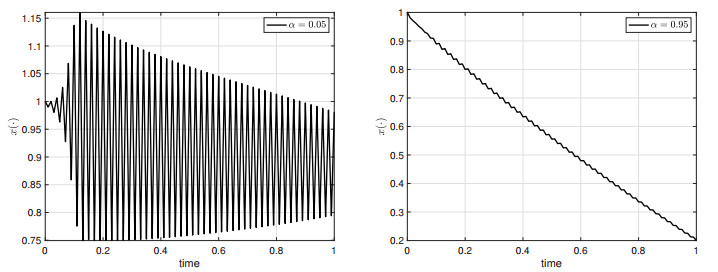
A major challenge in today's concrete construction is to lower its carbon footprint to the least possible level. This study provides insights on a new low-CO2 alternative concrete whereby glass fiber-reinforced polymer (GFRP) materials from waste wind-turbine blades (WWTB)—termed as WWTB-GFRP—was utilized as: (ⅰ) replacement of Portland cement at 0%, 10%, 20%, and as (ⅱ) lightweight aggregate replacement for natural aggregates at 0%, 50%, and 100%. The resulting WWTB-GFRP concretes were used in concrete-filled fibre-reinforced polymer (FRP) tubes (CFFTs) whereby the latter serves as a stay-in-place formwork and external reinforcement. Pristine WWTB-GFRP materials (containing wood) and processed ones (by wood removal) were investigated. Results indicate that while both WWTB-GFRP powder and aggregates adversely affect the compression resistance (due to, respectively, the retarding effect of the powder and the slippery surface of the aggregates leading to reduced bonding with the bulk cementitious matrix), the confinement of WWTB-GFRP concrete with FRP tubes offers an innovative tool to restore the strength loss. In fact, valorizing WWTB-GFRP concrete in CFFTs allowed to increase the compressive resistance by more than 100%. Interestingly, under axial compression, FRP tube confinement shifted the stress–strain response from the conventional brittle response to a ductile one whereby the confinement affected the elastic and plastic responses differently. While FRP confinement increased in the elastic limit at higher WWTB-GFRP aggregate content, it resulted in lower slope of the plastic response at higher WWTB-GFRP aggregate content. An analytical assessment demonstrated that a WWTB-GFRP aggregate content of 55% will be optimum for enhancing both elastic and plastic responses. Building upon the ACI 440.2R-17 model for predicting the compressive response of confined concrete incorporating conventional aggregates, a modified model more sensitive to GFRP aggregates and with higher predictive ability was proposed. Research outcomes will contribute to recycling waste materials while endowing further sustainability to concrete.
Citation: Dmitry Baturkin, Ousmane A. Hisseine, Radhouane Masmoudi, Arezki Tagnit-Hamou, Slimane Metiche, Luc Massicotte. Compressive behavior of FRP-tube-confined concrete short columns using recycled FRP materials from wind turbine blades: Experimental investigation and analytical modelling[J]. Clean Technologies and Recycling, 2022, 2(3): 136-164. doi: 10.3934/ctr.2022008
[1] | Shahid Mubeen, Rana Safdar Ali, Iqra Nayab, Gauhar Rahman, Thabet Abdeljawad, Kottakkaran Sooppy Nisar . Integral transforms of an extended generalized multi-index Bessel function. AIMS Mathematics, 2020, 5(6): 7531-7547. doi: 10.3934/math.2020482 |
[2] | Mohamed Abdalla . On Hankel transforms of generalized Bessel matrix polynomials. AIMS Mathematics, 2021, 6(6): 6122-6139. doi: 10.3934/math.2021359 |
[3] | D. L. Suthar, A. M. Khan, A. Alaria, S. D. Purohit, J. Singh . Extended Bessel-Maitland function and its properties pertaining to integral transforms and fractional calculus. AIMS Mathematics, 2020, 5(2): 1400-1410. doi: 10.3934/math.2020096 |
[4] | Ruma Qamar, Tabinda Nahid, Mumtaz Riyasat, Naresh Kumar, Anish Khan . Gould-Hopper matrix-Bessel and Gould-Hopper matrix-Tricomi functions and related integral representations. AIMS Mathematics, 2020, 5(5): 4613-4623. doi: 10.3934/math.2020296 |
[5] | Rana Safdar Ali, Saba Batool, Shahid Mubeen, Asad Ali, Gauhar Rahman, Muhammad Samraiz, Kottakkaran Sooppy Nisar, Roshan Noor Mohamed . On generalized fractional integral operator associated with generalized Bessel-Maitland function. AIMS Mathematics, 2022, 7(2): 3027-3046. doi: 10.3934/math.2022167 |
[6] | Gauhar Rahman, Shahid Mubeen, Kottakkaran Sooppy Nisar . On generalized k-fractional derivative operator. AIMS Mathematics, 2020, 5(3): 1936-1945. doi: 10.3934/math.2020129 |
[7] | D. L. Suthar, D. Baleanu, S. D. Purohit, F. Uçar . Certain k-fractional calculus operators and image formulas of k-Struve function. AIMS Mathematics, 2020, 5(3): 1706-1719. doi: 10.3934/math.2020115 |
[8] | Georgia Irina Oros, Gheorghe Oros, Daniela Andrada Bardac-Vlada . Certain geometric properties of the fractional integral of the Bessel function of the first kind. AIMS Mathematics, 2024, 9(3): 7095-7110. doi: 10.3934/math.2024346 |
[9] | Shahid Khan, Saqib Hussain, Maslina Darus . Inclusion relations of q-Bessel functions associated with generalized conic domain. AIMS Mathematics, 2021, 6(4): 3624-3640. doi: 10.3934/math.2021216 |
[10] | Thongchai Botmart, Soubhagya Kumar Sahoo, Bibhakar Kodamasingh, Muhammad Amer Latif, Fahd Jarad, Artion Kashuri . Certain midpoint-type Fejér and Hermite-Hadamard inclusions involving fractional integrals with an exponential function in kernel. AIMS Mathematics, 2023, 8(3): 5616-5638. doi: 10.3934/math.2023283 |
A major challenge in today's concrete construction is to lower its carbon footprint to the least possible level. This study provides insights on a new low-CO2 alternative concrete whereby glass fiber-reinforced polymer (GFRP) materials from waste wind-turbine blades (WWTB)—termed as WWTB-GFRP—was utilized as: (ⅰ) replacement of Portland cement at 0%, 10%, 20%, and as (ⅱ) lightweight aggregate replacement for natural aggregates at 0%, 50%, and 100%. The resulting WWTB-GFRP concretes were used in concrete-filled fibre-reinforced polymer (FRP) tubes (CFFTs) whereby the latter serves as a stay-in-place formwork and external reinforcement. Pristine WWTB-GFRP materials (containing wood) and processed ones (by wood removal) were investigated. Results indicate that while both WWTB-GFRP powder and aggregates adversely affect the compression resistance (due to, respectively, the retarding effect of the powder and the slippery surface of the aggregates leading to reduced bonding with the bulk cementitious matrix), the confinement of WWTB-GFRP concrete with FRP tubes offers an innovative tool to restore the strength loss. In fact, valorizing WWTB-GFRP concrete in CFFTs allowed to increase the compressive resistance by more than 100%. Interestingly, under axial compression, FRP tube confinement shifted the stress–strain response from the conventional brittle response to a ductile one whereby the confinement affected the elastic and plastic responses differently. While FRP confinement increased in the elastic limit at higher WWTB-GFRP aggregate content, it resulted in lower slope of the plastic response at higher WWTB-GFRP aggregate content. An analytical assessment demonstrated that a WWTB-GFRP aggregate content of 55% will be optimum for enhancing both elastic and plastic responses. Building upon the ACI 440.2R-17 model for predicting the compressive response of confined concrete incorporating conventional aggregates, a modified model more sensitive to GFRP aggregates and with higher predictive ability was proposed. Research outcomes will contribute to recycling waste materials while endowing further sustainability to concrete.
In this paper, we consider the minimization problem of
J(x0,u(⋅))=∫T0l(s,x(s),u(s))ds+h(x0,x(T)), | (1.1) |
subject to the Rn-valued integral-type state equation with α∈(0,1),
x(t)=x0+∫t0f(t,s,x(s),u(s))(t−s)1−αds,a.e.t∈[0,T], | (1.2) |
and the terminal state constraint
(x0,x(T))∈F⊂R2n. | (1.3) |
In (1.1)–(1.3), x(⋅)∈Rn is the state with the initial state x0∈Rn, and u(⋅)∈U is the control with U being the control space (see Section 2). Hence, we consider the following terminal state-constrained optimal control problem:
(P)infu(⋅)∈Up[0,T]J(x0,u(⋅)),subject to (1.2) and (1.3), |
where Up[0,T] is the set of admissible controls of (1.2). The problem aforementioned is referred to as (P) throughout this paper, and the precise problem statement of (P) is provided in Section 2. Note that the optimal control problems with and without state constraints capture various practical aspects of systems in science, biology, engineering, and economics [2,4,5,6,29,41,43].
The state equation in (1.2) is known as a class of Volterra integral equations. The main feature of Volterra integral equations is the effect of memories, which does not appear in ordinary (state) differential equations. In fact, Volterra integral equations of various kinds have been playing an important role in modeling and analyzing of practical physical, biological, engineering, and other phenomena that are governed by memory effects [7,17,19,32,34]. We note that one major distinction between (1.2) and other classical Volterra integral equations is that (1.2) has a kernel f(t,s,x,u)(t−s)1−α, which becomes singular at s=t. In fact, α∈(0,1) in the singular kernel f(t,s,x,u)(t−s)1−α of (1.2) determines the amount of the singularity, in which the large singular behavior occurs with small α∈(0,1). Hence, the singular kernel in (1.2) can be applied to various areas of science, engineering, finance and economics, in which certain extraordinary phenomena have to be described. For example, the model of fluid dynamics may have some singular phenomena, leading to the deformation of the corresponding dynamic equation [17,33,36].
The study of optimal control problems for various kinds of Volterra integral equations via the maximum principle have been studied extensively in the literature; see [3,12,13,18,20,22,23,30,35,39,40] and the references therein. Specifically, the first study on optimal control for Volterra integral equations (using the maximum principle) can be traced back to [40]. Several different formulations (with and without state constraints and/or delay) of optimal control for Volterra integral equations and their generalizations are reported in [3,7,12,18,20,30,35,39]. Some recent progress in different directions including stochastic frameworks can be found in [13,22,23,26,27,42]. We note that the above-mentioned existing works (with the exception of the stochastic case in [26,27,42]) considered the situation with nonsingular kernels only in Volterra integral equations, which corresponds to the case of f(t,s,x,u)=(t−s)1−αg(t,s,x,u) in (1.2). Hence, the problem settings in the earlier works can be viewed as a special case of (P).
Recently, (P) without the terminal state constraint in (1.3) was studied in [34]. Due to the presence of the singular kernel, the technical analysis including the maximum principle (without the terminal state constraint) in [34] should be different from that of the existing works mentioned above. In particular, the proof for the well-posedness and estimates of Volterra integral equations in [34, Theorem 3.1] requires a new type of Gronwall's inequality. Furthermore, the maximum principle without the terminal state constraint in [34, Theorem 4.3] needs a different duality and variational analysis via spike perturbation. More recently, the linear-quadratic optimal control problem (without the state constraint) for linear Volterra integral equations with singular kernels was studied in [28].
We note that the state equation in (1.2) is strongly related to classical state differential equations and (Caputo) fractional order differential equations. In particular, let DCα[x(⋅)] be the Caputo fractional derivative operator of order α∈(0,1) [32, Chapter 2.4]. Subsequently, by [32, Theorem 3.24 and Corollary 3.23], (1.2) becomes (Γ(⋅) denotes the gamma function)
DCα[x(⋅)](t)=f(t,x(t),u(t))⇔x(t)=x0+1Γ(α)∫t0f(s,x(s),u(s))(t−s)1−αds. | (1.4) |
In addition, when f(t,s,x,u)=(t−s)1−αg(s,x,u), (1.2) is specialized to
dx(t)dt=g(t,x(t),u(t))⇔x(t)=x0+∫t0g(s,x(s),u(s))ds. | (1.5) |
As (1.4) and (1.5) are special cases of our state equation in (1.2), the state equation in (1.2) is able to describe various types of differential equations including fractional and ordinary differential equations. We also mention that there are several different results on optimal control for fractional differential equations; see [1,8,14,25,31] and the references therein.
The aim of this paper is to obtain the Pontryagin maximum principle for (P). As noted above, since [34] did not consider the state-constrained control problem, (P) can be viewed as a generalization of [34] to the terminal state-constrained control problem. The main theoretical significances and technical difficulties are the proof of the maximum principle in Theorem 3.1, which is presented in Section 5. In particular, due to (i) the inherent complex nature of the Volterra integral equation with singular kernels, (ii) the presence of the terminal state constraint, and (iii) the generalized standing assumptions of f, our maximum principle and its detailed proof must be different from those provided in the existing literature (e.g., [12,18,22,23,30,34,35,39]). Note also that as our state equation in (1.2) includes the case of Caputo fractional differential equation in (1.4), the maximum principle in Theorem 3.1 also covers [8, Theorem 3.12]. However, as the variational analysis of this paper and that of [8] are different, the proof techniques including the characterization of the adjoint equation must be different. Indeed, the maximum principle of this paper is new in the optimal control problem context and its proof requires a different technique, compared with the existing literature.
The detailed statements of the main results of this paper are provided as follows:
● Regarding (ii), as mentioned above, the proof in Section 5 must be different from that of the unconstrained control problem in [34, Theorem 4.3]. Specifically, in contrast to [34, Theorem 4.3], due to the terminal state constraint in (1.3), we have to employ the Ekeland variational principle and the spike variation technique, together with the intrinsic properties of the distance function and the generalized Gronwall's inequality, to establish the duality analysis for Volterra-type forward variational and backward adjoint equations with singular kernels (see Lemma 5.2, Lemma 5.3 and Section 5.6). Note that this analysis leads to the desired necessary condition for optimality. Such a generalized proof of the maximum principle including the duality and variational analysis was not needed in [34, Theorem 4.3], as it did not consider the terminal state constraint in the corresponding optimal control problem. Note also that without the terminal state constraint, our maximum principle in Theorem 3.1 is reduced to the unconstrained case in [34, Theorem 4.3] (see Remark 3.1).
● As for (i), since our Volterra integral equation in (1.2) covers both singular and nonsingular kernels, the proof for the maximum principle in Section 5 should be different from that of the classical state-constrained maximum principle with the nonsingular kernel only (when f(t,s,x,u)=(t−s)1−αg(t,s,x,u) in (1.2)) studied in the existing literature (e.g., [12, Theorem 1], [22, Theorem 3.1] and [18,23,30,35,39]). In particular, due to the presence of the singular kernel in (1.2), we need a different proof for duality and variational analysis (see Lemma 3, Lemma 5.3 and Section 5.6). In addition, unlike the existing literature (e.g., [12, Theorem 1] and [22, Theorem 3.1]), our adjoint equation is obtained by the backward Volterra integral equation with the singular kernel, as a consequence of the new duality analysis with the singular kernel in Section 5.6 (see Theorem 3.1 and Remark 3.2).
● Concerning (iii), we mention that unlike the existing works for classical optimal control of Volterra integral equations with nonsingular kernels (e.g., [12,(4) and (5)], [22, page 3437 and (A3)], and [18,23,39]), our paper assumes neither the differentiability of (singular or nonsingular) kernels in (t,s,u) (time and control variables) nor the essentially boundedness of a class of admissible controls (see Remark 3.2). Hence, the detailed proof of the maximum principle provided in this paper must be different from those given in the existing literature.
As mentioned above, the main motivation of studying (P) is to provide the general maximum principle for terminal state-constrained optimal control problems of various classes of differential and integral type equations. Specifically, as mentioned above, (1.2) covers Volterra integral equations with singular and nonsingular kernels (see Remark 3.2), ordinary differential equations (see (1.5)), and Caputo-type fractional differential equations (see (1.4)). Hence, the terminal state-constrained optimal control problems for such kind of (differential and integral) state equations can be solved via the maximum principle of this paper (see Theorem 3.1). As stated above, the maximum principle for (P) has not been presented in the existing literature.
We mention that Volterra integral equations and terminal state-constrained optimal control problems can be applied to various practical applications in science, engineering, economics, and mathematical finance; see [2,4,5,6,7,17,19,29,32,34,41,43] and the references therein. In particular, Volterra integral equations can be used to study the so-called tautochrone problem in mechanical applications, the heat transfer problem in diffusion models, the shock wave problem, the renewal equation in the theory of industrial engineering, the investment problem in economics, and the problem of American option pricing [7,17,19,32,34]. The interacting biological population model can be described by Volterra integral equations [7]. Furthermore, as mentioned above, in a practical point of views, the singular kernel in (1.2) can be applied to those Volterra integral equations to study their singular and/or peculiar behavior. Hence, we can use the modeling framework of (1.2) for any differential and Volterra integral equations to capture the memory and/or singular effects, which can be observed in real world (e.g., the deformation of fluid dynamics due to singular phenomena [17,33,36], and the dependency of the current stock price on past investment strategies over a period of time [7,17]). We also note that the (terminal) state-constrained optimal control problems can be formulated for these examples (e.g., the Volterra-type renewal integral equation in industrial applications, the Volterra-type heat transfer integral equation in diffusion models, and the Volterra-type investment integral equation in economics). In fact, there are huge potential applications of optimal control problems, and their various different practical examples in science, engineering, biology, economics, and mathematical finance, including the optimal control for partial differential equations (PDEs), can be found in [2,4,5,6,29,41,43] and the references therein.
In view of the preceding discussion, we believe that the maximum principle of this paper broadens both the theoretical generality and the applicability of the domain of optimal control problems.
The rest of this paper is organized as follows. The problem statement of (P) is given in Section 2. The statement of the maximum principle for (P) is provided in Section 3. We study an example of (P) in Section 4. The proof of the maximum principle is given in Section 5. Finally, we conclude this paper in Section 6.
Let Rn be an n-dimensional Euclidean space, where ⟨x,y⟩:=x⊤y is the inner product and |x|:=⟨x,x⟩1/2 is the norm for x,y∈Rn. For A∈Rm×n, A⊤ denotes the transpose of A. Let [0,T] be a time interval such that T<∞. Let 1S(⋅) be the indicator function of any set S. In this paper, C≥0 denotes a generic constant, whose value is different from line to line. For any differentiable function f:Rn→Rl, let fx:Rn→Rl×n be the partial derivative of f with respect to x∈Rn. fx=[f⊤1,x⋯f⊤l,x]⊤ with fj,x∈R1×n, and when l=1, fx∈R1×n. For f:Rn×Rl→Rl, fx:Rn×Rl→Rl×n for x∈Rn, and fy:Rn×Rl→Rl×l for y∈Rl. For 1≤p<∞, define
● Lp([0,T];Rn): The space of functions ψ:[0,T]→Rn such that ψ is measurable and satisfies ‖ψ(⋅)‖Lp([0,T];Rn):=(∫T0|ψ(t)|pdt)1/p<∞;
● C([0,T];Rn): The space of functions ψ:[0,T]→Rn such that ψ is continuous and satisfies ‖ψ(⋅)‖∞:=supt∈[0,T]|ψ(t)|<∞.
Next, we state the precise problem statement of (P) provided in Section 1. In (1.2), α∈(0,1) is the parameter of the singularity, x(⋅)∈Rn is the state with the initial state x0∈Rn, and u(⋅)∈U is the control with U being the control space. In (1.2), f(t,s,x,u)(t−s)1−α denotes the singular kernel (with the singularity appearing at s=t), with f:[0,T]×[0,T]×Rn×U→Rn being a generator. Note that α∈(0,1) determines the level of singularity of (1.2); see Figure 1. In (1.2), f is dependent on two time parameters, t and s. While t is the outer time variable to determine the current time, s is the inner time variable describing the path or memory of (1.2) from 0 to t. In (1.1), l:[0,T]×Rn×U→R is the running cost, while h:Rn×Rn→R is the terminal cost.
Assumption 1. (i) (U,ρ) is a separable metric space. f and fx are continuous on [0,T]×[0,T] and Lipschitz continuous in (x,u)∈Rn×U with the Lipschitz constant K≥0. |f(t,s,0,u)|≤K for (t,s,u)∈[0,T]×[0,T]×U.
(ii) l and lx are continuous on [0,T] and Lipschitz continuous in (x,u)∈Rn×U with the Lipschitz constant K≥0. |l(t,0,u)|≤K for (t,u)∈[0,T]×U. h, hx0, and hx are Lipschitz continuous in both variables with the Lipschitz constant K≥0.
(iii) F is a nonempty closed convex subset of R2n.
For p>1α and u0∈U, we define the space of admissible controls for (1.2) as
Up[0,T]={u:[0,T]→U|u is measurable&ρ(u(⋅),u0)∈Lp([0,T];R+)}. |
Note that p>1α is needed for the well-posedness of (1.2), which is stated in the following lemma [34, Theorem 3.1 and Proposition 3.3]:
Lemma 2.1. Let (i) of Assumption 1 hold and p>1α. For any (x0,u(⋅))∈Rn×Up[0,T], (1.2) admits a unique solution in C([0,T];Rn), and there is a constant C≥0 such that ‖x(⋅)‖Lp([0,T];Rn)≤C(1+|x0|+‖ρ(u(⋅),u0)‖Lp([0,T];R+)).
Under Assumption 1, the main objective of this paper is to solve (P) via the Pontryagin maximum principle. Note that Assumption 1 is crucial for the well-posedness of the state equation in (1.2) by Lemma 2.1 as well as the maximum principle of (P). Assumptions similar to Assumption 1 have been used in various optimal control problems and their maximum principles; see [9,12,13,15,16,20,22,23,27,33,34,37,39,40,43] and the references therein. Note also that we do not need the differentiability of f in (t,s,u), which was assumed in the existing literature (e.g., [12,(4) and (5)], [22, page 3437 and (A3)], and [18,23,39]); see Remark 3.2.
We provide the statement of the maximum principles for (P). The proof is given in Section 5.
Theorem 3.1. Let Assumption 1 hold. Suppose that (¯u(⋅),¯x(⋅))∈Up[0,T]×C([0,T];Rn) is the optimal pair for (P), where ¯x(⋅)∈C([0,T];Rn) is the corresponding optimal state trajectory of (1.2). Then there exists a pair (λ,ξ), where λ∈R and ξ=(ξ1,ξ2)∈R2n, such that the following conditions hold:
● Nontriviality condition: (λ,ξ)≠0, where λ≥0 and ξ∈NF(¯x0,¯x(T)) with NF(x) being the normal cone to the convex set F defined in (5.1);
● Adjoint equation: p(⋅)∈Lp([0,T];Rn) is the unique solution to the following backward Volterra integral equation with the singular kernel:
p(t)=−λlx(t,¯x(t),¯u(t))⊤+∫Ttfx(r,t,¯x(t),¯u(t))⊤(r−t)1−αp(r)dr−1[0,T)(t)fx(T,t,¯x(t),¯u(t))⊤(T−t)1−α(λhx(¯x0,¯x(T))+ξ⊤2)⊤,a.e.t∈[0,T]; |
● Transversality conditions:
(i)0≤⟨ξ1,¯x0−y1⟩+⟨ξ2,¯x(T)−y2⟩,∀y=(y1,y2)∈F,(ii)∫T0p(t)dt=ξ1+ξ2+λhx0(¯x0,¯x(T))⊤+λhx(¯x0,¯x(T))⊤; |
● Hamiltonian-like maximum condition:
−λl(t,¯x(t),¯u(t))+∫Ttp(r)⊤f(r,t,¯x(t),¯u(t))(r−t)1−αdr−1[0,T)(t)(λhx(¯x0,¯x(T))+ξ⊤2)f(T,t,¯x(t),¯u(t))(T−t)1−α=maxu∈U{−λl(t,¯x(t),u)+∫Ttp(r)⊤f(r,t,¯x(t),u)(r−t)1−αdr−1[0,T)(t)(λhx(¯x0,¯x(T))+ξ⊤2)f(T,t,¯x(t),u)(T−t)1−α},a.e.t∈[0,T]. |
Remark 3.1. Without the terminal state constraint in (1.3), Theorem 3.1 holds with λ=1 and ξ=0. This is equivalent to [34, Theorem 4.3].
Remark 3.2. By taking f(t,s,x,u)=(t−s)1−αg(t,s,x,u) in Theorem 3.1, we obtain the maximum principle for the optimal control problems of classical Volterra integral equations with nonsingular kernels. Note that Theorem 3.1 is more general than the existing maximum principles for Volterra integral equations with nonsingular kernels (e.g., [12, Theorem 1], [22, Theorem 3.1] and [18,23,30,35,39]), where Theorem 3.1 assumes neither the differentiability of the corresponding kernel with respect to time and control variables nor the essential boundedness of a class of admissible controls (e.g., [12,(4) and (5)], [22, page 3437 and (A3)]). In addition, unlike the existing literature, the adjoint equation in Theorem 3.1 must be obtained in integral form, as a consequence of the new duality analysis with singular kernels in Section 5.6.
The motivation of this section is to demonstrate the applicability of Theorem 3.1 to the state-constrained linear-quadratic problem for Volterra integral equations with singular kernels. We consider the minimization of the objective functional of J(x0,u(⋅))=∫T0[x(s)+12u(s)2]ds subject to the R-valued Volterra integral equation with the singular kernel:
x(t)=x0+∫t0t2u(s)(t−s)1−αds,a.e. t∈[0,T]. | (4.1) |
For Case I, the terminal state constraint is given by (x0,x(T))∈F={x0=10,x(T)=10}⊂R2. Here, we may assume that U is an appropriate sufficiently large compact set satisfying Assumption 1. Note that this example is closely related to the (terminal) state-constrained linear-quadratic control problem, which can be applied to study the singular aspects of various applications in science, engineering, and economics.
Let T=3. By Theorem 3.1, the adjoint equation is p(t)=−λ. From the first transversality condition in (i), we have ξ∈R2. Then by the second transversality condition in (ii), ∫30p(t)dt=−3λ=ξ1+ξ2. Hence, we can choose λ=1 and ξ1=ξ2=−1.5. By the Hamiltonian-like maximum condition and the first-order optimality condition, the (candidate) optimal solution of Case I is as follows:
¯u(t)=∫3t−t2(r−t)1−αdr+t21.51[0,3)(t)(3−t)1−α,a.e. t∈[0,3]. | (4.2) |
We apply (4.2) to get the optimal state trajectory of (4.1). For Case I, the optimal state trajectory of (4.1) controlled by (4.2) when α=0.1 and α=0.5 is shown in Figure 2. Similar1y, when T=10, the optimal state trajectory is depicted in Figure 3.
We now consider Case II, where the (terminal) state constraint is generalized by
(x0,x(T))∈F={x0=10,x(T)∈[−10,10]}⊂R2. |
Similar to Case I, the adjoint equation is given by p(t)=−λ. The first transversality condition in (i) implies that ξ1∈R and ξ2=0. From the second transversality condition in (ii), it follows that ∫30p(t)dt=−3λ=ξ1. Hence, we can choose λ=1, ξ1=−3, and ξ2=0. Then analogous to (4.2), the (candidate) optimal solution of Case II is given by ¯u(t)=∫3t−t2(r−t)1−αdr, a.e. t∈[0,3]. Figure 4 depicts the optimal state trajectory (with α=0.1 and α=0.5) when T=3, and Figure 5 shows the case when T=10. Note that the numerical results of this section are obtained by the finite-difference method to approximate the solutions of (4.1) and (4.2).
Remark 4.1. The purpose of the examples in this section is to show an analytic method for applying Theorem 3.1. In the future research problem, a shooting-like method has to be developed to solve (P) numerically, which can be applied to several different complex situations of (P). This requires extending the classical approach (e.g., [11,16]) to the case of Volterra integral equations with singular kernels.
This section provides the proof of Theorem 3.1.
Recall that F is a nonempty closed convex subsets of R2n. Let dF:R2n→R+ be the standard Euclidean distance function to F defined by dF(x):=infy∈F|x−y| for x∈R2n. Note that dF(x)=0 when x∈F. Then it follows from the projection theorem [38, Theorem 2.10] that there exists a unique PF(x)∈F with PF(x):R2n→F⊂R2n, the projection of x∈R2n onto F, such that dF(x)=infy∈F|x−y|=|x−PF(x)|. By [38, Lemma 2.11], PF(x)∈F is the corresponding projection if and only if ⟨x−PF(x),y−PF(x)⟩≤0 for all y∈F, which leads to the characterization of PF(x). In view of [38, Definition 2.37], we have x−PF(x)∈NF(PF(x)) for x∈R2n, where NF(x) is the normal cone to the convex set F at a point x∈F defined by
NF(x):={y∈R2n|⟨y,y′−x⟩≤0,∀y′∈F}. | (5.1) |
Based on the distance function dF, (1.3) can be written by dF(¯x0,¯x(T))=0⇔(¯x0,¯x(T))∈F.
Lemma 5.1. [24, page 167] and [21, Proposition 2.5.4] The function dF(x)2 is Fréchet differentiable on R2n with the Fréchet differentiation of dF(x)2 at x∈R2n given by DdF(x)2(h)=2⟨x−PF(x),h⟩ for h∈R2n.
Recall that the pair (¯x(⋅),¯u(⋅))∈C([0,T];Rn)×Up[0,T] is the optimal pair of (P). Note that the pair (¯x0,¯x(T)) holds the terminal state constraint in (1.3). The optimal cost of (P) under (¯x(⋅),¯u(⋅)) can be written by J(¯x0,¯u(⋅)).
Recall the distance function dF in Section 5.1. For ϵ>0, we define the penalized objective functional as follows:
Jϵ(x0,u(⋅))=(([J(x0,u(⋅))−J(¯x0,¯u(⋅))+ϵ]+)2+dF(x0,x(T))2)12. | (5.2) |
We can easily observe that Jϵ(¯x0,¯u(⋅))=ϵ>0, i.e., (¯x0,¯u(⋅))∈Rn×Up[0,T] is the ϵ-optimal solution of (5.2). We define the Ekeland metric ˆd:(Rn×Up[0,T])×(Rn×Up[0,T])→R+:
ˆd((x0,u(⋅)),(˜x0,˜u(⋅))):=|x0−˜x0|+¯d(u(⋅),˜u(⋅)), | (5.3) |
where
¯d(u(⋅),˜u(⋅)):=|{t∈[0,T]|u(t)≠˜u(t)}|,∀u(⋅),˜u(⋅)∈Up[0,T]. | (5.4) |
Note that (Rn×Up[0,T],ˆd) is a complete metric space, and Jϵ(x0,u) in (5.2) is continuous on (Rn×Up[0,T],ˆd) [33, Proposition 3.10 and Corollary 4.2,Chapter 4].
In view of (5.2)–(5.4), we have
{Jϵ(x0,u(⋅))>0,∀(x0,u(⋅))∈Rn×Up[0,T],Jϵ(¯x0,¯u(⋅))=ϵ≤inf(x0,u(⋅))∈Rn×Up[0,T]Jϵ(x0,u(⋅))+ϵ. | (5.5) |
By the Ekeland variational principle [33, Corollary 2.2,Chapter 4], there exists a pair (xϵ0,uϵ)∈Rn×Up[0,T] such that
ˆd((xϵ0,uϵ(⋅)),(¯x0,¯u(⋅)))≤√ϵ, | (5.6) |
and for any (x0,u(⋅))∈Rn×Up[0,T],
{Jϵ(xϵ0,uϵ(⋅))≤Jϵ(¯x0,¯u(⋅))=ϵ,Jϵ(xϵ0,uϵ(⋅))≤Jϵ(x0,u(⋅))+√ϵˆd((xϵ0,uϵ(⋅)),(x0,u(⋅))). | (5.7) |
We write (xϵ(⋅),uϵ(⋅))∈C([0,T];Rn)×Up[0,T], where xϵ(⋅) is the state trajectory of (1.2) under (xϵ0,uϵ(⋅))∈Rn×Up[0,T].
The next step is to derive the necessary condition for (xϵ0,uϵ(⋅))∈Rn×Up[0,T]. To this end, we employ the spike variation technique, as U does not have any algebraic structure. Hence, standard (convex) variations cannot be used.
For δ∈(0,1), we define Eδ:={E∈[0,T]||E|=δT}, where |E| denotes the Lebesgue measure of E. For Eδ∈Eδ, we introduce the spike variation associated with uϵ:
uϵ,δ(s):={uϵ(s),s∈[0,T]∖Eδ,u(s),s∈Eδ, |
where u(⋅)∈Up[0,T]. Clearly, uϵ,δ(⋅)∈Up[0,T]. By definition of ¯d in (5.4),
¯d(uϵ,δ(⋅),uϵ(⋅))≤|Eδ|=δT. | (5.8) |
We also consider the variation of the initial state given by xϵ0+δa, where a∈Rn. Let xϵ,δ(⋅)∈C([0,T];Rn) be the state trajectory of (1.2) under (xϵ0+δa,uϵ,δ(⋅))∈Rn×Up[0,T]. By (5.7) and (5.8), it follows that
−√ϵ(|a|+T)≤1δ(Jϵ(xϵ0+δa,uϵ,δ(⋅))−Jϵ(xϵ0,uϵ(⋅))). | (5.9) |
We now study variational analysis of (5.9).
Lemma 5.2. The following result holds:
supt∈[0,T]|xϵ,δ(t)−xϵ(t)−δZϵ(t)|=o(δ), |
where Zϵ is the solution to the first variational equation related to the optimal pair (xϵ0,uϵ(⋅))∈Rn×Up[0,T] given by
Zϵ(t)=a+∫t0[fx(t,s,xϵ(s),uϵ(s))(t−s)1−αZϵ(s)ds+ˆf(t,s)(t−s)1−α]ds,a.e.t∈[0,T], |
with
ˆf(t,s):=f(t,s,xϵ(s),u(s))−f(t,s,xϵ(s),uϵ(s)). |
Proof. For δ∈(0,1), let Zϵ,δ(t):=xϵ,δ(t)−xϵ(t)δ, a.e. t∈[0,T], where based on the Taylor expansion, Zϵ,δ can be written as (note that fϵ,δx(t,s):=∫10fx(t,s,xϵ(s)+r(xϵ,δ(s)−xϵ(s)),uϵ,δ(s))dr)
Zϵ,δ(t)=a+∫t0(fϵ,δx(t,s)(t−s)1−αZϵ,δ(s)+1Eδ(s)δˆf(t,s)(t−s)1−α)ds,a.e.t∈[0,T]. |
Then we complete the proof by showing that limδ↓0supt∈[0,T]|Zϵ,δ(t)−Zϵ(t)|=0.
By Assumption 1, we have |ˆf(t,s)|≤4K+4K|xϵ(s)|+4Kρ(uϵ(s),u(s))=:˜ψ(s) and |fϵ,δx(t,s)|≤K. Based on Assumption 1, we can show that
|xϵ,δ(t)−xϵ(t)|≤b(t)+∫t0C(t−s)1−α|xϵ,δ(s)−xϵ(s)|ds, | (5.10) |
where b(t)=|δa|+∫t01Eδ(s)˜ψ(s)(t−s)1−αds. By [34, Lemma 2.3], it follows that
supt∈[0,T]|b(t)|≤|δa|+|∫t01Eδ(s)˜ψ(s)(t−s)1−αds|≤C(|δa|+|Eδ|). |
We apply the Gronwall's inequality in [34, Lemma 2.4] to get
supt∈[0,T]|xϵ,δ(t)−xϵ(t)|≤C(|δa|+|Eδ|)→0,as δ↓0 due to (5.8). | (5.11) |
From Lemma 2.1, Zϵ is a well-defined linear Volterra integral equation. Then with ˆb(t):=|a|+∫t0˜ψ(s)(t−s)1−αds and using a similar approach, we can show that
supt∈[0,T]|Zϵ(t)|≤C(|a|+‖˜ψ(⋅)‖Lp([0,T];R)). | (5.12) |
We obtain
Zϵ,δ(t)−Zϵ(t)=∫t0fϵ,δx(t,s)(t−s)1−α[Zϵ,δ(s)−Zϵ(s)]ds+∫t0(1Eδ(s)δ−1)ˆf(t,s)(t−s)1−αds+∫t0fϵ,δx(t,s)−fx(t,s,xϵ(s),uϵ(s))(t−s)1−αZϵ(s)ds. | (5.13) |
Notice that by Assumption 1, together with (5.11) and (5.12), it follows that as δ↓0,
sup(t,s)∈Δ|(fϵ,δx(t,s)−fx(t,s,xϵ(s),uϵ(s)))Zϵ(s)|≤C(|δa|+|Eδ|)→0. | (5.14) |
For convenience, let
b(1,1)(t):=∫t0|fϵ,δx(t,s)−fx(t,s,xϵ(s),uϵ(s))(t−s)1−αZϵ(s)|ds |
and
b(1,2)(t):=|∫t0(1Eδ(s)δ−1)ˆf(t,s)(t−s)1−αds|. |
By (5.14), limδ↓0supt∈[0,T]b(1,1)(t)=0. In addition, by invoking [34, Lemma 5.3.2], it follows that supt∈[0,T]b(1,2)(t)≤δ.. Then from (5.13), we have
|Zϵ,δ(t)−Zϵ(t)|≤b(1,1)(t)+δ+∫t0Zϵ,δ(s)−Zϵ(s)(t−s)1−αds. |
By applying [34, Lemma 2.4], it follows that
supt∈[0,T]|Zϵ,δ(t)−Zϵ(t)|≤C(supt∈[0,T]b(1,1)(t)+δ). |
Hence, we have
limδ↓0supt∈[0,T]|Zϵ,δ(t)−Zϵ(t)|=0. |
This completes the proof.
Based on the Taylor expansion,
1δ(J(xϵ0+δa,uϵ,δ(⋅))−J(xϵ0,uϵ(⋅)))=∫T0lϵ,δx(s)Zϵ,δ(s)ds+∫T01Eδδˆl(s)ds+hϵ,δx0(T)a+hϵ,δx(T)Zϵ,δ(T), |
where
ˆl(s):=l(s,xϵ(s),u(s))−l(s,xϵ(s),uϵ(s)), |
lϵ,δx(s):=∫10lx(s,xϵ(s)+r(xϵ,δ(s)−xϵ(s)),uϵ,δ(s))dr, |
hϵ,δx0(T):=∫10hx0(xϵ0+rδa,xϵ(T)+r(xϵ,δ(T)−xϵ(T)))dr, |
and
hϵ,δx(T):=∫10hx(xϵ0+rδa,xϵ(T)+r(xϵ,δ(T)−xϵ(T)))dr. |
Let us define
ˆZϵ(T)=∫T0lx(s,xϵ(s),uϵ(s))Zϵ(s)ds+∫T0ˆl(s)ds+hx0(xϵ0,xϵ(T))a+hx(xϵ0,xϵ(T))Zϵ(T). |
By Lemma 2.1, ˆZϵ is a well-defined Volterra integral equation. It then follows that
1δ(J(xϵ0+δa,uϵ,δ(⋅))−J(xϵ0,uϵ(⋅)))−ˆZϵ(T)=∫T0lϵ,δx(s)[Zϵ,δ(s)−Zϵ(s)]ds+∫T0[lϵ,δx(s)−lx(s,xϵ(s),uϵ(s))]Zϵ(s)ds+∫T0(1Eδδ−1)ˆl(s)ds+[hϵ,δx0(T)−hx0(xϵ0,xϵ(T))]a+hϵ,δx(T)[Zϵ,δ(T)−Zϵ(T)]+[hϵ,δx(T)−hx(xϵ0,xϵ(T))]Zϵ(T). |
Notice that limδ↓0supt∈[0,T]|Zϵ,δ(t)−Zϵ(t)|=0 by Lemma 5.2. By [33, Corollary 3.9,Chapter 4], it follows that supt∈[0,T]|∫t0(1δ1Eδ(s)−1)ˆl(s)ds|≤δ. Hence, similar to Lemma 5.2, we have
limδ↓0|1δ(J(xϵ0+δa,uϵ,δ)−J(xϵ0,uϵ))−ˆZϵ(T)|=0. | (5.15) |
From (5.9), we have
−√ϵ(|a|+T)≤1Jϵ(xϵ0+δa,uϵ,δ(⋅))+Jϵ(xϵ0,uϵ(⋅))×1δ(([J(xϵ0+δa,uϵ,δ(⋅))−J(¯x0,¯u(⋅))+ϵ]+)2−([J(xϵ0,uϵ(⋅))−J(¯x0,¯u(⋅))+ϵ]+)2+dF(xϵ0+δa,xϵ,δ(T))2−dF(xϵ0,xϵ(T))2). | (5.16) |
Let
λϵ:=[J(xϵ0,uϵ(⋅))−J(¯x0,¯u(⋅))+ϵ]+Jϵ(xϵ0,uϵ(⋅))≥0, | (5.17) |
For ξϵ∈R2n (ξϵ1∈Rn and ξϵ2∈Rn), let
ξϵ:=[ξϵ1ξϵ2]:={[xϵ0xϵ(T)]−PF(xϵ0,xϵ(T))Jϵ(xϵ0,uϵ(⋅))∈NF(PF(xϵ0,xϵ(T))),(xϵ0,xϵ(T))∉F,0∈NF(PF(xϵ0,xϵ(T))),(xϵ0,xϵ(T))∈F. | (5.18) |
Then from (5.15), (5.17), and (5.18), as δ↓0, (5.16) becomes
−√ϵ(|a|+T)≤λϵˆZϵ(T)+⟨ξϵ1,a⟩+⟨ξϵ2,Zϵ(T)⟩, | (5.19) |
where by (5.2), (5.17), and (5.18) (see also the discussion in Section 5.1),
|λϵ|2+|ξϵ|2=1. | (5.20) |
Lemma 5.3. For any (a,u(⋅))∈Rn×Up[0,T], the following results hold:
(i) limϵ↓0{|xϵ0−¯x0|+¯d(uϵ(⋅),¯u(⋅))}=0;
(ii) supt∈[0,T]|Zϵ(t)−Z(t)|=o(1),|ˆZϵ(T)−ˆZ(T)|=o(1), where
Z(t)=a+∫t0[fx(t,s,¯x(s),¯u(s))(t−s)1−αZ(s)ds+˜f(t,s)(t−s)1−α]ds,ˆZ(T)=∫T0[lx(s,¯x(s),¯u(s))Z(s)+˜l(s)]ds+¯hx0a+¯hxZ(T), |
with ˜f(t,s):=f(t,s,¯x(s),u(s))−f(t,s,¯x(s),¯u(s)), ˜l(s):=l(s,¯x(s),u(s))−l(s,¯x(s),¯u(s)), ¯hx0:=hx0(¯x0,¯x(T)), and ¯hx:=hx(¯x0,¯x(T)).
Proof. Part (i) is due to (5.6). The proof of (ii) is similar to Lemma 5.2.
We consider the limit of ϵ↓0. Instead of taking the limit with respect to ϵ↓0, let {ϵk} be the sequence of ϵ such that ϵk≥0 and ϵk↓0 as k→∞. We replace ϵ with ϵk. Then by (5.20), the sequences ({λϵk},{ξϵk}) are bounded for k≥0. From the standard compactness argument, we may extract a subsequence of {ϵk}, still denoted by {ϵk}, such that
({λϵk},{ξϵk})→(λ0,ξ0)=:(λ,ξ),as k→∞. | (5.21) |
By (5.17) and the property of the limiting normal cones [41, page 43],
λ≥0,ξ∈NF(PF(¯x0,¯x(T)))=NF(¯x0,¯x(T)). | (5.22) |
From (5.21) and (5.20), together with Lemma 5.3, as k→∞, it follows that
λϵkˆZϵk(T)≤λˆZ(T)+|ˆZϵk(T)−ˆZ(T)|+|λϵk−λ|ˆZ(T)→λˆZ(T),⟨ξϵk1,a⟩=⟨ξ1,a⟩+⟨ξϵk1,a⟩−⟨ξ1,a⟩→⟨ξ1,a⟩,⟨ξϵk2,Zϵk(T)⟩≤⟨ξ2,Z(T)⟩+|Zϵk(T)−Z(T)|+|ξϵk2−ξ2||Z(T)|→⟨ξ2,Z(T)⟩. |
Therefore, as k→∞, (5.19) becomes for any (a,u)∈Rn×Up[0,T],
0≤λˆZ(T)+⟨ξ1,a⟩+⟨ξ2,Z(T)⟩. | (5.23) |
When λ>0, the nontriviality condition in Theorem 3.1 holds. When λ=0, from (5.20)–(5.22), we must have ξ∈NF(¯x0,¯x(T)) and |ξϵk|→|ξ|=1. Hence, we have (λ,ξ)≠0, i.e., they cannot be zero simultaneously. This proves the nontriviality condition in Theorem 3.1.
Then by using the variational equations in Lemma 5.3, (5.23) becomes
0≤⟨ξ1+λ¯h⊤x0,a⟩+⟨ξ2+λ¯h⊤x,a⟩+∫T0λ˜l(s)ds+∫T0(λ¯hx+ξ⊤2)1[0,T)(s)fx(T,s,¯x(s),¯u(s))(T−s)1−αZ(s)ds+∫T0[λlx(s,¯x(s),¯u(s))Z(s)+(λ¯hx+ξ⊤2)1[0,T)(s)˜f(T,s)(T−s)1−α]ds. | (5.24) |
With the adjoint equation p(⋅)∈Lp([0,T];Rn) in Theorem 3.1, (5.24) becomes
0≤⟨ξ1+λ¯h⊤x0,a⟩+⟨ξ2+λ¯h⊤x,a⟩+∫T0λ˜l(s)ds+∫T0[−p(s)+∫Tsfx(r,s,¯x(s),¯u(s))⊤(r−s)1−αp(r)dr]⊤Z(s)ds+∫T0(λ¯hx+ξ⊤2)1[0,T)(s)˜f(T,s)(T−s)1−αds. | (5.25) |
In (5.25), the standard Fubini's formula and Lemma 5.3 lead to
∫T0[−p(s)+∫Tsfx(r,s,¯x(s),¯u(s))⊤(r−s)1−αp(r)dr]⊤Z(s)ds=∫T0−p(s)⊤[Z(s)−∫s0fx(s,r,¯x(r),¯u(r))(s−r)1−αZ(r)dr]ds=∫T0−p(s)⊤[a+∫s0˜f(s,r)(s−r)1−αdr]ds. |
Moreover, by definition of NF in (5.1), it follows that for any y=(y1,y2)∈F,
⟨ξ1,a⟩+⟨ξ2,a⟩≤⟨ξ1,¯x0−y1+a⟩+⟨ξ2,¯x(T)−y2+a⟩. |
Hence, (5.25) becomes for any (a,u)∈Rn×Up[0,T] and y=(y1,y2)∈F,
0≤⟨ξ1,¯x0−y1+a⟩+⟨ξ2,¯x(T)−y2+a⟩+λ¯hx0a+λ¯hxa−⟨∫T0p(s)ds,a⟩+∫T0−p(s)⊤∫s0˜f(s,r)(s−r)1−αdrds+∫T0(λ¯hx+ξ⊤2)1[0,T)(s)˜f(T,s)(T−s)1−αds+∫T0λ˜l(s)ds. | (5.26) |
We use (5.26) to prove the transversality conditions and the Hamiltonian-like maximum condition.
In (5.26), when u=¯u, for any y=(y1,y2)∈F, we have
0≤⟨ξ1,¯x0−y1+a⟩+⟨ξ2,¯x(T)−y2+a⟩+λ¯hx0a+λ¯hxa−⟨∫T0p(s)ds,a⟩. |
When (y1,y2)=(¯x0,¯x(T)), the above inequality holds for any a,−a∈Rn, which implies
∫T0p(s)ds=ξ1+ξ2+λ¯h⊤x0+λ¯h⊤x. | (5.27) |
Under (5.27), the above inequality becomes 0≤⟨ξ1,¯x0−y1⟩+⟨ξ2,¯x(T)−y2⟩ for any y=(y1,y2)∈F. This, together with (5.27), proves the transversality conditions in Theorem 3.1. In addition, as p(⋅)∈Lp([0,T];Rn), the nontriviality condition implies the nontriviality of the adjoint equation in Theorem 3.1.
We prove the Hamiltonian-like maximum condition in Theorem 3.1. Define
Λ(s,¯x(s),u):=−λl(s,¯x(s),u)+∫Tsp(r)⊤f(r,s,¯x(s),u)(r−s)1−αdr−1[0,T)(s)(λ¯hx+ξ⊤2)f(T,s,¯x(s),u)(T−s)1−α. |
When (y1,y2)=(¯x0,¯x(T)) and a=0 in (5.26), by the Fubini's formula, (5.26) can be written as
∫T0Λ(s,¯x(s),u(s))ds≤∫T0Λ(s,¯x(s),¯u(s))ds. | (5.28) |
As U is separable, there exists a countable dense set U0={ui,i≥1}⊂U. Moreover, there exists a measurable set Si⊂[0,T] such that |Si|=T and any t∈Si is the Lebesgue point of Λ(t,¯x(t),u(t)), i.e., it holds that limτ↓012τ∫t+τt−τΛ(s,¯x(s),u(s))ds=Λ(t,¯x(t),u(t)) [10, Theorem 5.6.2]. We fix ui∈U0. For any t∈Si, define
u(s):={¯u(s),s∈[0,T]∖(t−τ,t+τ),ui,s∈(t−τ,t+τ). |
Then (5.28) becomes
0≤limτ↓012τ∫t+τt−τ[Λ(s,¯x(s),¯u(s))−Λ(s,¯x(s),ui)]ds=Λ(t,¯x(t),¯u(t))−Λ(t,¯x(t),ui). |
This is implies that
Λ(t,¯x(t),ui)≤Λ(t,¯x(t),¯u(t)),∀ui∈U0,t∈∩i≥1Si. | (5.29) |
Since ∩i≥1Si=[0,T] by the fact that U0 is countable, Λ is continuous in u∈U, and U is separable, (5.29) implies the Hamiltonian-like maximum condition in Theorem 3.1. This completes the proof.
In this paper, we have studied the Pontryagin maximum principle for the optimal control problem of Volterra integral equations with singular kernels. The main technical difference in the proof compared with the existing literature is the variational and duality analysis. In fact, the variational analysis in our paper needs to handle the singular kernel, and the duality analysis requires to characterize the integral-type adjoint equation with the singular kernel to get the desired Hamiltonian-like maximization condition.
There are several important potential future research problems. One {direction} is to study (P) with additional generalized state constraints including the running state constraint. Another {direction} is to study the numerical aspects of solving (P) such as the shooting method and the Lagrangian collocation approach. Finally, one can extend (P) to the infinite-dimensional problem, which can be applied to studying optimal control of integral-type partial differential equations and integral-type systems with delays and singular kernels.
The authors declare they have not used Artificial Intelligence (AI) tools in the creation of this article.
This research was supported in part by the Korea Institute of Energy Technology Evaluation and Planning (KETEP) and the Ministry of Trade, Industry & Energy (MOTIE) of the Republic of Korea (RS-2023-00235742), in part by the National Research Foundation of Korea (NRF) Grant funded by the Ministry of Science and ICT, South Korea (NRF-2021R1A2C2094350) and in part by Institute of Information & communications Technology Planning & Evaluation (IITP) grant funded by the Korea government (MSIT) (No. 2020-0-01373, Artificial Intelligence Graduate School Program (Hanyang University)).
The author declares no conflict of interest in this paper.
[1] | GWEC, Global Wind Report Annual Market Update 2015. Global Wind Energy Council, 2015. Available form: https://www.gwec.net/wp-content/uploads/vip/GWEC-Global-Wind-2015-Report_April-2016_22_04.pdf. |
[2] | Beauson J, Brøndsted P (2016) Wind turbine blades: An end of life perspective, In: Ostachowicz W, McGugan M, Schröder-Hinrichs JU, et al., MARE-WINT New Materials and Reliability in Offshore Wind Turbine Technology, Cham: Springer Nature, 421–432. https://doi.org/10.1007/978-3-319-39095-6_23 |
[3] | European Council, Conclusions on 2030 Climate and Energy Policy Framework. Council of the EU, 2014. Available form: http://www.consilium.europa.eu/en/press/press-releases/2014/10/pdf/European-Council-%2823-and-24-October-2014%29-Conclusions-on-2030-Climate-and-Energy-Policy-Framework/. |
[4] | Canadian Renewable Energy Association, Wind Energy. Canadian Wind Energy Association, 2021. Available form: https://canwea.ca/wind-facts/why-wind-works/. |
[5] | Fox T (2016) Recycling wind turbine blade composite material as aggregate in concrete [Master's thesis]. Iowa State University, United States. |
[6] |
Nagle AJ, Delaney EL, Bank LC, et al. (2020) A Comparative Life Cycle Assessment between landfilling and Co-Processing of waste from decommissioned Irish wind turbine blades. J Cleaner Prod 277: 123321. https://doi.org/10.1016/j.jclepro.2020.123321 doi: 10.1016/j.jclepro.2020.123321
![]() |
[7] | D'Souza N, Gbegbaje-Das E, Shonfield P (2011) Life Cycle Assessment of Electricity Production from a Vestas V112 Turbine Wind Plant, Copenhagen: Vestas Wind Systems A/S. |
[8] |
Meira Castro AC, Carvalho JP, Ribeiro MCS, et al. (2014) An integrated recycling approach for GFRP pultrusion wastes: Recycling and reuse assessment into new composite materials using Fuzzy Boolean Nets. J Cleaner Prod 66: 420–430. https://doi.org/10.1016/j.jclepro.2013.10.030 doi: 10.1016/j.jclepro.2013.10.030
![]() |
[9] |
Correia JR, Almeida NM, Figueira JR (2011) Recycling of FRP composites: reusing fine GFRP waste in concrete mixtures. J Cleaner Prod 19: 1745–1753. https://doi.org/10.1016/j.jclepro.2011.05.018 doi: 10.1016/j.jclepro.2011.05.018
![]() |
[10] |
Asokan P, Osmani M, Price A (2010) Improvement of the mechanical properties of glass fibre reinforced plastic waste powder filled concrete. Constr Build Mater 24: 448–460. https://doi.org/10.1016/j.conbuildmat.2009.10.017 doi: 10.1016/j.conbuildmat.2009.10.017
![]() |
[11] |
Asokan P, Osmani M, Price ADF (2009) Assessing the recycling potential of glass fibre reinforced plastic waste in concrete and cement composites. J Cleaner Prod 17: 821–829. https://doi.org/10.1016/j.jclepro.2008.12.004 doi: 10.1016/j.jclepro.2008.12.004
![]() |
[12] |
Baturkin D, Hisseine OA, Masmoudi R, et al. (2021) Valorization of recycled FRP materials from wind turbine blades in concrete. Resour Conserv Recy 174: 105807. https://doi.org/10.1016/j.resconrec.2021.105807 doi: 10.1016/j.resconrec.2021.105807
![]() |
[13] |
Oliveira PS, Antunes MLP, da Cruz NC, et al. (2020) Use of waste collected from wind turbine blade production as an eco-friendly ingredient in mortars for civil construction. J Cleaner Prod 274: 122948. https://doi.org/10.1016/j.jclepro.2020.122948 doi: 10.1016/j.jclepro.2020.122948
![]() |
[14] |
Limbachiya MC (2004) Performance of recycled aggregate concrete, RILEM International Symposium on Environment-Conscious Materials and Systems for Sustainable Development, 127–136. https://doi.org/10.1617/2912143640.015 doi: 10.1617/2912143640.015
![]() |
[15] | Hofmeister M (2012) Recycling turbine blade composites: Concrete aggregate and reinforcement, Wind Energy Science, Engineering and Policy Undergraduate Research Symposium Proceedings, 9/1–9/8. |
[16] |
Boumarafi A, Abouzied A, Masmoudi R (2015) Harsh environments effects on the axial behaviour of circular concrete-filled fibre reinforced-polymer (FRP) tubes. Compos Part B-Eng 83: 81–87. https://doi.org/10.1016/j.compositesb.2015.08.054 doi: 10.1016/j.compositesb.2015.08.054
![]() |
[17] |
Abouzied A, Masmoudi R (2017) Flexural behavior of rectangular FRP-tubes filled with reinforced concrete: Experimental and theoretical studies. Eng Struct 133: 59–73. https://doi.org/10.1016/j.engstruct.2016.12.010 doi: 10.1016/j.engstruct.2016.12.010
![]() |
[18] | Mohamed H, Masmoudi R (2008) Compressive behavior of reinforced concrete-filled FRP tubes. American Concrete Institute (ACI) Special Publications, SP-257–06, ACI, Farmington Hills, Mich, 91–108. |
[19] |
Mohamed HM, Masmoudi R (2010) Axial load capacity of concrete-filled FRP tube columns: Experimental versus theoretical predictions. J Compos Constr 14: 231–243. https://doi.org/10.1061/(ASCE)CC.1943-5614.0000066 doi: 10.1061/(ASCE)CC.1943-5614.0000066
![]() |
[20] |
El-Zefzafy H, Mohamed HM, Masmoudi R (2013) Evaluation effects of the short-and long-term freeze-thaw exposure on the axial behavior of concrete-filled glass fiber-reinforced-polymer tubes. J Compos 2013: 340672. https://doi.org/10.1155/2013/340672 doi: 10.1155/2013/340672
![]() |
[21] | ASTM C1157/C1157M-20a, Standard Performance Specification for Hydraulic Cement. ASTM International, 2021. Available from: https://www.astm.org/c1157_c1157m-20a.html. |
[22] | ASTM C33/C33M-11, Standard Specification for Concrete Aggregates. ASTM International, 2011. Available from: https://www.astm.org/c0033_c0033m-11.html. |
[23] | ACI 318-19, Building Code Requirements for Structural Concrete (ACI 318-19) and Commentary. ACI Committee, 2019. Available from: https://www.concrete.org/publications/internationalconcreteabstractsportal.aspx?m=details&ID=51716937. |
[24] | ASTM C192/C192M-19, Standard Practice for Making and Curing Concrete Test Specimens in the Laboratory. ASTM International, 2016. Available from: https://www.astm.org/c0192_c0192m-19.html. |
[25] | ASTM D4464-15, Standard Test Method for Particle Size Distribution of Catalytic Materials by Laser Light Scattering. ASTM International, 2020. Available from: https://www.astm.org/d4464-15r20.html. |
[26] | ASTM D3039/D3039M-17, Standard Test Method for Tensile Properties of Polymer Matrix Composite Materials. ASTM International, 2017. Available from: https://www.astm.org/d3039_d3039m-17.html. |
[27] | ASTM D695-15, Standard Test Method for Compressive Properties of Rigid Plastics. ASTM International, 2016. Available from: https://www.astm.org/d0695-15.html. |
[28] | ASTM D2290-19a, Standard Test Method for Apparent Hoop Tensile Strength of Plastic or Reinforced Plastic Pipe. ASTM International, 2019. Available from: https://www.astm.org/Standards/D2290.htm. |
[29] | ASTM C39/C39M-16, Standard Test Method for Compressive Strength of Cylindrical Concrete Specimens. ASTM International, 2016. Available from: https://www.astm.org/c0039_c0039m-16.html. |
[30] | ACI PRC-440.2-17, Guide for the Design and Construction of Externally Bonded FRP Systems for Strengthening Concrete Structures. ACI Committee 440, 2017. Available from: https://www.concrete.org/store/productdetail.aspx?ItemID=440217&Language=English&Units=US_AND_METRIC. |
[31] |
Faella C, Lima C, Martinelli E, et al. (2016) Mechanical and durability performance of sustainable structural concretes: An experimental study. Cem Concr Compos 71: 85–96. https://doi.org/10.1016/j.cemconcomp.2016.05.009 doi: 10.1016/j.cemconcomp.2016.05.009
![]() |
[32] |
Lima C, Caggiano A, Faella C, et al. (2013) Physical properties and mechanical behaviour of concrete made with recycled aggregates and fly ash. Constr Build Mater 47: 547–559. https://doi.org/10.1016/j.conbuildmat.2013.04.051 doi: 10.1016/j.conbuildmat.2013.04.051
![]() |
[33] | ACI 213R-14, Guide for Structural Lightweight-Aggregate Concrete. ACI Committee 213, 2014. Available from: https://www.concrete.org/store/productdetail.aspx?ItemID=21314&Language=English&Units=US_AND_METRIC. |
[34] |
Hisseine OA, Omran AF, Tagnit-Hamou A (2018) Influence of cellulose filaments on cement paste and concrete. J Mater Civil Eng 30: 04018109. https://doi.org/10.1061/(ASCE)MT.1943-5533.0002287 doi: 10.1061/(ASCE)MT.1943-5533.0002287
![]() |
[35] |
Jiang T, Teng JG (2007) Analysis-oriented stress–strain models for FRP-confined concrete. Eng Struct 29: 2968–2986. https://doi.org/10.1016/j.engstruct.2007.01.010 doi: 10.1016/j.engstruct.2007.01.010
![]() |
[36] |
Yang JQ, Feng P (2020) Analysis-oriented models for FRP-confined concrete: 3D interpretation and general methodology. Eng Struct 216: 110749. https://doi.org/10.1016/j.engstruct.2020.110749 doi: 10.1016/j.engstruct.2020.110749
![]() |
[37] |
Kwan AKH, Dong CX, Ho JCM (2015) Axial and lateral stress–strain model for FRP confined concrete. Eng Struct 99: 285–295. https://doi.org/10.1016/j.engstruct.2015.04.046 doi: 10.1016/j.engstruct.2015.04.046
![]() |
[38] |
Lam L, Teng JG (2003) Design-oriented stress–strain model for FRP-confined concrete. Constr Build Mater 17: 471–489. https://doi.org/10.1016/S0950-0618(03)00045-X doi: 10.1016/S0950-0618(03)00045-X
![]() |
1. | Faïçal Ndaïrou, Delfim F. M. Torres, Pontryagin Maximum Principle for Incommensurate Fractional-Orders Optimal Control Problems, 2023, 11, 2227-7390, 4218, 10.3390/math11194218 | |
2. | Yuna Oh, Jun Moon, The infinite-dimensional Pontryagin maximum principle for optimal control problems of fractional evolution equations with endpoint state constraints, 2024, 9, 2473-6988, 6109, 10.3934/math.2024299 | |
3. | Andres Cardenas, Sergio Pulido, Rafael Serrano, Existence of optimal controls for stochastic Volterra equations, 2025, 31, 1292-8119, 30, 10.1051/cocv/2025020 |