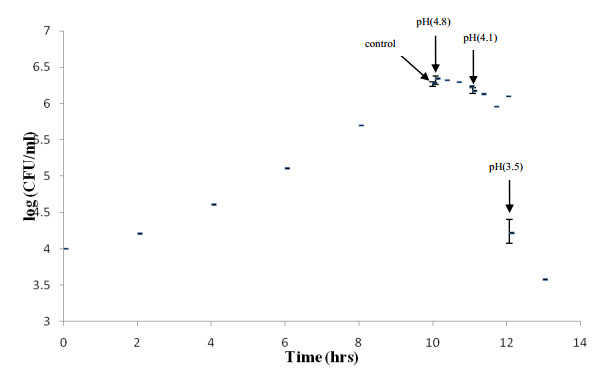
Citation: Xiaxia Kang, Jie Yan, Fan Huang, Ling Yang. On the mechanism of antibiotic resistance and fecal microbiota transplantation[J]. Mathematical Biosciences and Engineering, 2019, 16(6): 7057-7084. doi: 10.3934/mbe.2019354
[1] | Hasan Zulfiqar, Rida Sarwar Khan, Farwa Hassan, Kyle Hippe, Cassandra Hunt, Hui Ding, Xiao-Ming Song, Renzhi Cao . Computational identification of N4-methylcytosine sites in the mouse genome with machine-learning method. Mathematical Biosciences and Engineering, 2021, 18(4): 3348-3363. doi: 10.3934/mbe.2021167 |
[2] | Jianhua Jia, Mingwei Sun, Genqiang Wu, Wangren Qiu . DeepDN_iGlu: prediction of lysine glutarylation sites based on attention residual learning method and DenseNet. Mathematical Biosciences and Engineering, 2023, 20(2): 2815-2830. doi: 10.3934/mbe.2023132 |
[3] | Jianhua Jia, Yu Deng, Mengyue Yi, Yuhui Zhu . 4mCPred-GSIMP: Predicting DNA N4-methylcytosine sites in the mouse genome with multi-Scale adaptive features extraction and fusion. Mathematical Biosciences and Engineering, 2024, 21(1): 253-271. doi: 10.3934/mbe.2024012 |
[4] | Jianhua Jia, Lulu Qin, Rufeng Lei . DGA-5mC: A 5-methylcytosine site prediction model based on an improved DenseNet and bidirectional GRU method. Mathematical Biosciences and Engineering, 2023, 20(6): 9759-9780. doi: 10.3934/mbe.2023428 |
[5] | Pingping Sun, Yongbing Chen, Bo Liu, Yanxin Gao, Ye Han, Fei He, Jinchao Ji . DeepMRMP: A new predictor for multiple types of RNA modification sites using deep learning. Mathematical Biosciences and Engineering, 2019, 16(6): 6231-6241. doi: 10.3934/mbe.2019310 |
[6] | Eric Ke Wang, liu Xi, Ruipei Sun, Fan Wang, Leyun Pan, Caixia Cheng, Antonia Dimitrakopoulou-Srauss, Nie Zhe, Yueping Li . A new deep learning model for assisted diagnosis on electrocardiogram. Mathematical Biosciences and Engineering, 2019, 16(4): 2481-2491. doi: 10.3934/mbe.2019124 |
[7] | Keruo Jiang, Zhen Huang, Xinyan Zhou, Chudong Tong, Minjie Zhu, Heshan Wang . Deep belief improved bidirectional LSTM for multivariate time series forecasting. Mathematical Biosciences and Engineering, 2023, 20(9): 16596-16627. doi: 10.3934/mbe.2023739 |
[8] | Hui Li, Xintang Liu, Dongbao Jia, Yanyan Chen, Pengfei Hou, Haining Li . Research on chest radiography recognition model based on deep learning. Mathematical Biosciences and Engineering, 2022, 19(11): 11768-11781. doi: 10.3934/mbe.2022548 |
[9] | Honglei Wang, Wenliang Zeng, Xiaoling Huang, Zhaoyang Liu, Yanjing Sun, Lin Zhang . MTTLm6A: A multi-task transfer learning approach for base-resolution mRNA m6A site prediction based on an improved transformer. Mathematical Biosciences and Engineering, 2024, 21(1): 272-299. doi: 10.3934/mbe.2024013 |
[10] | Guanghua Fu, Qingjuan Wei, Yongsheng Yang . Bearing fault diagnosis with parallel CNN and LSTM. Mathematical Biosciences and Engineering, 2024, 21(2): 2385-2406. doi: 10.3934/mbe.2024105 |
As a potentially fatal food-borne pathogen, the Gram-positive bacterium Listeria monocytogenes threatens public health as well as the food industry. This pathogen is known for its ability to survive harsh environments, for instance non-growth-permissive acidic conditions including the mild fermentation–based preservation processes used for many low-pH foods, such as fermented meat, cheese, orange juice, salad dressing, and yogurt. L. monocytogenes infection can lead to gastroenteritis, meningitis, encephalitis, mother-to-fetus infections, and septicemia in immunocompromised individuals, pregnant women, and newborns, with a mortality rate of nearly 28% [1,2,3,4].
Proteomic-based approaches have been applied to better understand the mechanisms of L. monocytogenes response to a wide range of physiological stressors relevant to food preservation, processing, and sanitation of food production facilities. Stressors assessed include mineral and organic acids, salt, cold, heat, alkaline substances, detergents, oxidative chemicals, organic antimicrobials, and high hydrostatic pressure (e.g., [5,6,7]). Proteomics has revealed that cellular responses to stress can be complex. By measuring relative protein changes by methods such as iTRAQ technology, proteomic profiles can provide useful information about manifested phenotypes linked to protein function, metabolic pathways, and regulatory networks.
The response to acidic stress in isolation mainly affects the biochemical processes related to managing oxidative stress and energy production, nutrient uptake, and cell envelope integrity. The effects on cells are highly temperature-dependent [8,9,10,11]. Understanding the extent to which proteins form and how they merge to form a functional unit in order to cope with environmental stress also provides relevant information for understanding how organisms respond to different scenarios, particularly over short timescales and when in specific physiological contexts. Furthermore, the understanding of pathogen metabolism to stress can be achieved by reconstructing metabolic pathways and understanding de novo end-product metabolite formation such as lipids, proteins, and high-energy intermediates. The complexity during stress response and adaptation arises because stress-related proteins are involved in a variety of cellular activities including gene regulation, intermediary metabolism, compatible solute uptake, protein folding, ATP-dependent proteolysis, and DNA repair. Proteome data can be best interpreted to provide indications of the following: i) which responses lead to adapted physiological states (phenotypes), which requires prior knowledge of the related proteins; ii) responses that are more compensatory in nature, and so maintain the cellular status quo and not a new phenotype; and iii) the main regulatory networks, which requires knowledge of the associated regulons. Proteomic studies have shown that prior adaptation of L. monocytogenes to mild (growth-permissive) acidic conditions increases the tolerance of cells to more severe acid stress [10]. Furthermore, high but growth-permissive temperatures induce cross-protection against acidic and other stressful conditions [12,13]. In both of these situations, abundances of proteins change to ensure maximal cell wall stability, osmotic control (via osmolyte uptake transporters), cytoplasmic homeostasis, overall proteostasis (avoiding protein aggregation), and cytoplasmic membrane integrity [12]. Disruptions to energy production and depletion of cell ATP pools are typical effects of acid stress and indirectly lead to membrane and cell wall damage, uncontrolled protein aggregation, and subsequent cell death. Depending on the nature of energy availability hurdles, L. monocytogenes can compensate by increasing carbohydrate metabolism [13], while altering ATP synthesis helps to maintain some ATP. It was found that once ATP drops below a critical threshold, cells become permanently inactivated (dead).
In the present study, we aimed to identify differentially expressed proteins where L. monocytogenes was exposed to different pH during the early stationary growth phase (pH 4.8, 4.1, and 3.5, adjusted using HCl) and forced into a process leading to a state that was unrecoverable. Rapid changes in protein abundances that occur due to the imposed acid stress were quantified using iTRAQ techniques with the aim of obtaining new insights into the cellular response strategies developed by L. monocytogenes to survive in non-growth permissive low-pH stress conditions. The milder pH levels studied cross the boundary of growth permissiveness of L. monocytogenes, which grows down to pH 4.2–4.3 in rich organic media at 25 ℃ [14]. In previous studies, we observed that temperature is the main factor that governs the inactivation rate of vegetative bacteria under growth-preventing conditions [15] and that underlying this is an array of responses that are dynamic, complex, and interconnected by regulatory processes [8,10]. By examining the responses of L. monocytogenes to growth/no growth boundary pH conditions, the goal is to determine whether there is a window for protein synthesis to aid survival and also determine the extent of this capability at the lethal side of the growth/no growth boundary. There is a tendency for L. monocytogenes to show complex inactivation responses also due to inherent physiological variation in its cell populations. We have observed previously that a small proportion of cells (depending on the temperature) can survive non-growth-permissive acidic conditions potentially over an extended time. This survival increases at lower temperatures [8,15]. The experiments also seek to understand what traits may be linked to these survivor sub-populations. One major hurdle is the strain choice, as L. monocytogenes does not exhibit homogenous responses to acid stress; thus, choosing a representative strain does not cover the extent of diversity of the species [10]. To overcome this, the decision was to examine acid stress in a strain that is more vulnerable to acid chemical exposure, which is a factor that complicates acid adaptation in L. monocytogenes. The experiments were thus performed using the strain F2365. Due to stop codon mutations, this strain has a moderately impaired ability to tolerate acid and oxidative stress and also cell invasiveness [16,17]. The mutations likely weaken its cell wall envelope integrity compared with other L. monocytogenes strains such as ScottA [10]. This makes F2365 slightly more vulnerable to infiltration of protons during acid shock; otherwise, its acid tolerance response seems fully intact. This study helps to determine what other traits are key to acid stress survival, besides those that are linked to the stationary growth phase or influenced by other stress responses. Understanding the lethal and sub-lethal effects of acid stress will deepen our understanding of the survival of the pathogen L. monocytogenes in acidic foods and food production facilities. Such knowledge can be informative in developing new approaches that intervene against and control L. monocytogenes contamination in susceptible food products.
L. monocytogenes strain F2365 was routinely grown using tryptone soya broth supplemented with 0.6% yeast extract (TSB-YE, Oxoid, Beijing, China) at 37 ℃. For inactivation treatments, strains were grown to the early stationary growth phase (12 h incubation at 37 ℃ in a shaking water bath, 100 rpm) in 1 L of TSB-YE broth, and filter-sterilized HCl (pH 3.0) was gradually added to the culture; at pH 4.8, 4.1, and 3.5, samples were taken. Cultures pelleted were centrifuged and plate-counted by using brain heart infusion agar supplemented with 0.1% pyruvate (BHA-P) to determine the culturable cell population. These experiments were performed in triplicate.
We followed the method of [18]: 1 g of wet weight of cell pellet from each sample, including three independent biological replicates, were washed with phosphate-buffered saline (PBS) (pH 7.4), to remove flocculent precipitation; then, samples were dissolved in 1/10 volumes of SDT buffer (4% SDS, 100 mM DTT, and 150 mM Tris–HCl at pH 8.0). After 3 min incubation in boiling water, the suspensions were ultrasonicated with 10 rounds of 80 W sonication for 10 s with 15-s intervals. The crude extract was clarified by centrifugation at 13,000 × g at 20 ℃ for 15 min. Protein concentration was determined using the Bicinchoninic acid (BCA) Protein Assay Kit (Promega, USA), and supernatants were stored at −80 ℃ until further analysis.
Protein digestion was performed according to the filter-aided sample processing (FASP) procedure, as described previously [19]. In brief, 200 μg of proteins in each sample was added to 30 μL of SDT buffer (4% SDS, 100 mM DTT, 150 mM Tris-HCl at pH 8.0). Detergent, DTT, and other low-molecular-weight components were removed by UA buffer (8 M urea, 150 mM Tris-HCl at pH 8.0) by repeated ultrafiltration (Pall units, 10 kD). After addition of 100 μL of iodoacetamide (50 mM in UA), samples were shaken at 600 rpm for 1 min, incubated in the dark for 30 min, and then centrifuged at 13,000 × g for 10 min. Filters were washed three times with 100 μL of UA buffer and two times with 100 μL of DS buffer (50 mM triethylammonium bicarbonate at pH 8.5). Protein suspensions were digested with 2 μg of trypsin (Promega, USA) in 40 μL of DS buffer at 37 ℃ for 16 h. Resulting peptides were collected as the filtrate. Peptide concentration was determined by UV light spectral density at 280 nm from an extinction coefficient of 1.1 of 0.1% (g/L) solution.
The resulting peptide was labeled using the 4-plex iTRAQ reagent according to the manufacturer's instructions (Applied Biosystems, California, USA). Each iTRAQ reagent was dissolved in 70 μL of ethanol and added to the respective peptide mixture. Samples were then labeled as (C)-113, (T1)-114, (T2)-115, or (T3)-116, with three independent biological replicates. Samples were incubated for 2 h at room temperature and, after terminating the labeling reaction, the labeled samples were mixed and vacuum dried.
The labeled samples were separated by SCX chromatography using the AKTA Purifier system (GE Healthcare, Fairfield, CT, USA). Briefly, the dried peptide mixture was reconstituted in 2 mL of buffer A (10 mM KH2PO4 in 25% of CAN at pH 2.7) and loaded onto a PolySULFOETHYL 4.6 × 100 mm column (5 µm, 200 Å, PolyLC Inc, Maryland, USA). The peptides were then eluted at a flow rate of 0.9 mL/min with a gradient of 0%–10% buffer B (500 mM KCl, 10 mM KH2PO4 in 25% of CAN at pH 2.7) from 0 to 2 min, 10%–20% buffer B until 27 min, 20%–45% buffer B until 32 min, and 50%–100% buffer B until 37 min. The elution was monitored at 214 nm, and fractions were collected every minute for a total of 35 fractions. The collected fractions were then combined into 10 pools and desalted on C18 cartridges (66872-U, Sigma, USA). Each fraction was vacuum freeze dried by vacuum centrifugation and reconstituted in 40 µL of 0.1% (v/v) trifluoroacetic acid.
Finally, all samples were kept at −80 ℃ until further analysis.
Experiments were analyzed on a Q Exactive mass spectrometer coupled to an Easy nLC system (Thermo Fisher Scientific, Waltham, MA, USA). For nano LC-MS/MS analysis, 6 μL of each fraction was injected. The peptide (5 μg) was loaded onto a Thermo Scientific EASY column (2 cm × 100 mm, 5 mm C18) using an autosampler. Peptides were eluted onto an analytical Thermo Scientific EASY column (75 mm × 100 mm, 3 mm C18) and separated with a linear gradient of buffer B (0.1% formic acid and 80% acetonitrile) at a flow rate of 250 nL/min over 60 min.
Mass spectrometry data were obtained in peptide recognition mode using a survey scan of 300–1800 m/z for the 10 most abundant precursor ions after high-energy collision disfragmentation.
The duration of the dynamic exclusion was 50 s. The measured scans had a resolution of 70,000 at m/z 200 and the resolution for HCD spectra was set at 17,500 at m/z 200. The normalized collision energy was 30 eV, and the underfill ratio was defined as 0.1%. The instrument was run with the peptide recognition mode enabled.
The raw data of mass spectrometry were searched using MASCOT engine (Matrix Science, London, UK; version 2.2). The MASCOT engine was embedded into Proteome Discoverer 1.4 (Thermo Electron, San Jose, CA) against the UniProt Listeria database (268,763 sequences, download at 08/08/2014) and the Decoy database. The following search parameters were used for protein identification: peptide mass tolerance 20 ppm, MS/MS tolerance 0.1 Da, enzyme trypsin, missed cleavage 2, fixed modified carbamidomethyl (C), iTRAQ 4-plex (K), and iTRAQ 4-plex (N-term), variable modification oxidation (M). All reported data were based on a false discovery rate (FDR) of less than 1% confidence for protein identification.
The Gene Ontology (GO) program Blast2GO (https://www.blast2go.com/) was used to annotate differential expression proteins (DEPs) to create histograms of GO annotation, including biological process, cellular component, and molecular function [20,21]. For pathway analysis, the DEPs were mapped to the terms from KEGG (Kyoto Encyclopedia of Genes and Genomes) [22] database using the KAAS program (http://www.genome.jp/kaas-bin/kaas_main). Protein–protein interaction networks were analyzed using the publicly available program STRING (http://string-db.org/) with the required minimum interaction score set to 0.400.
To further check the levels of protein expression gained through label-free analysis, additional quantification through LC-PRM MS analysis [23] was applied. Briefly, the iTRAQ protocol was used for peptide preparation. The stable isotope AQUA peptide was spiked in each sample and used as a standard internal reference. Tryptic peptides were loaded on stage tips of C18 for desalting prior to reversed-phase chromatography on one of the nLC-1200 easy systems (Thermo Scientific). Then, 1 h liquid chromatography gradients were performed with 5%–35% acetonitrile for 45 min. Q Exactive MS was applied for PRM analysis. Optimized methods for measuring the energy of collision, state of charge, and retention time of the most crucial peptides were gained from experiments involving unique peptides with high intensities; therefore, each targeted protein could be handled properly. The analysis of raw data was realized via Skyline (MacCoss Lab, University of Washington) [24], wherein the intensity of signal produced by a certain peptide sequence could be quantified with respect to each sample and referenced to standards via normalization for each protein.
Previous studies have performed proteomics analyses on L. monocytogenes exposed to single- and multi-stress (e.g., acid and salt) conditions. Acid stress is imposed by exposure to mineral acids, organic acids, or both [6,7,8,10,12,25,26,27]. However, extending from these studies, the proteome of L. monocytogenes in response to progressive mineral acid exposure in a time course experiment focusing on the strain F2365 provides an opportunity to explore the boundaries of acid stress survival in tandem with relatively rapid inactivation (cell death). F2365, a 4b serotype (lineage I) strain as tested here has a reduced ability to tolerate acid stress due to stop codon mutations [17]. Research performed on other strains, such as ScottA (4b serotype strain), shows that they can mount an extensive defense to acidic conditions, even with other stressors present (such as extreme osmotic stress), depending on the ambient temperature [8]. The experiment thus allows a comparison to "tough" strains, such as ScottA. The experiments utilized stationary growth phase cells. In that respect, standard defenses arising from the general stress response, induced when entering the stationary growth phase—which included heat shock proteins and oxidative stress management proteins—would be maximally activated, as these were not detected in the iTRAQ data obtained here. What is shown here are the specific responses to non-thermal stress above and beyond stress responses that enable rapid adaptation and cross-protection in L. monocytogenes, critical for host and external environmental survival. The focus of the experimental strategy is placed on responses that essentially precede a permanent inactivation state. It is important to note that studying a single strain will not capture the extent of responses that may occur in the full diversity of L. monocytogenes. Rather, the observations here are specifically targeted to explore responses of the highly studied species and relatively genetically diverse L. monocytogenes to inactivation scenarios, which we have previously explored [8,28] to better understand food-borne pathogen death kinetics and its underlying physiological and molecular basis.
The complex effects of organic and mineral acids on cell survival and gene expression response, underlying inactivation that is non-linear (as described extensively in [8]), seem to relate to the ability of cell populations to maintain cytoplasmic membrane integrity and thus cytoplasm homeostasis. The loss of integrity in the cell wall and cytoplasm is highlighted by a depletion of cytoplasmic ATP accompanied by a decrease in the intracytoplasmic pH [29]. The rapidity of these changes and the associated rate of cellular inactivation can affect the ability to detect changes in protein expression due to loss of proteostasis, protein aggregation, and collapse of the intricate networks of protein interactions enabling life [30,31]. Proteostasis is the basic function in cells that maintains protein integrity by rescuing and refolding proteins or disposing of proteins that cannot be recovered. This is an energy-intensive process [32], and when it fails it heralds the inevitable death of the cell. In the experiments here, we assume this process is at its limit since there is likely no ATP to spare, and abundances of proteins involved in proteostasis are at maximal levels. Thus, slow changes in pH were used to avoid the rapid collapse of F2365's ability to cope with acid stress. Even extreme acidophiles, which possess multiple copies of highly conserved proteins involved in key steps of protein disaggregation and proteostasis (such as DnaK, DnaJ, GroEL/GroES, ClpP, Lon, FtsH, HtrA, Hsp20, SurA, and RidA) possess an upper boundary for resilience to protons infiltrating the cytoplasm, indicative of a hard boundary for life in this respect [33].
To ensure the accuracy of the proteome information, the F2365 cell viability was enumerated at each sampling time, and culture purity was confirmed before proteomics analysis as shown in Figure 1. The mean viability of early stationary growth phase L. monocytogenes F2365 was 6.27 log CFU/mL. Cells were then exposed to a solution of pH 3.0 (HCl). At 5 min sampling at pH 4.8, the mean viability was 6.34 log CFU/mL; at the 1 h sampling time, pH had dropped to 4.1 and the mean count was 6.16 log CFU/mL. Further HCl addition reduced the pH to 3.5 by the 2 h mark, well below the point of growth permissiveness [14]. At this point, inactivation was rapid with the mean viability declining almost 2.06 log units to 4.21 log CFU/mL. For the sample point at pH 4.8, changes in population viability were negligible, while at pH 4.1 the 0.2 log unit viability loss (compared to the 0 time control) appears to herald that cell decline was starting to occur. The inactivation process (death kinetics) requires time, and it is assumed that most of the viability loss occurs once a boundary of cellular acid tolerance has passed. At 37 ℃, this appears to be around pH 4.2 [14]; thus at 1 h the inactivation was only just beginning, and between 1 h and 2 h the rate of death had likely become log-linear. This would be consistent with previous findings [8,15], where the size and continued viability of the cell population depends on the temperature during inactivation and the loss rate accelerates with temperature increase.
To better understand the underlying response mechanisms of L. monocytogenes to progressive acidic shock, this study used iTRAQ technology to examine genetic and protein changes in L. monocytogenes from the same samples described above to determine their viability. The raw data were then analyzed using MASCOT, and the proteins were quantified. From the iTRAQ 4x-plex experiments, a total of 2131 proteins were identified. Of these, 291 proteins generated had a significant difference in abundance (≥ 1.20 or ≤ 0.83 fold change, p < 0.01), while 90 proteins were in the range ≥ 1.50 or ≤ 0.67 fold change. A Venn diagram shows the details of the overlap between treatments and the proteins unique to each processed dataset (Figure 2A). A total of 15 proteins significantly changed in abundance at all treatment points. Table 1 lists the proteins showing both significant (p < 0.01) and sizable changes (≥ 1.50 or ≤ 0.67 fold change). Enrichment analysis of these proteins based on GO terms is shown in Figure 2B. Among the proteins that underwent significant changes, the GO terms of level 2 associated with 34 GO categories were found. The 14 GO term groups were based on derived biological processes, including metabolic, cellular, and single-biological processes. Effects could be linked to cellular components, mainly including the whole cell, cell wall, and cell membranes, as well as to molecular functions, including catalytic activity and binding. These results suggest that responses are quite global in nature, affecting many aspects of the cell down to specific enzyme functions.
F2365 Loci | EGD-e gene name | pH 4.8/C | Log ratio pH 4.1/C | pH 3.5/C | Known or predicted specific function |
LMOf2365_2631 | lmo1034 | 0.94 | 1.32 | 2.43 | glycerol kinase |
LMOf2365_0212 | plcA | 1.04 | 1.65 | 2.15 | phosphatidylinositol-specific phospholipase C PlcA |
LMOf2365_0148 | lmo0130 | 1.02 | 1.15 | 1.61 | putative cell surface anchored 5'-nucleotidase |
LMOf2365_0136 | lmo0118 | 1.13 | 1.84 | 2.09 | putative phage tail protein, phage_tail_2_superfamily; antigen A protein LmaA |
LMOf2365_0941 | lmo0919 | 0.97 | 0.97 | 1.68 | putative ABC transporter, ATP-binding protein |
LMOf2365_1771 | lmo1746 | 0.93 | 0.87 | 0.57 | putative bacitracin (drug) efflux ABC transporter, permease protein |
LMOf2365_2553 | lmo2581 | 1 | 1.06 | 2.59 | MacB-like ABC transporter, ATP-binding protein |
LMOf2365_2810 | lmo2819 | 1.02 | 1 | 1.63 | putative N-acyl-L-amino acid amidohydrolase, M20_dimer superfamily |
LMOf2365_1535 | lmo1516 | 1 | 1.08 | 1.92 | ammonium uptake transporter |
LMOf2365_1653 | trpD | 1.03 | 1.08 | 1.7 | anthranilate phosphoribosyltransferase |
LMOf2365_0213 | hly | 0.97 | 1.88 | 3.24 | membrane protein, HlyIII superfamily |
LMOf2365_2165 | lmo2131 | 0.94 | 0.77 | 2.38 | putative Crp-like transcriptional regulator, CAP_ED superfamily |
LMOf2365_2394 | lmo2423 | 1.02 | 1.01 | 1.58 | putative metal cation exporter, cation_efflux superfamily |
LMOf2365_2694 | lmo2714 | 1.11 | 1.55 | 2.8 | putative cell wall sorted protein |
LMOf2365_1723 | lmo1699 | 0.67 | 0.65 | 0.65 | methyl-accepting chemotaxis protein |
LMOf2365_0722 | lmo0686 | 0.79 | 0.94 | 1.61 | flagellar motor rotation protein B |
LMOf2365_2440 | lmo2467 | 0.93 | 1.29 | 3.44 | putative chitin binding protein, chitin_bind_3/FN3/chtBD3 superfamily |
LMOf2365_0295 | lmo0275 | 0.92 | 2.38 | 3.22 | putative metallo-beta-lactamase superfamily protein |
LMOf2365_2166 | lmo2132 | 0.77 | 0.79 | 0.62 | putative Crp-like transcriptional regulator, CAP_ED superfamily |
LMOf2365_1718 | lmo1694 | 1.2 | 1.09 | 3.46 | putative nucleoside-diphosphate sugar epimerase, NADB_Rossmann superfamily |
LMOf2365_1193 | lmo1183 | 1.25 | 1.4 | 3.48 | putative ethanolamine utilization-associated protein |
LMOf2365_0726 | flaA | 0.33 | 0.36 | 0.54 | flagellin Fla |
LMOf2365_1816 | azoR2 | 0.9 | 0.93 | 2.05 | NADH-azoreductase, FMN-dependent |
LMOf2365_0948 | lmo0927 | 1.01 | 1.98 | 3.64 | exported glycerolphosphate lipoteichoic acid synthetase |
LMOf2365_0334 | thiM | 1.09 | 1.29 | 1.57 | hydroxyethylthiazole kinase |
LMOf2365_0471 | inlA | 1.1 | 1.25 | 1.68 | internalin A; cell wall protein with LRR and B repeat domains InlA |
LMOf2365_2282 | lmo2249 | 1.09 | 1.06 | 1.66 | low-affinity inorganic phosphate transporter |
LMOf2365_1812 | inlB | 1.01 | 2.29 | 4.44 | unanchored cell wall internalin B InlB |
LMOf2365_0283 | inlE | 1.03 | 1.17 | 2.33 | internalin E |
LMOf2365_2178 | lmo2146 | 0.96 | 1.01 | 0.65 | putative transcriptional regulator, PBP2_CysL_like family |
LMOf2365_0932 | lmo0910 | 0.97 | 0.97 | 1.86 | DUF1648 superfamily protein |
LMOf2365_2272 | lmo2239 | 0.96 | 1.04 | 1.73 | uncharacterized protein |
LMOf2365_0139 | lmo0121 | 1.13 | 1.36 | 2.18 | putative phage-associated membrane protein, COG5412 family |
LMOf2365_0214 | mpl | 0.99 | 1.41 | 2.11 | putative L-lactate dehydrogenase |
LMOf2365_0125 | lmo0107 | 1.19 | 1.22 | 3.68 | putative export ABC transporter, ATP-binding/permease |
LMOf2365_1540 | lmo1521 | 1.01 | 1.54 | 2.53 | N-acetylmuramoyl-L-alanine amidase |
LMOf2365_1225 | lmo1216 | 1.01 | 1.89 | 3.11 | exoglucosaminidase/muramidase |
LMOf2365_2568 | lmo2595 | 1.03 | 1.51 | 2.62 | uncharacterized protein |
LMOf2365_1900 | 1.03 | 1.94 | 3.39 | uncharacterized protein | |
LMOf2365_0570 | lmo0541 | 1.02 | 1.23 | 2.5 | iron complex uptake ABC transporter, substrate binding protein FecB-like |
LMOf2365_0145 | lmo0127 | 1.24 | 1.54 | 1.91 | putative phage protein (Gp20 Listeria phage A118) |
LMOf2365_0667 | 1.03 | 2.04 | 3.86 | uncharacterized protein | |
LMOf2365_0162 | lmo0146 | 1.25 | 1.36 | 1.82 | uncharacterized protein |
LMOf2365_0144 | lmo0126 | 1.17 | 1.52 | 1.44 | putative phage protein |
LMOf2365_2195 | lmo2163 | 1.02 | 1.02 | 0.63 | putative dehydrogenase, GFO_IDH_MocA superfamily |
LMOf2365_1457 | pbpA | 1 | 1.23 | 1.68 | septal peptidoglycan transpeptidase |
LMOf2365_2071 | pbpB | 1.02 | 1.53 | 1.94 | septal peptidoglycan transpeptidase |
LMOf2365_0569 | lmo0540 | 1.09 | 1.39 | 2.24 | cell division–associated peptidoglycan endopeptidase |
LMOf2365_2477 | lmo2504 | 0.99 | 2.3 | 3.55 | putative murein endopeptidase |
LMOf2365_2478 | lmo2505 | 0.98 | 2.31 | 3.81 | peptidoglycan DL-endopeptidase P45 |
LMOf2365_0406 | lmo0394 | 0.89 | 1.35 | 2.07 | Nlp/60 family protein |
LMOf2365_0611 | iap | 0.99 | 1.25 | 5.56 | gamma-D-glutamate-meso-diaminopimelate muropeptidase; invasion-associated protein Iap |
LMOf2365_2472 | lmo2499 | 0.98 | 1.16 | 1.88 | phosphate ABC transporter, substrate binding protein |
LMOf2365_0216 | plcB | 1.05 | 1.65 | 2.66 | zinc-dependent phospholipase C PlcB |
LMOf2365_2620 | lmo2648 | 1.27 | 1.39 | 1.69 | putative phosphotriesterase, metallo-dependent hydrolase superfamily |
LMOf2365_1825 | lmo1798 | 0.96 | 1.39 | 6.27 | putative poly (glycerol-phosphate) alpha-glucosyltransferase |
LMOf2365_1202 | cobH | 0.93 | 1.58 | 0.41 | precorrin-8X methylmutase CobH |
LMOf2365_0016 | qoxA | 0.98 | 1.48 | 2.18 | AA3-600 quinol oxidase subunit II |
LMOf2365_2139 | lmo2106 | 1.19 | 1.09 | 1.67 | putative phosphohydrolase, MPP_YkuE_C family protein |
LMOf2365_2615 | lmo2642 | 1.2 | 1.35 | 1.7 | putative metal-binding phosphohydrolase, Icc superfamily |
LMOf2365_0675 | lmo0644 | 1.01 | 1.84 | 3.07 | putative sulfatase or phosphoglycerol transferase, alkaline phosphatase superfamily |
LMOf2365_2208 | lmo2176 | 1 | 0.97 | 0.53 | putative ArcR-like transcriptional regulator, TetR_N superfamily |
LMOf2365_2420 | lmo2477 | 0.83 | 0.97 | 0.6 | putative transcriptional regulator, HTH_XRE/Rgg_CTERM superfamily |
LMOf2365_0288 | lmo0269 | 0.92 | 1.03 | 2.95 | putative ABC transporter, permease |
LMOf2365_1777 | lmo1752 | 1.02 | 1.23 | 2.49 | uncharacterized secreted protein |
LMOf2365_1223 | lmo1214 | 0.92 | 0.95 | 0.63 | DUF2812 superfamily protein |
LMOf2365_1350 | lmo1333 | 1.2 | 1.37 | 2.83 | putative secreted protein, YceG-like superfamily |
LMOf2365_0135 | lmo0117 | 1.17 | 1.43 | 1.73 | putative phage protein; Antigen B protein LmaB |
LMOf2365_1077 | lmo1056 | 1.13 | 1.12 | 1.51 | uncharacterized protein |
LMOf2365_2689 | lmo2709 | 1.07 | 1.3 | 1.51 | uncharacterized protein |
LMOf2365_0134 | lmo0116 | 1.24 | 1.41 | 1.73 | putative phage transcriptional activator, phage_ArpU superfamily; antigen C protein LmaC |
LMOf2365_0197 | lmo0186 | 1.01 | 2.38 | 3.63 | putative resuscitation-promoting factor/stationary-phase survival autolysin, Rpf/Sps_SpsB subfamily |
LMOf2365_0254 | lmo0242 | 1.18 | 1.12 | 1.59 | DUF901 superfamily protein with PIN domain |
LMOf2365_2813 | lmo2822 | 0.98 | 0.9 | 1.67 | uncharacterized protein |
LMOf2365_0379 | lmo0359 | 0.9 | 1.42 | 2.89 | putative fructose-1, 6, -bisphosphate aldolase |
LMOf2365_2769 | lmo2778 | 0.99 | 1.23 | 0.57 | uncharacterized protein |
LMOf2365_0143 | lmo0125 | 1.28 | 1.62 | 1.94 | putative phage protein |
LMOf2365_2693 | lmo2713 | 1 | 1.93 | 3.08 | secreted protein, COG3786 superfamily |
LMOf2365_2188 LMOf2365_0431 LMOf2365_0037 LMOf2365_2564 LMOf2365_0346 LMOf2365_0140 LMOf2365_0900 LMOf2365_0428 LMOf2365_2387 LMOf2365_1130 LMOf2365_1885 LMOf2365_1590 |
lmo2156 lmo0412 lmo2591 lmo0328 lmo0122 lmo0881 lmo0408 lmo2416 lmo1124 lmo1857 lmo1568 |
0.97 0.97 1.03 0.91 0.92 1.17 0.98 1.04 1.06 1.04 0.99 1.07 |
2.14 1.63 1.9 1.86 1.11 1.43 1.39 1.31 1.26 0.94 1.03 1.12 |
3.58 3.65 3.89 3.96 0.62 1.73 1.8 1.82 2 2.5 2.08 1.53 |
YxeA family (DUF1093 superfamily) protein uncharacterized membrane-associated secreted protein uncharacterized protein exoglucosaminidase/muramidase uncharacterized protein putative phage tail protein, sipho_tail superfamily uncharacterized secreted protein DUF1312 superfamily secreted protein uncharacterized secreted protein uncharacterized protein DUF1250 (COG4479) superfamily protein DUF441 superfamily protein |
Note: When p value < 0.05, log ratio values are in bold type. |
In Figure 3, which shows GO terms grouped by timepoint, and in Figure 4, which is a heat map showing specific protein responses, it is visible that the most dramatic changes in protein abundances occurred at the time point (2 h) at which pH 3.5 was achieved. This includes proteins having reduced and increased abundance, with a skew to the latter (Figure 4). By comparison, very few proteins change with pH 4.8, and a moderate number change at pH 4.1. The results demonstrate that F2365, even though lacking an ATR, still mounts a response to acid stress and has an inherent acid tolerance up to around pH 4.1. Clearly beyond the range of growth-permissiveness, this capacity collapses and, from former studies, inevitably leads to cell death [8].
In order to reveal the interaction network associated with low pH stresses towards early stationary phase L. monocytogenes F2365, a protein–protein interaction (PPI) network was constructed using the STRING protein–protein interaction database, KEGG pathway, and GO biological process analyses (Supplementary Figure S1). Protein names were represented by the names or locus numbers of the homologous proteins in L. monocytogenes EGD-e in the PPI map and were included in Table 1. The nodes of the network show proteins that interact over 14 categories, including transcriptional regulation, ribosomal proteins, nucleic acid/nucleotide metabolism, amino acid–related metabolism, ABC-type transporters, central glycolytic/intermediary pathways, lipid-related metabolism, membrane bioenergetics, prophage genome, phosphotransferase systems, cell wall biogenesis, and other transporter proteins, as well as proteins that have a function that is only a generalized prediction (based on conserved domains) or that is uncharacterized.
The proteomic results were in accordance with the verification analysis by parallel reaction monitoring (PRM) assays (Table 2). The expression levels of 15 candidate proteins with potential salt-tolerant functions were chosen for quantification by PRM to verify the iTRAQ results. The Pearson correlation coefficient between iTRAQ and PRM results was R2 = 0.67 (p < 0.01), showing that these results had a significant correlation. In general, all proteins detected by PRM had changes that were consistent with the iTRAQ quantification results. Our PRM assay illustrated that iTRAQ results were credible as a means of analysis.
F2365 Loci | Gene name | Log ratio pH 4.8/C | iTRAQ/PRM pH 4.1/C | Results pH 3.5/C | Known or predicted specific function |
LMOf2365_2702 | lmo1671 | 0.98/0.60 | 0.96/0.50 | 0.75/0.43 | zinc uptake ABC transporter, substrate-binding protein |
LMOf2365_1520 | lmo1501 | 0.94/0.59 | 0.90/0.41 | 0.75/0.32 | DUF1292 superfamily protein |
LMOf2365_0912 | rsbV | 0.98/0.64 | 0.93/0.56 | 0.80/0.54 | positive (anti-sigma B) regulatory factor (acts against RsbW) |
LMOf2365_1561 | rplU | 0.96/0.63 | 0.92/0.55 | 0.80/0.50 | ribosomal protein L21 |
LMOf2365_2532 | lmo2560 | 0.89/0.73 | 0.94/0.89 | 0.81/0.71 | RNA polymerase, delta subunit |
LMOf2365_1579 | engB | 0.98/0.73 | 0.97/0.68 | 0.81/0.55 | ribosome assembly GTP-binding protein |
LMOf2365_2603 | rplW | 0.94/0.70 | 0.94/0.60 | 0.81/0.58 | ribosomal protein L23 |
LMOf2365_2148 | lmo2115 | 0.97/0.66 | 0.93/0.59 | 0.82/0.65 | putative drug/toxic substance efflux ABC transporter, permease protein |
LMOf2365_1708 | lmo1684 | 0.93/0.68 | 0.90/0.51 | 0.82/0.57 | putative D-isomer 2-hydroxy acid dehydrogenase, NADB_Rossmann superfamily |
LMOf2365_0140 LMOf2365_0136 LMOf2365_0213 LMOf2365_0948 LMOf2365_2478 LMOf2365_0611 |
lmo0122 lmaA lmo0202 lmo0927 lmo2505 iap |
1.17/1.27 1.13/1.24 0.97/0.64 1.01/1.09 0.98/0.62 0.99/0.95 |
1.43/1.70 1.84/4.05 1.88/1.89 1.98/17.47 2.31/6.62 1.25/1.17 |
1.73/2.36 2.09/4.97 3.24/2.64 3.64/45.00 3.81/18.86 5.56/11.32 |
putative phage tail protein, sipho_tail superfamily putative phage tail protein, phage_tail_2_superfamily; antigen A protein LmaA listeriolysin O LLO exported glycerolphosphate lipoteichoic acid synthetase peptidoglycan DL-endopeptidase P45 gamma-D-glutamate-meso-diaminopimelate muropeptidase; invasion-associated protein Iap |
Note: When p value ﹤ 0.05, log ratio value are in bold type. |
Changes in protein abundance due to acidic stresses were determined by comparing the profiles obtained after the shift to that of a reference sample taken prior to the shift. Table 1 and Figure 4 include complete lists of the number of proteins with significant abundance changes from each treatment. To investigate a possible interdependence of the proteins within functional groups and to visualize their expression patterns under low pH stress, we performed hierarchical clustering to find associated changes in the acid-responsive proteins as indicated in Table 1 and Figure 4. Two main clusters were observed in this cluster tree on the left side in Figure 4. It was found that the proportions for upregulated proteins were greater than for downregulated proteins. The progressive sub-lethal to lethal acidic shock, even without an overlayed ATR, results in a response involving a dispersed range of proteins (Table 1, Figures 3 and 4) and affects multiple metabolic pathways (summarized in Figure S2). In Figure 3, several functional categories are enriched with proteins with significant abundance changes. Broadly, these proteins include ABC-type transporters involved in specific aspects of amino acid and carbohydrate-related metabolism, cell surface proteins/internalins including several proteins of the main pathogenicity island LIP1 (Hyl, Mpl, PlcB) as well as InlA and InlC (but not InlB, the gene for which is a pseudogene in F2365), cell wall biogenesis and co-factor-related metabolism, cytokinesis, lipid-related metabolism, membrane bioenergetics, motility/chemotaxis, nucleic acid/nucleotide metabolism, associated with a conserved prophage remnant (lmo0114-lmo0128), and specific transcriptional regulators but excluding master regulators responsive to stress (i.e., SigB, CodY, HtrA).
Amongst ABC-type transporter systems, Lmo1746 (VirA) was downregulated, while Lmo0919 (VgaL), Lmo2499, Lmo0541, Lmo2581, and Lmo0107 were upregulated. VirA contributes to LiaR-mediated salt-induced nisin resistance and virulence and could be linked to cell wall stability [34]. On the other hand, VgaL and Lmo0107 are part of different efflux complexes likely conferring resistance to certain macrolides (lincosamide, streptogramin A) or other unspecified toxic metabolites [35,36]. The other induced transporter proteins are instead involved in inorganic phosphate (Lmo2249), iron complex (Lmo0541), and heme (Lmo2581) uptake. The upregulation of heme-degrading enzyme Lmo0484 [37] also suggests that the response is geared toward either iron extraction or precursors for heme synthesis. Though the findings for antimicrobial resistance are not interpretable, there seems to be some evidence that F2365 attempts to compensate acid shock by phosphate, iron, and heme uptake. All of these resources would be useful in combating the oxidative damage derived from acid stress and conceivably compensate or bolster levels of protective heme-containing enzymes such as catalase, as well as aiding proton extrusion via ATPase, a process that requires phosphate for ATP synthesis and for replenishment of other processes, depending on phosphorylation reactions [10,29]
Relevant to amino acid–related metabolism, only Lmo2819 and TrpD were upregulated. The M20 family carboxypeptidase Lmo2819 is uncharacterized and whether it has specific protein targets is unknown. TrpD (anthranilate phosphoribosyltransferase) is a phosphate-dependent enzyme needed to generate the phosphoribosyl anthranilate intermediate [38]. This could suggest that tryptophan synthesis is a bottleneck for proteostasis. Though not studied in bacteria, tryptophan has been shown to have some importance in delaying protein aggregation [39].
Carbohydrate-related-metabolism proteins showing increased abundance included glycerol kinase GlpK (Lmo1034), class II fructose bisphosphate aldolase FbaA (Lmo0359), and chitin-binding protein (Lmo2467), all upregulated. These proteins have multifunctional roles, some of which are yet to be understood. GlpK is needed to activate glycerol for catabolism and is used purely for energy supply [40]. FbaA has a central role in both glycolysis and gluconeogenesis and is also expressed on the surface of cells [41]; thus, besides enabling energy production, this enzyme mainly shows a moonlighting role [42] of unknown nature, which could though be linked to stress survival. For Lmo2467, its role as a chitin-binding protein has been shown, but it is also needed for host survival although no chitin is available in human hosts [43]. This suggests that Lmo2467 has another role, which is stimulated under acid-stress conditions like FbaA. The results indicate specific responses and not wholesale increases in pathways, for example for synthesis of building blocks or catabolism. Glycerol is generally more available in human host cells, so F2365 could be geared to that substrate in the advent of energy stress brought about by acid exposure, as would occur in macrophage phagosomes. ThiM (thiaminase) was observed to have increased abundance; this change could suggest salvage of thiamine increases [44]. FbaA is a thiamine and phosphate-requiring protein; ThiM protein may act to keep thiamine readily available for FbaA function. Also relevant to energy generation and membrane bioenergetics, the AA3-600 quinol oxidase subunit QoxA had increased abundance. It is presumed that the quinol oxidase complex enables L. monocytogenes to generate energy within host cells [45]; thus, an increase in the activity of this complex could be compensatory for lower electron transport chain activity due to the high external proton concentrations. Typically, the quinol oxidase complex has quite low abundance under standard growth conditions, as L. monocytogenes relies largely on heterofermentation for ATP synthesis including when under growth-permissive acidic conditions [10].
Proteins in the LPI1 (PlcA, PlcB, Hyl, Mpl) and LIP2 (InlA) pathogenicity islands and also InlC (internalin C) were upregulated. These proteins confer the means for host cell attachment, invasion, cell-to-cell spread, and also, via InlC, immune system subversion [46]. Data indicate that the increased InlA and InlC abundance is accompanied by an increase in the main sortase (SrtA, Lmo2714) that organizes and binds internalins to the outer region of the peptidoglycan layer [47]. Overall, this result is interesting since F2365, due to mutations, has lost the ability to invade host cells. This is partly due to disruption of the inlB gene [16]. It is possible that the rapid drop in pH signals responses akin to gastric acid exposure and the presence of acidic conditions in the intestinal tract. PrfA, which activates LIP-1 and internal gene expression [3,4], is known to be controlled by temperature, suppressed by the availability of metabolizable carbohydrates, and activated by the availability of glutathione [48]. A specific experiment measuring intracellular glutathione concentrations is required to determine if this is linked to the acid stress response; however, no enzymes associated with glutathione were noted from the experiment. A simpler explanation is that available carbohydrates are simply depleted or not being metabolized. This is partly due to being in the stationary growth phase but could be due to a lack of proton motive force to drive the uptake of external carbohydrates. Depletion of sugar is known to transiently induce PrfA abundance and subsequent virulence gene expression [49], and thus the response for F2365 essentially harks to a response that energy flow in the cell has been disrupted.
Parallelling the increased abundance of sortase SrtA, numerous proteins associated with the cell wall and involved in its maintenance also became more abundant, as listed in Table 1 [i.e., PbpB, Lmo1216, Lmo0927, Lmo0186, Lmo2504, Lmo2505 (CwlO/Spl), Lmo2591, Iap (Lmo0582) and Lmo1798]. These proteins are either involved with peptidoglycan turnover and cell wall integrity (PpbB, Iap, CwlO/Spl, N-acetylmuramoyl-L-alanine amidases Lmo1216, Lmo1521, and Lmo2591) or with lipoteichoic acid synthesis [Fmt (Lmo0540), Lmo0644, Lmo0927, Lmo1798]. Cell wall–binding proteins Lmo0186 and Lmo2504 may also have a regenerative role in the cell envelope, but their exact function remains unclear [50]. Iap and CwlO/Spl are interesting in that they are required for viability and have key roles in managing peptidoglycan distribution, including the septation process of cell division. Acid stress may imbalance peptidoglycan synthesis (due to a lack of cell energy), and so these and the other proteins may act as a last means of maintaining cell wall integrity by recycling peptidoglycan. Disruption of the process could lead to complete loss of viability since cell division would be blocked and individual cells rapidly inactivate due to the degeneration of the cell envelope. Old Listeria cells can transit to L-forms [51], which are elongated, leaky, or autolyzed, as suggested by DNA leaking into the supernatant of cultures. These cells also are depleted in ATP and lack catabolic enzyme activity [52]. This phenomenon could be linked to the effects of cell wall–integrity disruption and loss of cytoplasmic homeostasis. To avoid protein aggregate accumulation [53], maintenance of proteostasis is needed as well as effective coordination of cell division, which allows continual redistribution of proteins within the cytoplasm.
As expected, acidic conditions suppress motility and chemotaxis. It is now well known that FlaA, the main flagella protein subunit [54], is extremely responsive to physiological stress—its abundance plunges when acid stress is applied at 25 ℃, a temperature in which cells are normally actively motile [10]. At 37 ℃, the levels of FlaA and cellular motility are much lower than at 25 ℃ due to the flagella synthesis being thermoregulated [55]; however, further reduction is expected since cells generate precursor flagella subunits to enable rapid responses to environmental change. Thus, lethal acid stress (pH 4.1 and 3.5), as applied here, likely eliminates both chemotaxis and adherence under non-growth-permissive acidic conditions.
None of the stress-responsive major master transcriptional regulators were affected by acidification in F2365. This is different to strain ScottA, which showed distinct responses when under sub-lethal to near lethal acid stress [10]. The hypothesis is that activation/repression of many of these regulators is transient and, by the stationary growth phase in F2365, the regulators had stable levels and thus already had cascaded downstream expression responses. There were some regulators affected in this experiment, including TetR family regulator Lmo2176 (SpxA1) and uncharacterized regulators Lmo2132 (Crp-family) and Lmo2447. SpxA1 has been found to activate genes for heme synthesis, thus influencing the expression of heme-containing proteins like catalase, enabling L. monocytogenes to have good aerobic growth [56]. As mentioned above, specific proteins linked to heme uptake and turnover were stimulated, as well as those associated with aerobic respiration and catabolism (e.g., QoxA, GlpK), thus these could be linked indirectly in this situation.
Numerous uncharacterized proteins or proteins with elusive functions respond to lethal acid stress (Table 1). This includes increased levels of several proteins of a decayed prophage locus (Lmo0114-0128). This set of proteins includes LmaABCD [57], which seem to be needed for good host survival despite their role remaining mysterious. The other uncharacterized proteins, all mostly having domains of unknown function or lipoproteins (e.g., Lmo0394), may have disparate but secondary roles in L. monocytogenes, with none being essential under standard growth conditions in rich media at 37 ℃ [58]. Only a few have any predicted enzyme function, where they could serve, for example, a remediation role in the cytoplasm removing toxic metabolites. In this respect, the azoreductase AzoR1 (Lmo0786) (studied in E. coli [59]) became more abundant, despite its actual substrate(s) remaining unknown for L. monocytogenes. Another protein, Lmo2642, is probably engaged as second messenger, being a putative cyclic nucleotide phosphodiesterase [60]; however, its role in signaling is yet to be deduced. Similarly, the function of other proteins, such as Lmo2648, which is a putative lactonase or phosphotriesterase, can only be speculated on.
Under normal circumstances, most L. monocytogenes strains can survive non-growth-permissive acid stress for extended periods, especially when at sub-optimal growth temperatures. For example, at 25 ℃ or lower, the strain ScottA lasts for multiple days when in a brine solution under what would be lethal acid conditions (pH 3.0 [8]). At 37 ℃, the infiltration rate of protons across the cytoplasmic membrane increases rapidly, inevitably overwhelming cytoplasmic homeostatic mechanisms, as shown for ATCC 19115 in a similar study [28]. For isolates such as 4b serotype strains ScottA, the rate of inactivation is slower than in strains like F2365. This could be due to its more robust cell wall, a feature in which F2365 is weakened due to stop codon mutations in around 20 genes [16,17]. The overall results indicate that sub-lethal and lethal acid stress in F2365 includes far fewer responses than observed in ScottA [10], and instead only includes mainly specific changes in metabolism that likely either compensate or bolster the associated processes. Data suggest that some responses seem akin to the response L. monocytogenes has in general when it adapts to the human host cells (for example, virulence proteins). This too was observed to occur in ScottA, including an increase in the abundance of InlB. In common to ScottA, strain F2365 showed stimulation of proteins that have major roles in the maintenance of the cell envelope, for example CwlO/Spl, Iap, and peptidoglycan turnover proteins, when experiencing severe growth inhibitory stress by acidification. One reason for these proteins being activated could simply be the flow of sugars into the peptidoglycan monomer synthesis pathway being blocked due to lack of cell energy for synthesis. A key enzyme of glycolysis/gluconeogenesis, FbaA, was more abundant in F2365, while in ScottA the impact on the glycolysis pathway (and central metabolism) was greater in extent. This could relate to the cellular physiology being more impacted in F2365, impeding the ability to create more or rescue proteins, an energy-intensive process. In the end, the process of cell wall renewal is a basic necessity to survive under stress conditions, including in merely the stationary growth phase [61]. Once the protections created by the transition of stationary growth phase have been exceeded, as occurs when pH drops below pH 4.1, the options for F2365 seem to be very limited. It can only compensate its metabolism, which it does rather feebly compared to ScottA, which was found to much more active and widespread; instead, most efforts seems placed on futile cell wall maintenance and a last gasp attempt to escape via cell invasion.
The authors declare we have not used Artificial Intelligence (AI) tools in the creation of this article.
The study was funded by Hebei Natural Science Foundation (Grant No. C2021411001) and was funded by Science Research Project of Hebei Education Department (Grant No. ZD2021001). We thank Shanghai Applied Protein Technology Co. Ltd. for providing technical support.
There is no conflict of interest for this article.
[1] | S. Nancey, J. Bienvenu, B. Coffin, et al., Butyrate strongly inhibits in vitro stimulated release of cytokines in blood, Dig. Dis. Sci., 47 (2002), 921–928. |
[2] | S. M. Finegold, S. E. Dowd, V. Gontcharova, et al., Pyrosequencing study of fecal microflora of autistic and control children, Anaerobe, 16 (2010), 444–453. |
[3] | A. C. Ericsson, S. Akter, M. M. Hanson, et al., Differential susceptibility to colorectal cancer due to naturally occurring gut microbiota, Oncotarget, 6 (2015), 33689–33704. |
[4] | H. E. Jakobsson, C. Jernberg, A. F. Andersson, et al., Short-term antibiotic treatment has differing long-term impacts on the human throat and gut microbiome, PLoS One, 5 (2010), e9836. |
[5] | L. Dethlefsen and D. A. Relman, Incomplete recovery and individualized responses of the human distal gut microbiota to repeated antibiotic perturbation, Proc. Natl. Acad. Sci. U. S. A., 108 (2011), 4554–4561. |
[6] | J. J. Faith, J. L. Guruge, M. Charbonneau, et al., The long-term stability of the human gut microbiota, Science, 341 (2013), 1237439. |
[7] | I. Gustafsson, M. Sjolund, E. Torell, et al., Bacteria with increased mutation frequency and antibiotic resistance are enriched in the commensal flora of patients with high antibiotic usage, J. Antimicrob. Chemother., 52 (2003), 645–650. |
[8] | D. Artis, Epithelial-cell recognition of commensal bacteria and maintenance of immune homeostasis in the gut, Nat. Rev. Immunol., 8 (2008), 411–420. |
[9] | V. Bucci, C. D. Nadell and J. B. Xavier, The evolution of bacteriocin production in bacterial biofilms, Am. Nat., 178 (2008), E162–173. |
[10] | J. Zheng, M. G. Ganzle, X. B. Lin, et al., Diversity and dynamics of bacteriocins from human microbiome, Environ Microbiol, 17 (2015), 2133–2143. |
[11] | F. Zhang, B. Cui, X. He, et al., Microbiota transplantation: concept, methodology and strategy for its modernization, Protein. Cell., 9 (2018), 462–473. |
[12] | S. N. Gopalsamy, M. H. Woodworth, T. Wang, et al., The Use of Microbiome Restoration Therapeutics to Eliminate Intestinal Colonization With Multidrug-Resistant Organisms, Am. J. Med. Sci., 356 (2018), 433–440. |
[13] | Y. Wei, J. Gong, W. Zhu, et al., Fecal microbiota transplantation restores dysbiosis in patients with methicillin resistant Staphylococcus aureus enterocolitis, BMC Infect. Dis., 15 (2015), 265. |
[14] | D. Ishikawa, T. Sasaki, T. Osada, et al., Changes in Intestinal Microbiota Following Combination Therapy with Fecal Microbial Transplantation and Antibiotics for Ulcerative Colitis, Inflamm. Bowel. Dis., 23 (2017), 116–125. |
[15] | E. van Nood, A. Vrieze, M. Nieuwdorp, et al., Duodenal infusion of donor feces for recurrent Clostridium difficile, N. Engl. J. Med., 368 (2013), 407–415. |
[16] | C. Ubeda, V. Bucci, S. Caballero, et al., Intestinal microbiota containing Barnesiella species cures vancomycin-resistant Enterococcus faecium colonization, Infect. Immun., 81 (2013), 965–973. |
[17] | L. J. Brandt, American Journal of Gastroenterology Lecture: Intestinal microbiota and the role of fecal microbiota transplant (FMT) in treatment of C. difficile infection, Am. J. Gastroenterol., 108 (2013), 177–185. |
[18] | M. C. Zanella Terrier, M. L. Simonet, P. Bichard, et al., Recurrent Clostridium difficile infections: the importance of the intestinal microbiota, World J. Gastroenterol., 20 (2014), 7416–7423. |
[19] | S. Jamot, V. Raghunathan, K. Patel, et al., Factors Associated with the Use of Fecal Microbiota Transplant in Patients with Recurrent Clostridium difficile Infections, Infect. Control. Hosp. Epidemiol., 39 (2018), 302–306. |
[20] | G. Cammarota, G. Ianiro and A. Gasbarrini, Fecal microbiota transplantation for the treatment of Clostridium difficile infection: a systematic review, J. Clin. Gastroenterol., 48 (2014), 693–702. |
[21] | Y. Li, A. Karlin, J. D. Loike, et al., Determination of the critical concentration of neutrophils required to block bacterial growth in tissues, J. Exp. Med., 200 (2004), 613–622. |
[22] | A. Heinken and I. Thiele, Anoxic Conditions Promote Species-Specific Mutualism between Gut Microbes In Silico, Appl. Environ. Microbiol., 81 (2015), 4049–4061. |
[23] | T. J. Wiles, M. Jemielita, R. P. Baker, et al., Host Gut Motility Promotes Competitive Exclusion within a Model Intestinal Microbiota, PLoS Biol., 14 (2016), e1002517. |
[24] | T. E. Gibson, A. Bashan, H. T. Cao, et al., On the Origins and Control of Community Types in the Human Microbiome, PLoS Comput. Biol., 12 (2016), e1004688. |
[25] | D. Gonze, L. Lahti, J. Raes, et al., Multi-stability and the origin of microbial community types, ISME J., 11 (2017), 2159–2166. |
[26] | A. L. Gomes, J. E. Galagan and D. Segre, Resource competition may lead to effective treatment of antibiotic resistant infections, PLoS One, 8 (2013), e80775. |
[27] | E. M. D'Agata, M. Dupont-Rouzeyrol, P. Magal, et al., The impact of different antibiotic regimens on the emergence of antimicrobial-resistant bacteria, PLoS One, 3 (2008), e4036. |
[28] | V. Bucci, S. Bradde, G. Biroli, et al., Social interaction, noise and antibiotic-mediated switches in the intestinal microbiota, PLoS Comput. Biol., 8 (2012), e1002497. |
[29] | S. Estrela and S. P. Brown, Community interactions and spatial structure shape selection on antibiotic resistant lineages, PLoS Comput. Biol., 14 (2018), e1006179. |
[30] | S. W. Wu, H. de Lencastre and A. Tomasz, Recruitment of the mecA gene homologue of Staphylococcus sciuri into a resistance determinant and expression of the resistant phenotype in Staphylococcus aureus, J. Bacteriol., 183 (2001), 2417–2424. |
[31] | S. Gottig, S. Riedel-Christ, A. Saleh, et al., Impact of blaNDM-1 on fitness and pathogenicity of Escherichia coli and Klebsiella pneumoniae, Int. J. Antimicrob. Agents., 47 (2016), 430–435. |
[32] | R. Freter, H. Brickner, J. Fekete, et al., Survival and implantation of Escherichia coli in the intestinal tract, Infect. Immun., 39 (1983), 686–703. |
[33] | M. P. Leatham, S. Banerjee, S. M. Autieri, et al., Precolonized human commensal Escherichia coli strains serve as a barrier to E. coli O157:H7 growth in the streptomycin-treated mouse intestine, Infect. Immun., 77 (2009), 2876–2886. |
[34] | K. Tabita, S. Sakaguchi, S. Kozaki, et al., Comparative studies on Clostridium botulinum type A strains associated with infant botulism in Japan and in California, USA, Jpn. J. Med. Sci. Biol., 43 (1990), 219–231. |
[35] | Y. Yamashiro, Gut Microbiota in Health and Disease, Ann. Nutr. Metab., 71 (2017), 242–246. |
[36] | C. Cordonnier, G. Le Bihan, J. G. Emond-Rheault, et al., Vitamin B12 Uptake by the Gut Commensal Bacteria Bacteroides thetaiotaomicron Limits the Production of Shiga Toxin by Enterohemorrhagic Escherichia coli, Toxins (Basel), 8 (2016), E14. |
[37] | R. A. Sorg, L. Lin, G. S. van Doorn, et al., Collective Resistance in Microbial Communities by Intracellular Antibiotic Deactivation, PLoS Biol., 14 (2016), e2000631. |
[38] | T. Ito, K. Okuma, X. X. Ma, et al., Insights on antibiotic resistance of Staphylococcus aureus from its whole genome: genomic island SCC, Drug Resist Updat., 6 (2003), 41–52. |
[39] | H. Nicoloff and D. I. Andersson, Indirect resistance to several classes of antibiotics in cocultures with resistant bacteria expressing antibiotic-modifying or -degrading enzymes, J. Antimicrob. Chemother., 71 (2016), 100–110. |
[40] | C. A. Lozupone, J. I. Stombaugh, J. I. Gordon, et al., Diversity, stability and resilience of the human gut microbiota, Nature, 489, (2012), 220–230. |
[41] | C. Manichanh, J. Reeder, P. Gibert, et al., Reshaping the gut microbiome with bacterial transplantation and antibiotic intake, Genome. Res., 20 (2010), 1411–1419. |
[42] | E. K. Costello, C. L. Lauber, M. Hamady, et al., Bacterial community variation in human body habitats across space and time, Science, 326 (2009), 1694–1697. |
[43] | P. J. Turnbaugh, M. Hamady, T. Yatsunenko, et al., A core gut microbiome in obese and lean twins, Nature, 457 (2009), 480–484. |
[44] | A. Uygun, K. Ozturk, H. Demirci, et al., Fecal microbiota transplantation is a rescue treatment modality for refractory ulcerative colitis, Medicine (Baltimore), 96 (2017), e6479. |
[45] | B. Cui, Q. Feng, H. Wang, et al., Fecal microbiota transplantation through mid-gut for refractory Crohn's disease: safety, feasibility, and efficacy trial results, J. Gastroenterol. Hepatol., 30 (2015), 51–58. |
[46] | C. R. Kelly, S. Kahn, P. Kashyap, et al., Update on Fecal Microbiota Transplantation 2015: Indications, Methodologies, Mechanisms, and Outlook, Gastroenterology, 149 (2015), 223–237. |
[47] | S. Vermeire, M. Joossens, K. Verbeke, et al., Donor Species Richness Determines Faecal Microbiota Transplantation Success in Inflammatory Bowel Disease, J. Crohns. Colitis, 10 (2016), 387–394. |
[48] | H. Seedorf, N. W. Griffin, V. K. Ridaura, et al., Bacteria from diverse habitats colonize and compete in the mouse gut, Cell, 159 (2014), 253–266. |
[49] | S. K. Ji, H. Yan, T. Jiang, et al., Preparing the Gut with Antibiotics Enhances Gut Microbiota Reprogramming Efficiency by Promoting Xenomicrobiota Colonization, Front. Microbiol., 8 (2017), 1208. |
[50] | D. L. Suskind, M. J. Brittnacher, G. Wahbeh, et al., Fecal microbial transplant effect on clinical outcomes and fecal microbiome in active Crohn's disease, Inflamm. Bowel. Dis., 21 (2015), 556–563. |
[51] | A. K. Seth, P. Rawal, R. Bagga, et al., Successful colonoscopic fecal microbiota transplantation for active ulcerative colitis: First report from India, Indian. J. Gastroenterol., 35 (2016), 393–395. |
[52] | S. X. Liu, Y. H. Li, W. K. Dai, et al., Fecal microbiota transplantation induces remission of infantile allergic colitis through gut microbiota re-establishment, World J. Gastroenterol., 23 (2017), 8570–8581. |
[53] | J. Zhang, J. J. Cunningham, J. S. Brown, et al., Integrating evolutionary dynamics into treatment of metastatic castrate-resistant prostate cancer, Nat. Commun., 8 (2017), 1816. |
[54] | R. B. Montgomery, E. A. Mostaghel, R. Vessella, et al., Maintenance of intratumoral androgens in metastatic prostate cancer: a mechanism for castration-resistant tumor growth, Cancer Res., 68 (2008), 4447–4454. |
[55] | J. M. Hyatt, D. E. Nix, C. W. Stratton, et al., In vitro pharmacodynamics of piperacillin, piperacillin-tazobactam, and ciprofloxacin alone and in combination against Staphylococcus aureus, Klebsiella pneumoniae, Enterobacter cloacae, and Pseudomonas aeruginosa, Antimicrob. Agents Chemother., 39 (1995), 1711–1716. |
[56] | D. M. Chaput de Saintonge, D. F. Levine, I. T. Savage, et al., Trial of three-day and ten-day courses of amoxycillin in otitis media, Br. Med. J. (Clin. Res. Ed.), 284 (1982), 1078–1081. |
[57] | C. Llor and L. Bjerrum, Antimicrobial resistance: risk associated with antibiotic overuse and initiatives to reduce the problem, Ther. Adv. Drug Saf., 5, (2014), 229–241. |
[58] | I. van Langeveld, R. C. Gagnon, P. F. Conrad, et al., Multiple-Drug Resistance in Burn Patients: A Retrospective Study on the Impact of Antibiotic Resistance on Survival and Length of Stay, J. Burn. Care. Res., 38, (2017), 99–105. |
1. | Jian Jin, Jie Feng, iDHS-RGME: Identification of DNase I hypersensitive sites by integrating information on nucleotide composition and physicochemical properties, 2024, 734, 0006291X, 150618, 10.1016/j.bbrc.2024.150618 |
F2365 Loci | EGD-e gene name | pH 4.8/C | Log ratio pH 4.1/C | pH 3.5/C | Known or predicted specific function |
LMOf2365_2631 | lmo1034 | 0.94 | 1.32 | 2.43 | glycerol kinase |
LMOf2365_0212 | plcA | 1.04 | 1.65 | 2.15 | phosphatidylinositol-specific phospholipase C PlcA |
LMOf2365_0148 | lmo0130 | 1.02 | 1.15 | 1.61 | putative cell surface anchored 5'-nucleotidase |
LMOf2365_0136 | lmo0118 | 1.13 | 1.84 | 2.09 | putative phage tail protein, phage_tail_2_superfamily; antigen A protein LmaA |
LMOf2365_0941 | lmo0919 | 0.97 | 0.97 | 1.68 | putative ABC transporter, ATP-binding protein |
LMOf2365_1771 | lmo1746 | 0.93 | 0.87 | 0.57 | putative bacitracin (drug) efflux ABC transporter, permease protein |
LMOf2365_2553 | lmo2581 | 1 | 1.06 | 2.59 | MacB-like ABC transporter, ATP-binding protein |
LMOf2365_2810 | lmo2819 | 1.02 | 1 | 1.63 | putative N-acyl-L-amino acid amidohydrolase, M20_dimer superfamily |
LMOf2365_1535 | lmo1516 | 1 | 1.08 | 1.92 | ammonium uptake transporter |
LMOf2365_1653 | trpD | 1.03 | 1.08 | 1.7 | anthranilate phosphoribosyltransferase |
LMOf2365_0213 | hly | 0.97 | 1.88 | 3.24 | membrane protein, HlyIII superfamily |
LMOf2365_2165 | lmo2131 | 0.94 | 0.77 | 2.38 | putative Crp-like transcriptional regulator, CAP_ED superfamily |
LMOf2365_2394 | lmo2423 | 1.02 | 1.01 | 1.58 | putative metal cation exporter, cation_efflux superfamily |
LMOf2365_2694 | lmo2714 | 1.11 | 1.55 | 2.8 | putative cell wall sorted protein |
LMOf2365_1723 | lmo1699 | 0.67 | 0.65 | 0.65 | methyl-accepting chemotaxis protein |
LMOf2365_0722 | lmo0686 | 0.79 | 0.94 | 1.61 | flagellar motor rotation protein B |
LMOf2365_2440 | lmo2467 | 0.93 | 1.29 | 3.44 | putative chitin binding protein, chitin_bind_3/FN3/chtBD3 superfamily |
LMOf2365_0295 | lmo0275 | 0.92 | 2.38 | 3.22 | putative metallo-beta-lactamase superfamily protein |
LMOf2365_2166 | lmo2132 | 0.77 | 0.79 | 0.62 | putative Crp-like transcriptional regulator, CAP_ED superfamily |
LMOf2365_1718 | lmo1694 | 1.2 | 1.09 | 3.46 | putative nucleoside-diphosphate sugar epimerase, NADB_Rossmann superfamily |
LMOf2365_1193 | lmo1183 | 1.25 | 1.4 | 3.48 | putative ethanolamine utilization-associated protein |
LMOf2365_0726 | flaA | 0.33 | 0.36 | 0.54 | flagellin Fla |
LMOf2365_1816 | azoR2 | 0.9 | 0.93 | 2.05 | NADH-azoreductase, FMN-dependent |
LMOf2365_0948 | lmo0927 | 1.01 | 1.98 | 3.64 | exported glycerolphosphate lipoteichoic acid synthetase |
LMOf2365_0334 | thiM | 1.09 | 1.29 | 1.57 | hydroxyethylthiazole kinase |
LMOf2365_0471 | inlA | 1.1 | 1.25 | 1.68 | internalin A; cell wall protein with LRR and B repeat domains InlA |
LMOf2365_2282 | lmo2249 | 1.09 | 1.06 | 1.66 | low-affinity inorganic phosphate transporter |
LMOf2365_1812 | inlB | 1.01 | 2.29 | 4.44 | unanchored cell wall internalin B InlB |
LMOf2365_0283 | inlE | 1.03 | 1.17 | 2.33 | internalin E |
LMOf2365_2178 | lmo2146 | 0.96 | 1.01 | 0.65 | putative transcriptional regulator, PBP2_CysL_like family |
LMOf2365_0932 | lmo0910 | 0.97 | 0.97 | 1.86 | DUF1648 superfamily protein |
LMOf2365_2272 | lmo2239 | 0.96 | 1.04 | 1.73 | uncharacterized protein |
LMOf2365_0139 | lmo0121 | 1.13 | 1.36 | 2.18 | putative phage-associated membrane protein, COG5412 family |
LMOf2365_0214 | mpl | 0.99 | 1.41 | 2.11 | putative L-lactate dehydrogenase |
LMOf2365_0125 | lmo0107 | 1.19 | 1.22 | 3.68 | putative export ABC transporter, ATP-binding/permease |
LMOf2365_1540 | lmo1521 | 1.01 | 1.54 | 2.53 | N-acetylmuramoyl-L-alanine amidase |
LMOf2365_1225 | lmo1216 | 1.01 | 1.89 | 3.11 | exoglucosaminidase/muramidase |
LMOf2365_2568 | lmo2595 | 1.03 | 1.51 | 2.62 | uncharacterized protein |
LMOf2365_1900 | 1.03 | 1.94 | 3.39 | uncharacterized protein | |
LMOf2365_0570 | lmo0541 | 1.02 | 1.23 | 2.5 | iron complex uptake ABC transporter, substrate binding protein FecB-like |
LMOf2365_0145 | lmo0127 | 1.24 | 1.54 | 1.91 | putative phage protein (Gp20 Listeria phage A118) |
LMOf2365_0667 | 1.03 | 2.04 | 3.86 | uncharacterized protein | |
LMOf2365_0162 | lmo0146 | 1.25 | 1.36 | 1.82 | uncharacterized protein |
LMOf2365_0144 | lmo0126 | 1.17 | 1.52 | 1.44 | putative phage protein |
LMOf2365_2195 | lmo2163 | 1.02 | 1.02 | 0.63 | putative dehydrogenase, GFO_IDH_MocA superfamily |
LMOf2365_1457 | pbpA | 1 | 1.23 | 1.68 | septal peptidoglycan transpeptidase |
LMOf2365_2071 | pbpB | 1.02 | 1.53 | 1.94 | septal peptidoglycan transpeptidase |
LMOf2365_0569 | lmo0540 | 1.09 | 1.39 | 2.24 | cell division–associated peptidoglycan endopeptidase |
LMOf2365_2477 | lmo2504 | 0.99 | 2.3 | 3.55 | putative murein endopeptidase |
LMOf2365_2478 | lmo2505 | 0.98 | 2.31 | 3.81 | peptidoglycan DL-endopeptidase P45 |
LMOf2365_0406 | lmo0394 | 0.89 | 1.35 | 2.07 | Nlp/60 family protein |
LMOf2365_0611 | iap | 0.99 | 1.25 | 5.56 | gamma-D-glutamate-meso-diaminopimelate muropeptidase; invasion-associated protein Iap |
LMOf2365_2472 | lmo2499 | 0.98 | 1.16 | 1.88 | phosphate ABC transporter, substrate binding protein |
LMOf2365_0216 | plcB | 1.05 | 1.65 | 2.66 | zinc-dependent phospholipase C PlcB |
LMOf2365_2620 | lmo2648 | 1.27 | 1.39 | 1.69 | putative phosphotriesterase, metallo-dependent hydrolase superfamily |
LMOf2365_1825 | lmo1798 | 0.96 | 1.39 | 6.27 | putative poly (glycerol-phosphate) alpha-glucosyltransferase |
LMOf2365_1202 | cobH | 0.93 | 1.58 | 0.41 | precorrin-8X methylmutase CobH |
LMOf2365_0016 | qoxA | 0.98 | 1.48 | 2.18 | AA3-600 quinol oxidase subunit II |
LMOf2365_2139 | lmo2106 | 1.19 | 1.09 | 1.67 | putative phosphohydrolase, MPP_YkuE_C family protein |
LMOf2365_2615 | lmo2642 | 1.2 | 1.35 | 1.7 | putative metal-binding phosphohydrolase, Icc superfamily |
LMOf2365_0675 | lmo0644 | 1.01 | 1.84 | 3.07 | putative sulfatase or phosphoglycerol transferase, alkaline phosphatase superfamily |
LMOf2365_2208 | lmo2176 | 1 | 0.97 | 0.53 | putative ArcR-like transcriptional regulator, TetR_N superfamily |
LMOf2365_2420 | lmo2477 | 0.83 | 0.97 | 0.6 | putative transcriptional regulator, HTH_XRE/Rgg_CTERM superfamily |
LMOf2365_0288 | lmo0269 | 0.92 | 1.03 | 2.95 | putative ABC transporter, permease |
LMOf2365_1777 | lmo1752 | 1.02 | 1.23 | 2.49 | uncharacterized secreted protein |
LMOf2365_1223 | lmo1214 | 0.92 | 0.95 | 0.63 | DUF2812 superfamily protein |
LMOf2365_1350 | lmo1333 | 1.2 | 1.37 | 2.83 | putative secreted protein, YceG-like superfamily |
LMOf2365_0135 | lmo0117 | 1.17 | 1.43 | 1.73 | putative phage protein; Antigen B protein LmaB |
LMOf2365_1077 | lmo1056 | 1.13 | 1.12 | 1.51 | uncharacterized protein |
LMOf2365_2689 | lmo2709 | 1.07 | 1.3 | 1.51 | uncharacterized protein |
LMOf2365_0134 | lmo0116 | 1.24 | 1.41 | 1.73 | putative phage transcriptional activator, phage_ArpU superfamily; antigen C protein LmaC |
LMOf2365_0197 | lmo0186 | 1.01 | 2.38 | 3.63 | putative resuscitation-promoting factor/stationary-phase survival autolysin, Rpf/Sps_SpsB subfamily |
LMOf2365_0254 | lmo0242 | 1.18 | 1.12 | 1.59 | DUF901 superfamily protein with PIN domain |
LMOf2365_2813 | lmo2822 | 0.98 | 0.9 | 1.67 | uncharacterized protein |
LMOf2365_0379 | lmo0359 | 0.9 | 1.42 | 2.89 | putative fructose-1, 6, -bisphosphate aldolase |
LMOf2365_2769 | lmo2778 | 0.99 | 1.23 | 0.57 | uncharacterized protein |
LMOf2365_0143 | lmo0125 | 1.28 | 1.62 | 1.94 | putative phage protein |
LMOf2365_2693 | lmo2713 | 1 | 1.93 | 3.08 | secreted protein, COG3786 superfamily |
LMOf2365_2188 LMOf2365_0431 LMOf2365_0037 LMOf2365_2564 LMOf2365_0346 LMOf2365_0140 LMOf2365_0900 LMOf2365_0428 LMOf2365_2387 LMOf2365_1130 LMOf2365_1885 LMOf2365_1590 |
lmo2156 lmo0412 lmo2591 lmo0328 lmo0122 lmo0881 lmo0408 lmo2416 lmo1124 lmo1857 lmo1568 |
0.97 0.97 1.03 0.91 0.92 1.17 0.98 1.04 1.06 1.04 0.99 1.07 |
2.14 1.63 1.9 1.86 1.11 1.43 1.39 1.31 1.26 0.94 1.03 1.12 |
3.58 3.65 3.89 3.96 0.62 1.73 1.8 1.82 2 2.5 2.08 1.53 |
YxeA family (DUF1093 superfamily) protein uncharacterized membrane-associated secreted protein uncharacterized protein exoglucosaminidase/muramidase uncharacterized protein putative phage tail protein, sipho_tail superfamily uncharacterized secreted protein DUF1312 superfamily secreted protein uncharacterized secreted protein uncharacterized protein DUF1250 (COG4479) superfamily protein DUF441 superfamily protein |
Note: When p value < 0.05, log ratio values are in bold type. |
F2365 Loci | Gene name | Log ratio pH 4.8/C | iTRAQ/PRM pH 4.1/C | Results pH 3.5/C | Known or predicted specific function |
LMOf2365_2702 | lmo1671 | 0.98/0.60 | 0.96/0.50 | 0.75/0.43 | zinc uptake ABC transporter, substrate-binding protein |
LMOf2365_1520 | lmo1501 | 0.94/0.59 | 0.90/0.41 | 0.75/0.32 | DUF1292 superfamily protein |
LMOf2365_0912 | rsbV | 0.98/0.64 | 0.93/0.56 | 0.80/0.54 | positive (anti-sigma B) regulatory factor (acts against RsbW) |
LMOf2365_1561 | rplU | 0.96/0.63 | 0.92/0.55 | 0.80/0.50 | ribosomal protein L21 |
LMOf2365_2532 | lmo2560 | 0.89/0.73 | 0.94/0.89 | 0.81/0.71 | RNA polymerase, delta subunit |
LMOf2365_1579 | engB | 0.98/0.73 | 0.97/0.68 | 0.81/0.55 | ribosome assembly GTP-binding protein |
LMOf2365_2603 | rplW | 0.94/0.70 | 0.94/0.60 | 0.81/0.58 | ribosomal protein L23 |
LMOf2365_2148 | lmo2115 | 0.97/0.66 | 0.93/0.59 | 0.82/0.65 | putative drug/toxic substance efflux ABC transporter, permease protein |
LMOf2365_1708 | lmo1684 | 0.93/0.68 | 0.90/0.51 | 0.82/0.57 | putative D-isomer 2-hydroxy acid dehydrogenase, NADB_Rossmann superfamily |
LMOf2365_0140 LMOf2365_0136 LMOf2365_0213 LMOf2365_0948 LMOf2365_2478 LMOf2365_0611 |
lmo0122 lmaA lmo0202 lmo0927 lmo2505 iap |
1.17/1.27 1.13/1.24 0.97/0.64 1.01/1.09 0.98/0.62 0.99/0.95 |
1.43/1.70 1.84/4.05 1.88/1.89 1.98/17.47 2.31/6.62 1.25/1.17 |
1.73/2.36 2.09/4.97 3.24/2.64 3.64/45.00 3.81/18.86 5.56/11.32 |
putative phage tail protein, sipho_tail superfamily putative phage tail protein, phage_tail_2_superfamily; antigen A protein LmaA listeriolysin O LLO exported glycerolphosphate lipoteichoic acid synthetase peptidoglycan DL-endopeptidase P45 gamma-D-glutamate-meso-diaminopimelate muropeptidase; invasion-associated protein Iap |
Note: When p value ﹤ 0.05, log ratio value are in bold type. |
F2365 Loci | EGD-e gene name | pH 4.8/C | Log ratio pH 4.1/C | pH 3.5/C | Known or predicted specific function |
LMOf2365_2631 | lmo1034 | 0.94 | 1.32 | 2.43 | glycerol kinase |
LMOf2365_0212 | plcA | 1.04 | 1.65 | 2.15 | phosphatidylinositol-specific phospholipase C PlcA |
LMOf2365_0148 | lmo0130 | 1.02 | 1.15 | 1.61 | putative cell surface anchored 5'-nucleotidase |
LMOf2365_0136 | lmo0118 | 1.13 | 1.84 | 2.09 | putative phage tail protein, phage_tail_2_superfamily; antigen A protein LmaA |
LMOf2365_0941 | lmo0919 | 0.97 | 0.97 | 1.68 | putative ABC transporter, ATP-binding protein |
LMOf2365_1771 | lmo1746 | 0.93 | 0.87 | 0.57 | putative bacitracin (drug) efflux ABC transporter, permease protein |
LMOf2365_2553 | lmo2581 | 1 | 1.06 | 2.59 | MacB-like ABC transporter, ATP-binding protein |
LMOf2365_2810 | lmo2819 | 1.02 | 1 | 1.63 | putative N-acyl-L-amino acid amidohydrolase, M20_dimer superfamily |
LMOf2365_1535 | lmo1516 | 1 | 1.08 | 1.92 | ammonium uptake transporter |
LMOf2365_1653 | trpD | 1.03 | 1.08 | 1.7 | anthranilate phosphoribosyltransferase |
LMOf2365_0213 | hly | 0.97 | 1.88 | 3.24 | membrane protein, HlyIII superfamily |
LMOf2365_2165 | lmo2131 | 0.94 | 0.77 | 2.38 | putative Crp-like transcriptional regulator, CAP_ED superfamily |
LMOf2365_2394 | lmo2423 | 1.02 | 1.01 | 1.58 | putative metal cation exporter, cation_efflux superfamily |
LMOf2365_2694 | lmo2714 | 1.11 | 1.55 | 2.8 | putative cell wall sorted protein |
LMOf2365_1723 | lmo1699 | 0.67 | 0.65 | 0.65 | methyl-accepting chemotaxis protein |
LMOf2365_0722 | lmo0686 | 0.79 | 0.94 | 1.61 | flagellar motor rotation protein B |
LMOf2365_2440 | lmo2467 | 0.93 | 1.29 | 3.44 | putative chitin binding protein, chitin_bind_3/FN3/chtBD3 superfamily |
LMOf2365_0295 | lmo0275 | 0.92 | 2.38 | 3.22 | putative metallo-beta-lactamase superfamily protein |
LMOf2365_2166 | lmo2132 | 0.77 | 0.79 | 0.62 | putative Crp-like transcriptional regulator, CAP_ED superfamily |
LMOf2365_1718 | lmo1694 | 1.2 | 1.09 | 3.46 | putative nucleoside-diphosphate sugar epimerase, NADB_Rossmann superfamily |
LMOf2365_1193 | lmo1183 | 1.25 | 1.4 | 3.48 | putative ethanolamine utilization-associated protein |
LMOf2365_0726 | flaA | 0.33 | 0.36 | 0.54 | flagellin Fla |
LMOf2365_1816 | azoR2 | 0.9 | 0.93 | 2.05 | NADH-azoreductase, FMN-dependent |
LMOf2365_0948 | lmo0927 | 1.01 | 1.98 | 3.64 | exported glycerolphosphate lipoteichoic acid synthetase |
LMOf2365_0334 | thiM | 1.09 | 1.29 | 1.57 | hydroxyethylthiazole kinase |
LMOf2365_0471 | inlA | 1.1 | 1.25 | 1.68 | internalin A; cell wall protein with LRR and B repeat domains InlA |
LMOf2365_2282 | lmo2249 | 1.09 | 1.06 | 1.66 | low-affinity inorganic phosphate transporter |
LMOf2365_1812 | inlB | 1.01 | 2.29 | 4.44 | unanchored cell wall internalin B InlB |
LMOf2365_0283 | inlE | 1.03 | 1.17 | 2.33 | internalin E |
LMOf2365_2178 | lmo2146 | 0.96 | 1.01 | 0.65 | putative transcriptional regulator, PBP2_CysL_like family |
LMOf2365_0932 | lmo0910 | 0.97 | 0.97 | 1.86 | DUF1648 superfamily protein |
LMOf2365_2272 | lmo2239 | 0.96 | 1.04 | 1.73 | uncharacterized protein |
LMOf2365_0139 | lmo0121 | 1.13 | 1.36 | 2.18 | putative phage-associated membrane protein, COG5412 family |
LMOf2365_0214 | mpl | 0.99 | 1.41 | 2.11 | putative L-lactate dehydrogenase |
LMOf2365_0125 | lmo0107 | 1.19 | 1.22 | 3.68 | putative export ABC transporter, ATP-binding/permease |
LMOf2365_1540 | lmo1521 | 1.01 | 1.54 | 2.53 | N-acetylmuramoyl-L-alanine amidase |
LMOf2365_1225 | lmo1216 | 1.01 | 1.89 | 3.11 | exoglucosaminidase/muramidase |
LMOf2365_2568 | lmo2595 | 1.03 | 1.51 | 2.62 | uncharacterized protein |
LMOf2365_1900 | 1.03 | 1.94 | 3.39 | uncharacterized protein | |
LMOf2365_0570 | lmo0541 | 1.02 | 1.23 | 2.5 | iron complex uptake ABC transporter, substrate binding protein FecB-like |
LMOf2365_0145 | lmo0127 | 1.24 | 1.54 | 1.91 | putative phage protein (Gp20 Listeria phage A118) |
LMOf2365_0667 | 1.03 | 2.04 | 3.86 | uncharacterized protein | |
LMOf2365_0162 | lmo0146 | 1.25 | 1.36 | 1.82 | uncharacterized protein |
LMOf2365_0144 | lmo0126 | 1.17 | 1.52 | 1.44 | putative phage protein |
LMOf2365_2195 | lmo2163 | 1.02 | 1.02 | 0.63 | putative dehydrogenase, GFO_IDH_MocA superfamily |
LMOf2365_1457 | pbpA | 1 | 1.23 | 1.68 | septal peptidoglycan transpeptidase |
LMOf2365_2071 | pbpB | 1.02 | 1.53 | 1.94 | septal peptidoglycan transpeptidase |
LMOf2365_0569 | lmo0540 | 1.09 | 1.39 | 2.24 | cell division–associated peptidoglycan endopeptidase |
LMOf2365_2477 | lmo2504 | 0.99 | 2.3 | 3.55 | putative murein endopeptidase |
LMOf2365_2478 | lmo2505 | 0.98 | 2.31 | 3.81 | peptidoglycan DL-endopeptidase P45 |
LMOf2365_0406 | lmo0394 | 0.89 | 1.35 | 2.07 | Nlp/60 family protein |
LMOf2365_0611 | iap | 0.99 | 1.25 | 5.56 | gamma-D-glutamate-meso-diaminopimelate muropeptidase; invasion-associated protein Iap |
LMOf2365_2472 | lmo2499 | 0.98 | 1.16 | 1.88 | phosphate ABC transporter, substrate binding protein |
LMOf2365_0216 | plcB | 1.05 | 1.65 | 2.66 | zinc-dependent phospholipase C PlcB |
LMOf2365_2620 | lmo2648 | 1.27 | 1.39 | 1.69 | putative phosphotriesterase, metallo-dependent hydrolase superfamily |
LMOf2365_1825 | lmo1798 | 0.96 | 1.39 | 6.27 | putative poly (glycerol-phosphate) alpha-glucosyltransferase |
LMOf2365_1202 | cobH | 0.93 | 1.58 | 0.41 | precorrin-8X methylmutase CobH |
LMOf2365_0016 | qoxA | 0.98 | 1.48 | 2.18 | AA3-600 quinol oxidase subunit II |
LMOf2365_2139 | lmo2106 | 1.19 | 1.09 | 1.67 | putative phosphohydrolase, MPP_YkuE_C family protein |
LMOf2365_2615 | lmo2642 | 1.2 | 1.35 | 1.7 | putative metal-binding phosphohydrolase, Icc superfamily |
LMOf2365_0675 | lmo0644 | 1.01 | 1.84 | 3.07 | putative sulfatase or phosphoglycerol transferase, alkaline phosphatase superfamily |
LMOf2365_2208 | lmo2176 | 1 | 0.97 | 0.53 | putative ArcR-like transcriptional regulator, TetR_N superfamily |
LMOf2365_2420 | lmo2477 | 0.83 | 0.97 | 0.6 | putative transcriptional regulator, HTH_XRE/Rgg_CTERM superfamily |
LMOf2365_0288 | lmo0269 | 0.92 | 1.03 | 2.95 | putative ABC transporter, permease |
LMOf2365_1777 | lmo1752 | 1.02 | 1.23 | 2.49 | uncharacterized secreted protein |
LMOf2365_1223 | lmo1214 | 0.92 | 0.95 | 0.63 | DUF2812 superfamily protein |
LMOf2365_1350 | lmo1333 | 1.2 | 1.37 | 2.83 | putative secreted protein, YceG-like superfamily |
LMOf2365_0135 | lmo0117 | 1.17 | 1.43 | 1.73 | putative phage protein; Antigen B protein LmaB |
LMOf2365_1077 | lmo1056 | 1.13 | 1.12 | 1.51 | uncharacterized protein |
LMOf2365_2689 | lmo2709 | 1.07 | 1.3 | 1.51 | uncharacterized protein |
LMOf2365_0134 | lmo0116 | 1.24 | 1.41 | 1.73 | putative phage transcriptional activator, phage_ArpU superfamily; antigen C protein LmaC |
LMOf2365_0197 | lmo0186 | 1.01 | 2.38 | 3.63 | putative resuscitation-promoting factor/stationary-phase survival autolysin, Rpf/Sps_SpsB subfamily |
LMOf2365_0254 | lmo0242 | 1.18 | 1.12 | 1.59 | DUF901 superfamily protein with PIN domain |
LMOf2365_2813 | lmo2822 | 0.98 | 0.9 | 1.67 | uncharacterized protein |
LMOf2365_0379 | lmo0359 | 0.9 | 1.42 | 2.89 | putative fructose-1, 6, -bisphosphate aldolase |
LMOf2365_2769 | lmo2778 | 0.99 | 1.23 | 0.57 | uncharacterized protein |
LMOf2365_0143 | lmo0125 | 1.28 | 1.62 | 1.94 | putative phage protein |
LMOf2365_2693 | lmo2713 | 1 | 1.93 | 3.08 | secreted protein, COG3786 superfamily |
LMOf2365_2188 LMOf2365_0431 LMOf2365_0037 LMOf2365_2564 LMOf2365_0346 LMOf2365_0140 LMOf2365_0900 LMOf2365_0428 LMOf2365_2387 LMOf2365_1130 LMOf2365_1885 LMOf2365_1590 |
lmo2156 lmo0412 lmo2591 lmo0328 lmo0122 lmo0881 lmo0408 lmo2416 lmo1124 lmo1857 lmo1568 |
0.97 0.97 1.03 0.91 0.92 1.17 0.98 1.04 1.06 1.04 0.99 1.07 |
2.14 1.63 1.9 1.86 1.11 1.43 1.39 1.31 1.26 0.94 1.03 1.12 |
3.58 3.65 3.89 3.96 0.62 1.73 1.8 1.82 2 2.5 2.08 1.53 |
YxeA family (DUF1093 superfamily) protein uncharacterized membrane-associated secreted protein uncharacterized protein exoglucosaminidase/muramidase uncharacterized protein putative phage tail protein, sipho_tail superfamily uncharacterized secreted protein DUF1312 superfamily secreted protein uncharacterized secreted protein uncharacterized protein DUF1250 (COG4479) superfamily protein DUF441 superfamily protein |
Note: When p value < 0.05, log ratio values are in bold type. |
F2365 Loci | Gene name | Log ratio pH 4.8/C | iTRAQ/PRM pH 4.1/C | Results pH 3.5/C | Known or predicted specific function |
LMOf2365_2702 | lmo1671 | 0.98/0.60 | 0.96/0.50 | 0.75/0.43 | zinc uptake ABC transporter, substrate-binding protein |
LMOf2365_1520 | lmo1501 | 0.94/0.59 | 0.90/0.41 | 0.75/0.32 | DUF1292 superfamily protein |
LMOf2365_0912 | rsbV | 0.98/0.64 | 0.93/0.56 | 0.80/0.54 | positive (anti-sigma B) regulatory factor (acts against RsbW) |
LMOf2365_1561 | rplU | 0.96/0.63 | 0.92/0.55 | 0.80/0.50 | ribosomal protein L21 |
LMOf2365_2532 | lmo2560 | 0.89/0.73 | 0.94/0.89 | 0.81/0.71 | RNA polymerase, delta subunit |
LMOf2365_1579 | engB | 0.98/0.73 | 0.97/0.68 | 0.81/0.55 | ribosome assembly GTP-binding protein |
LMOf2365_2603 | rplW | 0.94/0.70 | 0.94/0.60 | 0.81/0.58 | ribosomal protein L23 |
LMOf2365_2148 | lmo2115 | 0.97/0.66 | 0.93/0.59 | 0.82/0.65 | putative drug/toxic substance efflux ABC transporter, permease protein |
LMOf2365_1708 | lmo1684 | 0.93/0.68 | 0.90/0.51 | 0.82/0.57 | putative D-isomer 2-hydroxy acid dehydrogenase, NADB_Rossmann superfamily |
LMOf2365_0140 LMOf2365_0136 LMOf2365_0213 LMOf2365_0948 LMOf2365_2478 LMOf2365_0611 |
lmo0122 lmaA lmo0202 lmo0927 lmo2505 iap |
1.17/1.27 1.13/1.24 0.97/0.64 1.01/1.09 0.98/0.62 0.99/0.95 |
1.43/1.70 1.84/4.05 1.88/1.89 1.98/17.47 2.31/6.62 1.25/1.17 |
1.73/2.36 2.09/4.97 3.24/2.64 3.64/45.00 3.81/18.86 5.56/11.32 |
putative phage tail protein, sipho_tail superfamily putative phage tail protein, phage_tail_2_superfamily; antigen A protein LmaA listeriolysin O LLO exported glycerolphosphate lipoteichoic acid synthetase peptidoglycan DL-endopeptidase P45 gamma-D-glutamate-meso-diaminopimelate muropeptidase; invasion-associated protein Iap |
Note: When p value ﹤ 0.05, log ratio value are in bold type. |