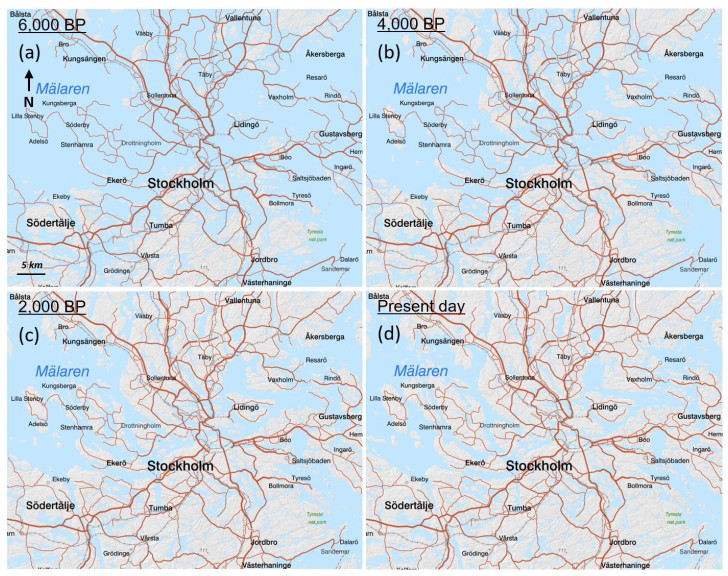
Academic interest in understanding the role of financial technology (FinTech) in sustainable development has grown exponentially in recent years. Many studies have highlighted the context, yet no reviews have explored the integration of FinTech and sustainability through the lens of the banking aspect. Therefore, this study sheds light on the literature trends associated with FinTech and sustainable banking using an integrated bibliometric and systematic literature review (SLR). The bibliometric analysis explored publication trends, keyword analysis, top publisher, and author analysis. With the SLR approach, we pondered the theory-context-characteristics-methods (TCCM) framework with 44 articles published from 2002 to 2023. The findings presented a substantial nexus between FinTech and sustainable banking, showing an incremental interest among global scholars. We also provided a comprehensive finding regarding the dominant theories (i.e., technology acceptance model and autoregressive distributed lag model), specific contexts (i.e., industries and countries), characteristics (i.e., independent, dependent, moderating, and mediating variables), and methods (i.e., research approaches and tools). This review is the first to identify the less explored tie between FinTech and sustainable banking. The findings may help policymakers, banking service providers, and academicians understand the necessity of FinTech in sustainable banking. The future research agenda of this review will also facilitate future researchers to explore the research domain to find new insights.
Citation: Md. Shahinur Rahman, Iqbal Hossain Moral, Md. Abdul Kaium, Gertrude Arpa Sarker, Israt Zahan, Gazi Md. Shakhawat Hossain, Md Abdul Mannan Khan. FinTech in sustainable banking: An integrated systematic literature review and future research agenda with a TCCM framework[J]. Green Finance, 2024, 6(1): 92-116. doi: 10.3934/GF.2024005
[1] | Quanquan Yao, Yuanlin Chen, Peiyong Zhu, Tianxiu Lu . Some stronger forms of mean sensitivity. AIMS Mathematics, 2024, 9(1): 1103-1115. doi: 10.3934/math.2024054 |
[2] | Jie Zhou, Tianxiu Lu, Jiazheng Zhao . The expansivity and sensitivity of the set-valued discrete dynamical systems. AIMS Mathematics, 2024, 9(9): 24089-24108. doi: 10.3934/math.20241171 |
[3] | Jaicer López-Rivero, Hugo Cruz-Suárez, Carlos Camilo-Garay . Nash equilibria in risk-sensitive Markov stopping games under communication conditions. AIMS Mathematics, 2024, 9(9): 23997-24017. doi: 10.3934/math.20241167 |
[4] | Liyuan Lan, Zhiyuan Zhou, Hanqing Liu, Xing Wei, Fajie Wang . An ACA-BM-SBM for 2D acoustic sensitivity analysis. AIMS Mathematics, 2024, 9(1): 1939-1958. doi: 10.3934/math.2024096 |
[5] | Turki D. Alharbi, Md Rifat Hasan . Global stability and sensitivity analysis of vector-host dengue mathematical model. AIMS Mathematics, 2024, 9(11): 32797-32818. doi: 10.3934/math.20241569 |
[6] | Yuanlin Ma, Xingwang Yu . Stochastic analysis of survival and sensitivity in a competition model influenced by toxins under a fluctuating environment. AIMS Mathematics, 2024, 9(4): 8230-8249. doi: 10.3934/math.2024400 |
[7] | Xiaofang Yang, Tianxiu Lu, Waseem Anwar . Transitivity and sensitivity for the p-periodic discrete system via Furstenberg families. AIMS Mathematics, 2022, 7(1): 1321-1332. doi: 10.3934/math.2022078 |
[8] | Chang Hou, Qiubao Wang . The influence of an appropriate reporting time and publicity intensity on the spread of infectious diseases. AIMS Mathematics, 2023, 8(10): 23578-23602. doi: 10.3934/math.20231199 |
[9] | Weili Kong, Yuanfu Shao . The effects of fear and delay on a predator-prey model with Crowley-Martin functional response and stage structure for predator. AIMS Mathematics, 2023, 8(12): 29260-29289. doi: 10.3934/math.20231498 |
[10] | Mohamed Abdelsabour Fahmy, Mohammed O. Alsulami, Ahmed E. Abouelregal . Sensitivity analysis and design optimization of 3T rotating thermoelastic structures using IGBEM. AIMS Mathematics, 2022, 7(11): 19902-19921. doi: 10.3934/math.20221090 |
Academic interest in understanding the role of financial technology (FinTech) in sustainable development has grown exponentially in recent years. Many studies have highlighted the context, yet no reviews have explored the integration of FinTech and sustainability through the lens of the banking aspect. Therefore, this study sheds light on the literature trends associated with FinTech and sustainable banking using an integrated bibliometric and systematic literature review (SLR). The bibliometric analysis explored publication trends, keyword analysis, top publisher, and author analysis. With the SLR approach, we pondered the theory-context-characteristics-methods (TCCM) framework with 44 articles published from 2002 to 2023. The findings presented a substantial nexus between FinTech and sustainable banking, showing an incremental interest among global scholars. We also provided a comprehensive finding regarding the dominant theories (i.e., technology acceptance model and autoregressive distributed lag model), specific contexts (i.e., industries and countries), characteristics (i.e., independent, dependent, moderating, and mediating variables), and methods (i.e., research approaches and tools). This review is the first to identify the less explored tie between FinTech and sustainable banking. The findings may help policymakers, banking service providers, and academicians understand the necessity of FinTech in sustainable banking. The future research agenda of this review will also facilitate future researchers to explore the research domain to find new insights.
This paper describes the geotechnical characteristics of index and deformation properties of clays in the greater Stockholm area, south-eastern Sweden. The properties of these clays vary greatly geographically and with depth, and ranges from highly organic clays with high plasticity to silty clays with low plasticity.
Clays in the Stockholm area have historically been thoroughly investigated, and the properties of these clays have had a major influence on the development on both field and laboratory tests and ground improvement techniques. Starting in the 1910s, Atterberg [1,2] established the consistency limits, to a large extent based on tests on Stockholm clays, which later was introduced in the international geotechnical community by Casagrande in 1932 [3]. In the 1910s and 1920s, the Swedish Railway Geotechnical Commission performed extensive research, including the development of the Swedish fall cone test [4], later re-developed by Hansbo in the 1950s [5]. After the establishment of the Swedish Geotechnical Institute (SGI) in 1944, extensive research was performed on both sample quality [6,7,8] and development of the in situ vane test [9] among other methods [10,11]. The trial embankments for settlement purposes at Lilla Mellösa and Skå-Edeby, established in 1945 and 1957 [12], respectively, are also located in the Stockholm area. For ground improvement purposes, prefabricated vertical drains in the 1940s, vacuum preloading in the 1950s and dry deep mixing in the 1970s were developed [13].
Despite all these research projects and extensive testing of Stockholm clays, there is no available description of its general engineering properties and the geological preconditions. The previously mentioned research describes the ground conditions at the respective sites in detail; however, since the Stockholm clays exhibit a high spatial variability the conditions do not necessarily apply elsewhere. How, for example, deformation properties depend on the index properties is also by and large unknown. The intent of this paper is thus to present a general characterization of the Stockholm clays based on the results of routine and advanced laboratory tests from a large number of sites. The characterisation covers index properties such as bulk density, natural water content, plasticity and index undrained shear strength and deformation properties from CRS (constant rate of strain) oedometer tests such as preconsolidation pressure, deformation moduli and permeability.
The vast majority of all data presented herein originates from laboratory tests performed in commercial projects. The data is collected in two generic databases containing around 3,500 and 1,600 data points, respectively, originating from around 520 different sites from a geographical area spanning roughly 4,500 km2. These databases are thus big indirect data [14] that is not relevant to one particular site but are nonetheless valuable as it represents the current state of practice. As such, it is however important to consider inherent uncertainties, e.g. spatial variability, measurement errors or correlation uncertainties [15]. For the databases presented herein, which originates from real-life commercial projects, it is important to consider sample quality, i.e. a type of measurement error. A key focus of the paper is therefore to discuss and show effects of sample quality on engineering properties and correlations. Taking this into consideration, the data is valuable to improve the geotechnical engineers understanding of soil behavior and the presented results will form a useful reference to engineers working on similar soils worldwide.
Although statistical analyses are not within the scope of this study, it is shown that several deformation properties such as preconsolidation pressure, oedometer moduli before and after the preconsolidation pressure depends on the soils natural water content and plasticity. Differences in sample quality is shown to highly affect some properties, and criterions for sample quality for this type of clay is proposed. The paper will hopefully contribute to the efforts being made within data-driven site characterisation [16,17].
Stockholm is situated between the Mälaren lake and the Baltic sea. The Scandinavian ice sheet withdrew from the area around 11,000–11,500 years before present day (BP), and the area was then completely submerged by the so-called Baltic ice lake or Yoldia sea [18]. The ice sheet eroded the bedrock and deposited a till layer. Over time, as the ice sheet withdrew, sand and silt were deposited from melting water, whilst the tectonic uplift created more land and several islands which increased in size. An overview of the tectonic uplift and the resulting shorelines over time are shown in Figure 1. As the distance from the ice front increased, the soil deposits became sedimented in an increasingly lower energy environment since coarse particles will deposit close to the river mouth and finer particles will deposit at further distance where the energy is lower. This creates a typical soil stratigraphy from coarse-grained at the bottom to more fine-grained at the top. High areas of bedrock were washed of soil and is now visible rock outcrops up to 40–60 m above sea level in general. As a result, only low-lying areas are covered in clay deposits. A geological map is shown in Figure 2, illustrating the varying soil conditions.
The increasingly lower energy environment over time has created common characteristics of Stockholm clays as illustrated in Figure 3 which shows two typical soil profiles; one without organic deposits and one with organic deposits of peat and gyttja. Figure 4 shows photographs from the varying soil deposits to illustrate the heterogeneity. In general, the grain fraction increases with depth, both with regards to the silt content in the clay fabric and with regards to the frequency and thickness of silt layers, which also includes sand layers varying from a few millimeters to several centimeters in thickness. Underneath the clay there is typically silt and sand deposit on top of the till.
Because of this geological condition, there is typically a general pattern that the water content, plasticity and organic content decreases with depth, whilst its unit weight increases with depth. Due to the topographical characteristics, there have been no considerable alteration of the landscape due to erosion or landslides, and the clay is thus normal to lightly over consolidated due to aging [20]. In urban areas there is also considerable ongoing consolidation settlements, i.e. the clay is normally consolidated.
Most of the clay is characterized as glacial clay which is seen by the varves which generally increases in thickness with depth. Typically, the more homogeneous grey post-glacial clay is limited to around 0, 5 m in thickness on land but can be several meters near-shore [21,22].
In areas without organic soil (Figure 3), the dry crust layer is usually 1–2 m thick, sometimes up to 3–4 m, created by drying and freezing cycles. The ground water table (GWT) is typically located at the bottom of the dry crust with hydrostatical conditions with depth.
In areas with organic soil the GWT is typically at the ground surface. The typical bottom soil profile in these areas are similar to that of non-organic soils, but instead of a dry-crust there is a gradual transition from gyttja (the soil is classified as gyttja when the organic content is over 6%). The organic content originates from plant and animal remains high in fat and protein, and the resulting soil is greenish and highly elastic. The gyttja was normally sedimented in waters with little movement, e.g. in bays. Over time, the uplift withdrew the water table and the soil surface was overgrown by vegetation and peat was formed, e.g. organic soil rich in carbohydrates from desiccated plant remains. Often, a thin layer of sand is seen between the gyttja and peat as a result of a small historical shoreline (beach). The typical depths of the organic soils are limited up to 3–5 m. Near-shore however, the thickness of the gyttja or organic clay can be 10–15 m.
Another feature of Stockholm's quaternary geology is the geographical differences. Typically, a higher silt content is seen towards the south, often classified as clayey silt or silt. Higher sensitivity clays are typically seen towards the northwest, where quick clay is also found, whilst towards the northeast the sulphide content increases. In Sweden, quick clay is defined as sensitivity > 50 and remoulded shear strength < 0.4 kPa.
Most of the data presented in this paper originates from tests on samples taken with the standard Swedish piston sampler [23]. This sampler has been in use since the mid-1960s as a result of an extensive research project performed by the Swedish Geotechnical Institute in the 1950s and 1960s, e.g. [6,7,8,24]. The piston sampler including some geometrical properties is shown schematically in Figure 5. The inner diameter is 50 mm (or 60 mm for a re-designed sampler in the 2020s) with an inside clearance of 0.2 mm allowing the sample to swell somewhat when entering the sampler. The angle of the cutting shoe is around 5°. There are three inner cylinders with 170 mm height which are separated in field and handled and stored separately, which enables an easy handling of the samples, although this involves trimming in the field which can be cumbersome at times. The sampler and the sampling technique are normally assumed to give samples with sufficient quality; however, this depends on the type of clay [8,25].
A few tests presented herein originates from known high-quality sampling using the mini-block sampler developed at the Norwegian University of Technology and Science (NTNU) [26], see photographs in Figure 6. The sampler uses cutting knives and water flushing at the bottom and carves out a 160 mm diameter cylindrical sample up to 350 mm height. The sampler has been tested extensively in Norway and Sweden and is shown to give high-quality samples [26,27,28,29].
All laboratory tests were performed according to their respective ISO EN standard where applicable. Only a few exceptions were made in which the most important is execution and interpretation of the Swedish fall cone test for determination of the index undrained shear strengths. Here, the older Swedish practice was followed where the execution is performed with a higher accuracy than specified in ISO EN 17892–6 [30], and a cone factor is used which is calibrated so that the strength corresponds to the in-situ vane test [31,32]. It is further corrected for effect of plasticity [31].
It is also noted that the plasticity of the clay is described by the liquid limit alone following Swedish practice, and hence the plastic limit nor the plasticity index are available. This is because it has been shown that this type of clay form a narrow band just above the A-line in the Casagrande plot [30,33,34], and there is thus a very good correlation between the liquid limit and the plasticity index. The material behavior is thus described well by the liquid limit alone. This is also seen for Norwegian clays [35]. As the testing procedure for the plastic limit in addition is somewhat cumbersome and time-consuming, especially for clays with low remoulded shear strengths which needs considerable drying, it is seldom determined in Swedish engineering practice.
The CRS oedometer test is performed according to the Swedish standard, also described by Sällfors [36,37]. Samples of 50 × 20 mm are compressed with a constant rate of strain of 0.75% per hour for at least 24 hours where 18% strain is obtained. The top of the sample is drained, and the bottom is undrained to measure the pore pressure response. Teflon oedometer rings coated with high resistance silicon fat are used to lessen the friction between the sample and the ring. All oedometer tests are performed in a climate room where the temperature is held at around 7 degrees to simulate field conditions. This has shown to be of great importance and will affect properties such as preconsolidation pressure and moduli [38,39].
The interpretation of the CRS oedometer test is illustrated in Figure 7. The method is based on Sällfors' method [36,37,40] and has been used exclusively in Sweden since the 1980s. The preconsolidation pressure (σ'c) is interpreted by fitting two straight lines of the oedometer curve before and after the apparent σ'c, i.e. Mi and ML, respectively. Between these an isosceles triangle is drawn with its based tangential to the oedometer curve as shown in the figure, and the σ'c is defined as the intersection of the baseline and the Mi-line. Sällfors [36] showed that this approach gave similar values as incremental oedometer tests and field measurements, and the method thus yields a relatively "conservative" value of σ'c to take the high rate of strain in CRS tests into consideration. It is believed that this method will give somewhat lower values than other methods as summarized in for example Paniagua et al. [41]. It is noted that this interpretation method is seldom used internationally and a direct comparison between other tests results should therefore be done with this in mind.
The moduli Mi and ML are today also in use in Norway to interpret CRS oedometer tests [42]. It should however be noted that the modulus Mi is not used for settlement calculation in Swedish practice as it is highly affected by sample disturbance and loss of radial stress. It is normally assumed that the modulus representative for field conditions, referred to Mo, is around 3–5 times the measured Mi.
The limit pressure (σ'L) is defined as the onset of modulus increase, i.e. the end of the ML-line, however, it is adjusted by a strain rate factor "c", see Figure 7. The parameter M' is equivalent to "m" in the Janbu terminology [43].
The initial permeability is defined as the intersect between the fitted straight line and the x-axis, i.e. at 0% strain, see Figure 7. The change of permeability with strain is referred to as βk.
Worth noting is the correction for false deformation which is done when referring to strain values of the oedometer curve, e.g. for assessing sample quality by strain to in-situ effective stresses [44]. This false deformation typically originates from poor alignment between the sample and the filter or a thin water film at the undrained bottom of the sample, which lately also is noted on Norwegian clays [45]. Normally, this can be avoided by gently pressing the top filter stone onto the sample before mounting the piston and completing the test set up, however, this is not always successful. It is however believed by the authors that this false deformation does not affect the interpreted values.
The data presented herein are divided into two generic databases. The first is labelled CLAY/7/3578 according to [46] and includes results from routine analyses, i.e., depth, bulk density, water content, liquid limit, intact undrained shear strength from fall cone tests and remoulded shear strength from fall cone tests and sensitivity. Basic values are summarized in Table 1. The second database is labelled CLAY/16/1675, which, in addition to results from routine analyses, also includes results from the CRS oedometer tests, i.e., preconsolidation pressure, several moduli and permeability properties. Basic values are summarized in Table 2.
Min. | Average | Max. | Unit | |
Density | 1.15 | 1.69 | 2.13 | t/m3 |
Water content | 19 | 56 | 357 | % |
Liquid limit, wL | 18 | 52 | 309 | % |
Remoulded shear strength, cur,FC | 0.07 | 1.4 | 28 | kPa |
Index shear strength, cu,FC | 1.0 | 14.8 | 114 | kPa |
Sensitivity, St | 1.8 | 17 | 186 | - |
Min. | Average | Max. | Unit | |
Preconsolidation pressure, σ'c | 8 | 61 | 305 | kPa |
Modulus, Mi | 133 | 3,216 | 47,000 | kPa |
Modulus, ML | 65 | 652 | 5,000 | kPa |
Modulus number M' | 5.5 | 15.7 | 34 | - |
Limit pressure, σ'L | 19 | 64 | 550 | kPa |
Permeability, k | 8.5E-11 | 7.1E-10 | 2.3E-08 | m/s |
Change of k with strain, βk | 1.5 | 4.0 | 8.4 | - |
Coefficient of consolidation, cv,NC | 0.05 | 0.73 | 20.14 | m2/s |
Histograms of selected index and deformation properties are shown in Figure 8 and Figure 9, respectively. A total of 92% of all samples are taken between 1 and 10 m depth from the ground surface which also reflects the typical maximum clay depths in the Stockholm area, although samples are not always taken all the way to the bottom. Notably, there are a few areas with up to around 30 m of clay. Most of the samples have natural water contents (wN) between 40 and 80% with an average of 56%. The liquid limit is commonly in the same range as the natural water content, which is typical for Stockholm clays except when the sensitivity is high. The index undrained strength (cu,FC), determined by the Swedish fall cone test [31], shows most values from 5 to 20 kPa with an average value of about 15 kPa. This strength is equivalent to the direct undrained strength according to the ADP-concept of active-direct-passive shear strengths [44,47]. The interpreted preconsolidation pressures (σ'c) ranges between 8 and 305 kPa with an average of around 61 kPa and oedometer modulus in the normally consolidated range (ML) between 65 and 5,000 kPa with an average of 650 kPa.
It is noted that the range of e.g. index properties, strength and preconsolidation pressure are similar to that of for example Finnish clays [48], but are low in comparison to many other natural clays [49].
Index properties from the CLAY/7/3578 database are plotted against depth in Figure 10. Average and median values are plotted together with the 10th and 90th percentile to give indications of the variability. Most properties have a close to normal distribution at each depth interval. Only values between 2 m and 10 m are plotted to avoid non-representative data from desiccated samples at depths above 2 m and due to too few data points below 10 m.
It is seen that the bulk density increases somewhat with depth and that the water content along with the liquid limit decreases somewhat. This is expected from the previous geological description with decreasing grain fraction with depth. The index undrained shear strength determined by the fall cone test increases slightly, and it is seen that the variability decreases with depth which most probably is a result of the effect of sites with organic soils. The remoulded shear strength decreases with depth between 2 and around 4 m, probably due to desiccation effects at the top. As a result, the sensitivity increases with depth and ranges from around 10 at 2 m depth to around 20 at 10 m depth.
Similar plots for deformation properties from the CLAY/16/1675 database are shown in Figure 11. The preconsolidation pressure increases from around 40 kPa at 2 m depth to around 80 at 10 m depth. The rate of increase is around 5.4 kPa/m, slightly less than the average increase of in-situ effective stress assuming an average density of 1.69 t/m3 (Table 1) and hydrostatic pore water pressure, giving a 6.9 kPa/m increase. There is no apparent effect of desiccation at the top, most probably since oedometer tests normally are avoided if there are visual signs of desiccation.
The modulus in the normally consolidated range (ML) and the modulus number M' also exhibits a slight increase with depth. The variability of ML is however large. The lowest values are around and slightly below 100 kPa, illustrating the high compressibility of clays in some areas. From the authors' experience, the lowest values of ML are seen on samples of organic clays and inorganic clays with water contents around 80–100%.
In Figure 11 it is also seen that the permeability decreases somewhat with depth, despite the expected increasing grain fraction, i.e. decreasing clay fraction, with depth. It has however been shown that the permeability is not affected by clay fraction alone, but also highly depends on plasticity, clay fabric etc. [50]. The change of permeability with strain (βk) is however fairly constant with depth with an average around 4. The coefficient of consolidation at normally consolidated state (cv,NC) is also fairly constant with an average around 0.5–1.0 m2/year, although with considerable variability. The values of is relatively low compared to many other clays.
The moduli Mi, ML and M' and permeability properties are plotted against natural water contents (wN) in Figure 12. The figure distinguishes between samples taken with the standard piston sampler and the mini block sampler to illustrate effects of sample quality. Despite considerable scatter, it is seen that the moduli Mi and ML decreases with increasing water contents. For water contents above 100–120%, i.e. typically organic clays and gyttja for Swedish clays [21,32,33], ML never seem to exceed 250 kPa. Similarly, the Mi is lower than 500. The samples of known high quality, e.g. the mini-block samples, show higher Mi and lower ML. This is discussed later in the paper.
The modulus number M' decreases with increasing water content, and even though the scatter is large the data follows the empirical correlation given by Larsson and Sällfors [37] as well that seen on Norwegian clays [42]. All these moduli seem to have similar correlations with the liquid limit.
The data presented in these figures naturally exhibit a large scatter since it originates from the tests on the highly heterogeneous Stockholm clay from a large number of sites. Based on the authors experience it is however often possible to find relatively similar results from limited geographical areas. An example is shown in Figure 13. Despite the large variation in natural water content there is a clear correlation between both ML and water content and Mi and the preconsolidation pressure.
For the permeability properties such as initial permeability and change of permeability with strain there seem to be a slight change with water content; however, there is considerable scatter and no conclusion can be drawn based on these results. This was also noted by Tavenas et al. [50] who describes that the permeability is also highly affected by clay fabric and plasticity. The coefficient of consolidation decreases with increasing water content, which is because the modulus ML decreases with increasing water content. For change of permeability with strain (βk) there is no established Swedish correlation, but the values are similar to those for Norwegian clays [42].
Figure 14 plots the moduli Mi and ML with the preconsolidation pressure and the difference between the limit stress and preconsolidation pressure, i.e. the length of the ML-line, respectively. There is a clear correlation between Mi and the preconsolidation pressure where Mi ranges between around 15 and 70 times the preconsolidation pressure, on average Mi≈30×σ'c. If one assumes that the Mi underestimates the in-situ modulus M0 by a factor 3 to 5, as explained in chapter 3, the correlation 90×σ'c≲M0≲150×σ'c can be used for settlement calculations. This is much higher than empirical correlations given by Larsson et al. [51]. Correlating with the index undrained shear strength one obtains on average Mi≈145×cu, i.e. 400×cu≲M0≲700×cu for settlement calculations.
There is also an increase of ML with increasing preconsolidation pressure. The scatter is however much larger, and no conclusive correlations can be given. This is unfortunate since ML, i.e., settlement modulus in the normally consolidated range, governs much of the total settlements for the typical normally consolidated Stockholm clay. It is however generally seen that it decreases with increasing sample quality.
Figure 14d shows a correlation between the length of the ML-line and the absolute value of ML -the longer the ML-line, the higher ML. The authors are not aware of any previous research on this, and with the current available data is has not been possible to analyse this behavior further. The reason for this is therefore unknown, but it might indicate that the linear part of the oedometer curve after the preconsolidation pressure is limited by a strain interval instead of a stress interval.
Tavenas et al. [50] and Leroueil et al. [52] compiled results from oedometer tests on Canadian and some Swedish clays with regards to e.g. the permeability change index Ck, defined as the slope of a curve in a void ratio vs. permeability plot (Ck=(1+e0/βk). It was concluded that Ck can be simplified to 0.5 times the initial void ratio, e0 with a possible lower bound of around 0.4 times e0, also noted by [53].
Figure 15 shows a plot of the Ck vs. initial void ratios for all tests. Here, the initial void ratio is calculated by the natural water content assuming full saturation and a grain density of 2.65 [54]. It is seen that the correlation Ck≈0.4×e0 is a better fit for Stockholm clays.
The anisotropy has investigated on samples from a few sites where samples were extracted using the redesigned standard piston sampler with 60 mm inner diameter. This allows for trimming of 20 mm high specimens for CRS oedometer tests in both vertical and horizontal direction. Preconsolidation pressure evaluated from tests on the same sample depth indicates the degree of anisotropy, i.e. values of K0, assuming that the OCR is equal in both vertical and horizontal direction. Results from tests on samples south of Stockholm are shown in Figure 16 along with an empirical correlation by Larsson et al. [55] given as K0,NC=0.31+0.71×(wL−0.2) which is valid for normally consolidated clays. Despite few data points and a considerable scatter, the data indicates a slight dependency of the liquid limit on K0 and a linear regression is similar to the empirical correlation by [55].
This dependence of plasticity on K0 have also been shown by other researchers on Swedish clays [56], whilst tests on Norwegian clays have shown negligible effects of plasticity [57]. The Norwegian clays, however, had liquid limits ranging between 30 and 74 [57], a far smaller range than the Swedish clays ranging from around 40 to 150 [55,56], and between around 40 and 90 in this study (Figure 16). The difference is thus probably due to the relatively narrow range of liquid limits for Norwegian clays where any plasticity effect is hidden by the typical scatter. For both clays, K0 is highly dependent on OCR, however, this effect is not investigated herein.
Sample quality have been shown to be of vital importance for establishing relevant engineering properties [27,58,59,60,61]. Sample disturbance, i.e. altered properties of the soil sample from its in-situ state to its state at the time of laboratory testing, can be categorized as follows: changes in effective stresses, changes in water content, structural changes and chemical changes [62].
A few studies on sample quality and comparative testing between the standard piston sampler and block samplers on Swedish clays have been made [25,27,29,63,64]. These have shown varying results, ranging from large differences in quality to in practice equal quality between different samplers. In general, it is seen that low plastic clays are more prone to disturbance than high-plastic clays. Results from [27] is presented in Figures 17 and 18, see location in Figure 2. Examples of comparative CRS oedometer tests results are shown in Figure 17. These clearly indicates large differences in both the oedometer curves and moduli curves, affecting several properties such as preconsolidation pressure and moduli. Figure 19 show resulting preconsolidation pressures and ML against depth in one location. There is clearly a large difference in both these properties. It can be generalized that with increasing sample quality:
• The initial modulus Mi increases;
• The modulus ML decreases;
• The difference between the limit stress (σ'L) and the preconsolidation pressure (σ'c), i.e. the length of the linear part of the oedometer curve (the ML-line), decreases;
• The modulus M' is not affected;
• Often higher preconsolidation pressure, especially for large sampling depths;
• Less scatter of several properties, e.g. preconsolidation pressure.
Most of these findings are also supported by comparative sampling with the standard piston sampler and the mini block sampler performed by SGI at the Skå-Edeby test field [65].
There are several methods proposed to quantify sample quality, e.g., change in void ratio in relation to initial void ratio during reconsolidation to in situ effective stress [61], volumetric strain to in situ effective stress [66], moduli quotient between Mi and ML [42] and shear wave velocity and suction [67]. Recommendations from SGI, most often are used in Sweden to assess sample quality, involve a redevelopment of the change in void ratio in relation to initial void ratio proposed by Lunne et al. [61]. Here, the change in void ratio have been re-calculated to total strain taking the "false" deformation into account (Figure 7). By plotting this against the natural water content instead of initial void ratio one can use the Lunne et al. [61] criteria for assessing sample quality, as described by Larsson et al. [51]. In general, the lower strain, the higher quality since the clay structure is less altered.
There is no available data on in situ effective stresses in the database CLAY/16/1675, however, this procedure has been simplified by the strain to 85% of the preconsolidation pressure (ϵ0.85σ'c), i.e. strain to in situ effective stress if OCR is 1/0.85 ≈ 1.15–1.2, which are typical for Stockholm clays. These values have been plotted against natural water content and the difference between the limit stress and the preconsolidation pressure in Figure 19a and b. There seems to be no correlation, which supports the authors' experience that the strain to in situ effective stresses are not applicable to CRS oedometer tests. For example, a stiff response (high Mi) with a low strain value can be obtained on a visually clearly disturbed clay sample with desiccation effects, and vice versa for extremely soft samples which inherently have considerably lower values of Mi and hence large strains to in situ stresses.
The methodology proposed by Karlsrud and Hernandez-Martinez [42], i.e., criteria for the moduli quotient Mi/ML, herein referred to the sample quality index (SQI), have been assessed for the Stockholm clays. Figure 19 (c) and (d) plots this SQI against the natural water content and the difference between the limit stress and the preconsolidation pressure, respectively. It is seen that the SQI in general increases with increasing water content, and a decrease with increasing difference between limit stress and the preconsolidation pressure. Karlsrud and Hernandez-Martinez [42] proposed the following criteria for sample quality: < 1 very poor; 1–1.5 poor; 1.5–2 good to fair and; > 2 very good to excellent sample quality. From Figure 19 it is obvious that these criteria are not suitable for Stockholm clays since most samples would be classified as "very good to excellent", which is not the case. Notably, from the authors' experience, these criteria are not suitable for any type of Swedish clays along the East or West coast.
Unfortunately, there is no "perfect" value of the SQI (Mi/ML), compared to an ideal 0% strain to in situ effective stress for a "perfect" sample with no alteration of the clay structure. The higher Mi/ML, the higher quality; however, the highest possible value will differ between the exact type of clay. Any type of criteria on sample quality based on this methodology will thus have to be based on a relative distribution for different types of clays. Figure 20 shows histograms of Mi/ML, and strain to 85% of the preconsolidation pressure. Based on this and Figure 19 sample quality criteria in Table 3 is proposed for Stockholm clays. The table also notes its respective frequency is the database CLAY/16/1675. Around 20% of all samples are here classified having a poor quality, whilst around 45% are classified having good to very good quality. Most of the mini block samples are thus good to very good quality. It is believed that this simple methodology with these criteria is suitable in engineering practice for this type of clay.
Mi/ML | Frequency | |
Poor quality | < 2 | ~20% |
Fair quality | 2 – 4 | ~35% |
Good quality | 4 – 8 | ~32% |
Very good quality | > 8 | ~13% |
This paper presents data from two generic databases to characterise the index and deformation properties of Stockholm clays. It is found that the clays are highly heterogeneous with highly variable natural water contents, plasticity and other index properties, as well as yield stresses and moduli. Due to its geological history, the Stockholm clays are normally consolidated with low index shear strengths and high compressibility.
It is found that the natural water content, or the liquid limit, affects the moduli, such as Mi and ML, and the modulus number M' with decreasing moduli with increasing water content. The initial permeability is however not particularly affected by the water content, except the coefficient of consolidation which decreases with increasing water content (as an effect of ML). The permeability change index is found to be around 0.4 times the initial void ratio.
The modulus Mi is further highly correlated to the preconsolidation pressure, however these values are not representative for field conditions. The modulus ML is also increasing with increasing preconsolidation pressure, but there is a considerable scatter. The modulus ML also increases with increasing difference between the limit stress and preconsolidation pressure.
Effects of samples quality is analyzed. It is found that the quality affects several deformation properties, especially preconsolidation pressure and the moduli Mi and ML, however, M' is not affected. A methodology of assessing sample quality using the sample quality index Mi/ML is found to be suitable for CRS oedometer tests; however, it is noted that previously recommended criteria is not applicable for Stockholm clays, and new criteria are thus proposed.
The authors are thankful to the meticulous work by the laboratory technicians at LabMind geotechnical laboratory in Stockholm, Sweden.
The authors are also grateful to Jean-Sébastien L'Heureux at the Norwegian Geotechnical Institute and the anonymous reviewers for reviewing the manuscript and giving valuable comments.
The authors declare no conflict of interest.
[1] |
Abdul-Rahim R, Bohari SA, Aman A, et al. (2022) Benefit–risk perceptions of FinTech adoption for sustainability from bank consumers' perspective: The moderating role of fear of COVID-19. Sustainability 14: 8357. https://doi.org/10.3390/su14148357 doi: 10.3390/su14148357
![]() |
[2] |
Aduba JJ (2021) On the determinants, gains and challenges of electronic banking adoption in Nigeria. Int J Soc Econ 48: 1021–1043. https://doi.org/10.1108/IJSE-07-2020-0452 doi: 10.1108/IJSE-07-2020-0452
![]() |
[3] |
Alaabed A, Masih M, Mirakhor A (2016) Investigating risk shifting in Islamic banks in the dual banking systems of OIC member countries: an application of two-step dynamic GMM. Risk Manage 18: 236–263. https://doi.org/10.1057/s41283-016-0007-3 doi: 10.1057/s41283-016-0007-3
![]() |
[4] |
Aracil E, Nájera-Sánchez JJ, Forcadell FJ (2021) Sustainable banking: A literature review and integrative framework. Financ Res Lett 42: 101932. https://doi.org/10.1016/j.frl.2021.101932 doi: 10.1016/j.frl.2021.101932
![]() |
[5] | Ashrafi DM, Dovash RH, Kabir MR (2022) Determinants of fintech service continuance behavior: moderating role of transaction security and trust. J Global Bus Technol 18. Available from: https://www.proquest.com/docview/2766511260?pq-origsite = gscholar & fromopenview = true & sourcetype = Scholarly%20Journals. |
[6] |
Ashta A, Herrmann H (2021) Artificial intelligence and fintech: An overview of opportunities and risks for banking, investments, and microfinance. Strateg Change 30: 211–222. https://doi.org/10.1002/jsc.2404 doi: 10.1002/jsc.2404
![]() |
[7] |
Banna H, Hassan MK, Ahmad R, et al. (2022) Islamic banking stability amidst the COVID-19 pandemic: the role of digital financial inclusion. Int J Islamic Middle 15: 310–330. https://doi.org/10.1108/IMEFM-08-2020-0389 doi: 10.1108/IMEFM-08-2020-0389
![]() |
[8] |
Boratyńska K (2019) Impact of digital transformation on value creation in Fintech services: an innovative approach. J Promot Manage 25: 631–639. https://doi.org/10.1080/10496491.2019.1585543 doi: 10.1080/10496491.2019.1585543
![]() |
[9] |
Bose S, Khan HZ, Rashid A, et al. (2018) What drives green banking disclosure? An institutional and corporate governance perspective. Asia Pac J Manage 35: 501–527. https://doi.org/10.1007/s10490-017-9528-x doi: 10.1007/s10490-017-9528-x
![]() |
[10] |
Brahmi M, Esposito L, Parziale A, et al. (2023) The role of greener innovations in promoting financial inclusion to achieve carbon neutrality: an integrative review. Economies 11: 194. https://doi.org/10.3390/economies11070194 doi: 10.3390/economies11070194
![]() |
[11] |
Çera G, Phan QPT, Androniceanu A, et al. (2020) Financial capability and technology implications for online shopping. EaM Ekon Manag. https://doi.org/10.15240/tul/001/2020-2-011 doi: 10.15240/tul/001/2020-2-011
![]() |
[12] |
Chang HY, Liang LW, Liu YL (2021) Using environmental, social, governance (ESG) and financial indicators to measure bank cost efficiency in Asia. Sustainability 13: 11139. https://doi.org/10.3390/su132011139 doi: 10.3390/su132011139
![]() |
[13] |
Coffie CPK, Zhao H, Adjei Mensah I (2020) Panel econometric analysis on mobile payment transactions and traditional banks effort toward financial accessibility in Sub-Sahara Africa. Sustainability 12: 895. https://doi.org/10.3390/su12030895 doi: 10.3390/su12030895
![]() |
[14] |
Cumming D, Johan S, Reardon R (2023) Global fintech trends and their impact on international business: a review. Multinatl Bus Rev 31: 413–436. https://doi.org/10.1108/MBR-05-2023-0077 doi: 10.1108/MBR-05-2023-0077
![]() |
[15] |
Danladi S, Prasad M, Modibbo UM, et al. (2023) Attaining Sustainable Development Goals through Financial Inclusion: Exploring Collaborative Approaches to Fintech Adoption in Developing Economies. Sustainability 15: 13039. https://doi.org/10.3390/su151713039 doi: 10.3390/su151713039
![]() |
[16] |
Davis FD, Bagozzi RP, Warshaw PR (1989) User acceptance of computer technology: A comparison of two theoretical models. Manage Sci 35: 982–1003. https://doi.org/10.1287/mnsc.35.8.982 doi: 10.1287/mnsc.35.8.982
![]() |
[17] |
Dewi IGAAO, Dewi IGAAP (2017) Corporate social responsibility, green banking, and going concern on banking company in Indonesia stock exchange. Int J Soc Sci Hum 1: 118–134. https://doi.org/10.29332/ijssh.v1n3.65 doi: 10.29332/ijssh.v1n3.65
![]() |
[18] |
Diep NTN, Canh TQ (2022) Impact analysis of peer-to-peer Fintech in Vietnam's banking industry. J Int Stud 15. https://doi.org/10.14254/2071-8330.2022/15-3/12 doi: 10.14254/2071-8330.2022/15-3/12
![]() |
[19] |
Dong Y, Chung M, Zhou C, et al. (2018) Banking on "mobile money": The implications of mobile money services on the value chain. Manuf Serv Oper Manag. https://doi.org/10.1287/msom.2018.0717 doi: 10.1287/msom.2018.0717
![]() |
[20] |
Eisingerich AB, Bell SJ (2008) Managing networks of interorganizational linkages and sustainable firm performance in business‐to‐business service contexts. J Serv Mark 22: 494–504. https://doi.org/10.1108/08876040810909631 doi: 10.1108/08876040810909631
![]() |
[21] |
Ellili NOD (2022) Is there any association between FinTech and sustainability? Evidence from bibliometric review and content analysis. J Financ Serv Mark 28: 748–762. https://doi.org/10.1057/s41264-022-00200-w doi: 10.1057/s41264-022-00200-w
![]() |
[22] |
Fenwick M, Vermeulen EP (2020) Banking and regulatory responses to FinTech revisited-building the sustainable financial service'ecosystems' of tomorrow. Singap J Legal Stud 2020: 165–189. https://doi.org/10.2139/ssrn.3446273 doi: 10.2139/ssrn.3446273
![]() |
[23] |
Gangi F, Meles A, Daniele LM, et al. (2021) Socially responsible investment (SRI): from niche to mainstream. The Evolution of Sustainable Investments and Finance: Theoretical Perspectives and New Challenges, 1–58. https://doi.org/10.1007/978-3-030-70350-9_1 doi: 10.1007/978-3-030-70350-9_1
![]() |
[24] |
Gbongli K, Xu Y, Amedjonekou KM, et al. (2020) Evaluation and classification of mobile financial services sustainability using structural equation modeling and multiple criteria decision-making methods. Sustainability 12: 1288. https://doi.org/10.3390/su12041288 doi: 10.3390/su12041288
![]() |
[25] |
Goodell JW, Kumar S, Lim WM, et al. (2021) Artificial intelligence and machine learning in finance: Identifying foundations, themes, and research clusters from bibliometric analysis. J Behav Exp Financ 32: 100577. https://doi.org/10.1016/j.jbef.2021.100577 doi: 10.1016/j.jbef.2021.100577
![]() |
[26] |
Gozman D, Willcocks L (2019) The emerging Cloud Dilemma: Balancing innovation with cross-border privacy and outsourcing regulations. J Bus Res 97: 235–256. https://doi.org/10.1016/j.jbusres.2018.06.006 doi: 10.1016/j.jbusres.2018.06.006
![]() |
[27] |
Gruin J, Knaack P (2020) Not just another shadow bank: Chinese authoritarian capitalism and the 'developmental'promise of digital financial innovation. New Polit Econ 25: 370–387. https://doi.org/10.1080/13563467.2018.1562437 doi: 10.1080/13563467.2018.1562437
![]() |
[28] |
Guang-Wen Z, Siddik AB (2023) The effect of Fintech adoption on green finance and environmental performance of banking institutions during the COVID-19 pandemic: the role of green innovation. Environ Sci Pollut Res 30: 25959–25971. https://doi.org/10.1007/s11356-022-23956-z doi: 10.1007/s11356-022-23956-z
![]() |
[29] |
Guo Y, Holland J, Kreander N (2014) An exploration of the value creation process in bank-corporate communications. J Commun manage 18: 254–270. https://doi.org/10.1108/JCOM-10-2012-0079 doi: 10.1108/JCOM-10-2012-0079
![]() |
[30] | Hassan MK, Rabbani MR, Ali MAM (2020) Challenges for the Islamic Finance and banking in post COVID era and the role of Fintech. J Econ Coop Dev 41: 93–116. Available from: https://www.proquest.com/docview/2503186452?pq-origsite = gscholar & fromopenview = true & sourcetype = Scholarly%20Journals. |
[31] | Hassan SM, Rahman Z, Paul J (2022) Consumer ethics: A review and research agenda. Psychol Market 39: 111–130. |
[32] |
He J, Zhang S (2022) How digitalized interactive platforms create new value for customers by integrating B2B and B2C models? An empirical study in China. J Bus Res 142: 694–706. https://doi.org/10.1016/j.jbusres.2022.01.004 doi: 10.1016/j.jbusres.2022.01.004
![]() |
[33] |
Hommel K, Bican PM (2020) Digital entrepreneurship in finance: Fintechs and funding decision criteria. Sustainability 12: 8035. https://doi.org/10.3390/su12198035 doi: 10.3390/su12198035
![]() |
[34] |
Hyun S (2022) Current Status and Challenges of Green Digital Finance in Korea. Green Digital Finance and Sustainable Development Goals, 243–261. https://doi.org/10.1007/978-981-19-2662-4_12 doi: 10.1007/978-981-19-2662-4_12
![]() |
[35] |
Ⅱ WWC, Demrig I (2002) Investment and capitalisation of firms in the USA. Int J Technol Manage 24: 391–418. https://doi.org/10.1504/IJTM.2002.003062 doi: 10.1504/IJTM.2002.003062
![]() |
[36] |
Iman N (2018) Is mobile payment still relevant in the fintech era? Electron Commer Res Appl 30: 72–82. https://doi.org/10.1016/j.elerap.2018.05.009 doi: 10.1016/j.elerap.2018.05.009
![]() |
[37] |
Ji F, Tia A (2022) The effect of blockchain on business intelligence efficiency of banks. Kybernetes 51: 2652–2668. https://doi.org/10.1108/K-10-2020-0668 doi: 10.1108/K-10-2020-0668
![]() |
[38] |
Jibril AB, Kwarteng MA, Botchway RK, et al. (2020) The impact of online identity theft on customers' willingness to engage in e-banking transaction in Ghana: A technology threat avoidance theory. Cogent Bus Manag 7: 1832825. https://doi.org/10.1080/23311975.2020.1832825 doi: 10.1080/23311975.2020.1832825
![]() |
[39] |
Khan A, Goodell JW, Hassan MK, et al. (2022) A bibliometric review of finance bibliometric papers. Financ Res Lett 47: 102520. https://doi.org/10.1016/j.frl.2021.102520 doi: 10.1016/j.frl.2021.102520
![]() |
[40] |
Khan HU, Sohail M, Nazir S, et al. (2023) Role of authentication factors in Fin-tech mobile transaction security. J Big Data 10: 138. https://doi.org/10.1186/s40537-023-00807-3 doi: 10.1186/s40537-023-00807-3
![]() |
[41] |
Kumar S, Lim WM, Sivarajah U, et al. (2023) Artificial intelligence and blockchain integration in business: trends from a bibliometric-content analysis. Inform Syst Front 25: 871–896. https://doi.org/10.1007/s10796-022-10279-0 doi: 10.1007/s10796-022-10279-0
![]() |
[42] |
Kumari A, Devi NC (2022) The Impact of FinTech and Blockchain Technologies on Banking and Financial Services. Technol Innov Manage Rev 12. https://doi.org/10.22215/timreview/1481 doi: 10.22215/timreview/1481
![]() |
[43] |
Lai X, Yue S, Guo C, et al. (2023) Does FinTech reduce corporate excess leverage? Evidence from China. Econ Anal Policy 77: 281–299. https://doi.org/10.1016/j.eap.2022.11.017 doi: 10.1016/j.eap.2022.11.017
![]() |
[44] |
Lee WS, Sohn SY (2017) Identifying emerging trends of financial business method patents. Sustainability 9: 1670. https://doi.org/10.3390/su9091670 doi: 10.3390/su9091670
![]() |
[45] |
Lekakos G, Vlachos P, Koritos C (2014) Green is good but is usability better? Consumer reactions to environmental initiatives in e-banking services. Ethics Inf Technol 16: 103–117. https://doi.org/10.1007/s10676-014-9337-6 doi: 10.1007/s10676-014-9337-6
![]() |
[46] | Mądra-Sawicka M (2020) Financial management in the big data era. In Management in the Era of Big Data, 71–81, Auerbach Publications. https://doi.org/10.1201/9781003057291-6 |
[47] |
Mejia-Escobar JC, González-Ruiz JD, Duque-Grisales E (2020) Sustainable financial products in the Latin America banking industry: Current status and insights. Sustainability 12: 5648. https://doi.org/10.3390/su12145648 doi: 10.3390/su12145648
![]() |
[48] | Mhlanga D (2023) FinTech for Sustainable Development in Emerging Markets with Case Studies. In FinTech and Artificial Intelligence for Sustainable Development: The Role of Smart Technologies in Achieving Development Goals, 337–363, Springer. https://doi.org/10.1007/978-3-031-37776-1_15 |
[49] |
Mohr I, Fuxman L, Mahmoud AB (2022) A triple-trickle theory for sustainable fashion adoption: the rise of a luxury trend. J Fash Mark Manag Int J 26: 640–660. https://doi.org/10.1108/JFMM-03-2021-0060 doi: 10.1108/JFMM-03-2021-0060
![]() |
[50] |
Muniz Jr AM, Schau HJ (2005) Religiosity in the abandoned Apple Newton brand community. J Consum Res 31: 737–747. https://doi.org/10.1086/426607 doi: 10.1086/426607
![]() |
[51] |
Naruetharadhol P, Ketkaew C, Hongkanchanapong N, et al. (2021) Factors affecting sustainable intention to use mobile banking services. Sage Open 11: 21582440211029925. https://doi.org/10.1177/21582440211029925 doi: 10.1177/21582440211029925
![]() |
[52] |
Nenavath S (2022) Impact of fintech and green finance on environmental quality protection in India: By applying the semi-parametric difference-in-differences (SDID). Renew Energ 193: 913–919. https://doi.org/10.1016/j.renene.2022.05.020 doi: 10.1016/j.renene.2022.05.020
![]() |
[53] |
Nosratabadi S, Pinter G, Mosavi A, et al. (2020) Sustainable banking; evaluation of the European business models. Sustainability 12: 2314. https://doi.org/10.3390/su12062314 doi: 10.3390/su12062314
![]() |
[54] |
Ortas E, Burritt RL, Moneva JM (2013) Socially Responsible Investment and cleaner production in the Asia Pacific: does it pay to be good? J Cleaner Prod 52: 272–280. https://doi.org/10.1016/j.jclepro.2013.02.024 doi: 10.1016/j.jclepro.2013.02.024
![]() |
[55] |
Oseni UA, Adewale AA, Omoola SO (2018) The feasibility of online dispute resolution in the Islamic banking industry in Malaysia: An empirical legal analysis. Int J Law Manag 60: 34–54. https://doi.org/10.1108/IJLMA-06-2016-0057 doi: 10.1108/IJLMA-06-2016-0057
![]() |
[56] |
Paiva BM, Ferreira FA, Carayannis EG, et al. (2021) Strategizing sustainability in the banking industry using fuzzy cognitive maps and system dynamics. Int J Sustain Dev World Ecol 28: 93–108. https://doi.org/10.1080/13504509.2020.1782284 doi: 10.1080/13504509.2020.1782284
![]() |
[57] |
Parmentola A, Petrillo A, Tutore I, et al. (2022) Is blockchain able to enhance environmental sustainability? A systematic review and research agenda from the perspective of Sustainable Development Goals (SDGs). Bus Strateg Environ 31: 194–217. https://doi.org/10.1002/bse.2882 doi: 10.1002/bse.2882
![]() |
[58] |
Paul J, Lim WM, O'Cass A, et al. (2021) Scientific procedures and rationales for systematic literature reviews (SPAR‐4‐SLR). Int J Consum Stud 45: O1–O16. https://doi.org/10.1111/ijcs.12695 doi: 10.1111/ijcs.12695
![]() |
[59] |
Puschmann T, Hoffmann CH, Khmarskyi V (2020) How green FinTech can alleviate the impact of climate change—the case of Switzerland. Sustainability 12: 10691. https://doi.org/10.3390/su122410691 doi: 10.3390/su122410691
![]() |
[60] |
Rahman S, Moral IH, Hassan M, et al. (2022) A systematic review of green finance in the banking industry: perspectives from a developing country. Green Financ 4: 347–363. https://doi.org/10.3934/GF.2022017 doi: 10.3934/GF.2022017
![]() |
[61] |
Ryu HS, Ko KS (2020) Sustainable development of Fintech: Focused on uncertainty and perceived quality issues. Sustainability 12: 7669. https://doi.org/10.3390/su12187669 doi: 10.3390/su12187669
![]() |
[62] |
Sagnier C, Loup-Escande E, Lourdeaux D, et al. (2020) User acceptance of virtual reality: an extended technology acceptance model. Int J Hum–Comput Interact 36: 993–1007. https://doi.org/10.1080/10447318.2019.1708612 doi: 10.1080/10447318.2019.1708612
![]() |
[63] |
Sethi P, Chakrabarti D, Bhattacharjee S (2020) Globalization, financial development and economic growth: Perils on the environmental sustainability of an emerging economy. J Policy Model 42: 520–535. https://doi.org/10.1016/j.jpolmod.2020.01.007 doi: 10.1016/j.jpolmod.2020.01.007
![]() |
[64] |
Singh RK, Mishra R, Gupta S, et al. (2023) Blockchain applications for secured and resilient supply chains: A systematic literature review and future research agenda. Comput Ind Eng 175: 108854. https://doi.org/10.1016/j.cie.2022.108854 doi: 10.1016/j.cie.2022.108854
![]() |
[65] |
Sun Y, Luo B, Wang S, et al. (2021) What you see is meaningful: Does green advertising change the intentions of consumers to purchase eco‐labeled products? Bus Strateg Environ 30: 694–704. https://doi.org/10.1002/bse.2648 doi: 10.1002/bse.2648
![]() |
[66] |
Talom FSG, Tengeh RK (2019) The impact of mobile money on the financial performance of the SMEs in Douala, Cameroon. Sustainability 12: 183. https://doi.org/10.3390/su12010183 doi: 10.3390/su12010183
![]() |
[67] |
Taneja S, Siraj A, Ali L, et al. (2023) Is fintech implementation a strategic step for sustainability in today's changing landscape? An empirical investigation. IEEE T Eng Manage. https://doi.org/10.3390/su12010183 doi: 10.3390/su12010183
![]() |
[68] |
Tara K, Singh S, Kumar R, et al. (2019) Geographical locations of banks as an influencer for green banking adoption. Prabandhan: Indian J Manag 12: 21–35. https://doi.org/10.17010/pijom/2019/v12i1/141425 doi: 10.17010/pijom/2019/v12i1/141425
![]() |
[69] |
Tchamyou VS, Erreygers G, Cassimon D (2019) Inequality, ICT and financial access in Africa. Technol Forecast Soc Change 139: 169–184. https://doi.org/10.1016/j.techfore.2018.11.004 doi: 10.1016/j.techfore.2018.11.004
![]() |
[70] |
Tripathi R (2023) Framework of green finance to attain sustainable development goals: an empirical evidence from the TCCM approach. Benchmarking. https://doi.org/10.1108/BIJ-05-2023-0311 doi: 10.1108/BIJ-05-2023-0311
![]() |
[71] |
Truby J, Brown R, Dahdal A (2020) Banking on AI: mandating a proactive approach to AI regulation in the financial sector. Law Financ Mark Rev 14: 110–120. https://doi.org/10.1080/17521440.2020.1760454 doi: 10.1080/17521440.2020.1760454
![]() |
[72] |
Tsindeliani IA, Proshunin MM, Sadovskaya TD, et al. (2022) Digital transformation of the banking system in the context of sustainable development. J Money Laund Contro 25: 165–180. https://doi.org/10.1108/JMLC-02-2021-0011 doi: 10.1108/JMLC-02-2021-0011
![]() |
[73] |
Ullah A, Pinglu C, Ullah S, et al. (2023) Impact of intellectual capital efficiency on financial stability in banks: Insights from an emerging economy. Int J Financ Econ 28: 1858–1871. https://doi.org/10.1002/ijfe.2512 doi: 10.1002/ijfe.2512
![]() |
[74] |
Yadav MS (2010) The decline of conceptual articles and implications for knowledge development. J Mark 74: 1–19. https://doi.org/10.1509/jmkg.74.1.1 doi: 10.1509/jmkg.74.1.1
![]() |
[75] |
Yan C, Siddik AB, Yong L, et al. (2022) A two-staged SEM-artificial neural network approach to analyze the impact of FinTech adoption on the sustainability performance of banking firms: The mediating effect of green finance and innovation. Systems 10: 148. https://doi.org/10.3390/systems10050148 doi: 10.3390/systems10050148
![]() |
[76] |
Yang C, Masron TA (2022) Impact of digital finance on energy efficiency in the context of green sustainable development. Sustainability 14: 11250. https://doi.org/10.3390/su14181125 doi: 10.3390/su14181125
![]() |
[77] |
Yigitcanlar T, Cugurullo F (2020) The sustainability of artificial intelligence: An urbanistic viewpoint from the lens of smart and sustainable cities. Sustainability 12: 8548. https://doi.org/10.3390/su1220854 doi: 10.3390/su1220854
![]() |
[78] |
Zhang Y (2023) Impact of green finance and environmental protection on green economic recovery in South Asian economies: mediating role of FinTech. Econ Chang Restruct 56: 2069–2086. https://doi.org/10.1007/s10644-023-09500-0 doi: 10.1007/s10644-023-09500-0
![]() |
[79] | Zhao D, Strotmann A (2015) Analysis and visualization of citation networks. Morgan & Claypool Publishers. |
[80] |
Zhao Q, Tsai PH, Wang JL (2019) Improving financial service innovation strategies for enhancing china's banking industry competitive advantage during the fintech revolution: A Hybrid MCDM model. Sustainability 11: 1419. https://doi.org/10.3390/su11051419 doi: 10.3390/su11051419
![]() |
[81] |
Zuo L, Strauss J, Zuo L (2021) The digitalization transformation of commercial banks and its impact on sustainable efficiency improvements through investment in science and technology. Sustainability 13: 11028. https://doi.org/10.3390/su131911028 doi: 10.3390/su131911028
![]() |
Min. | Average | Max. | Unit | |
Density | 1.15 | 1.69 | 2.13 | t/m3 |
Water content | 19 | 56 | 357 | % |
Liquid limit, wL | 18 | 52 | 309 | % |
Remoulded shear strength, cur,FC | 0.07 | 1.4 | 28 | kPa |
Index shear strength, cu,FC | 1.0 | 14.8 | 114 | kPa |
Sensitivity, St | 1.8 | 17 | 186 | - |
Min. | Average | Max. | Unit | |
Preconsolidation pressure, σ'c | 8 | 61 | 305 | kPa |
Modulus, Mi | 133 | 3,216 | 47,000 | kPa |
Modulus, ML | 65 | 652 | 5,000 | kPa |
Modulus number M' | 5.5 | 15.7 | 34 | - |
Limit pressure, σ'L | 19 | 64 | 550 | kPa |
Permeability, k | 8.5E-11 | 7.1E-10 | 2.3E-08 | m/s |
Change of k with strain, βk | 1.5 | 4.0 | 8.4 | - |
Coefficient of consolidation, cv,NC | 0.05 | 0.73 | 20.14 | m2/s |
Mi/ML | Frequency | |
Poor quality | < 2 | ~20% |
Fair quality | 2 – 4 | ~35% |
Good quality | 4 – 8 | ~32% |
Very good quality | > 8 | ~13% |
Min. | Average | Max. | Unit | |
Density | 1.15 | 1.69 | 2.13 | t/m3 |
Water content | 19 | 56 | 357 | % |
Liquid limit, wL | 18 | 52 | 309 | % |
Remoulded shear strength, cur,FC | 0.07 | 1.4 | 28 | kPa |
Index shear strength, cu,FC | 1.0 | 14.8 | 114 | kPa |
Sensitivity, St | 1.8 | 17 | 186 | - |
Min. | Average | Max. | Unit | |
Preconsolidation pressure, σ'c | 8 | 61 | 305 | kPa |
Modulus, Mi | 133 | 3,216 | 47,000 | kPa |
Modulus, ML | 65 | 652 | 5,000 | kPa |
Modulus number M' | 5.5 | 15.7 | 34 | - |
Limit pressure, σ'L | 19 | 64 | 550 | kPa |
Permeability, k | 8.5E-11 | 7.1E-10 | 2.3E-08 | m/s |
Change of k with strain, βk | 1.5 | 4.0 | 8.4 | - |
Coefficient of consolidation, cv,NC | 0.05 | 0.73 | 20.14 | m2/s |
Mi/ML | Frequency | |
Poor quality | < 2 | ~20% |
Fair quality | 2 – 4 | ~35% |
Good quality | 4 – 8 | ~32% |
Very good quality | > 8 | ~13% |