(a),1)),1A′(L)=(▽L∘∘,△D(L)),0A′(L)=(△L∘∘,△D(L)). |
Example 3.2. Consider the principal MS-algebra L in Example 2.1. The lattices B(L) and A′(L) of all Boolean elements of L and all MS-congruence pairs of L of the form (θ(0,a),θ(φL(a∘),1)),a∈B(L) are given in the following Figure 4, respectively. It is clear that B(L) and A(L) are isomorphic Boolean algebras under the isomorphism a⟼(θ(0,a),θ(a∘∨z,1)). Also, A′(L) is a bounded sublattice of A(L).
In this section, we characterize the notion of 2-permutability of congruences of a principal MS-algebra by means of MS-congruence pairs.
Let L be an algebra. We say the congruences θ,Φ∈Con(L) are 2-permutable if θ∘Φ=Φ∘θ, that is, (x,a)∈θ and (a,y)∈Φ, x,y,a∈L imply (x,b)∈Φ and (b,y)∈θ for some b∈L. We call the algebra L has 2-permutable congruences (briefly permutable) if every pair of congruences of L permute.
Lemma 4.1. A principal MS-algebra L has 2-permutable congruences if and only if every pair of principle congruences of L permute.
Proof. Assume that every pair of principal congruences on L permute. Let θ and Φ be arbitrary congruences on L and (a,b)∈(θ∘Φ),a,b∈L. Then (a,t)∈θ and (t,b)∈Φ for some t∈L. Then (a,t)∈θ(a,t) and (t,b)∈θ(t,b) imply that (a,b)∈θ(a,t)∘θ(t,b). Thus (a,b)∈θ(t,b)∘θ(a,t). Since θ(a,t)⊆θ and θ(t,b)⊆Φ, then (a,b)∈(Φ∘θ). Therefore θ and Φ permute. The second implication is obvious.
Define a relation Ψ on a principal MS-algebra L as follows:
(a,b)∈Ψ⇔a∘=b∘⇔a∘∘=b∘∘. |
Theorem 4.1. Let L be a principal MS-algebra with the smallest dense element dL. Then
(1) Ψ is a congruence relation on L with KerΨ={0} and CokerΨ=D(L),
(2) Ψ is closed congruence on L, that is, (x,x∘∘)∈Ψ,∀x∈L,
(3) max [x]Ψ=x∘∘,x∈L, where [x]Ψ={y∈L:y∘∘=x∘∘} is the congruence class of x modulo Ψ.
Proof.
(1) One can check that Ψ is a congruence relation on L. Now, we have
KerΨ={a∈L:(a,0)∈Ψ}={a∈L:a∘=1}={0}, |
and
CokerΨ={z∈L:(z,1)∈Ψ}={z∈L:z∘=1}=D(L)=[dL). |
(2) Since x∘∘∘∘=x∘∘ then x∘∘∈[x]Ψ. Thus (x∘∘,x)∈Ψ.
(3) Let y∈[x]Ψ. Then y≤y∘∘=x∘∘ and x∘∘∈[x]Ψ. Thus x∘∘ is the greatest member of the congruence class [x]Ψ.
Theorem 4.2. Let L be a principal MS-algebra. Then
(1) L/Ψ is a de Morgan algebra,
(2) L/Ψ and L∘∘ are isomorphic de Morgan algebras.
Proof.
(1) It is ready seen that (L/Ψ;∨,∧,{0},D(L)) is a bounded distributive lattice, where L/Ψ={[a]Ψ:a∈L} is the set of all congruence classes module Ψ and
[a]Ψ∨[b]Ψ=[a∨b]Ψ,[a]Ψ∧[b]Ψ=[a∧b]Ψ. |
Define ◻ on L/Ψ by ([a]Ψ)◻=[a∘]Ψ,∀a∈L. We have
([0]Ψ)◻=[1]Ψ,([a]Ψ)◻◻=[a∘∘]Ψ=[a]Ψ,([a]Ψ∧[b]Ψ)◻=([a]Ψ)◻∨([b]Ψ)◻. |
Then L/Ψ is a de Morgan algebra.
(2) Define g:L∘∘→L/Ψ by
g(a)=[a]Ψ,∀a∈L∘∘. |
Let a,b∈L∘∘ and a=b. Then a∘∘=b∘∘ implies [a]Ψ=[b]Ψ. It follows that g is well defined map of L∘∘ into L/Ψ. Let a,b∈L∘∘, we get
g(a∨b)=[a∨b]Ψ=[a]Ψ∨[b]Ψ=g(a)∨g(b), |
g(a∧b)=[a∧b]Ψ=[a]Ψ∧[b]Ψ=g(a)∧g(b), |
and
g(a∘)=[a∘]Ψ=([a]Ψ)◻=(g(a))◻. |
Let [a]Ψ=[b]Ψ. Then a∘∘=b∘∘ implies a=b. Therefore g is an injective map. Let [a]Ψ∈L/Ψ. Then [x]Ψ=[x∘∘]Ψ=g(x). Then g is a surjective map. This deduce that g is an isomorphism of de Morgan algebras.
Now, we observe that Ψ satisfies the following property,
Ψ∘θ=θ∘Ψ forall θ∈Con(L). |
Theorem 4.3. Let L be a principal MS-algebra. Then Ψ permutes with each congruence of L.
Proof. We prove that Ψ∘θ=θ∘Ψ for all θ∈Con(L). Let (a,b)∈Ψ∘θ. Then (a,z)∈Ψ and (z,b)∈θ for some z∈L. It follows that a∘∘=z∘∘ and (z,b)∈θ. Now
(z,b)∈θ⇒(z∘∘,b∘∘)∈θand(a∨dL,a∨dL)∈θ⇒(a∘∘∧(a∨dL),b∘∘∧(a∨dL))∈θ⇒(a,b∘∘∧(a∨dL))∈θ as a=a∘∘∧(a∨dL) |
Since [b∘∘∧(a∨dL)]∘∘=b∘∘, then (b∘∘∧(a∨dL),b)∈Ψ. Therefore (a,b∘∘∧(a∨dL))∈θ and (b∘∘∧(a∨dL),b)∈Ψ. imply (a,b)∈θ∘Ψ. Then Ψ∘θ=θ∘Ψ,∀θ∈Con(L).
Lemma 4.2. Let L be a principal MS-algebra. Then
(1) △L permutes with every congruence on L,
(2) ▽L permutes with every congruence on L.
Proof.
(1) Let (a,b)∈θ∘△L. Then (a,t)∈θ and (t,b)∈△L for some t∈L. Thus (a,t)∈θ and t=b. Then (a,a)∈△L and (a,b)∈θ imply (a,b)∈△L∘θ. Therefore θ∘△L=△L∘θ.
(2) It is obvious.
In the following theorem, we characterize 2-permutability of congruences of a principal MS-algebra L using MS-congruence pairs.
Theorem 4.4. Let L be a principal MS-algebra. Then the following conditions are equivalent:
(1) L has 2-permutable congruences.
(2) L∘∘ and D(L) have 2-permutable congruences.
Proof. To prove the conditions (1) and (2) are equivalent, we show that the two congruences θ,Φ∈Con(L) are 2-permutable if and only if their restrictions θL∘∘,ΦL∘∘ and θD(L),ΦD(L) have 2-permutable congruences on L∘∘ and D(L), respectively. Firstly, suppose that θ,Φ have 2-permutable congruences on L. Let (x,z)∈θL∘∘∘ΦL∘∘. Then there exist y∈L∘∘ such that (x,y)∈θL∘∘ and (y,z)∈ΦL∘∘. Then we have (x,y)∈θ and (y,z)∈Φ. Since θ,Φ are 2-permutable, then there exists a∈L such that (x,a)∈Φ and (a,y)∈θ. We get (x,a∘∘)=(x∘∘,a∘∘)∈ΦL∘∘ and (a∘∘,z∘∘)∈θL∘∘. Therefore θL∘∘,ΦL∘∘ are 2-permutable. Also, to prove that θD(L),ΦD(L) are 2-permutable, let (x,z)∈θD(L)∘ΦD(L). Then there exist y∈D(L) such that (x,y)∈θD(L) and (y,z)∈ΦD(L). Then we have (x,y)∈θ and (y,z)∈Φ. Since θ,Φ are 2-permutable, there exists a∈L such that
(x,a)∈Φand(a,z)∈θ⇒(x,a∨dL)=(x∨dL,a∨dL)∈Φand(a∨dL,z∨dL)∈θ⇒(x,a∨dL)∈Φand(a∨dL,z)∈θ,⇒(x,a∨dL)∈ΦD(L)and(a∨dL,z)∈θD(L),asx,a∨dL,z∈D(L)⇒(x,z)∈ΦD(L)∘θD(L). |
Thus θD(L),ΦD(L) have 2-permutable congruences on D(L).
Conversely, let θ,Φ∈Con(L). Assume that θL∘∘,ΦL∘∘ and θD(L),ΦD(L), have 2-permutable congruences on L∘∘ and D(L), respectively. Let (x,z)∈θ∘Φ. Suppose that x,y,z∈L with (x,y)∈θ and (y,z)∈Φ. Then, by using Theorem 2.3, we get the following statements.
(x∘∘,y∘∘),(y∘∘,z∘∘)∈ΦL∘∘,(x∨dL,y∨dL),(y∨dL,z∨dL)ΦD(L). |
Since θL∘∘,ΦL∘∘ have 2-permutable congruences on L∘∘, there exists an element a∈L∘∘ such that
(x∘∘,a)∈ΦL∘∘ and (a,z∘∘)∈θL∘∘. |
Since θD(L),ΦD(L) have 2-permutable congruences on D(L), there exists e∈D(L) such that (x∨dL,e)∈ΦDL and (e,z∨dL)∈θDL). It follows that (x∘∘,a)∈Φ,(a,z∘∘)∈θ and (x∨dL,e)∈Φ,(e,z∨dL)∈θ. Since L is a principal MS-algebra we have
x=x∘∘∧(x∨dL) and z=z∘∘∧(z∨dL). |
Since θ,Φ are compatible with the ∧ operation, then (x∘∘,a)∈Φ and (x∨dL,e)∈Φ imply (x,a∧e)=(x∘∘∧(x∨dL),a∧e)∈Φ. Also (a,z∘∘)∈θ and (e,z∨dL)∈θ imply (a∧e,z)=(a∧e,z∘∘∧(z∨dL))∈θ. Consequently, we deduce that (x,a∧e)∈Φ and (a∧e,z)∈θ,a∧e∈L. Then (x,z)∈Φ∘θ, and hence θ,Φ are 2-permutable.
Now, we construct two examples to clarify the above theorem.
Example 4.1. Consider the principal MS-algebra L in Example 2.1 (Figure 1). From Table 1, we show that L has 2-permutable congruences. Also, Tables 2 and 3 show that L∘∘andD(L) have 2-permutable congruences, respectively. Where αL∘∘=βL∘∘ and δL∘∘=△L∘∘.
∘ | △L | α | β | γ | δ | ▽L |
△L | △L | α | β | γ | δ | ▽L |
α | α | α | β | ▽L | β | ▽L |
β | β | β | β | ▽L | β | ▽L |
γ | γ | ▽L | ▽L | γ | ▽L | ▽L |
δ | δ | β | β | ▽L | δ | ▽L |
▽L | ▽L | ▽L | ▽L | ▽L | ▽L | ▽L |
∘ | △L∘∘ | αL∘∘ | γL∘∘ | ▽L∘∘ |
△L∘∘ | △L∘∘ | αL∘∘ | γL∘∘ | ▽L∘∘ |
αL∘∘ | αL∘∘ | αL∘∘ | ▽L∘∘ | ▽L∘∘ |
γL∘∘ | γL∘∘ | ▽L∘∘ | γL∘∘ | ▽L∘∘ |
▽L∘∘ | ▽L∘∘ | ▽L∘∘ | ▽L∘∘ | ▽L∘∘ |
∘ | △D(L) | ▽D(L) |
△D(L) | △D(L) | ▽D(L) |
▽D(L) | ▽D(L) | ▽D(L) |
In the following example, we give a principal MS-algebra L which has not 2-permutable congruences as well as D(L) has not also 2-permutable congruences.
Example 4.2. Consider the principal MS-algebra L in Figure 5.
It is clear that D(L)={z,z1,1} and L∘∘={0,c,a,b,d,1}. Consider θ,Φ∈Con(L) as follows:
θ=△L∪{{x1,b},{y1,d},{z1,1}},Φ=△L∪{{x,x1},{y,y1},{z,z1}}. |
We observe that θ∘Φ≠Φ∘θ as (z,1)∈Φ∘θ but (z,1)∉θ∘Φ.
Then L has not 2-permutable congruences and D(L) has not 2-permutable congruences as θD(L)∘ΦD(L)≠ΦD(L)∘θD(L).
We generalize the concept of 2-permutability to the concept of n-permutability of congruences of principal MS-algebras.
Definition 5.1. A principal MS-algebra L is said to have n-permutable congrunces if every two congruences θ,Φ of L are n-permutable, that is,
θ∘Φ∘θ∘...=Φ∘θ∘Φ∘...(n times). |
At first, we need the following two lemmas.
Lemma 5.1. Let L be a principal MS- algebra with the smallest dense element dL. Let θ,Φ be two congruences on L. Then we have
(i) (θ∘Φ∘θ∘...)L∘∘=θL∘∘∘ΦL∘∘∘...(n times),
(ii) (θ∘Φ∘θ∘...)D(L)=θD(L)∘ΦD(L)∘...(n times).
Proof.
(i) Recall that θL∘∘,ΦL∘∘ are the restrictions of θ,Φ on L∘∘, respectively. Let a,b∈L∘∘ and (a,b)∈(θL∘∘∘ΦL∘∘∘...). Then there exist c1,c2,...,cn−1∈L∘∘ such that
(a,c1)∈θL∘∘,(c1,c2)∈ΦL∘∘,...,(cn−1,b)∈Ψ,whereΨ={θL∘∘,ifnodd,ΦL∘∘,ifneven. |
Then
(a,c1)∈θ,(c1,c2)∈Φ,...,(cn−1,b)∈Ψ,whereΨ={θ,ifnodd,Φ,ifneven. |
Then (a,b)∈(θ∘Φ∘θ∘...) and hence (a,b)∈(θ∘Φ∘θ∘...)L∘∘. Therefore
θL∘∘∘ΦL∘∘∘θL∘∘...⊆(θ∘Φ∘θ∘...)L∘∘. |
Conversely, let a,b∈L∘∘ such that (a,b)∈(θ∘Φ∘θ∘...)L∘∘. Then (a,b)∈(θ∘Φ∘θ∘...). Then there exist c1,c2,...,cn−1∈L such that
(a,c1)∈θL∘∘,(c1,c2)∈ΦL∘∘,...,(cn−1,b)∈Ψ,whereΨ={θL∘∘,ifnodd,ΦL∘∘,ifneven. |
Then we get
(a,c1∘∘)∈θ,(c1∘∘,c2∘∘)∈Φ,...,(cn−1∘∘,b)∈Ψ,whereΨ={θ,ifnodd,Φ,ifneven. |
Since c1∘∘,c2∘∘,...,cn−1∘∘∈L∘∘, we have
(a,c1∘∘)∈θL∘∘,(c1∘∘,c2∘∘)∈ΦL∘∘,...,(cn−1∘∘,b)∈Ψ,whereΨ={θL∘∘,ifnodd,ΦL∘∘,ifneven. |
Therefore
(a,b)∈(θL∘∘∘ΦL∘∘∘θL∘∘∘...) |
and hence
(θ∘Φ∘θ∘...)L∘∘⊆θL∘∘∘ΦL∘∘∘θL∘∘∘...(ntimes). |
Then we get
(θ∘Φ∘θ∘...)L∘∘=θL∘∘∘ΦL∘∘∘θL∘∘∘...(ntimes). |
(ii) Take x,y∈D(L), let (x,y)∈(θD(L)∘ΦD(L)∘ΦD(L)∘θD(L)∘...). Then (x,y)∈(θ∘Φ∘θ∘...) and hence (a,b)∈(θ∘Φ∘θ∘...)D(L). Then (θ∘Φ∘θ∘...)⊆(θ∘Φ∘θ∘...)D(L).
Conversely, let (x,y)∈(θ∘Φ∘θ∘...)D(L), then (x,y)∈(θ∘Φ∘...). There exist d1,d2,...dn−1∈L, such that
(x,d1)∈θ,(d1,d2)∈Φ,...,(dn−1,y)∈ψ,whereψ={θ,ifnodd,Φ,ifneven. |
Since x,y≥dL, we get,
(x,d1)=(x∨dL,d1∨dL)∈θD(L),(d1∨dL,d2∨dL)∈ΦD(L),...,(dn−1,b∨dL1)=(dn−1,y)∈ψ,whereψ={θD(L),ifnodd,ΦD(L),ifneven. |
Hence (x,y)∈(θD(L)∘ΦD(L)∘θD(L)∘...). Then the required equality is proved.
Lemma 5.2. Let θ and Φ be congruences on a principal MS-algebra L. Let ψ denoted to the relation θ∘Φ∘θ∘...(n times). Then (a,b)∈ψ and (c,d)∈ψ imply (a∧c,b∧d)∈ψ.
Proof. Let (a,b)∈ψ and (c,d)∈ψ. Then there exist a1,a2,...,an−1,c1,c2,...,cn−1∈L such that
(a,a1)∈θ,(a1,a2)∈Φ,...,(an−1,b)∈Ψ,whereΨ={θ,ifnodd,Φ,ifneven. |
And
(c,c1)∈θ,(c1,c2)∈Φ,...,(cn−1,d)∈Ψ,whereΨ={θ,ifnodd,Φ,ifneven. |
Since θ,Φ are congruence on L then we get,
(a∧c,a1∧c1)∈θ,(a1∧c1,a2∧c2)∈Φ,...,(an−1∧cn−1,b∧d)∈Ψ,whereΨ={θ,ifnodd,Φ,ifneven. |
Thus (a∧c,b∧d)∈ψ.
Now, a characterization of n-permutability of principal MS-algebras is given.
Theorem 5.1. Two congruences θ,Φ on a principal MS-algebra L are n-permutable if and only if their restrictions θL∘∘,ΦL∘∘ and θD(L),ΦD(L) with respect to L∘∘ and D(L) respectively are n-permutable.
Proof. Let L has n-permutable congruences. Let θ,Φ be any two congruences on L. Then by (i) and (ii) of Lemma 5.1, respectively, we have
θL∘∘∘ΦL∘∘∘θL∘∘∘...=(θ∘Φ∘θ∘...)L∘∘=(Φ∘θ∘Φ∘...)L∘∘=ΦL∘∘∘θL∘∘∘ΦL∘∘∘..., |
and
θD(L)∘ΦD(L)∘θD(L)∘...=(θ∘Φ∘θ∘...)D(L)=(Φ∘θ∘Φ∘...)D(L)=ΦD(L)∘θD(L)∘ΦD(L)∘.... |
Therefore L∘∘ and D(L) have n-permutable congruences.
Conversely, let L∘∘ and D(L) have n-permutable congruences. If (a,b)∈(θ∘Φ∘θ∘...), using Theorem 2.3, we get
(a∘∘,b∘∘)∈(θ∘Φ∘θ∘...)L∘∘, |
and
(a∨dL,b∨dL)∈(θ∘Φ∘θ∘...)DL. |
By lemma 5.1, we get
(a∘∘,b∘∘)∈(θL∘∘∘ΦL∘∘∘θL∘∘∘...), |
and
(a∨dL,b∨dL)∈(θD(L)∘ΦD(L)∘ΦD(L)∘...). |
Since θL∘∘,ΦL∘∘ and θD(L),ΦD(L) are n-permutable on L∘∘,D(L), respectively, we have
(a∘∘,b∘∘)∈(ΦL∘∘∘θL∘∘∘ΦL∘∘∘...), |
and
(a∨dL≡b∨dL)∈(ΦD(L)∘θD(L)∘ΦD(L)∘...). |
It follows that, (a∘∘,b∘∘)∈(Φ∘θ∘Φ∘...) and (a∨dL,b∨dL)∈(Φ∘θ∘Φ∘...). Since L is a principal MS-algebra, then a=a∘∘∧(a∨dL) and b=b∘∘∧(b∨dL). By Lemma 5.2, we get
(a∘∘∧(a∨dL),b)=(a,b)∈(Φ∘θ∘Φ∘...). |
Thus (θ∘Φ∘θ∘...)⊆(Φ∘θ∘Φ∘...). Similarly we can show that (Φ∘θ∘Φ∘...)⊆(θ∘Φ∘θ∘...). Then L has n-permutable congruences.
With the aid of the technique of MS-congruence pairs, many properties were investigated for principal MS-algebras, deal with a characterization of congruence pairs of a principal MS-algebra (a Stone algebra), a description of the lattice A(L) of all MS-congruence pairs of L via Boolean elements of L, characterizations of 2-permutability and n-permutability of congruences of a principal MS-algebra. This work leads us in the future to study many aspects of principal MS-algebras and related structures, for instance, it can be applied to triple construction of principal MS-algebra, affine and locally complete of principal MS-algebras.
The authors declare they have not used Artificial Intelligence (AI) tools in the creation of this article.
The authors declare no conflict of interest.
[1] |
X. D. Li, J. H. Shen, H. Akca, R. Rakkiyappan, LMI-based stability for singularly perturbed nonlinear impulsive differential systems with delays of small parameter, Appl. Math. Comput., 250 (2015), 798–804. https://doi.org/10.1016/j.amc.2014.10.113 doi: 10.1016/j.amc.2014.10.113
![]() |
[2] |
X. D. Li, P. Li, Stability of time-delay systems with impulsive control involving stabilizing delays, Automatica, 124 (2021), 109336. https://doi.org/10.1016/j.automatica.2020.109336 doi: 10.1016/j.automatica.2020.109336
![]() |
[3] |
D. Yang, X. D. Li, J. L. Qiu, Output tracking control of delayed switched systems via state-dependent switching and dynamic output feedback, Nonlinear Anal-Hybri., 32 (2019), 294–305. https://doi.org/10.1016/j.nahs.2019.01.006 doi: 10.1016/j.nahs.2019.01.006
![]() |
[4] |
Y. Chen, J. X. Wu, J. Lan, Study on reasonable initialization enhanced Karnik-Mendel algorithms for centroid type-reduction of interval type-2 fuzzy logic systems, AIMS Mathematics, 5 (2020), 6149–6168. https://doi.org/10.3934/math.2020395 doi: 10.3934/math.2020395
![]() |
[5] |
Y. Chen, J. X. Wu, J. Lan, Study on weighted-based noniterative algorithms for centroid type-reduction of interval type-2 fuzzy logic systems, AIMS Mathematics, 5 (2020), 7719–7745. https://doi.org/10.3934/math.2020494 doi: 10.3934/math.2020494
![]() |
[6] |
H. K. Lam, L. D. Seneviratne, Stability analysis of interval type-2 fuzzy-model-based control systems, IEEE T. Syst. Man Cy. B, 38 (2008), 617–628. https://doi.org/10.1109/TSMCB.2008.915530 doi: 10.1109/TSMCB.2008.915530
![]() |
[7] |
T. Zhao, S. Y. Dian, State feedback control for interval type-2 fuzzy systems with time-varying delay and unreliable communication links, IEEE T. Fuzzy Syst., 26 (2018), 951–966. https://doi.org/10.1109/TFUZZ.2017.2699947 doi: 10.1109/TFUZZ.2017.2699947
![]() |
[8] |
L. Sheng, X. Y. Ma, Stability analysis and controller design of interval type-2 fuzzy systems with time delay, Int. J. Syst. Sci., 45 (2014), 977–993. https://doi.org/10.1080/00207721.2012.743056 doi: 10.1080/00207721.2012.743056
![]() |
[9] |
R. Kavikumar, R. Sakthivel, O. M. Kwon, B. Kaviarasan, Faulty actuator-based control synthesis for interval type-2 fuzzy systems via memory state feedback approach, Int. J. Syst. Sci., 51 (2020), 2958–2981. https://doi.org/10.1080/00207721.2020.1804643 doi: 10.1080/00207721.2020.1804643
![]() |
[10] |
H. K. Lam, H. Y. Li, C. Deters, E. L. Secco, H-A. Wurdemann, K. Althoefer, Control design for interval type-2 fuzzy systems under imperfect premise matching, IEEE T. Ind. Electron., 61 (2014), 956–968. https://doi.org/10.1109/TIE.2013.2253064 doi: 10.1109/TIE.2013.2253064
![]() |
[11] |
H. Y. Li, X. J. Sun, L. G. Wu, H. K. Lam, State and output feedback control of interval type-2 fuzzy systems with mismatched membership functions, IEEE T. Fuzzy Syst., 23 (2015), 1943–1957. https://doi.org/10.1109/TFUZZ.2014.2387876 doi: 10.1109/TFUZZ.2014.2387876
![]() |
[12] |
W. Zheng, Z. M. Zhang, H. B. Wang, H. R. Wang, Robust H∞ dynamic output feedback control for interval type-2 T-S fuzzy multiple time-varying delays systems with external disturbance, J. Franklin I., 357 (2020), 3193–3218. https://doi.org/10.1016/j.jfranklin.2019.03.039 doi: 10.1016/j.jfranklin.2019.03.039
![]() |
[13] | W. T. Song, S. C. Tong, Observer-based fuzzy event-triggered control for interval type-2 fuzzy systems, Int. J. Fuzzy Syst., (2021). https://doi.org/10.1007/s40815-021-01114-w |
[14] |
O. Uncu, I. B. Turksen, Discrete interval type 2 fuzzy system models using uncertainty in learning parameters, IEEE T. Fuzzy Syst., 15 (2007), 90–106. https://doi.org/10.1109/TFUZZ.2006.889765 doi: 10.1109/TFUZZ.2006.889765
![]() |
[15] |
Y. B. Gao, H. Y. Li, L. G. Wu, H. R. Karimi, H. K. Lam, Optimal control of discrete-time interval type-2 fuzzy-model-based systems with D-stability constraint and control saturation, Signal Process., 120 (2016), 409–421. https://doi.org/10.1016/j.sigpro.2015.09.007 doi: 10.1016/j.sigpro.2015.09.007
![]() |
[16] |
Q. Zhou, D. Liu, Y. B. Cao, H. K. Lam, R. Sakthivel, Interval type-2 fuzzy control for nonlinear discrete-time systems with time-varying delays, Neurocomputing, 157 (2015), 22–32. https://doi.org/10.1016/j.neucom.2015.01.042 doi: 10.1016/j.neucom.2015.01.042
![]() |
[17] |
T. Zhao, S. Y. Dian, Delay-dependent stabilization of discrete-time interval type-2 T- S fuzzy systems with time-varying delay, J. Franklin I., 354 (2017), 1542–1567. https://doi.org/10.1016/j.jfranklin.2016.12.002 doi: 10.1016/j.jfranklin.2016.12.002
![]() |
[18] | Y. Zeng, H. K. Lam, B. Xiao, L. G. Wu, L2−L∞ control of discrete-time state-delay interval type-2 fuzzy systems via dynamic output feedback, IEEE T. Cybernetics, (2020), 1–11. http://dx.doi.org/10.1109/TCYB.2020.3024754 |
[19] |
W. T. Song, S. C. Tong, Fuzzy decentralized output feedback event-triggered control for interval type-2 fuzzy systems with saturated inputs, Inform. Sciences, 575 (2021), 639–653. https://doi.org/10.1016/j.ins.2021.07.070 doi: 10.1016/j.ins.2021.07.070
![]() |
[20] |
F. Amato, M. Ariola, P. Dorato, Finite-time control of linear systems subject to parametric uncertainties and disturbances, Automatica, 37 (2001), 1459–1463. https://doi.org/10.1016/S0005-1098(01)00087-5 doi: 10.1016/S0005-1098(01)00087-5
![]() |
[21] |
Y. Li, L. Liu, G. Feng, Finite-time stabilization of a class of T-S fuzzy systems, IEEE T. Fuzzy Syst., 25 (2017), 1824–1829. https://doi.org/10.1109/TFUZZ.2016.2612301 doi: 10.1109/TFUZZ.2016.2612301
![]() |
[22] |
L. Han, C. Y. Qiu, J. Xiao, Finite-time H∞ control Synthesis for nonlinear switched systems using T-S fuzzy model, Neurocomputing, 171 (2016), 156–170. https://doi.org/10.1016/j.neucom.2015.06.028 doi: 10.1016/j.neucom.2015.06.028
![]() |
[23] |
M. Chen, J. Sun, H∞ finite time control for discrete time-varying system with interval time-varying delay, J. Franklin I., 355 (2018), 5037–5057. https://doi.org/10.1016/j.jfranklin.2018.05.031 doi: 10.1016/j.jfranklin.2018.05.031
![]() |
[24] |
Y. Q. Zhang, Y. Shi, P. Shi, Robust and non-fragile finite-time H∞ control for uncertain Markovian jump nonlinear systems, Appl. Math. Comput., 279 (2016), 125–138. https://doi.org/10.1016/j.amc.2016.01.012 doi: 10.1016/j.amc.2016.01.012
![]() |
[25] |
Y. Q. Zhang, Y. Shi, P. Shi, Resilient and robust finite-time H∞ control for uncertain discrete-time jump nonlinear systems, Appl. Math. Model., 49 (2017), 612–629. https://doi.org/10.1016/j.apm.2017.02.046 doi: 10.1016/j.apm.2017.02.046
![]() |
[26] |
Y. Q. Zhang, C. X. Liu, X. W. Mu, Robust finite-time H∞ control of singular stochastic systems via static output feedback, Appl. Math. Comput., 218 (2012), 5629–5640. https://doi.org/10.1016/j.amc.2011.11.057 doi: 10.1016/j.amc.2011.11.057
![]() |
[27] |
Y. Q. Zhang, P. Shi, S. K. Nguang, Observer-based finite-time H∞ control for discrete singular stochastic systems, Appl. Math. Lett., 38 (2014), 115–121. https://doi.org/10.1016/j.aml.2014.07.010 doi: 10.1016/j.aml.2014.07.010
![]() |
[28] |
T. Zhao, J. H. Liu, S. Y. Dian, Finite-time control for interval type-2 fuzzy time-delay systems with norm-bounded uncertainties and limited communication capacity, Inform. Sciences, 483 (2019), 153–173. https://doi.org/10.1016/j.ins.2019.01.044 doi: 10.1016/j.ins.2019.01.044
![]() |
[29] |
R. Kavikumar, R. Sakthivel, O. M. Kwon, B. Kaviarasan, Finite-time boundedness of interval type-2 fuzzy systems with time delay and actuator faults, J. Franklin I., 356 (2019), 8296–8324. https://doi.org/10.1016/j.jfranklin.2019.07.031 doi: 10.1016/j.jfranklin.2019.07.031
![]() |
[30] |
N. N. Rong, Z. S. Wang, H. G. Zhang, Finite-time stabilization for discontinuous interconnected delayed systems via interval type-2 T-S fuzzy model approach, IEEE T. Fuzzy Syst., 27 (2019), 249–261. https://doi.org/10.1109/TFUZZ.2018.2856181 doi: 10.1109/TFUZZ.2018.2856181
![]() |
[31] |
Z. S. Wang, N. N. Rong, H. G. Zhang, Finite-time decentralized control of IT2 T-S fuzzy interconnected systems with discontinuous interconnections, IEEE T. Cybernetics, 49 (2019), 3547–3556. https://doi.org/10.1109/TCYB.2018.2848626 doi: 10.1109/TCYB.2018.2848626
![]() |
[32] |
Y. Y. Cao, Z. L. Lin, Robust stability analysis and fuzzy-scheduling control for nonlinear systems subject to actuator saturation, IEEE T. Fuzzy Syst., 11 (2003), 57–67. https://doi.org/10.1109/TFUZZ.2002.806317 doi: 10.1109/TFUZZ.2002.806317
![]() |
[33] |
D. Saifia, C. Mohammed, S. Labiod, T. M. Guerra, Robust H∞ static output feedback stabilization of T-S fuzzy systems subject to actuator saturation, Int. J. Control Autom., 10 (2012), 613–622. https://doi.org/10.1007/s12555-012-0319-3 doi: 10.1007/s12555-012-0319-3
![]() |
[34] |
F. W. Yang, Z. D. Wang, Y. S. Hung, M. Gani, Robust H∞ control for networked systems with random communication delays, IEEE T. Automat. Contr., 51 (2006), 511–518. https://doi.org/10.1109/TAC.2005.864207 doi: 10.1109/TAC.2005.864207
![]() |
∘ | △L | α | β | γ | δ | ▽L |
△L | △L | α | β | γ | δ | ▽L |
α | α | α | β | ▽L | β | ▽L |
β | β | β | β | ▽L | β | ▽L |
γ | γ | ▽L | ▽L | γ | ▽L | ▽L |
δ | δ | β | β | ▽L | δ | ▽L |
▽L | ▽L | ▽L | ▽L | ▽L | ▽L | ▽L |
∘ | △L∘∘ | αL∘∘ | γL∘∘ | ▽L∘∘ |
△L∘∘ | △L∘∘ | αL∘∘ | γL∘∘ | ▽L∘∘ |
αL∘∘ | αL∘∘ | αL∘∘ | ▽L∘∘ | ▽L∘∘ |
γL∘∘ | γL∘∘ | ▽L∘∘ | γL∘∘ | ▽L∘∘ |
▽L∘∘ | ▽L∘∘ | ▽L∘∘ | ▽L∘∘ | ▽L∘∘ |
∘ | △D(L) | ▽D(L) |
△D(L) | △D(L) | ▽D(L) |
▽D(L) | ▽D(L) | ▽D(L) |