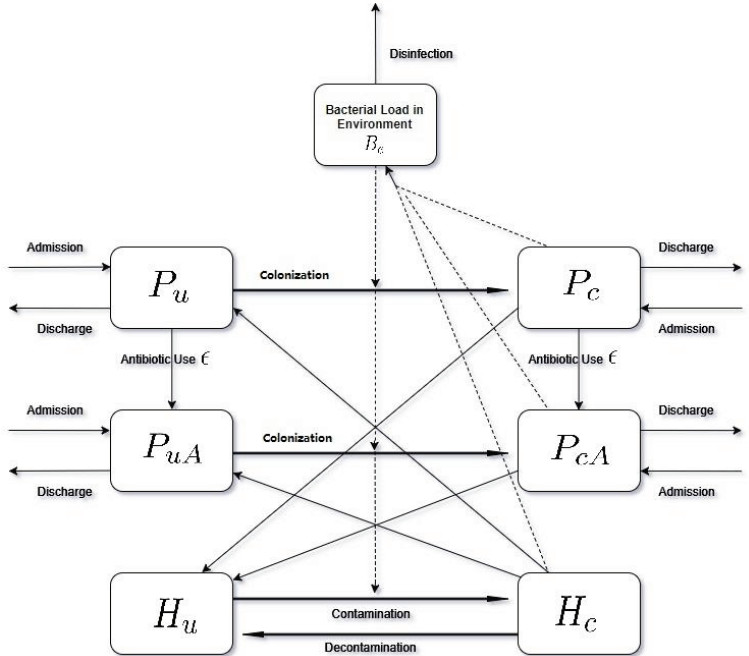
Due to their environmentally benign elemental components, suitable bandgap and high absorption coefficient in the visible-light range, Cu-based multinary sulfides exhibit excellent photocatalytic properties. Moreover, the adjustable atomic structure and unique electronic state of Cu-based multinary sulfide semiconductors can boost their ability to absorb visible light. In this review, we provide a summary of recent progress in photocatalytic applications of Cu-based multinary sulfide nanomaterials, including Cu-based ternary sulfides (CuInS2, CuIn5S8, Cu3SnS4, CuFeS2, etc.) and Cu-based quaternary sulfides (CuZnInS, Cu2ZnSnS4, CuZnGaS, CuInGaS, etc.). We start with a review of the bandgap alignments of Cu-based ternary sulfides and Cu-based quaternary sulfides, which are the key factors for the photocatalytic activity of semiconductor photocatalysts. Then, we discuss the advancements in photocatalytic applications of Cu-based multinary sulfide photocatalysts, including photocatalytic H2 production, CO2 reduction, organic synthesis and degradation of pollutants and photoelectrochemical H2 production. Finally, we end this review with a summary of the current challenges and opportunities of Cu-based multinary sulfides in future studies.
Citation: Liang Wu. Cu-based mutlinary sulfide nanomaterials for photocatalytic applications[J]. AIMS Materials Science, 2023, 10(5): 909-933. doi: 10.3934/matersci.2023049
[1] | Avner Friedman, Najat Ziyadi, Khalid Boushaba . A model of drug resistance with infection by health care workers. Mathematical Biosciences and Engineering, 2010, 7(4): 779-792. doi: 10.3934/mbe.2010.7.779 |
[2] | Hermann Mena, Lena-Maria Pfurtscheller, Jhoana P. Romero-Leiton . Random perturbations in a mathematical model of bacterial resistance: Analysis and optimal control. Mathematical Biosciences and Engineering, 2020, 17(5): 4477-4499. doi: 10.3934/mbe.2020247 |
[3] | Michele L. Joyner, Cammey C. Manning, Brandi N. Canter . Modeling the effects of introducing a new antibiotic in a hospital setting: A case study. Mathematical Biosciences and Engineering, 2012, 9(3): 601-625. doi: 10.3934/mbe.2012.9.601 |
[4] | Zongmin Yue, Yuanhua Mu, Kekui Yu . Dynamic analysis of sheep Brucellosis model with environmental infection pathways. Mathematical Biosciences and Engineering, 2023, 20(7): 11688-11712. doi: 10.3934/mbe.2023520 |
[5] | Tianfang Hou, Guijie Lan, Sanling Yuan, Tonghua Zhang . Threshold dynamics of a stochastic SIHR epidemic model of COVID-19 with general population-size dependent contact rate. Mathematical Biosciences and Engineering, 2022, 19(4): 4217-4236. doi: 10.3934/mbe.2022195 |
[6] | Robert E. Beardmore, Rafael Peña-Miller . Rotating antibiotics selects optimally against antibiotic resistance, in theory. Mathematical Biosciences and Engineering, 2010, 7(3): 527-552. doi: 10.3934/mbe.2010.7.527 |
[7] | Damilola Olabode, Libin Rong, Xueying Wang . Stochastic investigation of HIV infection and the emergence of drug resistance. Mathematical Biosciences and Engineering, 2022, 19(2): 1174-1194. doi: 10.3934/mbe.2022054 |
[8] | Xiaxia Kang, Jie Yan, Fan Huang, Ling Yang . On the mechanism of antibiotic resistance and fecal microbiota transplantation. Mathematical Biosciences and Engineering, 2019, 16(6): 7057-7084. doi: 10.3934/mbe.2019354 |
[9] | Tsanou Berge, Samuel Bowong, Jean Lubuma, Martin Luther Mann Manyombe . Modeling Ebola Virus Disease transmissions with reservoir in a complex virus life ecology. Mathematical Biosciences and Engineering, 2018, 15(1): 21-56. doi: 10.3934/mbe.2018002 |
[10] | Jing Jia, Yanfeng Zhao, Zhong Zhao, Bing Liu, Xinyu Song, Yuanxian Hui . Dynamics of a within-host drug resistance model with impulsive state feedback control. Mathematical Biosciences and Engineering, 2023, 20(2): 2219-2231. doi: 10.3934/mbe.2023103 |
Due to their environmentally benign elemental components, suitable bandgap and high absorption coefficient in the visible-light range, Cu-based multinary sulfides exhibit excellent photocatalytic properties. Moreover, the adjustable atomic structure and unique electronic state of Cu-based multinary sulfide semiconductors can boost their ability to absorb visible light. In this review, we provide a summary of recent progress in photocatalytic applications of Cu-based multinary sulfide nanomaterials, including Cu-based ternary sulfides (CuInS2, CuIn5S8, Cu3SnS4, CuFeS2, etc.) and Cu-based quaternary sulfides (CuZnInS, Cu2ZnSnS4, CuZnGaS, CuInGaS, etc.). We start with a review of the bandgap alignments of Cu-based ternary sulfides and Cu-based quaternary sulfides, which are the key factors for the photocatalytic activity of semiconductor photocatalysts. Then, we discuss the advancements in photocatalytic applications of Cu-based multinary sulfide photocatalysts, including photocatalytic H2 production, CO2 reduction, organic synthesis and degradation of pollutants and photoelectrochemical H2 production. Finally, we end this review with a summary of the current challenges and opportunities of Cu-based multinary sulfides in future studies.
Nosocomial infections caused by antibiotic-resistant bacteria are a major threat to global public health today. According to the Centers for Disease Control and Prevention (CDC) [1]:" Each year in the United States, at least 2 million people become infected with bacteria that are resistant to antibiotics, and at least 23,000 people die each year as a direct result of these infections." Furthermore, CDC identifies methicillin-resistant Staphylococcus aureus (MRSA), a Gram-positive bacterium, as one of the most common causes of hospital-acquired infections, especially in newborns and intensive-care unit patients. MRSA infections are usually treated by antibiotics, however, due to overprescribing and misprescribing, MRSA has been resistant to multiple commonly used antibiotics, which makes MRSA infections harder to be treated and even causes life-threatening cases in intensive care units.
An observation that patients with MRSA are about 64% more possible to die than patients with a non-resistant form of the infection in hospitals was revealed by a World Health Organization report in April 2014 [2]. In fact, some studies have observed a clear association between antibiotic exposure and MRSA isolation (Dancer [3], Tacconelli [4], Tacconelli et al. [5]). It has been shown that patients with prior antibiotic exposure are vulnerable to skin infections and are more likely to be colonized by MRSA. Furthermore, due to antibiotic exposure, patients may have a lower probability of successful treatment, a lengthier stay in hospitals and extra costs of treatment. Hence, it is necessary to take antibiotic exposure into account in modeling MRSA infections in hospitals. It is also found that under certain circumstances MRSA is capable of surviving for days, weeks or even months on environmental surfaces such as door handles, healthcare facilities, health-care workers' gloves (Boyce et al. [6], Dancer [7]). So environmental contamination is also a necessarily essential factor when we study the transmission of MRSA in hospitals.
In order to understand the diverse factors contributing to infections of antibiotic-resistant bacteria such as MRSA in hospitals, various models have been proposed and studied, see for example Austin and Anderson [8], Austin et al. [9], Bergstrom et al. [10], Bonhoeffer et al. [11], Bootsma et al. [12], Browne et al. [13], Browne and Webb [14], Chamchod and Ruan [15], Cooper et al. [16], D'Agata et al. [17,18], Hall et al. [19], Huang et al. [20,21], Lipsitch et al. [22], Smith et al. [23], Wang et al. [24], Wang and Ruan [25], Wang et al. [26,27], Webb [28], Webb et al. [29]. We refer to survey papers of Bonten et al. [30], Grundmann and Hellriegel [31], Temime et al. [32], van Kleef et al. [33] and the references cited therein on modeling antimicrobial resistance. These studies showed quantitatively how infection control measures such as hand washing, cohorting, and antibiotic restriction affect nosocomial cross-transmission. It is observed that the direct transmission via the hands of health-care workers (HCWs) is a crucial factor in the transmission of MRSA. In particular, D'Agata et al. [34] developed models to investigate the impact of persistent gastrointestinal colonization and antibiotic exposure on transmission dynamics of vancomycin resistant enterococci (VRE). Chamchod and Ruan [15] proposed models to investigate the effect of antibiotic exposure on the transmission of MRSA in hospitals. Mathematical models have also been developed to study the effect of environmental contamination on the spread of antibiotic-resistant bacteria in hospitals (Browne and Webb [14], McBryde and McElwain [35], Plipat et al. [36], Wang and Ruan [25], Wang et al. [26,27], Wolkewitz et al. [37]). Especially, Wang et al. [26,27] used both deterministic and stochastic models to focus on exploring the interaction between volunteers and their environment in the hospital system with a special care pattern in China. However, to the best of our knowledge, the combined effects of antibiotic exposure and environmental contamination have not been studied. This is the motivation for the current study.
In our previous studies (Wang et al. [24] and Wang and Ruan [25]) on nosocomial infections of MRSA in the emergency ward and respiratory intensive care unit in Beijing Tongren Hospital, Beijing, China, data on HCW, volunteers, patients, and environmental contamination were obtained. Wang et al. [24] constructed a mathematical model to determine the role of volunteers in the prevalence and persistence of MRSA in Beijing Tongren Hospital. Wang and Ruan [25] studied the effect of environmental contamination on the transmission MRSA in Beijing Tongren Hospital. Based on the data in [24,25], in this article, we first develop a deterministic ordinary differential equations model (ODE) to investigate the combined effects of antibiotic exposure and environmental contamination on the transmission dynamics of MRSA in hospitals. When there is no admission of colonized patients, we study the steady-states and estimate the basic reproduction number. Numerical simulations and sensitivity analysis are also provided. However, in hospital subunits, where the population is usually small, randomness may matter. Then we formulate a stochastic differential equations model (SDE) to study the transmission dynamics of MRSA that are not well described by the deterministic ODE model. Numerical simulations show that the average of multiple stochastic outputs aligns with the ODE output.
The patients, healthcare workers (HCWs), and free-living bacteria in the environment in hospitals are divided into the following seven compartments (see Figure 1):
Pu(t) – number of uncolonized patients without antibiotic exposure at time t;
PuA(t) – number of uncolonized patients with antibiotic exposure at time t;
Pc(t) – number of colonized patients without antibiotic exposure at time t;
PcA(t) – number of colonized patients with antibiotic exposure at time t;
Hu(t) – number of uncontaminated healthcare workers at time t;
Hc(t) – number of contaminated healthcare workers at time t;
Be(t) – density of the free-living bacteria in the environment at time t.
We define antibiotic exposure as having received antibiotics within one month on admission or receiving any antibiotics treatment currently in hospital [38]. We also assume that there is no cross-infection between patients, the hospital is always fully occupied, and the bacteria in the environment are uniformly distributed. Patients are in four compartments according to their status: uncolonized without or with antibiotic exposure (Pu and PuA, respectively), colonized without or with antibiotic exposure (Pc and PcA, respectively). Patients are recruited at a total rate Ω(t) from any of these four compartments with the corresponding fractions θu, θuA, θc, and θcA, respectively, and can be discharged from any of these four compartments at corresponding rates γu, γuA, γc, and γcA, respectively. We calculate the discharge rate as the reciprocal of the length of stay specific for each compartment.
Based on the assumption that the hospital is always fully occupied, it is reasonable to see that the inflow of patients is equivalent to the outflow of patients, that is Ω(t)=γuPu+γcPc+γuAPuA+γcAPcA, which results in a constant population size of patients Np=Pu+PuA+Pc+PcA. Note that the total number of HCWs is also a constant Nh=Hu+Hc. Besides, patients without antibiotic exposure would move to patients with antibiotic exposure at an antibiotic prescribing rate of ϵ per day [39]. Since we assume that there is no cross-infection between patients, uncolonized patients without antibiotic exposure Pu can be colonized either by direct contacting contaminated HCWs, αpβp(1−η)PuHc, or indirect transmission via free-living bacteria in the environment, κpPuBe. A similar process occurs as uncolonized patients with antibiotic exposure move to colonized patients with antibiotic exposure, αpβpA(1−η)PuAHc+κpAPuABe. αp is the contact rate per day per person, βp (βpA) is the probability of colonization after a contact with contaminated healthcare worker for Pu (PuA), η is the compliance rate with the hand hygiene, and κp (κpA) is the colonization rate from the environment for Pu(PuA). Meanwhile, HCWs move from uncontaminated state to contaminated state either by contacting colonized patients (without or with antibiotic exposure), αpβh(1−η)PcHu+αpβhA(1−η)PcAHu, or by the indirect transmission via free-living bacteria in the contaminated environment, κhHuBe, where κh is the contamination rate from the environment for HCWs. Because of hygiene standard of HCWs in hospitals, contaminated HCWs can move to uncontaminated HCWs, μcHc, in which μc is the decontamination rate for the HCWs. Even though free-living bacteria in the environment can survive for months, they cannot reproduce themselves in hospitals due to lack of enough reproduction conditions. Hence shedding bacteria into the environment from colonized patients, υpPc+υpAPcA, serves as an important source of transmission. As well contaminated HCWs contaminate the environment wherever they touch at the rate of υhHc. Here υp, υpA, and υh are the corresponding contaminated rates to the environment from Pc, PcA, and Hc. γb is the cleaning/disinfection rate of the environment.
Due to antibiotic exposure, patients may be more likely to have thrush, skin rashes, and gastrointestinal symptoms, and they have a higher probability of colonization, a lower probability of successful treatment, a lengthier stay, and a larger contamination rate to HCWs and the environment. We therefore assume that βpA≥βp, it was estimated that uncolonized patients with antibiotic exposure PuA is 1.67 times more likely to be colonized than uncolonized patients without antibiotic exposure Pu [39,15]. We also assume that βhA≥βh (a higher contamination rate to HCWs), γcA≤γc≤γuA≤γu (a lengthier stay or a lower discharge rate), υpA≥υp (a higher contamination (shedding) rate to the environment). Besides, of new admission, the fraction of patients having antibiotic exposure (θuA+θcA) is assumed to be 0.38 [40,15]. As this article is a continuation of our previous studies on modeling the effect of antibiotic exposure [15] and impact of environmental contamination in Beijing Tongren Hospitals [24,25], we adapt most parameter values from these papers.
Detailed parameter values are listed in Table 1. From the flowchart shown in Figure 1, we formulate an ordinary differential equations model describing the transition between compartments as follows:
Symbol | Description | Value | Reference |
ϵ0 | Antibiotic prescription rate (day−1) | 0.12 | [39][41] |
ϵ1 | Magnitude of change of antibiotic prescription rate (no dimension) | 0.25 | [56] |
θu | Proportion of Pu on admission (day−1) | 0.617 | [15][39] |
θuA | Proportion of PuA on admission (day−1) | 0.349 | [15][40] |
θc | Proportion of Pc on admission (day−1) | 0.003 | [15][39] |
θcA | Proportion of PcA on admission (day−1) | 0.031 | [15][40] |
γu | Discharge rate of Pu (day−1) | 0.2 | [15] |
γuA | Discharge rate of PuA (day−1) | 0.2 | [15] |
γc | Discharge rate of Pc (day−1) | 0.06 | [39] |
γcA | Discharge rate of PcA (day−1) | 0.055 | [15][39] |
γb | Disinfection (cleaning) rate of environment (day−1) | 0.7 | [25] |
αp | Contact rate (day−1 person−1) | 0.0435 | [25] |
βp | Probability of colonization for Pu after a contact with Hc (no dimension) | 0.42 | [25] |
βpA | Probability of colonization for PuA after a contact with Hc (no dimension) | 0.42*1.67 | [39][15] |
βh | Probability of contamination for HCW after a contact with Pc (no dimension) | 0.2 | [25][15] |
βhA | Probability of contamination for HCW after a contact with PcA (no dimension) | 0.25 | [15] |
η | Hand hygiene compliance with HCWs (no dimension) | 0.4 | [25] |
μc | Decontamination rate of HCWs (day−1) | 24 | [25] |
υp | Shedding rate to environment from Pc (day−1 person−1 ACC/cm2) | 235 | [25] |
υpA | Shedding rate to environment from PcA (day−1 person−1 ACC/cm2) | 470 | [26][39] |
υh | Contamination rate to environment by Hc (day−1 person−1 ACC/cm2) | 235 | [25] |
κp | Colonization rate from environment for Pu (day−1 (ACC/cm2)−1) | 0.000004 | [25] |
κpA | Colonization rate from environment for PuA (day−1 (ACC/cm2)−1) | 0.000005 | [15][25] |
κh | Colonization rate from environment for Hu (day−1 (ACC/cm2)−1) | 0.00001 | [25] |
Np | Total number of patients | 23 | [25] |
Nh | Total number of HCWs | 23 | [25] |
dPudt=θu(γuPu+γcPc+γuAPuA+γcAPcA)−αpβp(1−η)PuHc−κpPuBe−γuPu−ϵPu,dPcdt=θc(γuPu+γcPc+γuAPuA+γcAPcA)+αpβp(1−η)PuHc+κpPuBe−γcPc−ϵPc,dPuAdt=θuA(γuPu+γcPc+γuAPuA+γcAPcA)−αpβpA(1−η)PuAHc−κpAPuABe−γuAPuA+ϵPu,dPcAdt=θcA(γuPu+γcPc+γuAPuA+γcAPcA)+αpβpA(1−η)PuAHc+κpAPuABe−γcAPcA+ϵPc,dHudt=−αpβh(1−η)PcHu−αpβhA(1−η)PcAHu−κhHuBe+μcHc,dHcdt=αpβh(1−η)PcHu+αpβhA(1−η)PcAHu+κhHuBe−μcHc,dBedt=υpPc+υpAPcA+υhHc−γbBe | (2.1) |
with initial conditions Pu(0)=P0u, PuA(0)=P0uA, Pc(0)=P0c, PcA(0)=P0cA, Hu(0)=H0u, Hc(0)=H0c, Be(0)=B0e specified at time 0.
We know that one disadvantage of deterministic models is that they cannot directly reflect randomness in epidemic events. For nosocomial models in hospital subunits where randomness may matter, there is a need to formulate randomness more precisely. The natural extensions of a deterministic ordinary differential equations model are usually two types of stochastic setting, continuous-time Markov chains (CTMCs) and stochastic differential equations (SDEs), where the time variable is continuous, but the state variables are discrete or continuous, respectively. In the formulation of CTMCs, forward Kolmogorov differential equations for the transition probability density functions can be derived and they, in turn, lead directly to the SDEs. Even though it is difficult to find analytical solutions for multivariate processes, SDEs are useful to numerically simulate stochastic realizations (sample paths) of the process. It is believed that the SDEs are easier to solve numerically than the Kolmogorov differential equations and faster than simulating sample paths of the CTMCs model for multivariate processes [42]. Thus, we develop a CTMCs model, an SDE model and its simulations in the following ([43,25]).
By the assumption Pu+PuA+Pc+PcA=Np,Hu+Hc=Nh,∀t≥0, we have the process (Pc,PuA,PcA,Hc,Be) in R5 with Pu(t)=Np−PuA−Pc−PcA and Hu(t)=Nh−Hc. These five variables have a joint probability denoted by
p(s,j,k,m,n)(t)=Pr(Pc(t)=s,PuA(t)=j,PcA(t)=k,Hc(t)=m,Be(t)=n) |
with s≥0,j≥0,k≥0,0≤s+j+k≤Np,0≤m≤Nh and n≥0. Assume that △t>0 is sufficiently small, the transition probabilities associated with the stochastic process are defined for a small period of time △t>0 as follows:
p(s+i1,j+i2,k+i3,m+i4,n+i5);(s,j,k,m,n)(△t)=Pr[(Pc(t+△t),PuA(t+△t),PcA(t+△t),Hc(t+△t),Be(t+△t))=(s+i1,j+i2,k+i3,m+i4,n+i5)|(Pc(t),PuA(t),PcA(t),Hc(t),Be(t))=(s,j,k,m,n)], |
where i1,i2,i3,i4,i5∈{−1,0,1}, Hence the transition probability is as follow:
p(s+i1,j+i2,k+i3,m+i4,n+i5);(s,j,k,m,n)(△t)={{θc(γu(Np−s−j−k)+γcs+γuAj+γcAk)+αpβp(1−η)(Np−s−j−k)m+κp(Np−s−j−k)n}△t(i1,i2,i3,i4,i5)=(1,0,0,0,0)γcs△t(i1,i2,i3,i4,i5)=(−1,0,0,0,0)ϵs△t(i1,i2,i3,i4,i5)=(−1,0,1,0,0){θuA(γu(Np−s−j−k)+γcs+γuAj+γcAk)+ϵ(Np−s−j−k)}△t(i1,i2,i3,i4,i5)=(0,1,0,0,0)(κpAjn+γuAj)△t(i1,i2,i3,i4,i5)=(0,−1,0,0,0)αpβpA(1−η)jm△t(i1,i2,i3,i4,i5)=(0,−1,1,0,0){θcA(γu(Np−s−j−k)+γcs+γuAj+γcAk)+κpjn}△t(i1,i2,i3,i4,i5)=(0,0,1,0,0)γcAk△t(i1,i2,i3,i4,i5)=(0,0,−1,0,0){αpβh(1−η)s(Nh−m)+αpβhA(1−η)k(Nh−m)+κh(Nh−m)n}△t(i1,i2,i3,i4,i5)=(0,0,0,1,0)μcm△t(i1,i2,i3,i4,i5)=(0,0,0,−1,0)(υps+υpAk+υhm)△t(i1,i2,i3,i4,i5)=(0,0,0,0,1)γbn△t(i1,i2,i3,i4,i5)=(0,0,0,0,−1)0otherwise. | (2.2) |
We must choose the time step △t sufficiently small. In our case it is too complicated to express the transition matrix. Instead, we still are able to express the probabilities p(s,j,k,m,n)(t+△t) by using the Markov property:
p(s,j,k,m,n)(t+△t)=p(s−1,j,k,m,n)(t)[θc(γu(Np−s+1−j−k)+γcs+γuAj+γcAk)+αpβp(1−η)(Np−s+1−j−k)m+κp(Np−s+1−j−k)n]△t+p(s+1,j,k,m,n)(t)γc(s+1)△t+p(s+1,j,k−1,m,n)(t)ϵ(s+1)△t+p(s,j−1,k,m,n)(t)[θuA(γu(Np−s−j+1−k)+γcs+γuAj+γcAk)+ϵ(Np−s−j+1−k))]△t+p(s,j+1,k,m,n)(t)[κpA(j+1)n+γuA(j+1)]△t+p(s,j+1,k−1,m,n)(t)αpβpA(1−η)(j+1)m+p(s,j,k−1,m,n)(t)[θc(γu(Np−s−j−k+1)+γcs+γuAj+γcA(k+1))+κpjn]△t+p(s,j,k+1,m,n)(t)γcA(k+1)△t+p(s,j,k,m−1,n)(t)[αpβh(1−η)s(Nh−m+1)+αpβhA(1−η)k(Nh−m+1)+κh(Nh−m+1)n]△t+p(s,j,k,m+1,n)(t)μc(m+1)+p(s,j,k,m,n−1)(t)(υps+υpAk+υhm)△t+p(s,j,k,m,n+1)(t)γb(n+1)△t+∘(△t). |
Naturally, a system of forward Kolmogorov differential equations can be derived:
dps,j,k,m,ndt=p(s−1,j,k,m,n)[θc(γu(Np−s+1−j−k)+γcs+γuAj+γcAk)+αpβp(1−η)(Np−s+1−j−k)m+κp(Np−s+1−j−k)n]+p(s+1,j,k,m,n)(t)γc(s+1)+p(s+1,j,k−1,m,n)(t)ϵ(s+1)+p(s,j−1,k,m,n)(t)[θuA(γu(Np−s−j+1−k)+γcs+γuAj+γcAk)+ϵ(Np−s−j+1−k))]+p(s,j+1,k,m,n)(t)[κpA(j+1)n+γuA(j+1)]△t+p(s,j+1,k−1,m,n)(t)αpβpA(1−η)(j+1)m+p(s,j,k−1,m,n)(t)[θc(γu(Np−s−j−k+1)+γcs+γuAj+γcA(k+1))+κpjn]+p(s,j,k+1,m,n)(t)γcA(k+1)+p(s,j,k,m−1,n)(t)[αpβh(1−η)s(Nh−m+1)+αpβhA(1−η)k(Nh−m+1)+κh(Nh−m+1)n]+p(s,j,k,m+1,n)(t)μc(m+1)+p(s,j,k,m,n−1)(t)(υps+υpAk+υhm)+p(s,j,k,m,n+1)(t)γb(n+1). |
We now try to develop a stochastic differential equations model (SDE) from the deterministic epidemic model (2.1). The system has five variables with a joint probability defined by:
p(s,j,k,m,n)(t)=Pr{Pc(t)=s,PuA(t)=j,PcA(t)=k,Hc(t)=m,Be(t)=n} |
with s,j,k=0,...,Np,m=0...Nh, and n≥0, with transition probabilities given in (2.2). Let X(t)=(Pc(t),PuA(t),PcA(t),Hc(t),Be(t))T with infinitesimal
△X(t)=(△Pc(t),△PuA(t),△PcA(t),△Hc(t),△Be(t))T. |
We express the infinitesimal mean matrix f(X(t),t) as follows:
E(△X(t)|X(t)=(eceuAecAeheb)△t=f(X(t),t)△t, |
where
ec=θc(γu(Nh−Pc−PuA−PcA)+γcPc+γuAPuA+γcAPcA)+αpβp(1−η)(Nh−Pc−PuA−PcA)Hc+κpPuBe−γcPc−ϵPc,
euA=θuA(γu(Nh−Pc−PuA−PcA)+γcPc+γuAPuA+γcAPcA)−αpβpA(1−η)PuAHc−κpAPuABe−γuAPuA+ϵ(Nh−Pc−PuA−PcA),
ecA=θcA(γu(Nh−Pc−PuA−PcA)+γcPc+γuAPuA+γcAPcA)+αpβpA(1−η)PuAHc+κpAPuABe−γcAPcA+ϵPc,
eh=αpβh(1−η)Pc(Nh−Hc)+αpβhA(1−η)PcA(Nh−Hc)+κh(Nh−Hc)Be−μcHc,
eb=υpPc+υpAPcA+υhHc−γbBe.
and also the infinitesimal variance matrix Σ(X(t)t):
E(△X(t)(△X(t))T|X(t))=(δc0−ϵPc000δuA−αpβpA(1−η)PuAHc00−ϵPc−αpβpA(1−η)PuAHcδcA00000δh00000δb)△t=Σ(X(t),t)△t, |
where
δc=θc(γu(Nh−Pc−PuA−PcA)+γcPc+γuAPuA+γcAPcA)+αpβp(1−η)(Nh−Pc−PuA−PcA)Hc+κpPuBe+γcPc+ϵPc,
δuA=θuA(γu(Nh−Pc−PuA−PcA)+γcPc+γuAPuA+γcAPcA)+αpβpA(1−η)PuAHc+κpAPuABe+γuAPuA+ϵ(Nh−Pc−PuA−PcA),
δcA=θcA(γu(Nh−Pc−PuA−PcA)+γcPc+γuAPuA+γcAPcA)+αpβpA(1−η)PuAHc+κpAPuABe+γcAPcA+ϵPc,
δh=αpβh(1−η)Pc(Nh−Hc)+αpβhA(1−η)PcA(Nh−Hc)+κh(Nh−Hc)Be+μcHc,δb=υpPc+υpAPcA+υhHc+γbBe.
It is easy to check that δc,δuA,δcA,δh,δb are all nonnegative. Hence we have a matrix G satisfying GGT=Σ, where G is a 5× matrix to order △t,
G=(√a1−√a2−√a3000000000000√a4−√a5−√a600000000√a300√a6√a7−√a8000000000000√a9−√a10000000000000√a11−√a12), |
where
a1=θc(γu(Nh−Pc−PuA−PcA)+γcPc+γuAPuA+γcAPcA)+αpβp(1−η)(Nh−Pc−PuA−PcA)Hc+κpPuBe,
a2=γcPc,
a3=ϵPc,
a4=θuA(γu(Nh−Pc−PuA−PcA)+γcPc+γuAPuA)+ϵ(Nh−Pc−PuA−PcA),
a5=κpAPuABe+γuAPuA,
a6=αpβpA(1−η)PuAHc,
a7=θcA(γu(Nh−Pc−PuA−PcA)+γcPc+γuAPuA+γcAPcA)+κpAPuABe,
a8=γcAPcA,
a9=αpβh(1−η)Pc(Nh−Hc)+αpβhA(1−η)PcA(Nh−Hc)+κh(Nh−Hc)Be,
a10=μcHc,
a11=υpPc+υpAPcA+υhHc,
a12=γbBe.
Then the stochastic differential equations have the following form:
dX(t)=f(X(t),t)dt+G(X(t),t)dW(t). |
More precisely,
{dPc(t)=ecdt+√a1dW1−√a2dW2−√a3dW3,dPuA(t)=euAdt+√a4dW4−√a5dW5−√a6dW6,dPcA(t)=ecAdt+√a3dW3+√a6dW6+√a7dW7−√a8dW8,dHct=ehdt+√a9dW9−√a10dW10,dBe(t)=ebdt+√a11dW11−√a12dW12. | (2.3) |
where W1,⋯,W12 are twelve independent Wiener processes. Next we are able to run stochastic simulations.
In this section we provide detailed analysis of the deterministic ODE model (2.1).
Based on the biological background of model (2.1), we only consider solutions of model (2.1) starting at t=0 with nonnegative initial values:
P0u≥0,P0uA≥0,P0c≥0,P0cA≥0,H0u≥0,H0c≥0,B0e≥0. |
Lemma 1. If P0u,P0uA,P0c,P0cA,H0u,H0c,B0e≥0, then (Pu(t),PuA(t),Pc(t),PcA(t),Hu(t),Hc(t),Be(t)) the solutions of model (2.1) are nonnegative for all t≥0 and ultimately bounded. In particular, if P0u,P0uA,P0c,P0cA,H0u,H0c,B0e>0, then the solutions (Pu(t),PuA(t),Pc(t),PcA(t),Hu(t),Hc(t),Be(t)) are also positive for all t≥0.
Proof. Firstly, by the continuous dependence of solutions with respect to initial values, we only need to prove that when P0u,P0uA,P0c,P0cA,H0u,H0c,B0e>0, (Pu(t),PuA(t),Pc(t),PcA(t),Hu(t),Hc(t),Be(t)) the solutions are also positive for all t≥0. That is, the solutions remain in the positive cone if the initial conditions are in the positive cone of R7. Set
m(t)=min{Pu(t),PuA(t),Pc(t),PcA(t),Hu(t),Hc(t),Be(t)},∀t>0. |
Clearly, m(0)>0. Assuming that there exists a t1>0 such that m(t1)=0 and m(t)>0 for all t∈[ 0,t1).
If m(t1)=Pu(t1), from the first equation of model (2.1) it follows that dPudt≥−(αpβp(1−η)Hc(t)+κpBe(t)+γu+ϵ)Pu for all t∈[ 0,t1). Since Hc(t)>0,Be(t)>0 for all t∈[ 0,t1), we have
0=Pu(t1)≥P0uexp(−∫t10(αpβp(1−η)Hc(s)+κpBe(s)+γu+ϵ)ds)>0, |
which leads to a contradiction. Similar contradictions can be deduced in the cases of m(t1)=PuA(t1),m(t1)=Pc(t1),m(t1)=PcA(t1),m(t1)=Hu(t1),m(t1)=Hc(t1),m(t1)=Be(t1). Hence, the solutions remain in the positive cone if the initial conditions are in the positive cone R7.
Secondly, let T(t)=Pu(t)+PuA(t)+Pc(t)+PcA(t)+Hu(t)+Hc(t)+Be(t). Then
dT(t)dt=dBe(t)dt=υpPc+υpAPcA+υhHc−γbBe≤υpNp+υpANp+υhNh−γbBe(t), |
where Np=Pu(t)+PuA(t)+Pc(t)+PcA(t) and Nh=Hu(t)+Hc(t), which implies that
Be(t)≤(υpNp+υpANp+υhNh)γb(1−e−γbt)+B0ee−γbt. |
So Be(t) is bounded by a fixed number
M=(υpNp+υpANp+υhNh)γb+B0e. |
Let N=Np+Nh+M, we have
T(t)=Pu(t)+PuA(t)+Pc(t)+PcA(t)+Hu(t)+Hc(t)+Be(t)≤N. |
Thus, the solutions remain bounded in a positive cone of R7, and the system induces a global semiflow in the positively invariant set of R7. This completes the proof.
Remark 2. Denote set G as follows
G:={(Pu,PuA,Pc,PcA,Hu,Hc,Be)∈R7+:Pu+PuA+Pc+PcA+Hu+Hc+Be≤N)}. |
Then Lemma 1 implies that G is a positively invariant set with respect to model (2.1).
When θc = 0, θcA = 0, that is, there are no colonized patients admitted into hospitals, model (2.1) has a unique infection-free equilibrium (IFE) which is defined by
E0=(Pu,Pc,PuA,PcA,Hu,Hc,Be)=(N∗,0,Np−N∗,0,Nh,0,0),N∗=θuγuANpθuAγu+θuγuA+ϵ. |
We derive the basic reproduction number R0 for model (2.1) by using the techniques in Diekmann et al. [44] and van den Driessche and Watmough [45], which involves linearizing the original nonlinear ordinary differential equations at the infection-free equilibrium. Re-order the components of E0 as
E0=(Pc,PcA,Hc,Be,Pu,PuA,Hu)=(0,0,0,0,N∗,Np−N∗,Nh) |
and set
F=(αpβp(1−η)PuHc+κpPuBeαpβpA(1−η)PuAHc+κpAPuABeαpβh(1−η)PcHu+αpβhA(1−η)PcAHu+κhHuBe0000), |
V=(γcPc+ϵPc−θcΩγcAPcA−[ϵPc+θcAΩ]μcHcγbBe−(υpPc+υpAPcA+υhHc)αpβp(1−η)PuHc+κpPuBe+γuPu+ϵPu−θuΩαpβpA(1−η)PuAHc+κpAPuABe+γuAPuA−[ϵPu+θuAΩ]αpβh(1−η)PcHu+αpβhA(1−η)PcAHu+κhHuBe−μcHc), |
where Ω=(γuPu+γcPc+γuAPuA+γcAPcA),
V−=(γcPc+ϵPcγcAPcAμcHcγbBeαpβp(1−η)PuHc+κpPuBe+γuPu+ϵPuαpβpA(1−η)PuAHc+κpAPuABe+γuAPuAαpβh(1−η)PcHu+αpβhA(1−η)PcAHu+κhHuBe),V+=(θcΩϵPc+θcAΩ0υpPc+υpAPcA+υhHcθuΩϵPu+θuAΩμcHc). |
Since θc = 0, θcA = 0, then we can derive that
F=(00αpβp(1−η)N∗κpN∗00αpβpA(1−η)(Np−N∗)κpA(Np−N∗)αpβh(1−η)NhαpβhA(1−η)Nh0κhNh0000), |
V=(γc+ϵ000−ϵγcA0000μc0−υp−υpA−υhγb). |
Therefore, let ω1=υpγcA+υpAϵγbγcA(γc+ϵ), ω2=υpAγbγcA, ω3=υhγbμc, we have
FV−1=(ω1κpN∗ω2κpN∗ω3κpN∗+αpβp(1−η)N∗μcκpN∗γbω1κpA(Np−N∗)ω2κpA(Np−N∗)ω3κpA(Np−N∗)+αpβpA(1−η)(Np−N∗)μcκpA(Np−N∗)γbω1κhNh+βhγcA+βhAϵγcA(γc+ϵ)αp(1−η)Nhω2κhNh+αpβhA(1−η)NhγcAω3κhNhκhNhγb0000) |
and the basic reproductive number is defined as the spectral radius of FV−1:
R0=sp(FV−1)=α3α1+α1+α2 | (3.1) |
where
α1=(√(α3627μ3cγ3cA+α5+α4)2−α33+α3627μ3cγ3cA+α5+α4)13,α2=α63μcγcA, α3=α73μcγcA+α269μ2cγ2cA, α4=α6α76μ2cγ2cA,α5=(βpκpA−βpAκp)N∗(Np−N∗)Nhα2p(1−η)2[ ω1βhA(γc+ϵ)−ω2(βhγcA+βhAϵ)] 2μcγcA(γc+ϵ),α6=μcγcA(ω1κpN∗+ω2κpA(Np−N∗)+ω3κhNh),α7=Nhα2p(1−η)2[βhAβpA(Np−N∗)+βhγcA+βhAϵγc+ϵβpN∗]+(Np−N∗)Nhαp(1−η)[ω2κhβpAγcA+ω3κpAβhAμc]+N∗Nhαp(1−η)[ω1βpκhγcA+ω3κpμcβhγcA+βhAϵγc+ϵ]. |
By Theorem 2 in van den Driessche and Watmough [45], we have the following theorem:
Theorem 3. If R0<1, then the infection-free equilibrium E0 is locally asymptotically stable; If R0>1, then E0 is unstable.
Moreover, from the proof of Theorem 2 in van den Driesshce and Watmough [45] or the proof of Lemma 2.1 in Wang and Zhao [46], we have the following observation: Denote
J1=F−V=(−γc−ϵ0αpβp(1−η)N∗κpN∗ϵ−γcAαpβpA(1−η)(Np−N∗)κpA(Np−N∗)αpβh(1−η)NhαpβhA(1−η)Nh−μcκhNhυpυpAυh−γb). |
Let s(J1) be the maximum real part of the eigenvalues of J1. Since J1 is irreducible and has non-negative off-diagonal elements, s(J1) is a simple eigenvalue of J1 with a positive eigenvector. Then we have the following corollary:
Corollary 4. There hold two equivalences:
R0<1⟺s(J1)<0;R0>1⟺s(J1)>0. |
The existence and stability of the infection-free equilibrium E0 indicates that the MRSA infection is vanishing.
Theorem 5. If R0<1, then the infection-free equilibrium E0 is globally asymptotically stable.
Proof. From Theorem 3 we know that E0 is locally asymptotically stable. Now we prove the global attractivity of the infection-free equilibrium E0.
By the first equation of model (2.1), non-negativity of the solutions and previous assumptions, we get
dPudt≤θu[γuPu+γuA(Np−Pu)]−γuPu−ϵPu. |
Since γuA=max{γuA,γc,γcA}, it implies that
dPudt≤θuγuANp−(−θuγu+θuγuA+γu+ϵ)Pu=θuγuANp−(θuAγu+θuγuA+ϵ)Pu. |
So ∀δ>0, there exists t1>0, such that Pu≤N∗+δ, for all t≥t1.
Similarly, by the third equation of model (2.1), non-negativity of the solutions and previous assumptions, we get
dPuAdt≤(1−θu)[γu(Np−PuA)+γuAPuA]−γuAPuA+ϵ(Np−PuA), |
that is,
dPuAdt≤(θuAγu+ϵ)Np−(θuAγu+θuγuA+ϵ)PuA. |
Then ∀δ>0, there exists t2>0, such that PuA≤Np−N∗+δ, for all t≥t2.
Let T=max{t1,t2}. If t>T, since θc=θcA=0, then
{P′c(t)≤αpβp(1−η)(N∗+δ)Hc+κp(N∗+δ)Be−γcPc−ϵPc,P′cA(t)≤αpβpA(Np−N∗+δ)Hc+κpA(Np−N∗+δ)Be−γcAPcA+ϵPc,H′c(t)≤αpβh(1−η)NhPc+αpβhA(1−η)NhPcA+κhNhBe−μcHc,B′e(t)≤υpPc+υpAPcA+υhHc−γbBe. | (3.2) |
Considering the following auxiliary system:
{~P′c(t)=αpβp(1−η)(N∗+δ)˜Hc+κp(N∗+δ)˜Be−γc˜Pc−ϵ˜Pc,~P′cA(t)=αpβpA(Np−N∗+δ)˜Hc+κpA(Np−N∗+δ)˜Be−γcA˜PcA+ϵ˜Pc,~H′c(t)=αpβh(1−η)Nh˜Pc+αpβhA(1−η)Nh˜PcA+κhNh˜Be−μc˜Hc,~B′e(t)=υp˜Pc+υpA˜PcA+υh˜Hc−γb˜Be. | (3.3) |
Define
J1(δ)=(−γc−ϵ0αpβp(1−η)(N∗+δ)κp(N∗+δ)ϵ−γcAαpβpA(1−η)(Np−N∗+δ)κpA(Np−N∗+δ)αpβh(1−η)NhαpβhA(1−η)Nh−μcκhNhυpυpAυh−γb). |
It follows from Corollary 4 that if R0<1, then s(J1(0))<0. Since s(J1(δ)) is continuous for small δ, so there exists δ small enough such that s(J1(δ))<0. Thus there is a negative eigenvalue of s(J1(δ)) with a positive eigenvector. Obviously if t→∞, then ~Pc,~PcA,~Hc,~Be→0. Then by the comparison principle we get
limt→∞Pc=0,limt→∞PcA=0,limt→∞Hc=0,limt→∞Be=0. |
Therefore, E0 is globally attractive when R0<1. This completes the proof.
Uniform persistence of system (2.1) demonstrates that all components of the dynamical model have positive lower bounds which in turn indicates that MRSA infection persists in the hospital.
Theorem 6. If R0>1, then model (2.1) is uniformly persistent.
Proof. We first define
X={(Pu,Pc,PuA,PcA,Hu,Hc,Be):Pu≥0,Pc≥0,PuA≥0,PcA≥0,Hu≥0,Hc≥0,Be≥0}, |
X0={(Pu,Pc,PuA,PcA,Hu,Hc,Be)∈X:Pc>0,PcA>0,Hc>0,Be>0},∂X0=X∖X0. |
It can be seen that both X and X0 are positively invariant with respect to model (2.1). Clearly, ∂X0 is relatively closed in X. Lemma 1 implies that model (2.1) is point dissipative, which implies that the solutions of model (2.1) admit a global attractor. Then we define
M∂={(Pu(0),Pc(0),PuA(0),PcA(0),Hu(0),Hc(0),Be(0)):(Pu(t),Pc(t),PuA(t),PcA(t),Hu(t),Hc(t),Be(t))∈∂X0,∀t≥0}. |
Now we prove that
M∂={(Pu,0,PuA,0,Hu,0,0):Pu≥0,PuA≥0,Hu=Nh}. |
For any point φ0=(Pu(0),Pc(0),PuA(0),PcA(0),Hu(0),Hc(0),Be(0)) in M∂, we suppose that one of Pc(0),PcA(0),Hc(0),Be(0) is not zero, that is to say, φ0∉{(Pu,0,PuA,0,Hu,0,0):Pu≥0,PuA≥0,Hu=Nh}. Without loss of generality, we suppose that Pc(0)=0,PcA(0)=0,Hc(0)=0,Be(0)>0. By the second, fourth, and sixth equations, we have
dPc(0)dt≥κpPu(0)Be(0)>0;dPcA(0)dt≥κpAPuA(0)Be(0)>0;dHc(0)dt≥κhHu(0)Be(0)>0. |
Thus, there exists δ0>0, if 0<t<δ0 then Pc(t)>0,PcA(t)>0,Hc(t)>0,Be(t)>0, which imply that φ0∉∂X0. we will get the similar result for other cases (Pc(0)>0, or PcA(0)>0, or Hc(0)>0). Thus φ0∉M∂. This gives us a contradiction. Hence φ0∈{(Pu,0,PuA,0,Hu,0,0):Pu≥0,PuA≥0,Hu=Nh}. So M∂⊆{(Pu,0,PuA,0,Hu,0,0):Pu≥0,PuA≥0,Hu=Nh}. Obviously we have {(Pu,0,PuA,0,Hu,0,0):Pu≥0,PuA≥0,Hu=Nh}⊆M∂, therefore, M∂={(Pu,0,PuA,0,Hu,0,0):Pu≥0,PuA≥0,Hu=Nh}. Let φ0 be an initial value. Clearly there is only one equilibrium E0=(N∗,0,Np−N∗,0,Nh,0,0) in M∂, so ∪φ0∈M∂ω(φ0)=E0. Therefore, {E0} is a compact and isolated invariant set in ∂X0.
Next we claim that there exists a positive constant ℓ such that for any solution of model (2.1), Ψt(φ0),φ0∈X0, we have
lim supt→∞d(Ψt(φ0),E0)≥ℓ, |
where d is a distant function in X0. We construct by contradiction so that we suppose the claim is not true. Then lim supt→∞d(Ψt(φ0),E0)≤ℓ for any ℓ>0, namely, there exists a positive constant T, such that N∗−ℓ≤Pu(t)≤N∗+ℓ, Pc(t)≤ℓ, Np−N∗−ℓ≤PuA(t)≤Np−N∗+ℓ, PcA(t)≤ℓ, Nh−ℓ≤Hu(t)≤Nh+ℓ, Hc(t)≤ℓ, Be(t)≤ℓ, for any t>T. While t>T, we have,
{P′c(t)≥αpβp(1−η)(N∗−ℓ)Hc+κp(N∗−ℓ)Be−γcPc−ϵPc,P′cA(t)≥αpβpA(Np−N∗−ℓ)Hc+κpA(Np−N∗−ℓ)Be−γcAPcA+ϵPc,H′c(t)≥αpβh(1−η)(Nh−ℓ)Pc+αpβhA(1−η)(Nh−ℓ)PcA+κh(Nh−ℓ)Be−μcHc,B′e(t)≥υpPc+υpAPcA+υhHc−γbBe. | (3.4) |
Consider the following auxiliary system:
{~P′c(t)=αpβp(1−η)(N∗−ℓ)~Hc+κp(N∗−ℓ)~Be−γc~Pc−ϵ~Pc,~P′cA(t)=αpβpA(Np−N∗−ℓ)~Hc+κpA(Np−N∗−ℓ)~Be−γcA~PcA+ϵ~Pc,~H′c(t)=αpβh(1−η)(Nh−ℓ)~Pc+αpβhA(1−η)(Nh−ℓ)~PcA+κh(Nh−ℓ)~Be−μc~Hc,~B′e(t)=υp~Pc+υpA~PcA+υh~Hc−γb~Be. | (3.5) |
we define
J1(ℓ)=(−γc−ϵ0αpβp(1−η)(N∗−ℓ)κp(N∗−ℓ)ϵ−γcAαpβpA(1−η)(Np−N∗−ℓ)κpA(Np−N∗−ℓ)αpβh(1−η)(Nh−ℓ)αpβhA(1−η)(Nh−ℓ)−μcκh(Nh−ℓ)υpυpAυh−γb). |
For R0>1, by Corollary 4, we have s(J1(0))>0. Since s(J1(ℓ)) is continuous for small ℓ, so there exists a positive constant ℓ small enough such that s(J1(ℓ))>0. Thus, there is a positive eigenvalue of s(J1(δ)) with a positive eigenvector. It is easy to see if t→∞, then ~Pc,~PcA,~Hc,~Be→∞. Then by the comparison principle we get
limt→∞Pc=∞,limt→∞PcA=∞,limt→∞Hc=∞,limt→∞Be=∞. |
This contradicts our assumption and completes the proof of the claim.
The claim implies that {E0} is an isolated invariant set in X and Ws(E0)∩X0=∅. Therefore, system (2.1) is uniformly persistent if R0>1 by Theorem 1.3.1 in [47]. This completes the proof.
In this section we present numerical simulations on the deterministic model and sensitivity analysis of the basic reproduction number in terms of model parameters.
Our deterministic model is simulated for 365 days. Data [24] collected in Beijing Tongren Hospital, where a total of 23 beds were in the emergency ward and were always fully occupied, are used to estimate the initial values of patients and healthcare workers. We assume an initial bacteria density being 1000 ACC/cm2 as comparable to the measurement scale obtained by Bogusz et al. [48]. With the initial values (P0u,P0uA,P0c,P0cA,H0u,H0c,B0e)=(4,6,7,6,17,6,1000) and parameter values shown in Table 1, we simulate the following outcomes: numerical solutions of the deterministic model (2.1), prevalences of colonized patients without or with antibiotic exposure, and the basic reproduction number R0. Simulations are also performed to evaluate the effect of various interventions on changing the prevalence of colonized patients and R0.
Using the baseline parameters in Table 1, Figures 2 and 3 give the behaviors of solutions to the deterministic model (2.1), which imply that 36% of patients are colonized with MRSA with antibiotic exposure, and 4% are colonized without antibiotic exposure; Figure 4 shows that, while with no admission of MRSA-positive patients (θc=θcA=0), 21% of patients are colonized with MRSA with antibiotic exposure and 3% are colonized without antibiotic exposure; while with no admission of patients with history of antibiotic exposure (θuA=θcA=0), 27% of patients are colonized with MRSA with antibiotic exposure, and 7.5% are colonized without antibiotic exposure; while with no admission of patients with history of antibiotic exposure and MRSA-positive (θuA=θc=θcA=0), 14% of patients are colonized with MRSA with antibiotic exposure, and 3.5% are colonized without antibiotic exposure. Hence, to control hospital infections, we may need to reduce the proportion of colonized patients (θc and θcA) at admission by increasing the detection and isolation of the admitted MRSA patients and also reduce the proportion of uncolonized patients with antibiotic exposure (θuA) by strengthening the public education about how to use antibiotics properly in the community.
In the case where colonized patients are admitted into hospitals, the infections will always persist. When θc=0,θcA=0, that is no colonized patients are admitted into hospital, the infection-free equilibrium (IFE) is defined to be E0=(Pu,Pc,PuA,PcA,Hu,Hc,Be)=(N∗,0,Np−N∗,0,Nh,0,0) where N∗ = θuγuANpθuAγu+θuγuA+ϵ. By parameters listed in Table 1, the basic reproduction number is estimated to be 1.2860, which means that the infections are persistent. We want to reduce R0 to below unity by some interventions. Here we perform some simulations to evaluate the effect of the following interventions in reducing the prevalence of colonized patients with or without antibiotic exposure, and R0: (1) Prescription rate of antibiotics ϵ; (2) Hand hygiene compliance of HCWS η; (3) The discharge rate for colonized patients with or without antibiotic exposure γc and γcA, respectively; (4) Environmental cleaning rate γb; and (5) Decontamination rate of HCWs μc.
The predicted effects of individual interventions on reducing the prevalence of MRSA and the reproduction number R0 are shown in Figure 5. Figure 5A shows that increasing the compliance rate of hand hygiene for HCWs, η, from 0.4 (baseline) to 1, just reduces R0 from 1.2860 to 1.2197, and reduces the prevalence of colonized patients with or without antibiotic exposure by 4.51% (from 20.56% to 16.04%) and 0.54% (from 2.45% to 1.91%), respectively. When antibiotic prescribing rate is reduced from 0.12 (baseline) to 0 (no antibiotic use), we get a result in around 19% reduction in the prevalence of colonized patients with antibiotic exposure, while a little increase and then decrease in the prevalence of colonized patients without antibiotic exposure, and a change from 1.2860 to 0.9251 in R0 in Figure 5B. We investigate the discharge rate (i.e., the reciprocal of the length of stay) of colonized patients without antibiotic exposure γc, and with antibiotic exposure γcA, respectively, in Figures 5C-5D. When the discharge rate of Pc is increased from the baseline value 0.06 to 0.2 (i.e., the length of stay of Pc is decreased from 16.6 days to 5 days), R0 reduces to 1.1308, and the prevalence of PcA and Pc reduces by 9.58% (from 21.08% to 11.50%) and 1.72% (from 2.57% to 0.85%), respectively. Especially, we notice that if we decrease the discharge rate of PcA a little bit from the baseline value 0.055, there are dramatic increases in both R0 and the prevalence of PcA. However, many studies show that colonized patients with antibiotic exposure PcA usually lead to a lengthier stay [15], which in turns makes the situation worse. Hence, the rapid and efficient treatment of colonized patients, especially those with antibiotic exposure, is key in controlling MRSA infections. Furthermore, we find that improving environmental cleaning rate γb is the most effective intervention from Figure 5E. When the environmental cleaning rate is increased from 0.7 (baseline) to 1, we are able to decrease the prevalence of PcA and Pc from 20.56% to 1.99% and from 2.45% to 0.21%, respectively, and successfully reduce R0 to below unity. Figure 5F shows that decontamination rate of HCWs has little effect.
Furthermore, Figure 6 presents a direct simulation of the stability transition at R0=1 to support our above conclusion that when R0<1 the infection-free equilibrium is globally asymptotically stable and when R0>1 the infection is uniformly persistent.
Observing that individual invention is hard to reduce R0 to below unity, we examine the effects of combined interventions (Figure 7). When we decrease antibiotic use and in the meanwhile increase the discharge rate of PcA, we reduce R0 to below unity efficiently (Figure 7(b)). Similar result occurs when combining the increased environmental cleaning rate and decreased discharge rate of PcA (Figure 7(f)).
Latin hypercube sampling (LHS) method is used to engage a sensitivity analysis [49,50]. Partial rank correlation coefficients (PRCCs) are calculated for the following nine parameters against the prevalence of colonized patients and R0 over time: discharge rate for colonized patients with antibiotic exposure γcA, environmental cleaning rate γb, probability of colonization for PuA after a contact with a contaminated HCW βpA, probability of contamination for HCW after a contact with a colonized patient with antibiotic exposure βhA, hand hygiene compliance rate η, decontamination rate of HCWs μc, shedding rate to the environment by colonized patients with antibiotic exposure υpA, antibiotic prescribing rate ϵ, contamination rate from environment for uncolonized patients with antibiotic exposure κpA. We also test for significant PRCCs for the above parameters to evaluate which parameters are essential to our model. Since we find that the PRCC values vary little after about 100 days, it is reasonable and efficient for us to just study the PRCC values on this specific day 100 (Figure 8). Figure 8(d) implies that the first four parameters have the most impact on the outcome of R0, which are the environmental cleaning rate γb, shedding rate to the environment by colonized patients with antibiotic exposure υpA, contamination rate from the environment for uncolonized patients with antibiotic exposure κpA, and antibiotic prescribing rate ϵ. From Figures 8(a)-8(c), we illustrate the PRCC values of the nine examined parameters and corresponding p-values for different outcome parameters for Pc, PcA, and Be. All simulations are done by MATLAB and input parameters are assumed to be normally distributed, due to the lack of present data concerning distribution functions, as shown in Table 2.
Symbol | Distribution | reference |
γcA | N(0.055,0.005) | estimated by [26] |
γb | N(0.7,0.2) | estimated by [26] |
βpA | N(0.43,0.1) | estimated by [26] |
βhA | N(0.2,0.05) | estimated by [26] |
η | N(0.4,0.1) | estimated by [26] |
μc | N(24,5) | estimated by [26] |
υpA | N(470,150) | estimated by [26] |
ϵ | N(0.12,0.02) | estimated by [26] |
κpA | N(0.000005,0.0000006) | estimated by [26] |
Finally we run some numerical simulations of the stochastic model. Using the baseline parameters in Table 1 and initial values (P0u,P0uA,P0c,P0cA,H0u,H0c,B0e)=(4,6,7,6,17,6,1000), we run 100 stochastic simulations of the SDE model (2.3). In Figure 9, the blue curves, red curves, and shaded regions represent the averages of 100 runs, outputs of the deterministic model (2.1), and 90% bound of 100 runs, respectively. It is shown that the average of stochastic runs is consistent with the outcome of the deterministic model; however, randomness does make a difference in a single stochastic case. Also, we study the effect of environmental cleaning rate γb and antibiotic prescribing rate ϵ on the number of colonized patients in Figures 10 and 11, respectively. Compared with Figure 9, we increase environmental cleaning rate, γb=1, in Figure 10, which shows that increasing environmental cleaning rate can reduce the average number of colonized patients in the SDE model. Similarly, compared with Figure 9, we reduce the antibiotic use to an extreme case, ϵ=0, in Figure 11, which reduces the average number of colonized patients greatly in the SDE model.
Next, we consider the case with no admission of MRSA-positive patients, i.e., θc=θcA=0, so R0 can be calculated. We have proved that when R0<1, MRSA infections will go to extinction in the deterministic model (2.1). By choosing different parameter values to make R0<1 in both Figures 12 and 13, it is shown that MRSA infections do go to extinction in the deterministic model. However, the average number of colonized patients in the SDEs model persists, even though it is small, in Figures 12 and 13. In the above simulations, the number of colonized patients was treated as a real number rather than an integer. In order to intuitively see a difference between deterministic and stochastic models for a small population, in Figure 14, we round the real number to the nearest integer. This shows that with no admission of colonized patients, there are still reinfections after the number of colonized patients drops to zero, which indicates that the free-living bacteria may persist in the environment and later be transmitted back to the patients. That is to say, the free-living bacteria in the environment may be able to cause a later outbreak even though the infections have been died out from patients. Hence, hospitals should pay attention to environmental cleaning strategies to prevent MRSA infections.
Furthermore, in a relatively large population, where we consider initial values
(P0u,P0uA,P0c,P0cA,H0u,H0c,B0e)=(34,16,17,16,17,6,1000), |
Figure 15 shows that the average of multiple stochastic runs is consistent with the deterministic outcome.
We developed a comprehensive study of MRSA infections in hospitals, which includes crucial factors such as antibiotic exposure and environmental contamination. Both deterministic and stochastic mathematical models were developed to study the transmission dynamics of MRSA infections in hospitals, including uncolonized patients without and with antibiotic exposure, colonized patients without and with antibiotic exposure, uncontaminated and contaminated health-care workers, and free-living MRSA. Under the assumption that there is no admission of the colonized patients, the basic reproduction number R0 was calculated. It was shown that when R0<1 the infection-free equilibrium is globally asymptotically stable, and when R0>1 the infection is uniformly persistent.
For the deterministic model, numerical simulations were performed to demonstrate the behavior of the solutions, the effect of several interventions on reducing the prevalence of colonized patients and the basic reproduction number, and the dependence and sensitivity of the basic reproduction number of various parameters. Until recently, control strategies focus on the direct transmission between HCWs and patients, however, our results strongly supported that the environmental cleaning is the most effective intervention. When we increased the environmental cleaning rate, we could decrease the prevalence of colonized patients greatly and successfully reduce R0 to below unity in Figure 5. Sensitively analysis in Figure 8 also showed that the environmental cleaning rate γb, shedding rate to the environment by colonized patients with antibiotic exposure υpA, contamination rate from the environment for uncolonized patients with antibiotic exposure κpA, and antibiotic prescribing rate ϵ had remarkable impacts on the number of colonized patients and R0. Even though it is difficult to quantify the environmental cleaning, we suggest that hospitals should try to use more effective cleaning products, improve monitoring strategies such as providing feedback to cleaning teams, and even use new technology (cleaning robots) to supplement the manual cleanings. Besides, it was shown that a higher discharge rate is associated with a lower prevalence of MRSA. The rapid and efficient treatment of colonized patients, especially those with antibiotic exposure, is key in controlling MRSA infections. However, the discharge rate depends on the time required for treatment and cannot be arbitrarily modified at will, which makes the control of MRSA infections challenge. In the cases of outbreaks, hospitals should try proper isolation of those colonized patients. Also, screening and isolating colonized patients at admission are important control strategies. Our study also emphasized the importance of effective antimicrobial stewardship programs in reducing antibiotic usage both in hospitals and communities.
For the stochastic model, numerical simulations were also carried out to study the behavior of the stochastic model and the effect of antibiotic prescribing rate ϵ, and environmental cleaning rate γb, on the number of colonized patients, respectively. Moreover, we chose different parameter values to make R0<1 and found that MRSA infections go to extinction in the deterministic model; however, the average number of colonized patients in multiple stochastic runs persisted in Figures 12 and 13. In order to intuitively see a difference between deterministic and stochastic models for a small population, in Figure 14, we rounded the real number to the nearest integer. This shows that the free-living bacteria in the environment may be able to cause a later outbreak even though the infections have been died out from patients. Hence, hospitals should pay attention to environmental cleaning strategies to prevent MRSA infections.
In the proposed model, the heterogeneity in infection risk among different types of wards was omitted and free-living bacteria were assumed to be uniformly distributed for the sake of simplicity; however, heterogeneity should be taken into account in the future work for a more realistic consideration [12,14]. Also, model parameters were regarded as a constant, however, it is not necessarily true in the real world. In applications, parameters in the model need to be inferred from noisy data. Recent works have developed several methods to infer parameters in models with complex and flexible structures [51,52,53], which should be potentially considered in future works. In addition, in current model the complex contact pattern was simplified to a full mixing, however, it may be useful to take into account the contact network structure in future works to represent heterogeneity in population behavior, locations and contact patterns [54,55]. In current model setting, patients were assumed to be hospitalized and under antibiotic treatments because of other diseases, then for those patients with antibiotic treatment, they were just more likely to be colonized by MRSA, and then had a lower probability of successful treatment, a lengthier stay in hospitals and extra costs of treatment. That is to say, we ignored the difference between colonized patients and infected patients since treatment should be needed for infected patients. A more comprehensive work may be done in the future [15]. Furthermore, an extension should be specifically compared with this proposed model and its conclusions and applied to actual MRSA infection data in hospitals ([25,57]).
This research was partially supported by the University of Miami Provost's Research Award (UM PRA 2019-409). We would like to thank Dr. Xi Huo and three anonymous reviewers for their helpful comments and suggestions which helped us to improve the presentation of the paper.
All authors declare no conflicts of interest in this paper.
[1] |
Parvulescu VI, Epron F, Garcia H, et al. (2021) Recent progress and prospects in catalytic water treatment. Chem Rev 122: 2981–3121. https://doi.org/10.1021/acs.chemrev.1c00527 doi: 10.1021/acs.chemrev.1c00527
![]() |
[2] |
Wang Q, Domen K (2020) Particulate photocatalysts for light-driven water splitting: Mechanisms, challenges, and design strategies. Chem Rev 120: 919–985. https://doi.org/10.1021/acs.chemrev.9b00201 doi: 10.1021/acs.chemrev.9b00201
![]() |
[3] |
Rahman MZ, Edvinsson T, Gascon J (2022) Hole utilization in solar hydrogen production. Nat Rev Chem 6: 243–258. https://doi.org/10.1038/s41570-022-00366-w doi: 10.1038/s41570-022-00366-w
![]() |
[4] |
Zhou P, Luo M, Guo S (2022) Optimizing the semiconductor-metal-single-atom interaction for photocatalytic reactivity. Nat Rev Chem 6: 823–838. https://doi.org/10.1038/s41570-022-00434-1 doi: 10.1038/s41570-022-00434-1
![]() |
[5] |
Sokol KP, Andrei V (2022) Automated synthesis and characterization techniques for solar fuel production. Nat Rev Mater 7: 251–253. https://doi.org/10.1038/s41578-022-00432-1 doi: 10.1038/s41578-022-00432-1
![]() |
[6] |
Chen S, Takata T, Domen K (2017) Particulate photocatalysts for overall water splitting. Nat Rev Mater 2: 17050. http://doi.org/10.1038/natrevmats.2017.50 doi: 10.1038/natrevmats.2017.50
![]() |
[7] |
Chang K, Hai X, Ye JH (2016) Transition metal disulfides as noble-metal-alternative co-catalysts for solar hydrogen production. Adv Energy Mater 6: 1502555. https://doi.org/10.1002/aenm.201502555 doi: 10.1002/aenm.201502555
![]() |
[8] |
Wang F, Li Q, Xu D (2017) Recent progress in semiconductor-based nanocomposite photocatalysts for solar-to-chemical energy conversion. Adv Energy Mater 7: 1700529. https://doi.org/10.1002/aenm.201700529 doi: 10.1002/aenm.201700529
![]() |
[9] |
Fu CF, Wu X, Yang J (2018) Material design for photocatalytic water splitting from a theoretical perspective. Adv Mater 30: e1802106. https://doi.org/10.1002/adma.201802106 doi: 10.1002/adma.201802106
![]() |
[10] |
Li H, Zhou Y, Tu W, et al. (2015) State-of-the-art progress in diverse heterostructured photocatalysts toward promoting photocatalytic performance. Adv Funct Mater 25: 998–1013. http://doi.org/10.1002/adfm.201401636 doi: 10.1002/adfm.201401636
![]() |
[11] |
Sun X, Zhang X, Xie Y (2020) Surface defects in two-dimensional photocatalysts for efficient organic synthesis. Matter 2: 842–861. https://doi.org/10.1016/j.matt.2020.02.006 doi: 10.1016/j.matt.2020.02.006
![]() |
[12] |
Fan W, Zhang Q, Wang Y (2014) Semiconductor-based nanocomposites for photocatalytic H2 production and CO2 conversion. Phys Chem Chem Phys 15: 2632–2649. http://doi.org/10.1039/C2CP43524A doi: 10.1039/C2CP43524A
![]() |
[13] |
Zhang N, Gao C, Xiong Y (2019) Defect engineering: A versatile tool for tuning the activation of key molecules in photocatalytic reactions. J Energy Chem 37: 43–57. https://doi.org/10.1016/j.jechem.2018.09.010 doi: 10.1016/j.jechem.2018.09.010
![]() |
[14] |
Talapin DV, Lee JS, Kovalenko MV, et al. (2010) Prospects of colloidal nanocrystals for electronic and optoelectronic applications. Chem Rev 110: 389–458. https://doi.org/10.1021/cr900137k doi: 10.1021/cr900137k
![]() |
[15] |
Savage KJ, Hawkeye MM, Esteban R, et al. (2012) Revealing the quantum regime in tunnelling plasmonics. Nature 491: 574–577. https://doi.org/10.1038/nature11653 doi: 10.1038/nature11653
![]() |
[16] |
Meinardi F, Ehrenberg S, Dhamo L, et al. (2017) Highly efficient luminescent solar concentrators based on earth-abundant indirect-bandgap silicon quantum dots. Nature Photon 11: 177–185. https://doi.org/10.1038/nphoton.2017.5 doi: 10.1038/nphoton.2017.5
![]() |
[17] |
Smith AM, Mohs AM, Nie S (2019) Tuning the optical and electronic properties of colloidal nanocrystals by lattice strain. Nature Nanotech 4: 56–63. https://doi.org/10.1038/nnano.2008.360 doi: 10.1038/nnano.2008.360
![]() |
[18] |
Oh N, Kim BH, Cho SY, et al. (2017) Double-heterojunction nanorod light-responsive LEDs for display applications. Science 355: 616–619. https://doi.org/10.1126/science.aal2038 doi: 10.1126/science.aal2038
![]() |
[19] |
Du J, Singh R, Fedin I, et al. (2020) Spectroscopic insights into high defect tolerance of Zn: CuInSe2 quantum-dot-sensitized solar cells. Nat Energy 5: 409–417. https://doi.org/10.1038/s41560-020-0617-6 doi: 10.1038/s41560-020-0617-6
![]() |
[20] |
Chen R, Ren Z, Liang Y, et al. (2022) Spatiotemporal imaging of charge transfer in photocatalyst particles. Nature 610: 296–301. https://doi.org/10.1038/s41586-022-05183-1 doi: 10.1038/s41586-022-05183-1
![]() |
[21] |
Zhou P, Navid IA, Ma Y, et al. (2023) Solar-to-hydrogen efficiency of more than 9% in photocatalytic water splitting. Nature 613: 66–70. https://doi.org/10.1038/s41586-022-05399-1 doi: 10.1038/s41586-022-05399-1
![]() |
[22] |
Chen X, Liu L, Yu PY, et al. (2011) Increasing solar absorption for photocatalysis with black hydrogenated titanium dioxide nanocrystals. Science 331: 746–750. https://doi.org/10.1126/science.1200448 doi: 10.1126/science.1200448
![]() |
[23] |
Lotfi S, Fischer K, Schulze A, et al. (2022) Photocatalytic degradation of steroid hormone micropollutants by TiO2-coated polyethersulfone membranes in a continuous flow-through process. Nature Nanotech 17: 417–423. https://doi.org/10.1038/s41565-022-01074-8 doi: 10.1038/s41565-022-01074-8
![]() |
[24] |
Song S, Song H, Li L, et al. (2021) A selective Au-ZnO/TiO2 hybrid photocatalyst for oxidative coupling of methane to ethane with dioxygen. Nat Catal 4: 1032–1042. https://doi.org/10.1038/s41929-021-00708-9 doi: 10.1038/s41929-021-00708-9
![]() |
[25] |
Lin R, Wan J, Xiong Y, et al. (2018) Quantitative study of charge carrier dynamics in well-defined WO3 nanowires and nanosheets: Insight into the crystal facet effect in photocatalysis. J Am Chem Soc 140: 9078–9082. https://doi.org/10.1021/jacs.8b05293 doi: 10.1021/jacs.8b05293
![]() |
[26] |
Simon T, Bouchonville N, Berr MJ, et al. (2014) Redox shuttle mechanism enhances photocatalytic H2 generation on Ni-decorated CdS nanorods. Nat Mater 13: 1013–1018. https://doi.org/10.1038/nmat4049 doi: 10.1038/nmat4049
![]() |
[27] |
Han Z, Qiu F, Eisenberg R, et al. (2012) Robust photogeneration of H2 in water using semiconductor nanocrystals and a nickel catalyst. Science 338: 1321–1324. https://doi.org/10.1126/science.1227775 doi: 10.1126/science.1227775
![]() |
[28] |
Lin L, Lin Z, Zhang J, et al. (2020) Molecular-level insights on the reactive facet of carbon nitride single crystals photocatalysing overall water splitting. Nat Catal 3: 649–655. https://doi.org/10.1038/s41929-020-0476-3 doi: 10.1038/s41929-020-0476-3
![]() |
[29] |
Takata T, Jiang J, Sakata Y, et al. (2020) Photocatalytic water splitting with a quantum efficiency of almost unity. Nature 581: 411–414. https://doi.org/10.1038/s41586-020-2278-9 doi: 10.1038/s41586-020-2278-9
![]() |
[30] |
Regulacio MD, Han MY (2016) Multinary Ⅰ-Ⅲ-Ⅵ2 and Ⅰ2-Ⅱ-Ⅳ-Ⅵ4 semiconductor nanostructures for photocatalytic applications. Acc Chem Res 49: 511–519. https://doi.org/10.1021/acs.accounts.5b00535 doi: 10.1021/acs.accounts.5b00535
![]() |
[31] |
Ha E, Lee LY, Wang J, et al. (2014) Significant enhancement in photocatalytic reduction of water to hydrogen by Au/Cu2ZnSnS4 nanostructure. Adv Mater 26: 3496–3500. https://doi.org/10.1002/adma.201400243 doi: 10.1002/adma.201400243
![]() |
[32] |
Ye C, Regulacio MD, Lim SH, et al. (2012) Alloyed (ZnS)x(CuInS2)(1−x) semiconductor nanorods: Synthesis, bandgap tuning and photocatalytic properties. Chem Eur J 18: 11258–11263. https://doi.org/10.1002/chem.201201626 doi: 10.1002/chem.201201626
![]() |
[33] |
Li H, Li WJ, Li W, et al. (2020) Engineering crystal phase of polytypic CuInS2 nanosheets for enhanced photocatalytic and photoelectrochemical performance. Nano Res 13: 583–590. https://doi.org/10.1007/s12274-020-2665-4 doi: 10.1007/s12274-020-2665-4
![]() |
[34] |
Kageshima Y, Shiga S, Ode T, et al. (2021) Photocatalytic and photoelectrochemical hydrogen evolution from water over Cu2SnxGe1–xS3 particles. J Am Chem Soc 143: 5698–5708. https://doi.org/10.1021/jacs.0c12140 doi: 10.1021/jacs.0c12140
![]() |
[35] |
Fan FJ, Wu L, Yu SH (2013) Energetic Ⅰ–Ⅲ–Ⅵ2 and Ⅰ2–Ⅱ–Ⅳ–Ⅵ4 nanocrystals: Synthesis, photovoltaic and thermoelectric applications. Energy Environ Sci 7: 190–208. https://doi.org/10.1039/C3EE41437J doi: 10.1039/C3EE41437J
![]() |
[36] |
Chang ZX, Zhou WH, Kou DX, et al. (2014) Phase-dependent photocatalytic H2 evolution of copper zinc tin sulfide under visible light. Chem Commun 50: 12726–12729. https://doi.org/10.1039/c4cc05654j doi: 10.1039/c4cc05654j
![]() |
[37] |
Liu Q, Zhao Z, Lin Y, et al. (2011) Alloyed (ZnS)x(Cu2SnS3)1−x and (CuInS2)x(Cu2SnS3)1−x nanocrystals with arbitrary composition and broad tunable band gaps. Chem Commun 47: 964–966. http://doi.org/10.1039/C0CC03560B doi: 10.1039/C0CC03560B
![]() |
[38] |
Tan L, Liu Y, Mao B, et al. (2018) Effective bandgap narrowing of Cu-In-Zn-S quantum dots for photocatalytic H2 production via cocatalyst-alleviated charge recombination. Inorg Chem Front 5: 258–265. http://doi.org/10.1039/C7QI00607A doi: 10.1039/C7QI00607A
![]() |
[39] |
Wu L, Su F, Liu T, et al. (2022) Phosphorus-doped single-crystalline quaternary sulfide nanobelts enable efficient visible-light photocatalytic hydrogen evolution. J Am Chem Soc 144: 20620–20629. https://doi.org/10.1021/jacs.2c07313 doi: 10.1021/jacs.2c07313
![]() |
[40] |
Wu L, Wang Q, Zhuang TT, et al. (2020) Single crystalline quaternary sulfide nanobelts for efficient solar-to-hydrogen conversion. Nat Commun 11: 5194. https://doi.org/10.1038/s41467-020-18679-z doi: 10.1038/s41467-020-18679-z
![]() |
[41] |
Li Y, Wang Y, Pattengale B, et al. (2017) High-index faceted CuFeS2 nanosheets with enhanced behavior for boosting hydrogen evolution reaction. Nanoscale 9: 9230–9237. https://doi.org/10.1039/C7NR03182C doi: 10.1039/C7NR03182C
![]() |
[42] |
Zhang X, Xu Y, Zhang J, et al. (2018) Synthesis of wurtzite Cu2ZnSnS4 nanosheets with exposed high-energy (002) facets for fabrication of efficient Pt-free solar cell counter electrodes. Sci Rep 8: 248. https://doi.org/10.1038/s41598-017-18631-0 doi: 10.1038/s41598-017-18631-0
![]() |
[43] |
Wu L, Wang Q, Zhuang TT, et al. (2022) A library of polytypic copper-based quaternary sulfide nanocrystals enables efficient solar-to-hydrogen conversion. Nat Commun 13: 5414. https://doi.org/10.1038/s41467-022-33065-7 doi: 10.1038/s41467-022-33065-7
![]() |
[44] |
Chen SY, Gong XG, Walsh A, et al. (2009) Electronic structure and stability of quaternary chalcogenide semiconductors derived from cation cross-substitution of Ⅱ-Ⅵ and Ⅰ-Ⅲ-Ⅵ2 compounds. Phys Rev B 79: 165211. https://doi.org/10.1103/PhysRevB.79.165211 doi: 10.1103/PhysRevB.79.165211
![]() |
[45] |
Ozel F, Aslan E, Istanbullu B, et al. (2016) Photocatalytic hydrogen evolution based on Cu2ZnSnS4, Cu2NiSnS4 and Cu2CoSnS4 nanocrystals. Appl Catal B-Environ 198: 67–73. https://doi.org/10.1016/j.apcatb.2016.05.053 doi: 10.1016/j.apcatb.2016.05.053
![]() |
[46] |
Arcudi F, Đorđević L, Nagasing B, et al. (2021) Quantum dot-sensitized photoreduction of CO2 in water with turnover number > 80,000. J Am Chem Soc 143: 18131–18138. https://doi.org/10.1021/jacs.1c06961 doi: 10.1021/jacs.1c06961
![]() |
[47] |
Wang P, Minegishi T, Ma G, et al. (2012) Photoelectrochemical conversion of toluene to methylcyclohexane as an organic hydride by Cu2ZnSnS4-based photoelectrode assemblies. J Am Chem Soc 134: 2469–2472. https://doi.org/10.1021/ja209869k doi: 10.1021/ja209869k
![]() |
[48] |
Huang DW, Wang K, Yu L, et al. (2018) Over 1% efficient unbiased stable solar water splitting based on a sprayed Cu2ZnSnS4 photocathode protected by a HfO2 photocorrosion-resistant film. Acs Energy Lett 3: 1875–1881. https://doi.org/10.1021/acsenergylett.8b01005 doi: 10.1021/acsenergylett.8b01005
![]() |
[49] |
Semalti P, Sharma V, Sharma SN (2021) A novel method of water remediation of organic pollutants and industrial wastes by solution-route processed CZTS nanocrystals. J Materiomics 7: 904–919. https://doi.org/10.1016/j.jmat.2021.04.005 doi: 10.1016/j.jmat.2021.04.005
![]() |
[50] |
Akkerman QA, Genovese A, George C, et al. (2015) From binary Cu2S to ternary Cu-In-S and quaternary Cu-In-Zn-S nanocrystals with tunable composition via partial cation exchange. ACS Nano 9: 521–531. https://doi.org/10.1021/nn505786d doi: 10.1021/nn505786d
![]() |
[51] |
De Trizio L, Li H, Casu A, et al. (2014) Sn cation valency dependence in cation exchange reactions involving Cu2−xSe nanocrystals. J Am Chem Soc 136: 16277–16284. https://doi.org/10.1021/ja508161c doi: 10.1021/ja508161c
![]() |
[52] |
Pan D, An L, Sun Z, et al. (2018) Synthesis of Cu−In−S ternary nanocrystals with tunable structure and composition. J Am Chem Soc 130: 5620–5621. https://doi.org/10.1021/ja711027j doi: 10.1021/ja711027j
![]() |
[53] |
Akkari FC, Abdelkader D, Gallas B, et al. (2017) Ellipsometric characterization and optical anisotropy of nanostructured CuIn3S5 and CuIn5S8 thin films. Mat Sci Semicon Proc 71: 156–160. https://doi.org/10.1016/j.mssp.2017.07.023 doi: 10.1016/j.mssp.2017.07.023
![]() |
[54] |
Khemiri N, Kanzari M (2010) Comparative study of structural and morphological properties of CuIn3S5 and CuIn7S11 materials. Nucl Instrum Meth B 268: 268–272. https://doi.org/10.1016/j.nimb.2009.10.175 doi: 10.1016/j.nimb.2009.10.175
![]() |
[55] |
Li XD, Sun YF, Xu JQ, et al. (2019) Selective visible-light-driven photocatalytic CO2 reduction to CH4 mediated by atomically thin CuIn5S8 layers. Nat Energy 4: 690–699. https://doi.org/10.1038/s41560-019-0431-1 doi: 10.1038/s41560-019-0431-1
![]() |
[56] |
Ng C, Yun JH, Tan HL, et al. (2018) A dual-electrolyte system for photoelectrochemical hydrogen generation using CuInS2-In2O3-TiO2 nanotube array thin film. Sci China Mater 61: 895–904. https://doi.org/10.1007/s40843-017-9237-2 doi: 10.1007/s40843-017-9237-2
![]() |
[57] |
Xu W, Xie ZZ, Han WJ, et al. (2022) Rational design of interfacial energy level matching for CuGaS2 based photocatalysts over hydrogen evolution reaction. Int J Hydrogen Energy 47: 11853–11862. https://doi.org/10.1016/j.ijhydene.2022.01.209 doi: 10.1016/j.ijhydene.2022.01.209
![]() |
[58] |
Ghorpade UV, Suryawanshi MP, Shin SW, et al. (2016) Colloidal wurtzite Cu2SnS3 (CTS) nanocrystals and their applications in solar cells. Chem Mater 28: 3308–3317. https://doi.org/10.1021/acs.chemmater.6b00176 doi: 10.1021/acs.chemmater.6b00176
![]() |
[59] |
Jathar SB, Rondiya SR, Jadhav YA, et al. (2021) Ternary Cu2SnS3: Synthesis, structure, photoelectrochemical activity, and heterojunction band offset and alignment. Chem Mater 33: 1983–1993. https://doi.org/10.1021/acs.chemmater.0c03223 doi: 10.1021/acs.chemmater.0c03223
![]() |
[60] |
Yi LX, Wang D, Gao MY (2012) Synthesis of Cu3SnS4 nanocrystals and nanosheets by using Cu31S16 as seeds. CrystEngComm 14: 401–404. https://doi.org/10.1039/C1ce06153d doi: 10.1039/C1ce06153d
![]() |
[61] |
Lin J, Lim JM, Youn DH, et al. (2019) Cu4SnS4-rich nanomaterials for thin-film lithium batteries with enhanced conversion reaction. ACS Nano 13: 10671–10681. https://doi.org/10.1021/acsnano.9b05029 doi: 10.1021/acsnano.9b05029
![]() |
[62] |
Wang J, Bo T, Shao B, et al. (2021) Effect of S vacancy in Cu3SnS4 on high selectivity and activity of photocatalytic CO2 reduction. Appl Catal B-Environ 297: 120498. https://doi.org/10.1016/j.apcatb.2021.120498 doi: 10.1016/j.apcatb.2021.120498
![]() |
[63] |
Kamemoto S, Matsuda Y, Takahashi M, et al. (2022) Photocatalytic water splitting on Cu2SnS3 photoelectrode: Effects of Cu/Sn composite ratio on the photoelectrochemical performance. Catal Today 411–412: 113820. https://doi.org/10.1016/j.cattod.2022.06.035 doi: 10.1016/j.cattod.2022.06.035
![]() |
[64] |
An X, Kays JC, Lightcap IV, et al. (2022) Wavelength-dependent bifunctional plasmonic photocatalysis in Au/chalcopyrite hybrid nanostructures. ACS Nano 16: 6813–6824. https://doi.org/10.1021/acsnano.2c01706 doi: 10.1021/acsnano.2c01706
![]() |
[65] |
Sugathan A, Bhattacharyya B, Kishore VVR, et al. (2018) Why does CuFeS2 resemble gold? J Phys Chem Lett 9: 696–701. https://doi.org/10.1021/acs.jpclett.7b03190 doi: 10.1021/acs.jpclett.7b03190
![]() |
[66] |
Bhattacharyya B, Pandey A (2016) CuFeS2 quantum dots and highly luminescent CuFeS2 based core/shell structures: Synthesis, tunability, and photophysics. J Am Chem Soc 138: 10207–10213. https://doi.org/10.1021/jacs.6b04981 doi: 10.1021/jacs.6b04981
![]() |
[67] |
Tsuji I, Kato H, Kobayashi H, et al. (2005) Photocatalytic H2 evolution under visible-light irradiation over band-structure-controlled (CuIn)xZn2(1−x)S2 solid solutions. J Phys Chem B 109: 7323–7329. https://doi.org/10.1021/jp044722e doi: 10.1021/jp044722e
![]() |
[68] |
Goodman CHL (1958) The prediction of semiconducting properties in inorganic compounds. J Phys Chem Solids 6: 305–314. https://doi.org/10.1016/0022-3697(58)90050-7 doi: 10.1016/0022-3697(58)90050-7
![]() |
[69] |
Yan C, Huang J, Sun K, et al. (2018) Cu2ZnSnS4 solar cells with over 10% power conversion efficiency enabled by heterojunction heat treatment. Nat Energy 3: 764–772. https://doi.org/10.1038/s41560-018-0206-0 doi: 10.1038/s41560-018-0206-0
![]() |
[70] |
De Trizio L, Prato M, Genovese A, et al. (2012) Strongly fluorescent quaternary Cu–In–Zn–S nanocrystals prepared from Cu1−xInS2 nanocrystals by partial cation exchange. Chem Mater 24: 2400–2406. https://doi.org/10.1021/cm301211e doi: 10.1021/cm301211e
![]() |
[71] |
Cui Y, Wang G, Pan D (2012) Synthesis and photoresponse of novel Cu2CdSnS4 semiconductor nanorods. J Mater Chem 22: 12471. https://doi.org/10.1039/c2jm32034g doi: 10.1039/c2jm32034g
![]() |
[72] |
Ikeda S, Fujikawa S, Harada T, et al. (2019) Photocathode characteristics of a spray-deposited Cu2ZnGeS4 thin film for CO2 reduction in a CO2-saturated aqueous solution. ACS Appl Energy Mater 2: 6911–6918. https://doi.org/10.1021/acsaem.9b01418 doi: 10.1021/acsaem.9b01418
![]() |
[73] |
Singh A, Singh A, Ciston J, et al. (2015) Synergistic role of dopants on the morphology of alloyed copper chalcogenide nanocrystals. J Am Chem Soc 137: 6464–6467. https://doi.org/10.1021/jacs.5b02880 doi: 10.1021/jacs.5b02880
![]() |
[74] |
Huang S, He Q, Zai J, et al. (2015) The role of Mott–Schottky heterojunctions in PtCo–Cu2ZnGeS4 as counter electrodes in dye-sensitized solar cells. Chem Commun 51: 8950–8953. http://doi.org/10.1039/C5CC02584B doi: 10.1039/C5CC02584B
![]() |
[75] |
Septina W, Gunawan, Ikeda S, et al. (2015) Photosplitting of water from wide-gap Cu(In, Ga)S2 thin films modified with a CdS layer and Pt nanoparticles for a high-onset-potential photocathode. J Phys Chem C 119: 8576–8583. http://doi.org/10.1021/acs.jpcc.5b02068 doi: 10.1021/acs.jpcc.5b02068
![]() |
[76] |
Liu XY, Zhang G, Chen H, et al. (2017) Efficient defect-controlled photocatalytic hydrogen generation based on near-infrared Cu-In-Zn-S quantum dots. Nano Res 11: 1379–1388. https://doi.org/10.1007/s12274-017-1752-7 doi: 10.1007/s12274-017-1752-7
![]() |
[77] |
Ye C, Regulacio MD, Lim SH, et al. (2015) Alloyed ZnS-CuInS2 semiconductor nanorods and their nanoscale heterostructures for visible-light-driven photocatalytic hydrogen generation. Chem Eur J 21: 9514–9519. https://doi.org/10.1002/chem.201500781 doi: 10.1002/chem.201500781
![]() |
[78] |
Chen FK, Zai JT, Xu M, et al. (2013) 3D-hierarchical Cu3SnS4 flowerlike microspheres: Controlled synthesis, formation mechanism and photocatalytic activity for H2 evolution from water. J Mater Chem A 1: 4316–4323. https://doi.org/10.1039/C3ta01491f doi: 10.1039/C3ta01491f
![]() |
[79] |
Zhang P, Wang T, Chang X, et al. (2016) Effective charge carrier utilization in photocatalytic conversions. Acc Chem Res 49: 911–921. http://doi.org/10.1021/acs.accounts.6b00036 doi: 10.1021/acs.accounts.6b00036
![]() |
[80] |
Wei Y, Yang N, Huang K, et al. (2020) Steering hollow multishelled structures in photocatalysis: Optimizing surface and mass transport. Adv Mater 32: e2002556. http://doi.org/10.1002/adma.202002556 doi: 10.1002/adma.202002556
![]() |
[81] |
Chen Q, Liu Y, Gu X, et al. (2022) Carbon dots mediated charge sinking effect for boosting hydrogen evolution in Cu-In-Zn-S QDs/MoS2 photocatalysts. Appl Catal B-Environ 301: 120755. http://doi.org/10.1016/j.apcatb.2021.120755 doi: 10.1016/j.apcatb.2021.120755
![]() |
[82] |
Yu X, Shavel A, An X, et al. (2014) Cu2ZnSnS4-Pt and Cu2ZnSnS4-Au heterostructured nanoparticles for photocatalytic water splitting and pollutant degradation. J Am Chem Soc 136: 9236–9239. http://doi.org/10.1021/ja502076b doi: 10.1021/ja502076b
![]() |
[83] |
Ali N, Tsega TT, Cao Y, et al. (2021) Copper vacancy activated plasmonic Cu3−xSnS4 for highly efficient photocatalytic hydrogen generation: Broad solar absorption, efficient charge separation and decreased HER overpotential. Nano Res 14: 3358–3364. https://doi.org/10.1007/s12274-021-3604-8 doi: 10.1007/s12274-021-3604-8
![]() |
[84] |
Fan XB, Yu S, Zhan F, et al. (2017) Nonstoichiometric CuxInyS quantum dots for efficient photocatalytic hydrogen evolution. ChemSusChem 10: 4833–4838. https://doi.org/10.1002/cssc.201701950 doi: 10.1002/cssc.201701950
![]() |
[85] |
Yu X, An X, Genç A, et al. (2015) Cu2ZnSnS4–PtM (M = Co, Ni) nanoheterostructures for photocatalytic hydrogen evolution. J Phys Chem C 119: 21882–21888. http://doi.org/10.1021/acs.jpcc.5b06199 doi: 10.1021/acs.jpcc.5b06199
![]() |
[86] |
Lin T, Yang T, Cai Y, et al. (2023) Transformation-optics-designed plasmonic singularities for efficient photocatalytic hydrogen evolution at metal/semiconductor interfaces. Nano Lett 23: 5288–5296. http://doi.org/10.1021/acs.nanolett.3c01287 doi: 10.1021/acs.nanolett.3c01287
![]() |
[87] |
Jiang F, Pan B, You D, et al. (2016) Visible light photocatalytic H2-production activity of epitaxial Cu2ZnSnS4/ZnS heterojunction. Catal Commun 85: 39–43. http://doi.org/10.1016/j.catcom.2016.07.017 doi: 10.1016/j.catcom.2016.07.017
![]() |
[88] |
Yuan M, Wang JL, Zhou WH, et al. (2017) Cu2ZnSnS4-CdS heterostructured nanocrystals for enhanced photocatalytic hydrogen production. Catal Sci Technol 7: 3980–3984. http://doi.org/10.1039/c7cy01360d doi: 10.1039/c7cy01360d
![]() |
[89] |
Yuan M, Zhou WH, Kou DX, et al. (2018) Cu2ZnSnS4 decorated CdS nanorods for enhanced visible-light-driven photocatalytic hydrogen production. Int J Hydrogen Energy 43: 20408–20416. https://doi.org/10.1016/j.ijhydene.2018.09.161 doi: 10.1016/j.ijhydene.2018.09.161
![]() |
[90] |
Zhuang TT, Yu P, Fan FJ, et al. (2014) Controlled synthesis of kinked ultrathin ZnS nanorods/nanowires triggered by chloride ions: A case study. Small 10: 1394–1402. https://doi.org/10.1002/smll.201302656 doi: 10.1002/smll.201302656
![]() |
[91] |
Li Y, Zhuang TT, Fan F, et al. (2018) Pulsed axial epitaxy of colloidal quantum dots in nanowires enables facet-selective passivation. Nat Commun 9: 4947. https://doi.org/10.1038/s41467-018-07422-4 doi: 10.1038/s41467-018-07422-4
![]() |
[92] |
Li M, Zhao Z, Cheng T, et al. (2016) Ultrafine jagged platinum nanowires enable ultrahigh mass activity for the oxygen reduction reaction. Science 354: 1414–1419. https://doi.org/10.1126/science.aaf9050 doi: 10.1126/science.aaf9050
![]() |
[93] |
Chen D, Zhang H, Li Y, et al. (2018) Spontaneous formation of noble- and heavy-metal-free alloyed semiconductor quantum rods for efficient photocatalysis. Adv Mater 30: e1803351. https://doi.org/10.1002/adma.201803351 doi: 10.1002/adma.201803351
![]() |
[94] |
Zhang Z, Wu Q, Johnson G, et al. (2019) Generalized synthetic strategy for transition-metal-doped brookite-phase TiO2 nanorods. J Am Chem Soc 141: 16548–16552. https://doi.org/10.1021/jacs.9b06389 doi: 10.1021/jacs.9b06389
![]() |
[95] |
Gonce MK, Aslan E, Ozel F, et al. (2016) Dye-sensitized Cu2XSnS4 (X = Zn, Ni, Fe, Co, and Mn) nanofibers for efficient photocatalytic hydrogen evolution. ChemSusChem 9: 600–605. http://doi.org/10.1002/cssc.201501661 doi: 10.1002/cssc.201501661
![]() |
[96] |
Zhou Y, Zhang Y, Lin M, et al. (2015) Monolayered Bi2WO6 nanosheets mimicking heterojunction interface with open surfaces for photocatalysis. Nat Commun 6: 8340. http://doi.org/10.1038/ncomms9340 doi: 10.1038/ncomms9340
![]() |
[97] |
Tian B, Tian B, Smith B, et al. (2018) Facile bottom-up synthesis of partially oxidized black phosphorus nanosheets as metal-free photocatalyst for hydrogen evolution. Proc Natl Acad Sci 115: 4345–4350. http://doi.org/10.1073/pnas.1800069115 doi: 10.1073/pnas.1800069115
![]() |
[98] |
Nishioka S, Hojo K, Xiao L, et al. (2022) Surface-modified, dye-sensitized niobate nanosheets enabling an efficient solar-driven Z-scheme for overall water splitting. Sci Adv 8: eadc9115. https://doi.org/10.1126/sciadv.adc9115 doi: 10.1126/sciadv.adc9115
![]() |
[99] |
Ganguly P, Harb M, Cao Z, et al. (2019) 2D Nanomaterials for photocatalytic hydrogen production. ACS Energy Lett 4: 1687–1709. https://doi.org/10.1021/acsenergylett.9b00940 doi: 10.1021/acsenergylett.9b00940
![]() |
[100] |
Wang L, Wang W, Sun S (2012) A simple template-free synthesis of ultrathin Cu2ZnSnS4 nanosheets for highly stable photocatalytic H2 evolution. J Mater Chem 22: 6553–6555. https://doi.org/10.1039/c2jm16515e doi: 10.1039/c2jm16515e
![]() |
[101] |
Zheng L, Xu Y, Song Y, et al. (2009) Nearly monodisperse CuInS2 hierarchical microarchitectures for photocatalytic H2 evolution under visible light. Inorg Chem 48: 4003–4009. https://doi.org/10.1021/ic802399f doi: 10.1021/ic802399f
![]() |
[102] |
Huang Y, Chen J, Zou W, et al. (2015) Enhanced photocatalytic hydrogen evolution efficiency using hollow microspheres of (CuIn)xZn2(1−x)S2 solid solutions. Dalton Trans 44: 10991–10996. https://doi.org/10.1039/c5dt01269d doi: 10.1039/c5dt01269d
![]() |
[103] |
Chen Y, Qin Z, Guo X, et al. (2016) One-step hydrothermal synthesis of (CuIn)0.2Zn1.6S2 hollow sub-microspheres for efficient visible-light-driven photocatalytic hydrogen generation. Int J Hydrogen Energy 41: 1524–1534. https://doi.org/10.1016/j.ijhydene.2015.11.087 doi: 10.1016/j.ijhydene.2015.11.087
![]() |
[104] |
Li M, Zhao RJ, Su YJ, et al. (2016) Hierarchically CuInS2 nanosheet-constructed nanowire arrays for photoelectrochemical water splitting. Adv Mater Interfaces 3: 1600494. https://doi.org/10.1002/admi.201600494 doi: 10.1002/admi.201600494
![]() |
[105] |
Xu J, Hu Z, Zhang J, et al. (2017) Cu2ZnSnS4 and Cu2ZnSn(S1−xSex)4 nanocrystals: Room-temperature synthesis and efficient photoelectrochemical water splitting. J Mater Chem A 5: 25230–25236. http://doi.org/10.1039/C7TA06628G doi: 10.1039/C7TA06628G
![]() |
[106] |
Han Z, Eisenberg R (2014) Fuel from water: The photochemical generation of hydrogen from water. Acc Chem Res 47: 2537–2544. http://doi.org/10.1021/ar5001605 doi: 10.1021/ar5001605
![]() |
[107] |
Teitsworth TS, Hill DJ, Litvin SR, et al. (2023) Water splitting with silicon p–i–n superlattices suspended in solution. Nature 614: 270–274. https://doi.org/10.1038/s41586-022-05549-5 doi: 10.1038/s41586-022-05549-5
![]() |
[108] |
Li Z, Li R, Jing H, et al. (2023) Blocking the reverse reactions of overall water splitting on a Rh/GaN–ZnO photocatalyst modified with Al2O3. Nat Catal 6: 80–88. https://doi.org/10.1038/s41929-022-00907-y doi: 10.1038/s41929-022-00907-y
![]() |
[109] |
Zhang JH, Zhang M, Dong YY, et al. (2022) CdTe/CdSe-sensitized photocathode coupling with Ni-substituted polyoxometalate catalyst for photoelectrochemical generation of hydrogen. Nano Res 15: 1347–1354. https://doi.org/10.1007/s12274-021-3663-x doi: 10.1007/s12274-021-3663-x
![]() |
[110] |
Lee S, Ji L, De Palma AC, et al. (2021) Scalable, highly stable Si-based metal-insulator-semiconductor photoanodes for water oxidation fabricated using thin-film reactions and electrodeposition. Nat Commun 12: 3982. https://doi.org/10.1038/s41467-021-24229-y doi: 10.1038/s41467-021-24229-y
![]() |
[111] |
Cheng F, Lin G, Hu X, et al. (2019) Porous single-crystalline titanium dioxide at 2 cm scale delivering enhanced photoelectrochemical performance. Nat Commun 10: 3618. https://doi.org/10.1038/s41467-019-11623-w doi: 10.1038/s41467-019-11623-w
![]() |
[112] |
Liu Z, Lu X, Chen D (2018) Photoelectrochemical water splitting of CuInS2 photocathode collaborative modified with separated catalysts based on efficient photogenerated electron–hole separation. ACS Sustainable Chem Eng 6: 10289–10294. https://doi.org/10.1021/acssuschemeng.8b01607 doi: 10.1021/acssuschemeng.8b01607
![]() |
[113] |
Kaneko H, Minegishi T, Nakabayashi M, et al. (2016) Enhanced hydrogen evolution under simulated sunlight from neutral electrolytes on (ZnSe)0.85(CuIn0.7Ga0.3Se2)0.15 photocathodes prepared by a bilayer method. Angew Chem Int Ed 55: 15329–15333. https://doi.org/10.1002/anie.201609202 doi: 10.1002/anie.201609202
![]() |
[114] |
Tay YF, Kaneko H, Chiam SY, et al. (2018) Solution-processed Cd-substituted CZTS photocathode for efficient solar hydrogen evolution from neutral water. Joule 2: 537–548. http://doi.org/10.1016/j.joule.2018.01.012 doi: 10.1016/j.joule.2018.01.012
![]() |
[115] |
Guo P, Xiao Y, Mo R, et al. (2023) Coherent-twinning-enhanced solar water splitting in thin-film Cu2ZnSnS4 photocathodes. ACS Energy Lett 8: 494–501. https://doi.org/10.1021/acsenergylett.2c02626 doi: 10.1021/acsenergylett.2c02626
![]() |
[116] |
Yan C, Sun K, Huang J, et al. (2017) Beyond 11% efficient sulfide kesterite Cu2ZnxCd1–xSnS4 solar cell: Effects of cadmium alloying. ACS Energy Lett 2: 930–936. http://doi.org/10.1021/acsenergylett.7b00129 doi: 10.1021/acsenergylett.7b00129
![]() |
[117] |
Yang W, Oh Y, Kim J, et al. (2016) Molecular chemistry-controlled hybrid ink-derived efficient Cu2ZnSnS4 photocathodes for photoelectrochemical water splitting. ACS Energy Lett 1: 1127–1136. https://doi.org/10.1021/acsenergylett.6b00453 doi: 10.1021/acsenergylett.6b00453
![]() |
[118] |
Mali MG, Yoon H, Joshi BN, et al. (2015) Enhanced photoelectrochemical solar water splitting using a platinum-decorated CIGS/CdS/ZnO photocathode. ACS Appl Mater Interfaces 7: 21619–21625. https://doi.org/10.1021/acsami.5b07267 doi: 10.1021/acsami.5b07267
![]() |
[119] |
Sun W, Ye Y, You Y, et al. (2018) A top-down synthesis of wurtzite Cu2SnS3 nanocrystals for efficient photoelectrochemical performance. J Mater Chem A 6: 8221–8226. http://doi.org/10.1039/C8TA00851E doi: 10.1039/C8TA00851E
![]() |
[120] |
Li M, Zhao R, Su Y, et al. (2017) Synthesis of CuInS2 nanowire arrays via solution transformation of Cu2S self-template for enhanced photoelectrochemical performance. Appl Catal B-Environ 203: 715–724. https://doi.org/10.1016/j.apcatb.2016.10.051 doi: 10.1016/j.apcatb.2016.10.051
![]() |
[121] |
Nishi H, Kuwabata S, Torimoto T (2013) Composition-dependent photoelectrochemical properties of nonstoichiometric Cu2ZnSnS4 nanoparticles. J Phys Chem C 117: 21055–21063. https://doi.org/10.1021/jp405008m doi: 10.1021/jp405008m
![]() |
[122] |
Chen Y, Chuang CH, Lin KC, et al. (2014) Synthesis and photoelectrochemical properties of (Cu2Sn)xZn3(1–x)S3 nanocrystal films. J Phys Chem C 118: 11954–11963. http://doi.org/10.1021/jp500270d doi: 10.1021/jp500270d
![]() |
[123] |
Yokoyama D, Minegishi T, Jimbo K, et al. (2010) H2 evolution from water on modified Cu2ZnSnS4 photoelectrode under solar light. Appl Phy Express 3: 101202. https://doi.org/10.1143/APEX.3.101202 doi: 10.1143/APEX.3.101202
![]() |
[124] |
Wu XJ, Huang X, Qi X, et al. (2014) Copper-based ternary and quaternary semiconductor nanoplates: Templated synthesis, characterization, and photoelectrochemical properties. Angew Chem Int Ed 53: 8929–8933. https://doi.org/10.1002/anie.201403655 doi: 10.1002/anie.201403655
![]() |
[125] |
Yang W, Oh Y, Kim J, et al. Photoelectrochemical properties of vertically aligned CuInS2 nanorod arrays prepared via template-assisted growth and transfer. ACS Appl Mater Interfaces 8: 425–431. https://doi.org/10.1021/acsami.5b09241 doi: 10.1021/acsami.5b09241
![]() |
[126] |
Guijarro N, Prevot MS, Sivula K (2014) Enhancing the charge separation in nanocrystalline Cu2ZnSnS4 photocathodes for photoelectrochemical application: The role of surface modifications. J Phys Chem Lett 5: 3902–3908. https://doi.org/10.1021/jz501996s doi: 10.1021/jz501996s
![]() |
[127] |
Patra BK, Khilari S, Pradhan D, et al. (2016) Hybrid dot–disk Au-CuInS2 nanostructures as active photocathode for efficient evolution of hydrogen from water. Chem Mater 28: 4358–4366. http://doi.org/10.1021/acs.chemmater.6b01357 doi: 10.1021/acs.chemmater.6b01357
![]() |
[128] |
Hu Y, Pan Y, Wang Z, et al. Lattice distortion induced internal electric field in TiO2 photoelectrode for efficient charge separation and transfer. Nat Commun 11: 2129. https://doi.org/10.1038/s41467-020-15993-4 doi: 10.1038/s41467-020-15993-4
![]() |
[129] |
Kang Z, Si H, Zhang S, et al. (2019) Interface engineering for modulation of charge carrier behavior in ZnO photoelectrochemical water splitting. Adv Funct Mater 29: 1808032. https://doi.org/10.1002/adfm.201808032 doi: 10.1002/adfm.201808032
![]() |
[130] |
You YM, Tong X, Channa AI, et al. (2022) Tailoring the optoelectronic properties of eco-friendly CuGaS2/ZnSe core/shell quantum dots for boosted photoelectrochemical solar hydrogen production. Ecomat 4: e12206. https://doi.org/10.1002/eom2.12206 doi: 10.1002/eom2.12206
![]() |
[131] |
Channa AI, Tong X, Xu JY, et al. (2019) Tailored near-infrared-emitting colloidal heterostructured quantum dots with enhanced visible light absorption for high performance photoelectrochemical cells. J Mater Chem A 7: 10225–10230. http://doi.org/10.1039/C9TA01052A doi: 10.1039/C9TA01052A
![]() |
[132] |
Wang R, Xu X, Zhang Y, et al. (2015) Functionalized ZnO@TiO2 nanorod array film loaded with ZnIn0.25Cu0.02S1.395 solid-solution: Synthesis, characterization and enhanced visible light driven water splitting. Nanoscale 7: 11082–11092. http://doi.org/10.1039/C5NR02127H doi: 10.1039/C5NR02127H
![]() |
[133] |
Wu HL, Li XB, Tung CH, et al. (2018) Recent advances in sensitized photocathodes: From molecular dyes to semiconducting quantum dots. Adv Sci 5: 1700684. http://doi.org/10.1002/advs.201700684 doi: 10.1002/advs.201700684
![]() |
[134] |
Li TL, Teng H (2010) Solution synthesis of high-quality CuInS2 quantum dots as sensitizers for TiO2 photoelectrodes. J Mater Chem 20: 3656–3664. https://doi.org/10.1039/b927279h doi: 10.1039/b927279h
![]() |
[135] |
Liu C, Tong X, Channa AI, et al. (2021) Tuning the composition of heavy metal-free quaternary quantum dots for improved photoelectrochemical performance. J Mater Chem A 9: 5825–5832. http://doi.org/10.1039/D0TA11481B doi: 10.1039/D0TA11481B
![]() |
[136] |
Liu Q, Cao F, Wu F, et al. (2016) Partial ion exchange derived 2D Cu–Zn–In–S nanosheets as sensitizers of 1D TiO2 nanorods for boosting solar water splitting. ACS Appl Mater Interfaces 8: 26235–26243. http://doi.org/10.1021/acsami.6b08648 doi: 10.1021/acsami.6b08648
![]() |
[137] |
Jiang Z, Xu X, Ma Y, et al. (2020) Filling metal-organic framework mesopores with TiO2 for CO2 photoreduction. Nature 586: 549–554. http://doi.org/10.1038/s41586-020-2738-2 doi: 10.1038/s41586-020-2738-2
![]() |
[138] |
Loh JYY, Kherani NP, Ozin GA (2021) Persistent CO2 photocatalysis for solar fuels in the dark. Nat Sustain 4: 466–473. https://doi.org/10.1038/s41893-021-00681-y doi: 10.1038/s41893-021-00681-y
![]() |
[139] |
Gao W, Li S, He H, et al. (2021) Vacancy-defect modulated pathway of photoreduction of CO2 on single atomically thin AgInP2S6 sheets into olefiant gas. Nat Commun 12: 4747. https://doi.org/10.1038/s41467-021-25068-7 doi: 10.1038/s41467-021-25068-7
![]() |
[140] |
Li K, Peng B, Peng T (2016) Recent advances in heterogeneous photocatalytic CO2 conversion to solar fuels. ACS Catal 6: 7485–7527. http://doi.org/10.1021/acscatal.6b02089 doi: 10.1021/acscatal.6b02089
![]() |
[141] |
Zhou M, Wang S, Yang P, et al. (2018) Boron carbon nitride semiconductors decorated with CdS nanoparticles for photocatalytic reduction of CO2. ACS Catal 8: 4928–4936. https://doi.org/10.1021/acscatal.8b00104 doi: 10.1021/acscatal.8b00104
![]() |
[142] |
Pang H, Meng X, Li P, et al. (2019) Cation vacancy-initiated CO2 photoreduction over ZnS for efficient formate production. ACS Energy Lett 4: 1387–1393. https://doi.org/10.1021/acsenergylett.9b00711 doi: 10.1021/acsenergylett.9b00711
![]() |
[143] |
Yang Z, Yang J, Ji H, et al. (2022) Construction of S–Co–S internal electron transport bridges in Co-doped CuInS2 for enhancing photocatalytic CO2 reduction. Mater Today Chem 26: 101078. https://doi.org/10.1016/j.mtchem.2022.101078 doi: 10.1016/j.mtchem.2022.101078
![]() |
[144] |
Kong Y, Li Y, Zhang Y, et al. (2021) Unveiling the selectivity of CO2 reduction on Cu2ZnSnS4: The effect of exposed termination. J Phys Chem C 125: 24967–24973. https://doi.org/10.1021/acs.jpcc.1c08013 doi: 10.1021/acs.jpcc.1c08013
![]() |
[145] |
Yoshida T, Yamaguchi A, Umezawa N, et al. (2018) Photocatalytic CO2 reduction using a pristine Cu2ZnSnS4 film electrode under visible light irradiation. J Phys Chem C 122: 21695–21702. https://doi.org/10.1021/acs.jpcc.8b04241 doi: 10.1021/acs.jpcc.8b04241
![]() |
[146] |
Zhou S, Sun K, Huang J, et al. (2021) Accelerating electron-transfer and tuning product selectivity through surficial vacancy engineering on CZTS/CdS for photoelectrochemical CO2 reduction. Small 17: e2100496. https://doi.org/10.1002/smll.202100496 doi: 10.1002/smll.202100496
![]() |
[147] |
Sharma N, Das T, Kumar S, et al. (2019) Photocatalytic activation and reduction of CO2 to CH4 over single phase nano Cu3SnS4: Acombined experimental and theoretical study. ACS Appl Energy Mater 2: 5677–5685. https://doi.org/10.1021/acsaem.9b00813 doi: 10.1021/acsaem.9b00813
![]() |
[148] |
Zhao H, Duan J, Zhang Z, et al. (2022) S‐scheme heterojunction and defect site engineering on CuxIn5S8−Cu2−ySe for highly efficient photoreduction of CO2 to methanol. ChemCatChem 14: e202101733. https://doi.org/10.1002/cctc.202101733 doi: 10.1002/cctc.202101733
![]() |
[149] |
Lian S, Kodaimati MS, Dolzhnikov DS, et al. (2017) Powering a CO2 reduction catalyst with visible light through multiple sub-picosecond electron transfers from a quantum dot. J Am Chem Soc 139: 8931–8938. http://doi.org/10.1021/jacs.7b03134 doi: 10.1021/jacs.7b03134
![]() |
[150] |
Wang L, Zhang Z, Guan R, et al. (2022) Synergistic CO2 reduction and tetracycline degradation by CuInZnS-Ti3C2Tx in one photoredox cycle. Nano Res 15: 8010–8018. https://doi.org/10.1007/s12274-022-4661-3 doi: 10.1007/s12274-022-4661-3
![]() |
[151] |
Cheruvathoor Poulose A, Zoppellaro G, Konidakis I, et al. (2022) Fast and selective reduction of nitroarenes under visible light with an earth-abundant plasmonic photocatalyst. Nat Nanotechnol 17: 485–492. https://doi.org/10.1038/s41565-022-01087-3 doi: 10.1038/s41565-022-01087-3
![]() |
[152] |
Zhou S, Sun K, Toe CY, et al. (2022) Engineering a kesterite-based photocathode for photoelectrochemical ammonia synthesis from NOx reduction. Adv Mater 34: e2201670. https://doi.org/10.1002/adma.202201670 doi: 10.1002/adma.202201670
![]() |
[153] |
Xu X, Tang D, Cai J, et al. (2019) Heterogeneous activation of peroxymonocarbonate by chalcopyrite (CuFeS2) for efficient degradation of 2, 4-dichlorophenol in simulated groundwater. Appl Catal B-Environ 251: 273–282. https://doi.org/10.1016/j.apcatb.2019.03.080 doi: 10.1016/j.apcatb.2019.03.080
![]() |
[154] |
Liu K, Cui R, Liu Z, et al. (2023) Fractional thermal reduction of CuInS2 quantum dot-sensitized Bi2MoO6 hierarchical flowers on S-doped biochar for dual Z-scheme/Mott–Schottky heterojunction construction: A strategy for efficient photocatalytic biorefineries. ACS Sustainable Chem Eng 11: 5400–5407. https://doi.org/10.1021/acssuschemeng.2c06572 doi: 10.1021/acssuschemeng.2c06572
![]() |
[155] |
Zhang Y, Chen X, Ye Y, et al. (2023) Photocatalytic oxygen reduction reaction over copper-indium-sulfide modified polymeric carbon nitride S-scheme heterojunction photocatalyst. J Catal 419: 9–18. https://doi.org/10.1016/j.jcat.2023.01.021 doi: 10.1016/j.jcat.2023.01.021
![]() |
[156] |
Delekar SD, Dhodamani AG, More KV, et al. (2018) Structural and optical properties of nanocrystalline TiO2 with multiwalled carbon nanotubes and its photovoltaic studies using Ru(Ⅱ) sensitizers. ACS Omega 3:2743–2756. https://doi.org/10.1021/acsomega.7b01316 doi: 10.1021/acsomega.7b01316
![]() |
[157] |
Patil MS, Deshmukh PS, Dhodamani GA, et al. (2017) Different strategies for modification of titanium dioxide as heterogeneous catalyst in chemical transformations. Curr Org Chem 21: 821–833. http://doi.org/10.2174/1385272820666161013151816 doi: 10.2174/1385272820666161013151816
![]() |
[158] |
Hunge YM, Yadav AA, Kulkarni SB, et al. (2018) A multifunctional ZnO thin film based devices for photoelectrocatalytic degradation of terephthalic acid and CO2 gas sensing applications. Sens Actuators B Chem 274: 1–9. https://doi.org/10.1016/j.snb.2018.07.117 doi: 10.1016/j.snb.2018.07.117
![]() |
[159] |
Wei Z, Liang F, Liu Y, et al. (2017) Photoelectrocatalytic degradation of phenol-containing wastewater by TiO2/g-C3N4 hybrid heterostructure thin film. Appl Catal B-Environ 201: 600–606. https://doi.org/10.1016/j.apcatb.2016.09.003 doi: 10.1016/j.apcatb.2016.09.003
![]() |
[160] |
Semalti P, Sharma V, Sharma SN (2022) A solution-route processed multicomponent Cu2ZnSn(S1−xSex)4 nanocrystals: A potential low-cost photocatalyst. J Clean Prod 365: 132750. https://doi.org/10.1016/j.jclepro.2022.132750 doi: 10.1016/j.jclepro.2022.132750
![]() |
[161] |
Zheng XL, Yang YJ, Liu YH, et al. (2022) Fundamentals and photocatalytic hydrogen evolution applications of quaternary chalcogenide semiconductor: Cu2ZnSnS4. Rare Met 41: 2153–2168. https://doi.org/10.1007/s12598-021-01955-2 doi: 10.1007/s12598-021-01955-2
![]() |
[162] |
Hunge YM, Yadav AA, Liu S, et al. (2019) Sonochemical synthesis of CZTS photocatalyst for photocatalytic degradation of phthalic acid. Ultrason Sonochem 56: 284–289. https://doi.org/10.1016/j.ultsonch.2019.04.003 doi: 10.1016/j.ultsonch.2019.04.003
![]() |
[163] |
Tan Y, Lin ZQ, Ren WH, et al. (2012) Facile solvothermal synthesis of Cu2SnS3 architectures and their visible-light-driven photocatalytic properties. Mater Lett 89: 240–242. https://doi.org/10.1016/j.matlet.2012.08.117 doi: 10.1016/j.matlet.2012.08.117
![]() |
[164] |
Kush P, Deori K, Kumar A, et al. (2015) Efficient hydrogen/oxygen evolution and photocatalytic dye degradation and reduction of aqueous Cr(vi) by surfactant free hydrophilic Cu2ZnSnS4 nanoparticles. J Mater Chem A 3: 8098–8106. http://doi.org/10.1039/C4TA06551D doi: 10.1039/C4TA06551D
![]() |
1. | Selenne Banuelos, Hayriye Gulbudak, Mary Ann Horn, Qimin Huang, Aadrita Nandi, Hwayeon Ryu, Rebecca Segal, 2021, Chapter 6, 978-3-030-57128-3, 111, 10.1007/978-3-030-57129-0_6 | |
2. | Danfeng Pang, Yanni Xiao, Xiao-Qiang Zhao, A cross-infection model with diffusive environmental bacteria, 2022, 505, 0022247X, 125637, 10.1016/j.jmaa.2021.125637 | |
3. | Iqbal Ahmad, Hesham A. Malak, Hussein H. Abulreesh, Environmental antimicrobial resistance and its drivers: a potential threat to public health, 2021, 27, 22137165, 101, 10.1016/j.jgar.2021.08.001 | |
4. | Mohammad Irfan, Alhomidi Almotiri, Zeyad Abdullah AlZeyadi, Antimicrobial Resistance and Its Drivers—A Review, 2022, 11, 2079-6382, 1362, 10.3390/antibiotics11101362 | |
5. | Danfeng Pang, Yanni Xiao, Xiao-Qiang Zhao, A cross-infection model with diffusion and incubation period, 2022, 27, 1531-3492, 6269, 10.3934/dcdsb.2021316 | |
6. | Suttikiat Changruenngam, Charin Modchang, Dominique J. Bicout, Modelling of the transmission dynamics of carbapenem-resistant Klebsiella pneumoniae in hospitals and design of control strategies, 2022, 12, 2045-2322, 10.1038/s41598-022-07728-w | |
7. | Cara Jill Sulyok, Lindsey Fox, Hannah Ritchie, Cristina Lanzas, Suzanne Lenhart, Judy Day, Mathematically modeling the effect of touch frequency on the environmental transmission of Clostridioides difficile in healthcare settings, 2021, 340, 00255564, 108666, 10.1016/j.mbs.2021.108666 | |
8. | Kamal Raj Acharya, Jhoana P Romero-Leiton, Elizabeth Jane Parmley, Bouchra Nasri, Identification of the elements of models of antimicrobial resistance of bacteria for assessing their usefulness and usability in One Health decision making: a protocol for scoping review, 2023, 13, 2044-6055, e069022, 10.1136/bmjopen-2022-069022 | |
9. | Shyni Unni Kumaran, Lavanya Rajagopal, Manavaalan Gunasekaran, Sensitivity assessment of optimal control strategies and cost-effectiveness analysis of a novel Candida Auris environmental transmission model in intensive care facilities, 2024, 595, 00225193, 111931, 10.1016/j.jtbi.2024.111931 | |
10. | Reuben Iortyer Gweryina, Muhammadu Yahaya Kura, Timothy Terfa Ashezua, Optimal control model for the infectiology of staphylococcus aureus with dual transmission pathways, 2024, 14, 26667207, 100364, 10.1016/j.rico.2023.100364 | |
11. | Bilal Aslam, Rubab Asghar, Saima Muzammil, Muhammad Shafique, Abu Baker Siddique, Mohsin Khurshid, Muhammad Ijaz, Muhammad Hidayat Rasool, Tamoor Hamid Chaudhry, Afreenish Aamir, Zulqarnain Baloch, AMR and Sustainable Development Goals: at a crossroads, 2024, 20, 1744-8603, 10.1186/s12992-024-01046-8 | |
12. | Saeed Shafait, Shazia Nisar, Kinza Nawabi, Hassan Riaz, Ayesha Masood, Mehtab Ahmed, Comparison of Frequency of Pathogenic Micro-Organisms Causing Bloodstream Infections in Patients Admitted at Tertiary Care Hospital Rawalpindi, 2024, 2790-9352, 115, 10.54393/pjhs.v5i07.1435 | |
13. | Danfeng Pang, Yanni Xiao, Xiao-Qiang Zhao, A periodic reaction-diffusion model of hospital infection with crowding effects, 2024, 539, 0022247X, 128487, 10.1016/j.jmaa.2024.128487 |
Symbol | Description | Value | Reference |
ϵ0 | Antibiotic prescription rate (day−1) | 0.12 | [39][41] |
ϵ1 | Magnitude of change of antibiotic prescription rate (no dimension) | 0.25 | [56] |
θu | Proportion of Pu on admission (day−1) | 0.617 | [15][39] |
θuA | Proportion of PuA on admission (day−1) | 0.349 | [15][40] |
θc | Proportion of Pc on admission (day−1) | 0.003 | [15][39] |
θcA | Proportion of PcA on admission (day−1) | 0.031 | [15][40] |
γu | Discharge rate of Pu (day−1) | 0.2 | [15] |
γuA | Discharge rate of PuA (day−1) | 0.2 | [15] |
γc | Discharge rate of Pc (day−1) | 0.06 | [39] |
γcA | Discharge rate of PcA (day−1) | 0.055 | [15][39] |
γb | Disinfection (cleaning) rate of environment (day−1) | 0.7 | [25] |
αp | Contact rate (day−1 person−1) | 0.0435 | [25] |
βp | Probability of colonization for Pu after a contact with Hc (no dimension) | 0.42 | [25] |
βpA | Probability of colonization for PuA after a contact with Hc (no dimension) | 0.42*1.67 | [39][15] |
βh | Probability of contamination for HCW after a contact with Pc (no dimension) | 0.2 | [25][15] |
βhA | Probability of contamination for HCW after a contact with PcA (no dimension) | 0.25 | [15] |
η | Hand hygiene compliance with HCWs (no dimension) | 0.4 | [25] |
μc | Decontamination rate of HCWs (day−1) | 24 | [25] |
υp | Shedding rate to environment from Pc (day−1 person−1 ACC/cm2) | 235 | [25] |
υpA | Shedding rate to environment from PcA (day−1 person−1 ACC/cm2) | 470 | [26][39] |
υh | Contamination rate to environment by Hc (day−1 person−1 ACC/cm2) | 235 | [25] |
κp | Colonization rate from environment for Pu (day−1 (ACC/cm2)−1) | 0.000004 | [25] |
κpA | Colonization rate from environment for PuA (day−1 (ACC/cm2)−1) | 0.000005 | [15][25] |
κh | Colonization rate from environment for Hu (day−1 (ACC/cm2)−1) | 0.00001 | [25] |
Np | Total number of patients | 23 | [25] |
Nh | Total number of HCWs | 23 | [25] |
Symbol | Distribution | reference |
γcA | N(0.055,0.005) | estimated by [26] |
γb | N(0.7,0.2) | estimated by [26] |
βpA | N(0.43,0.1) | estimated by [26] |
βhA | N(0.2,0.05) | estimated by [26] |
η | N(0.4,0.1) | estimated by [26] |
μc | N(24,5) | estimated by [26] |
υpA | N(470,150) | estimated by [26] |
ϵ | N(0.12,0.02) | estimated by [26] |
κpA | N(0.000005,0.0000006) | estimated by [26] |
Symbol | Description | Value | Reference |
ϵ0 | Antibiotic prescription rate (day−1) | 0.12 | [39][41] |
ϵ1 | Magnitude of change of antibiotic prescription rate (no dimension) | 0.25 | [56] |
θu | Proportion of Pu on admission (day−1) | 0.617 | [15][39] |
θuA | Proportion of PuA on admission (day−1) | 0.349 | [15][40] |
θc | Proportion of Pc on admission (day−1) | 0.003 | [15][39] |
θcA | Proportion of PcA on admission (day−1) | 0.031 | [15][40] |
γu | Discharge rate of Pu (day−1) | 0.2 | [15] |
γuA | Discharge rate of PuA (day−1) | 0.2 | [15] |
γc | Discharge rate of Pc (day−1) | 0.06 | [39] |
γcA | Discharge rate of PcA (day−1) | 0.055 | [15][39] |
γb | Disinfection (cleaning) rate of environment (day−1) | 0.7 | [25] |
αp | Contact rate (day−1 person−1) | 0.0435 | [25] |
βp | Probability of colonization for Pu after a contact with Hc (no dimension) | 0.42 | [25] |
βpA | Probability of colonization for PuA after a contact with Hc (no dimension) | 0.42*1.67 | [39][15] |
βh | Probability of contamination for HCW after a contact with Pc (no dimension) | 0.2 | [25][15] |
βhA | Probability of contamination for HCW after a contact with PcA (no dimension) | 0.25 | [15] |
η | Hand hygiene compliance with HCWs (no dimension) | 0.4 | [25] |
μc | Decontamination rate of HCWs (day−1) | 24 | [25] |
υp | Shedding rate to environment from Pc (day−1 person−1 ACC/cm2) | 235 | [25] |
υpA | Shedding rate to environment from PcA (day−1 person−1 ACC/cm2) | 470 | [26][39] |
υh | Contamination rate to environment by Hc (day−1 person−1 ACC/cm2) | 235 | [25] |
κp | Colonization rate from environment for Pu (day−1 (ACC/cm2)−1) | 0.000004 | [25] |
κpA | Colonization rate from environment for PuA (day−1 (ACC/cm2)−1) | 0.000005 | [15][25] |
κh | Colonization rate from environment for Hu (day−1 (ACC/cm2)−1) | 0.00001 | [25] |
Np | Total number of patients | 23 | [25] |
Nh | Total number of HCWs | 23 | [25] |
Symbol | Distribution | reference |
γcA | N(0.055,0.005) | estimated by [26] |
γb | N(0.7,0.2) | estimated by [26] |
βpA | N(0.43,0.1) | estimated by [26] |
βhA | N(0.2,0.05) | estimated by [26] |
η | N(0.4,0.1) | estimated by [26] |
μc | N(24,5) | estimated by [26] |
υpA | N(470,150) | estimated by [26] |
ϵ | N(0.12,0.02) | estimated by [26] |
κpA | N(0.000005,0.0000006) | estimated by [26] |