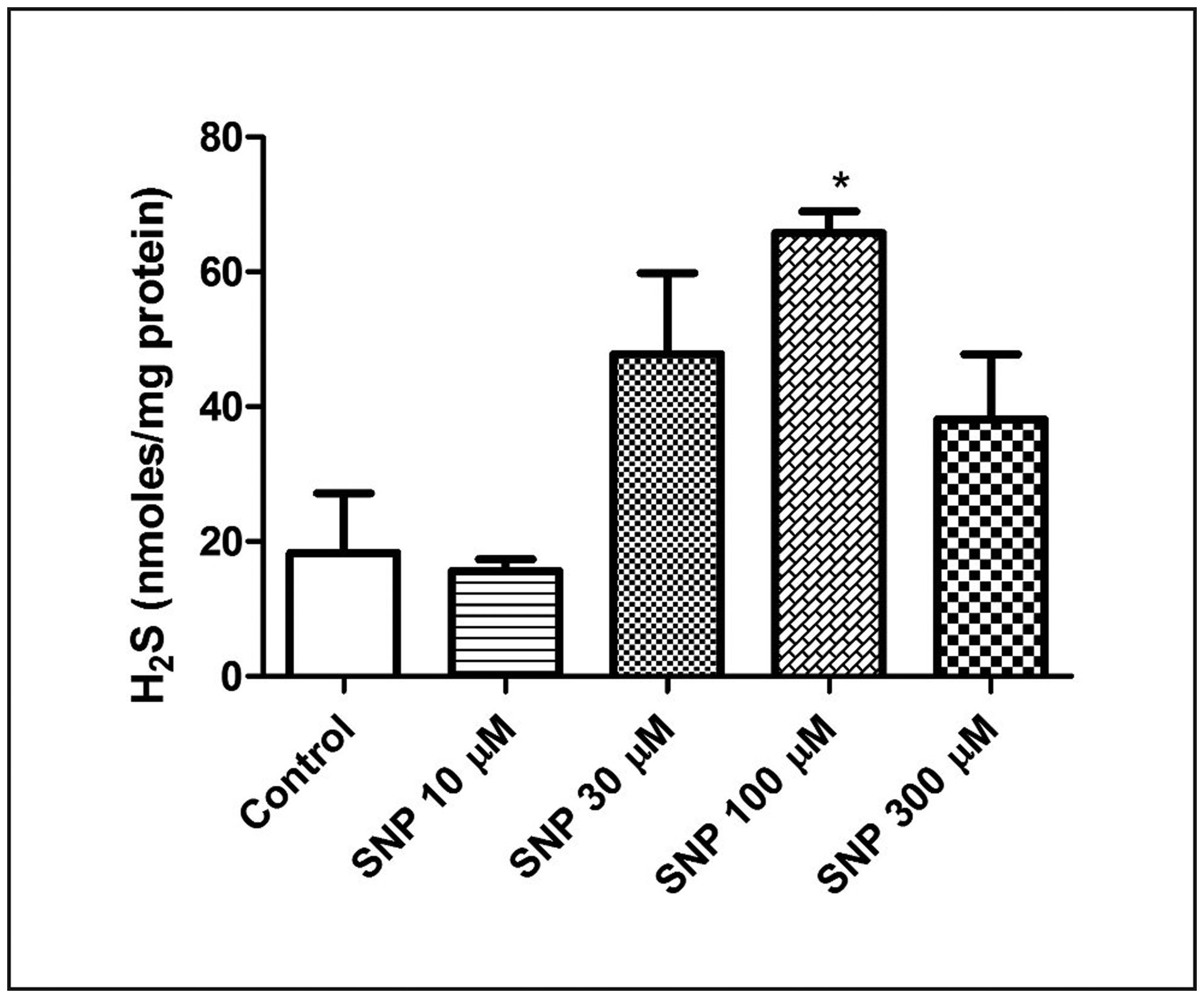
Citation: Farzad Fatehi, Yuliya N. Kyrychko, Konstantin B. Blyuss. Time-delayed model of autoimmune dynamics[J]. Mathematical Biosciences and Engineering, 2019, 16(5): 5613-5639. doi: 10.3934/mbe.2019279
[1] | I. Banegas, I. Prieto, A.B. Segarra, M. Martínez-Cañamero, M. de Gasparo, M. Ramírez-Sánchez . Angiotensin II, dopamine and nitric oxide. An asymmetrical neurovisceral interaction between brain and plasma to regulate blood pressure. AIMS Neuroscience, 2019, 6(3): 116-127. doi: 10.3934/Neuroscience.2019.3.116 |
[2] | Nicholas J. D. Wright . A review of the actions of Nitric Oxide in development and neuronal function in major invertebrate model systems. AIMS Neuroscience, 2019, 6(3): 146-174. doi: 10.3934/Neuroscience.2019.3.146 |
[3] | Valentina Bashkatova, Athineos Philippu . Role of nitric oxide in psychostimulant-induced neurotoxicity. AIMS Neuroscience, 2019, 6(3): 191-203. doi: 10.3934/Neuroscience.2019.3.191 |
[4] | Md. Mamun Al-Amin, Robert K. P. Sullivan, Suzy Alexander, David A. Carter, DanaKai Bradford, Thomas H. J. Burne . Impaired spatial memory in adult vitamin D deficient BALB/c mice is associated with reductions in spine density, nitric oxide, and neural nitric oxide synthase in the hippocampus. AIMS Neuroscience, 2022, 9(1): 31-56. doi: 10.3934/Neuroscience.2022004 |
[5] | Amber Woodard, Brandon Barbery, Reid Wilkinson, Jonathan Strozyk, Mathew Milner, Patrick Doucette, Jarred Doran, Kendra Appleby, Henry Atwill, Wade E. Bell, James E. Turner . The role of neuronal nitric oxide and its pathways in the protection and recovery from neurotoxin-induced de novo hypokinetic motor behaviors in the embryonic zebrafish (Danio rerio). AIMS Neuroscience, 2019, 6(1): 25-42. doi: 10.3934/Neuroscience.2019.1.25 |
[6] | Gigi Tevzadze, Tamar Barbakadze, Elisabed Kvergelidze, Elene Zhuravliova, Lali Shanshiashvili, David Mikeladze . Gut neurotoxin p-cresol induces brain-derived neurotrophic factor secretion and increases the expression of neurofilament subunits in PC-12 cells. AIMS Neuroscience, 2022, 9(1): 12-23. doi: 10.3934/Neuroscience.2022002 |
[7] | Ernest Greene . New encoding concepts for shape recognition are needed. AIMS Neuroscience, 2018, 5(3): 162-178. doi: 10.3934/Neuroscience.2018.3.162 |
[8] | Adeshina O. Adekeye, Adedamola A. Fafure, Ayoola E. Ogunsemowo, Linus A. Enye, Olusola S. Saka, Oluwatosin O. Ogedengbe . Naringin ameliorates motor dysfunction and exerts neuroprotective role against vanadium-induced neurotoxicity. AIMS Neuroscience, 2022, 9(4): 536-550. doi: 10.3934/Neuroscience.2022031 |
[9] | Nouhaila Chaoui, Hammou Anarghou, Meriem Laaroussi, Oumaima Essaidi, Mohamed Najimi, Fatiha Chigr . Long lasting effect of acute restraint stress on behavior and brain anti-oxidative status. AIMS Neuroscience, 2022, 9(1): 57-75. doi: 10.3934/Neuroscience.2022005 |
[10] | Ernest Greene, Michael J. Hautus . Demonstrating Invariant Encoding of Shapes Using A Matching Judgment Protocol. AIMS Neuroscience, 2017, 4(3): 120-147. doi: 10.3934/Neuroscience.2017.3.120 |
The presence of enzymes responsible for the biosynthesis of hydrogen sulfide (H2S) from the amino acid, L-cysteine in mammalian tissues has stimulated a surge of interest in its potential physiological significance in biological processes [1],[2]. There is evidence that H2S can share similar physiological and pharmacological actions with other gaseous transmitters such as nitric oxide (NO) and carbon monoxide (CO) [3]–[5]. In some pathophysiological conditions, the three gaseous molecules are present in specific concentrations in disease states such as Alzheimer's when their endogenous production is known to be compromised [6]–[8]. Although these gases possess similar features and modes of action, H2S has been reported to exhibit a number of distinct characteristics from both NO and CO [7]. For instance, H2S has greater water solubility and the lowest potential to penetrate lipid bilayers, and has a different pathway that leads to its biosynthesis when compared with its two gaseous counterparts [9]. The basal production of H2S is dependent upon the activity of two pyridoxal-5′-phosphate dependent-enzymes, cystathionine β-synthase (CBS) and cystathionine γ-lyase (CSE), whilst NO is synthesized from L-arginine by NO synthase and CO is endogenously produced from heme by heme oxygenase [10]–[15]. In the presence of aspartate aminotransferase, L-cysteine can also be converted to H2S by the enzyme, 3-mercaptopyruvate sulfur-transferase (3MST) [16]–[20]. Since the physiological and pharmacological targets for H2S are analogous to NO and CO, evidence available from literature supports an interaction between these gaseous transmitters in mammalian organs and tissues [7],[21],[22]. Indeed, studies in neuronal tissues and the vasculature show that the interaction between these gases can affect their biosynthetic pathways, as well as, induce changes in their biological responses [23]. While H2S has been shown to alter the activity and expression of NO producing enzyme (eNOS), there is evidence that NO substrate or donors can also modulate expression and activity of H2S producing enzymes [3],[7],[24]–[26]. Furthermore, studies by Whiteman et al. [27] suggest that H2S may interact with NO to form an unidentified nitrosothiol moiety, which may then regulate physiological functions of both NO and H2S [24]. Likewise, there is compelling evidence of an interaction between H2S and CO. H2S has been reported to play a regulatory role in CO/heme oxygenase pathways in cardiac and pulmonary diseases [28]. Indeed, under physiological conditions, CO/heme oxygenase and H2S/CSE pathways have been demonstrated to inhibit each other in aortic smooth muscle cells [29]. However, it remains to be determined whether NO and CO can interact with the pathway leading to H2S biosynthesis in the retina. The aim of the present study was, therefore, to investigate whether inhibitors and/or activators of both NO and CO pathways can alter H2S concentrations in the bovine isolated neural retina. Parts of the work described in this paper have been communicated in an abstract form [30].
Hemin, zinc protoporphyrin (ZnPP-IX), L-nitroarginine methyl ester (L-NAME), sodium nitroprusside (SNP), aminooxyacetic acid (AOAA), ethylenediamine tetra-acetic acid solution (EDTA), zinc acetate, ferric chloride, borate buffer (pH 11.0) and N, N-dimethyl-p-phenylenediamine sulfate were all purchased from Sigma–Aldrich, St. Louis, MO. All reagents were freshly prepared in distilled water, except N, N-dimethyl-p-phenylenediamine which was prepared in 7.2 M hydrochloric acid, immediately before use in the series of experiments.
Bovine eyes were obtained from Vision Tech, Dallas, TX within 24 hours following enucleation and transported to the laboratory on ice. An incision was made along the equator of each eye and the vitreous humor and lens were delicately removed. The neural retinae were isolated by gentle removal from the posterior segment of the eye and immediately immersed in ice-cold 50 mM potassium phosphate buffer (pH 7.4). Time elapsed between animal sacrifice and tissue preparation was less than 24 hours.
H2S production was measured using the Methylene Blue method, a widely used assay that has been shown to produce quantifiable measurements of H2S production in mammalian tissues comparable to data obtained using H2S probes [31]–[35]. The methodology employed for H2S measurement in retinal tissues was essentially the same as previously reported by Xia et al. [36] and Kulkarni et al. [37], with some minor modifications. In brief, isolated bovine neural retina were homogenized in freshly prepared 50 mM ice-cold potassium phosphate buffer (pH 7.4) using a hand held tissue grinder consisting of a pestle and mortar. The tissue homogenates (0.25 ml) were incubated in the presence or absence of NO synthase inhibitor, L-nitroarginine methyl ester (L-NAME; 300 nM–500 µM), NO donor, sodium nitroprusside (SNP; 10–300 µM), hemoxygenase-1 inhibitor, zinc protoporphyrin (ZnPP-IX; 300 nM–10 µM) and hemoxygenase-1 inducer, hemin (300 nM–20 µM). The drug concentrations chosen were based on previously published reports on the pharmacological effects of NO and CO on mammalian tissues and cells [29],[37]. Incubation was carried at 37 °C for 90 min in tightly sealed eppendorf tubes for the enzymatic reaction to take place for optimum production of H2S as reported by [36],[37]. EDTA (0.125 ml; 5 mM) was injected into the reaction tubes to stop the reaction, followed by 0.5 ml of zinc acetate (1%) and 0.5 ml of borate buffer (pH 11.0). The tubes were sealed and then incubated at 37°C for another 30 minutes. The reaction solution was mixed with 0.5 ml of N, N-dimethyl-p-phenylenediamine sulfate (20 mM, in 7.2 M HCl) and 0.5 ml of FeCl3 (30 mM) at 37 °C for an additional 10 minutes and then centrifuged at 5000g for 3 min. The absorbance of resulting solution was measured at 670 nm with a spectrophotometer. The H2S concentration was calculated against the calibration curve of standard H2S solutions (10–750 µM). The protein content of the residual pellet after centrifugation was measured using the Bradford method.
Results were expressed as nanomolar of H2S produced per milligram soluble protein. Values given are arithmetic means ± SEM. The means were determined from three separate experiments performed in triplicates. Significance of differences between control and treatment groups were evaluated using one-way analysis of variance (ANOVA) followed by Newman-Keul comparison test. (Graph Pad Prism Software, San Diego, CA). The level of significance was chosen as p < 0.05.
In a previous study, we showed evidence that endogenous concentrations of H2S in isolated bovine retinae were enhanced by the substrate, L-cysteine and attenuated by inhibitors of enzymes responsible for the biosynthesis of this gas [37]. In the present study, the NO donor, SNP (10–100 µM) caused a concentration-dependent increase in H2S levels reaching a maximum at 100 µM (65.7 nm/mg of protein) (Figure 1). On other hand, the NO inhibitor L-NAME (300 nM–500 µM) elicited a concentration-dependent decrease in the basal endogenous levels of H2S from 19.2 to 2.6 nm/mg of protein (Figure 2).
We next investigated the pharmacological action of the heme oxygenase-1 inducer, hemin (300 nM–20 µM) on basal concentrations of H2S in the bovine isolated retina. As shown in Figure 3, hemin produced a concentration-dependent attenuation of basal levels of H2S from 18.9 to 3.6 nm/mg of protein. Conversely, the heme oxygenase inhibitor, ZnPP-IX (300 nM–10 µM) significantly increased the concentration of H2S from 19.2 to 26.5 nm/mg of protein in a concentration-dependent manner, reaching a maximal effect at 10 µM (Figure 4).
It is well-established that H2S can serve as a gaseous neurotransmitter/neuromodulator in several mammalian tissues and organs. Indeed, there is abundant evidence that H2S is involved in several physiological and pathophysiological processes as diverse as learning and memory, inflammation, and the regulation of blood pressure [6],[38],[39]. In the cardiovascular system, H2S has been shown to play an important role in maintenance of vascular smooth muscle tone whereas in the central nervous system, this gas has been found to act as a neurotransmitter/neuromodulator at synapses [40]–[43]. Furthermore, H2S has also been reported to exert a neuroprotective action on neurons [11]. The observed biological actions of H2S in the vasculature and brain have been reported to resemble those of other established gaseous transmitters like CO and NO [44],[45]. Although, studies reported in literature have focused on understanding the physiological and pharmacological roles of H2S in cardiovascular, central nervous and immune systems, there is limited evidence of an interaction between NO, CO and H2S in regulating some of the observed responses in the above-named systems [8]. In the eye, changes in cyclic GMP levels have been reported due to an interaction between these gaseous transmitters in the salamander retina and the rabbit ophthalmic artery [46],[47]. In the present study, we investigated the possibility that activation or inhibition of pathways involved in NO and CO biosynthesis can alter basal endogenous levels of H2S in the bovine isolated retina.
There is evidence that in the cardiovascular and central nervous systems, nitric oxide synthase (NOS) substrates or NO donors can up-regulate the expression or activity of H2S producing enzymes [48]–[51]. Other investigators also report that L-arginine can attenuate pulmonary artery pressure by augmenting the expression and activity of CSE mRNA in lung tissue [52]. Furthermore, there is evidence suggesting that a synergistic interaction exists between NO and H2S in regulating vascular function [53]–[56]. In the present study, we measured endogenous levels of H2S in the presence of NO donor, SNP. The incubation time period for optimal production of endogenous basal levels of H2S was previously established in our laboratory [37]. We found that SNP increased the basal endogenous levels of H2S suggesting that increased NO availability can regulate the production of this gas in the retina. We also observed that at a high concentration of SNP (300 µM) the basal endogenous levels of H2S was decreased, suggesting that NO may also have the ability to inhibit H2S production. The precise mechanism for this effect is not clear. However, it may be due to the fact that at high concentrations of SNP, the NO generated binds to CBS and thus has the potential to inhibit its activity and therefore the generation of H2S. Despite this, our observations correlate well with studies in the vasculature depicting the ability of SNP to increase the biosynthesis of H2S [48]–[50]. Indeed, there is evidence that in the presence of NO, the pharmacological actions of H2S are significantly enhanced [8],[56]. The ability of NO to stimulate H2S production may be due to an augmented activity of enzymes responsible for the biosynthesis of H2S by the NO-induced S-nitrosylation of their cysteine residues or indirect stimulation of CSE by NO through an increase in the activity of cyclic GMP-dependent kinases [8],[35],[45]. Taken together, these results indicate that NO may interact directly with CBS and CSE to augment H2S production. To confirm the nature of this interaction, we investigated the effect of an inhibitor of NO synthase, L-NAME on endogenous H2S production. Our results demonstrate that L-NAME reduced basal H2S levels suggesting that endogenously generated NO can modulate the basal levels of H2S presumably through an interaction with pathways involved in H2S production. Other studies in the vasculature have also reported a similar inhibitory effect of L-NAME on H2S production and activity [8],[53]. The exact mechanisms +that underlie H2S and NO interactions in the neural retinae are unknown and merits further investigation.
In addition to studying the possible interaction between NO and H2S, we designed experiments to determine whether changes in CO production can regulate the endogenous levels of H2S in neural retinae. In these studies, hemin and ZnPP-IX were employed as an inducer and an inhibitor of heme oxygenase, respectively [57],[58]. We found that hemin caused a concentration-related decrease in basal levels of H2S. In contrast, ZnPP-IX elicited a concentration-dependent increase in endogenous H2S levels. Our results reveal that increasing CO production led to a decrease in basal concentrations of H2S whereas, inhibiting CO biosynthesis can cause an elevation of H2S levels. These results correlate well with studies reported by Jin et al. [29] who showed that endogenous CO down-regulates the H2S production in aortic smooth muscle cells. A similar interaction between these two gaseous transmitters has also been observed in the carotid body, the liver, and the microvasculature of the brain [59]–[61]. It appears that in the bovine retina, reduction of CO biosynthesis removes a tonic inhibitory action of this gas on H2S production. It is, however, unclear how CO acts to regulate the biosynthesis of H2S in the retina.
Previous evidence from our laboratory and others suggests that crosstalk between the three gasotransmitters exists in ocular tissues [46],[47],[62]–[64]. In the salamander retina, all three gases were found to play interactive roles in modulating retinal cGMP signaling, as NO and CO significantly increased cGMP-like immunoreactivity (cGMP-LI) both alone and synergistically [46]. NaHS was found to inhibit the NO-induced increase in cGMP-LI from the inner nuclear layer to the ganglion cell layer, indicating an interaction in these three gases in the retina [46]. H2S was found to enhance phenylephrine induced tone in the rabbit ophthalmic artery, while CO and NO both caused concentration dependent relaxations [47]. The mechanisms behind these pharmacological actions may be attributed to the gaseous transmitters' ability to modulate cGMP production in the rabbit model, as H2S was found to reduce cGMP levels and prevent a NO induced increase in cGMP [47]. The relaxing effect of hydrogen sulfide donors on smooth muscles in both bovine and porcine ocular tissues have also been found to be dependent, at least in part, on the presence and activity of nitric oxide [62]–[64]. Taken together, these studies implicate an interactive regulatory role of these gaseous modulators in ocular tissues.
In conclusion, changes in the biosynthesis and availability of both NO and CO can interfere with the pathway/s involved in the production of H2S in the bovine isolated retina. The present observation that basal levels of H2S can be altered by manipulation of pathways involved in NO/CO production is of great interest and merits further investigation.
Despite these novel findings, the results should be interpreted with prudence due to limitation of our study protocol. For instance the colorimetric methodology used for determination of endogenous production of H2S is based on the assumption that tissue concentration of sulfide are high, and hence it does not distinguish between acid labile sulfur stores or bound sulfane stores. In addition the enzyme inhibitors being used in the studies are pyridoxal-5′ phosphate (PLP) enzyme inhibitors that are not specific for H2S biosynthesizing enzymes, CBS and CSE as these enzymes are known to be involved in other cellular processes in mammals. Thus, it would be worthwhile to investigate whether H2S synthesis alters the expression of these biosynthesizing enzymatic proteins. Despite the limitations of the study protocol, the results provide a basis for future studies on the role of H2S in the posterior segment of the eye.
[1] | A. K. Abbas, A. H. H. Lichtman and S. Pillai, Cellular and Molecular Immunology, Elsevier Health Sciences, 2015. |
[2] | D. Mason, A very high level of crossreactivity is an essential feature of the T-cell receptor, Immunol. Today, 19 (1998), 395–404. |
[3] | E. M. Shevach, R. S. McHugh, C. A. Piccirillo, et al., Control of T-cell activation by CD4+CD25+ suppressor T cells, Immunol. Rev., 182 (2001), 58–67. |
[4] | A. M. Thornton and E. M. Shevach, CD4+CD25+ immunoregulatory T cells suppress polyclonal T cell activation in vitro by inhibiting interleukin 2 production, J. Exp. Med., 188 (1998), 287–296. |
[5] | A. Corthay, How do regulatory T cells work?, Scand. J. Immunol., 70 (2009), 326–336. |
[6] | D. Buljevac, H. Z. Flach, W. C. J. Hop, et al., Prospective study on the relationship between infections and multiple sclerosis exacerbations, Brain, 125 (2002), 952–960. |
[7] | D. Germolic, D. H. Kono, J. C. Pfau, et al., Animal models used to examine the role of environment in the development of autoimmune disease: findings from an NIEHS Expert Panel Workshop, J. Autoimmun., 39 (2012), 285–293. |
[8] | M. P. Mallampalli, E. Davies, D. Wood, et al., Role of environment and sex differences in the development of autoimmune disease: a roundtable meeting report, J. Womens Health, 22 (2013), 578–586. |
[9] | B. Krone and J. M. Grange, Multiple sclerosis: are protective immune mechanisms compromised by a complex infectious background?, Autoimmune Dis., 2011 (2010), 708750. |
[10] | M. Ohashi, N. Orlova, C. Quink, et al., Cloning of the Epstein-Barr virus-related rhesus lymphocryptovirus as a bacterial artificial chromosome: a loss-of-function mutation of the rhBARF1 immune evasion gene, J. Virol., 85 (2011), 1330–1339. |
[11] | D. Hober and P. Sauter, Pathogenesis of type 1 diabetes mellitus: interplay between enterovirus and host, Nat. Rev. Endocrinol., 6 (2010), 279–289. |
[12] | S. E. Myers, L. Brewer, D. P. Shaw, et al., Prevalent human coxsackie B-5 virus infects porcine islet cells primarily using the coxsackie-adenovirus receptor, Xenotransplantation, 11 (2004), 536–546. |
[13] | K. Döhner, K. Radtke, S. Schmidt, et al., Eclipse phase of herpes simplex virus type 1 infection: Efficient dynein-mediated capsid transport without the small capsid protein VP26, J. Virol., 80 (2006), 8211–8224. |
[14] | U. Maurer, B. Sodeik and K. Gruenewald, Native 3D intermediates of membrane fusion in herpes simplex virus 1 entry, Proc. Natl. Acad. Sci. USA, 105 (2008), 10559–10564. |
[15] | S. Manfredo Vieira, M. Hiltensperger, V. Kumar, et al., Translocation of a gut pathobiont drives autoimmunity in mice and humans, Science, 359 (2018), 1156–1161. |
[16] | R. S. Fujinami, Can virus infections trigger autoimmune disease?, J. Autoimmun., 16 (2001), 229–234. |
[17] | A. M. Ercolini and S. D. Miller, The role of infections in autoimmune disease, Clin. Exp. Immunol., 155 (2009), 1–15. |
[18] | R. S. Fujinami, M. G. von Herrath, U. Christen, et al., Molecular mimicry, bystander activation, or viral persistence: infections and autoimmune disease, Clin. Microbiol. Rev., 19 (2006), 80–94. |
[19] | R. S. Fujinami, M. B. Oldstone, Z. Wroblewska, et al., Molecular mimicry in virus infection: crossreaction of measles virus phosphoprotein or of herpes simplex virus protein with human intermediate filaments, Proc. Natl. Acad. Sci. USA, 80 (1983), 2346–2350. |
[20] | M. G. von Herrath and M. B. A. Oldstone, Virus-induced autoimmune disease, Curr. Opin. Immunol., 8 (1996), 878–885. |
[21] | C. Münz, J. D. Lünemann, M. T. Getts, et al., Antiviral immune responses: triggers of or triggered by autoimmunity?, Nat. Rev. Immunol., 9 (2009), 246. |
[22] | S. Bonhoeffer, R. M. May, G. M. Shaw, et al., Virus dynamics and drug therapy, Proc. Natl. Acad. Sci. USA, 94 (1997), 6971–6976. |
[23] | M. A. Nowak and C. R. Bangham, Population dynamics of immune responses to persistent viruses, Science-AAAS-Weekly Paper Edition, 272 (1996), 74–79. |
[24] | A. S. Perelson, Viral kinetics and mathematical models, Am. J. Med., 107 (Suppl 2) (1999), 49–52. |
[25] | A. S. Perelson, Modelling viral and immune system dynamics, Nat. Rev. Immunol., 2 (2002), 28–36. |
[26] | P. Baccam, C. Beauchemin, C. A. Macken, et al., Kinetics of influenza A infection in humans, J. Virol., 80 (2006), 7590–7599. |
[27] | C. A. A. Beauchemin, J. J. McSharry, G. L. Drusano, et al., Modeling amantadine treatment of influenza A virus in vitro, J. Theor. Biol., 254 (2008), 439–451. |
[28] | C. A. A. Beauchemin and A. Handel, A review of mathematical models of influenza A infections within a host or cell culture: lessons learned and challenges ahead, BMC Public Health, 11 (Suppl 1) (2011), S7. |
[29] | A. S. Perelson, A. Neumann, M. Markowitz, et al., HIV-1 dynamics in vivo: Virion clearance rate, infected cell life-span, and viral generation time, Science, 271 (1996), 1582–1586. |
[30] | A. S. Perelson, P. Essunger, Y. Cao, et al., Decay characteristics of HIV-1 infected compartments during combination therapy, Nature, 387 (1997), 188–191. |
[31] | M. A. Nowak, S. Bonhoeffer, A. M. Hill, et al., Viral dynamics in hepatitis b virus infection, Proc. Natl. Acad. Sci. USA, 93 (1996), 4398–4402. |
[32] | A. U. Neumann, N. P. Lam, H. Dahari, et al., Hepatitis C viral dynamics in vivo and the antiviral efficacy of interferon-alpha therapy, Science, 282 (1998), 103–107. |
[33] | A. U. Neumann, N. P. Lam, H. Dahari, et al., Differences in viral dynamics between genotypes 1 and 2 of hepatitis C virus, J. Infect. Dis., 182 (2000), 28–35. |
[34] | R. M. Ribeiro, J. Layden-Almer, K. A. Powers, et al., Dynamics of alanine aminotransferase during hepatitis C virus treatment, Hepatology, 38 (2003), 509–517. |
[35] | L. A. Segel, E. Jäger, D. Elias, et al., A quantitative model of autoimmune disease and T-cell vaccination: does more mean less?, Immunol. Today, 16 (1995), 80–84. |
[36] | J. A. M. Borghans and R. J. De Boer, A minimal model for T-cell vaccination, Proc. R. Soc. Lond. B Biol. Sci., 259 (1995), 173–178. |
[37] | J. A. M. Borghans, R. J. De Boer, E. Sercarz, et al., T cell vaccination in experimental autoimmune encephalomyelitis: a mathematical model, J. Immunol., 161 (1998), 1087–1093. |
[38] | S. Iwami, Y. Takeuchi, Y. Miura, et al., Dynamical properties of autoimmune disease models: tolerance, flare-up, dormancy, J. Theor. Biol., 246 (2007), 646–659. |
[39] | S. Iwami, Y. Takeuchi, K. Iwamoto, et al., A mathematical design of vector vaccine against autoimmune disease, J. Theor. Biol., 256 (2009), 382–392. |
[40] | K. León, R. Perez, A. Lage, et al., Modelling T-cell-mediated suppression dependent on interactions in multicellular conjugates, J. Theor. Biol., 207 (2000), 231–254. |
[41] | K. León, A. Lage and J. Carneiro, Tolerance and immunity in a mathematical model of T-cell mediated suppression, J. Theor. Biol., 225 (2003), 107–126. |
[42] | K. León, J. Faro, A. Lage, et al., Inverse correlation between the incidences of autoimmune disease and infection predicted by a model of T cell mediated tolerance, J. Autoimmun., 22 (2004), 31–42. |
[43] | J. Carneiro, T. Paixão, D. Milutinovic, et al., Immunological self-tolerance: lessons from mathematical modeling, J. Comput. Appl. Math., 184 (2005), 77–100. |
[44] | D. Wodarz and V. A. A. Jansen, A dynamical perspective of CTL cross-priming and regulation: implications for cancer immunology, Immunol. Lett., 86 (2003), 213–227. |
[45] | R. Root-Bernstein, Theories and modeling of autoimmunity, J. Theor. Biol., 375 (2015), 1–124. |
[46] | J. D. Fontenot, M. A. Gavin and A. Y. Rudensky, Foxp3 programs the development and function of CD4+CD25+ regulatory T cells, Nat. Immunol., 4 (2003), 330–336. |
[47] | S. Sakaguchi, Naturally arising CD4+ regulatory T cells for immunologic self-tolerance and negative control of immune responses, Annu. Rev. Immunol., 22 (2004), 531–562. |
[48] | S. Z. Josefowicz, L. F. Lu and A. Y. Rudensky, Regulatory T cells: Mechanisms of differentiation and function, Annu. Rev. Immunol., 30 (2012), 531–564. |
[49] | H. K. Alexander and L. M. Wahl, Self-tolerance and autoimmunity in a regulatory T cell model, Bull. Math. Biol., 73 (2011), 33–71. |
[50] | N. J. Burroughs, M. Ferreira, B. M. P. M. Oliveira, et al., A transcritical bifurcation in an immune response model, J. Differ. Equ. Appl., 17 (2011), 1101–1106. |
[51] | N. J. Burroughs, M. Ferreira, B. M. P. M. Oliveira, et al., Autoimmunity arising from bystander proliferation of T cells in an immune response model, Math. Comput. Model., 53 (2011), 1389–1393. |
[52] | Z. Grossman and W. E. Paul, Adaptive cellular interactions in the immune system: the tunable activation threshold and the significance of subthreshold responses, Proc. Natl. Acad. Sci. USA, 89 (1992), 10365–10369. |
[53] | Z. Grossman and A. Singer, Tuning of activation thresholds explains flexibility in the selection and development of T cells in the thymus, Proc. Natl. Acad. Sci. USA, 93 (1996), 14747–14752. |
[54] | Z. Grossman and W. E. Paul, Self-tolerance: context dependent tuning of T cell antigen recognition, Semin. Immunol., 12 (2000), 197–203. |
[55] | A. J. Noest, Designing lymphocyte functional structure for optimal signal detection: voilá, T cells, J. Theor. Biol., 207 (2000), 195–216. |
[56] | D. A. Peterson, R. J. DiPaolo, O. Kanagawa, et al., Cutting edge: negative selection of immature thymocytes by a few peptide-MHC complexes: differential sensitivity of immature and mature T cells, J. Immunol., 162 (1999), 3117–3120. |
[57] | L. B. Nicholson, A. C. Anderson and V. K. Kuchroo, Tuning T cell activation threshold and effector function with cross-reactive peptide ligands, Int. Immunol., 12 (2000), 205–213. |
[58] | P. Wong, G. M. Barton, K. A. Forbush et al., Dynamic tuning of T cell reactivity by self-peptide-major histocompatibility complex ligands, J. Exp. Med., 193 (2001), 1179–1187. |
[59] | A. D. Bitmansour, D. C. Douek, V. C. Maino, et al., Direct ex vivo analysis of human CD4+ memory T cell activation requirements at the single clonotype level, J. Immunol., 169 (2002), 1207–1218. |
[60] | I. Stefanová, J. R. Dorfman and R. N. Germain, Self-recognition promotes the foreign antigen sensitivity of naive T lymphocytes, Nature, 420 (2002), 429–434. |
[61] | G. Altan-Bonnet and R. N. Germain, Modeling T cell antigen discrimination based on feedback control of digital ERK responses, PLoS Biol., 3 (2005), e356. |
[62] | H. A. van den Berg and D. A. Rand, Dynamics of T cell activation threshold tuning, J. Theor. Biol., 228 (2004), 397–416. |
[63] | A. Scherer, A. Noest and R. J. de Boer, Activation-threshold tuning in an affinity model for the T-cell repertoire, Proc. R. Soc. Lond. B Biol. Sci., 271 (2004), 609–616. |
[64] | A. R. McLean, Modelling T cell memory, J. Theor. Biol., 170 (1994), 63–74. |
[65] | C. Utzny and N. J. Burroughs, Perturbation theory analysis of competition in a heterogeneous population, Physica D, 175 (2003), 109–126. |
[66] | N. J. Burroughs, B. M. P. M. de Oliveira and A. A. Pinto, Regulatory T cell adjustment of quorum growth thresholds and the control of local immune responses, J. Theor. Biol., 241 (2006), 134–141. |
[67] | N. J. Burroughs, B. M. P. M. Oliveira, A. A. Pinto, et al., Sensibility of the quorum growth thresholds controlling local immune responses, Math. Comput. Model., 47 (2008), 714–725. |
[68] | A. L. DeFranco, R. M. Locksley and M. Robertson, Immunity: The immune response in infectious and inflammatory disease, New Science Press Ltd., 2007. |
[69] | A. Toda and C. A. Piccirillo, Development and function of naturally occurring CD4+CD25+ regulatory T cells, J. Leukoc. Biol., 80 (2006), 458–470. |
[70] | P. S. Kim, P. P. Lee and D. Levy, Modeling regulation mechanisms in the immune system, J. Theor. Biol., 246 (2007), 33–69. |
[71] | B. M. P. M. Oliveira, R. Trinchet, M. V. O. Espinar, et al., Modelling the suppression of autoimmunity after pathogen infection, Math. Meth. Appl. Sci., 41 (2018), 8565–8570. |
[72] | J. Tam, Delay effect in a model for virus replication, IMA J. Math. Appl. Med. Biol., 16 (1999), 29–37. |
[73] | R. V. Culshaw and S. Ruan, A delay-differential equation model of HIV infection of CD4+ T-cells, Math. Biosci., 165 (2000), 27–39. |
[74] | P. W. Nelson and A. S. Perelson, Mathematical analysis of delay differential equation models of HIV-1 infection, Math. Biosci., 179 (2002), 73–94. |
[75] | X. Zhou, X. Song and X. Shi, Analysis of stability and Hopf bifurcation for an HIV infection model with time delay, Appl. Math. Comput., 199 (2008), 23–38. |
[76] | A. J. Yates, M. Van Baalen and R. Antia, Virus replication strategies and the critical CTL numbers required for the control of infection, PLoS Comput. Biol., 7 (2011), e1002274. |
[77] | G. J. M. Webster, S. Reignat, M. K. Maini, et al., Incubation phase of acute hepatitis B in man: dynamic of cellular immune mechanisms, Hepatology, 32 (2000), 1117–1124. |
[78] | M. S. Ciupe, B. L. Bivort, D. M. Bortz, et al., Estimating kinetic parameters from HIV primary infection data through the eyes of three different mathematical models, Math. Biosci., 200 (2006), 1–27. |
[79] | M. P. Davenport, R. M. Ribeiro and A. S. Perelson, Kinetics of virus-specific CD8+ T cells and the control of human immunodeficiency virus infection, J. Virol., 78 (2004), 10096–10103. |
[80] | R. Thimme, J. Bukh, H. C. Spangenberg, et al., Viral and immunological determinants of hepatitis C virus clearance, persistence, and disease, Proc. Natl. Acad. Sci. USA, 99 (2002), 15661–15668. |
[81] | K. B. Blyuss and L. B. Nicholson, The role of tunable activation thresholds in the dynamics of autoimmunity, J. Theor. Biol., 308 (2012), 45–55. |
[82] | K. B. Blyuss and L. B. Nicholson, Understanding the roles of activation threshold and infections in the dynamics of autoimmune disease, J. Theor. Biol., 375 (2015), 13–20. |
[83] | D. Ben Ezra and J. V. Forrester, Fundal white dots: the spectrum of a similar pathological process, Br. J. Ophthalmol., 79 (1995), 856–860. |
[84] | T. F. Davies, D. C. Evered, B. Rees Smith, et al., Value of thyroid-stimulating-antibody determination in predicting the short-term thyrotoxic relapse in Graves' disease, Lancet, 309 (1997), 1181–1182. |
[85] | A. Nylander and D. A. Hafler, Multiple sclerosis, J. Clin. Invest., 122 (2012), 1180–1188. |
[86] | F. Fatehi, Y. N. Kyrychko, R. Molchanov, et al., Bifurcations and multi-stability in a model of cytokine-mediated autoimmunity, Int. J. Bif. Chaos, 29 (2019), 1950034. |
[87] | F. Fatehi, Y. N. Kyrychko and K. B. Blyuss, Effects of viral and cytokine delays on dynamics of autoimmunity, Mathematics, 6 (2018), 66. |
[88] | F. Fatehi, S. N. Kyrychko, A. Ross, et al., Stochastic effects in autoimmune dynamics, Front. Physiol., 9 (2018), 45. |
[89] | D. A. Copland, M. S. Wertheim, W. J. Armitage, et al., The clinical time-course of Experimental Autoimmune Uveoretinitis using topical endoscopic fundal imaging with histologic and cellular infiltrate correlation, Invest. Ophthalmol. Vis. Sci., 49 (2008), 5458–5465. |
[90] | J. Boldison, T. K. Khera, D. A. Copland, et al., A novel pathogenic RBP-3 peptide reveals epitope spreading in persistent experimental autoimmune uveoretinitis, Immunology, 146 (2015), 301–311. |
[91] | P. Krishnapriya and M. Pitchaimani, Analysis of time delay in viral infection model with immune impairment, J. Appl. Math. Comput., 55 (2017), 421–453. |
[92] | S. D. Wolf, B. N. Dittel, F. Hardardottir, et al., Experimental autoimmune encephalomyelitis induction in genetically B cell-deficient mice, J. Exp. Med., 184 (1996), 2271–2278. |
[93] | H. J. Wu, I. I. Ivanov, J. Darce, et al., Gut-residing segmented filamentous bacteria drive autoimmune arthritis via T helper 17 cells, Immunity, 32 (2010), 815–827. |
[94] | I. Baltcheva, L. Codarri, G. Pantaleo, et al., Lifelong dynamics of human CD4+CD25+ regulatory T cells: Insights from in vivo data and mathematical modeling, J. Theor. Biol., 266 (2010), 307–322. |
[95] | J. Li, L. Zhang and Z. Wang, Two effective stability criteria for linear time-delay systems with complex coefficients, J. Syst. Sci. Complex., 24 (2011), 835–849. |
[96] | B. Rahman, K. B. Blyuss and Y. N. Kyrychko, Dynamics of neural systems with discrete and distributed time delays, SIAM J. Appl. Dyn. Syst., 14 (2015), 2069–2095. |
[97] | A. Skapenko, J. Leipe, P. E. Lipsky, et al., The role of the T cell in autoimmune inflammation, Arthritis Res. Ther., 7(Suppl 2) (2005), S4–S14. |
[98] | R. Antia, V. V. Ganusov and R. Ahmed, The role of models in understanding CD8+ T-cell memory, Nat. Rev. Immunol., 5 (2005), 101–111. |
1. | Davood Nourabadi, Tourandokht Baluchnejadmojarad, Seyed M. M. Zarch, Samira Ramazi, Morteza N. Serenjeh, Mehrdad Roghani, Fetal Hypothyroidism Impairs Aortic Vasorelaxation Responses in Adulthood: Involvement of Hydrogen Sulfide and Nitric Oxide Cross talk, 2021, 77, 0160-2446, 238, 10.1097/FJC.0000000000000948 | |
2. | Tamás Gáll, Dávid Pethő, Annamária Nagy, György Balla, József Balla, Therapeutic Potential of Carbon Monoxide (CO) and Hydrogen Sulfide (H2S) in Hemolytic and Hemorrhagic Vascular Disorders—Interaction between the Heme Oxygenase and H2S-Producing Systems, 2020, 22, 1422-0067, 47, 10.3390/ijms22010047 | |
3. | Zeinab Hamidizad, Mehri Kadkhodaee, Seyed Morteza Karimian, Mina Ranjbaran, Fatemeh Heidari, Enayatollah Bakhshi, Farzaneh Kianian, Elham Zahedi, Behjat Seifi, Therapeutic effects of CORM3 and NaHS in chronic kidney disease induced cognitive impairment via the interaction between carbon monoxide and hydrogen sulfide on Nrf2/HO-1 signaling pathway in rats, 2022, 368, 00092797, 110217, 10.1016/j.cbi.2022.110217 | |
4. | Luísa Gouveia Lana, Lara Matos de Araújo, Thamara Ferreira Silva, Luzia Valentina Modolo, Interplay between gasotransmitters and potassium is a K+ey factor during plant response to abiotic stress, 2021, 169, 09819428, 322, 10.1016/j.plaphy.2021.11.023 | |
5. | Yu-Bo Shi, Lin Cheng, Yue Lyu, Ze-Jing Shi, The new perspective of gasotransmitters in cancer metastasis, 2025, 10898603, 10.1016/j.niox.2025.02.002 |