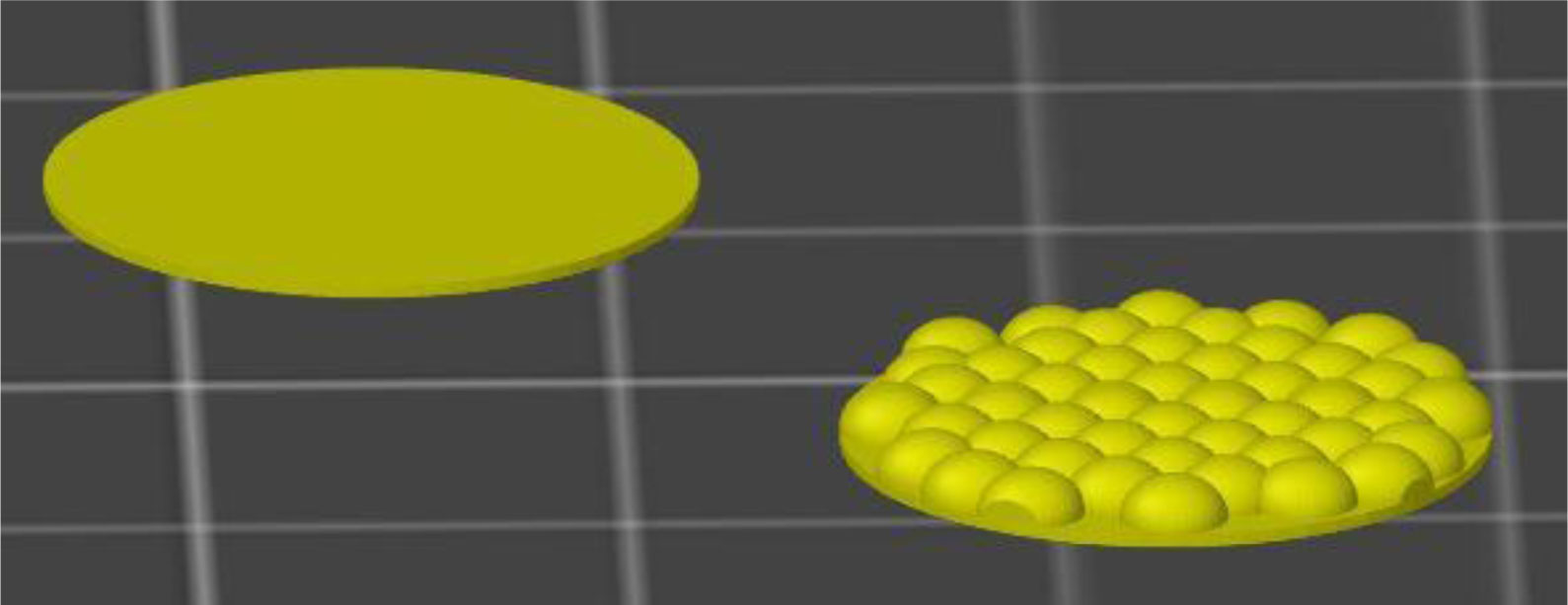
Citation: Sven Sölmann, Anke Rattenholl, Hannah Blattner, Guido Ehrmann, Frank Gudermann, Dirk Lütkemeyer, Andrea Ehrmann. Mammalian cell adhesion on different 3D printed polymers with varying sterilization methods and acidic treatment[J]. AIMS Bioengineering, 2021, 8(1): 25-35. doi: 10.3934/bioeng.2021004
[1] | Stephen W. Sawyer, Kairui Zhang, Jason A. Horton, Pranav Soman . Perfusion-based co-culture model system for bone tissue engineering. AIMS Bioengineering, 2020, 7(2): 91-105. doi: 10.3934/bioeng.2020009 |
[2] | Simran Rajesh Katyari, Prateeksha Lakhe, Amit Reche . Rapid prototyping: A future in orthodontic. AIMS Bioengineering, 2024, 11(1): 66-84. doi: 10.3934/bioeng.2024005 |
[3] | Tanishka Taori, Anjali Borle, Shefali Maheshwari, Amit Reche . An insight into the biomaterials used in craniofacial tissue engineering inclusive of regenerative dentistry. AIMS Bioengineering, 2023, 10(2): 153-174. doi: 10.3934/bioeng.2023011 |
[4] | Daria Wehlage, Hannah Blattner, Al Mamun, Ines Kutzli, Elise Diestelhorst, Anke Rattenholl, Frank Gudermann, Dirk Lütkemeyer, Andrea Ehrmann . Cell growth on electrospun nanofiber mats from polyacrylonitrile (PAN) blends. AIMS Bioengineering, 2020, 7(1): 43-54. doi: 10.3934/bioeng.2020004 |
[5] | Ana Letícia Braz, Ifty Ahmed . Manufacturing processes for polymeric micro and nanoparticles and their biomedical applications. AIMS Bioengineering, 2017, 4(1): 46-72. doi: 10.3934/bioeng.2017.1.46 |
[6] | E. Langford, C.A. Griffiths . The mechanical strength of additive manufactured intraosseous transcutaneous amputation prosthesis, known as the ITAP. AIMS Bioengineering, 2018, 5(3): 133-150. doi: 10.3934/bioeng.2018.3.133 |
[7] | Ana Y. Rioja, Maritza Muniz-Maisonet, Thomas J. Koob, Nathan D. Gallant . Effect of nordihydroguaiaretic acid cross-linking on fibrillar collagen: in vitro evaluation of fibroblast adhesion strength and migration. AIMS Bioengineering, 2017, 4(2): 300-317. doi: 10.3934/bioeng.2017.2.300 |
[8] | Poonam Sharma, Shalise Burch, Tejasvi Peesay, Susan M. Hamilla, Adam H. Hsieh, Carlos Luna Lopez . Downregulation of vimentin intermediate filaments affect human mesenchymal stem cell adhesion and formation of cellular projections. AIMS Bioengineering, 2020, 7(4): 272-288. doi: 10.3934/bioeng.2020023 |
[9] | Yihan Zhang . Manufacture of complex heart tissues: technological advancements and future directions. AIMS Bioengineering, 2021, 8(1): 73-92. doi: 10.3934/bioeng.2021008 |
[10] | Jingwen Zhang, Lu Wang, Huolin Chen, Tieying Yin, Yanqun Teng, Kang Zhang, Donghong Yu, Guixue Wang . Effect of Caspase Inhibitor Ac-DEVD-CHO on Apoptosis of Vascular Smooth Muscle Cells Induced by Artesunate. AIMS Bioengineering, 2014, 1(1): 13-24. doi: 10.3934/bioeng.2014.1.13 |
Chinese hamster ovary (CHO) cells are often used in biotechnological processing, e.g. for protein or antibody production [1]–[3], cytotoxicity measurements [4],[5], and other biotechnological experiments [6]. Although most cell lines used for biotechnological processes are grown in suspension, many processes involve adherent cells. For all these applications, not only based on CHO, but also on other mammalian cells including stem cells, it is useful to prepare high-density adherent cell cultures [7].
Many systems aiming at large-scale adherent cell cultivation are flat, such as multilayer flasks [8],[9]. In stirred bioreactors, cells can be grown on microcarriers [10]–[12]. It should be mentioned that such microcarriers do not always result in highest specific growth rate for CHO cells immobilized in this way [13]. This is why other techniques were made commercially available, such as packed-bed bioreactors [14],[15], hollow-fiber bioreactors [16],[17] or rolled scaffolds [7].
Recently, approaches using 3D printing, especially using the fused deposition modelling (FDM) process, are reported in the literature. The use of printed PLA and PET/PETG has been tested in tissue engineering applications [18]–[21]. However, the employment of 3D printed PLA or PETG as scaffolds for cell culture bioprocessing has not been reported yet. In this work, a modified PLA was used. This high temperature PLA (HT-PLA) [22] is a semi-crystalline material optimized for use at higher temperatures (printing temperature: 195–230 °C compared to 190–210 °C for conventional PLA). PETG is also highly interesting since pure PET, without the glycol modification supporting 3D printing, is a typical material for nonwoven macroporous carriers or membranes in bioreactors [23]–[25]. PETG displays a higher transparency and lower viscosity compared to PET. The third material tested in this study is HD-glass (heavy duty-glass), a more temperature-resistant, amorphous and food safe PETG blend with even more transparency than conventional PETG. These polymers were chosen because of their probable biocompatibility. In addition, we wanted to test the performance of HT-PLA and HD-glass during autoclaving.
It should be mentioned that PLA, while being biocompatible and regularly being used in medical implants, is not necessarily a good choice for cell adhesion. Similar to materials for medical stents which must combine biocompatibility with strongly impeded cell adhesion [26], previous studies on nanofiber revealed strongly reduced cell adhesion on biocompatible polyacrylonitrile (PAN) [27], as opposed to PAN with additional biopolymers [28],[29]. In addition, the surface roughness and waviness are influenced by 3D printing, sterilization processes and possible further after-treatment. Purely searching for biocompatible materials thus is insufficient to evaluate the potential cell adhesion on 3D printing polymers.
Generally, even small material modifications may result in large deviations of cell adhesion and growth. The sterilization process also significantly influences the suitability of a substrate for cell growth [29]. Thus, both these parameters are investigated here for three different commercially available FDM polymers which might be attractive candidates for the use as novel materials for microcarriers in eukaryotic cell culture bioprocesses. The CHO-DP12 cell line was used in this study as a proof of principle.
Substrates were prepared using a 3D printer I3 MK3 (Prusa Research A. S., Prague, Czech) with nozzle diameter 0.4 mm, applying a layer thickness of 0.15 mm. The filaments used are high temperature resistant PLA (HT-PLA, Proto-Pasta, ProtoPlant INC, Vancouver, WA, USA), PETG (Filamentworld, Neu-Ulm, Germany), and heavy duty glass (HD-glass, FormFutura, Nijmegen, Netherlands), (all filaments were purchased from Filamentworld). Nozzle and bed temperatures were 220 °C/60 °C (HT-PLA) and 240 °C/90 °C (PETG and HD-glass), respectively.
The printed samples with height 0.35 mm (even surface) to 1.25 mm (rippled surface) and diameter 15 mm were designed in Tinkercad (Autodesk Inc., San Rafael, CA, USA) and are depicted in Figure 1. Besides the samples with even surface, others were prepared with additional semi-circles on top, in this way introducing 2.5-dimensional structures and thus increasing the effective surface. This can be regarded as a first step to move from 2D to fully 3D structures for tissue engineering. Further surface structures will be tested in the future.
For all materials and surfaces, similar contact angles were found, as depicted in Table 1, indicating that differences between cell attachments are not mainly related to the hydrophilicity of the samples.
HT-PLA | HD-glass | PETG | |
Even surface | (74 ± 9)° | (83 ± 2)° | (79 ± 4)° |
Rippled surface | (72 ± 6)° | (83 ± 5)° | (85 ± 5)° |
Half of the samples were introduced in 1 M HCl for 1 h to increase positive surface charges and afterwards washed with ultrapure water until neutral pH. All samples were sterilized either by autoclaving in a VX-75 autoclave (Systec, Linden, Germany) at 121 °C for 15 min (after HCl treatment) or by inserting them in 1% sodium dodecyl sulfate (SDS) for 2 d (before washing briefly with PBS followed by HCl treatment).
The CHO DP-12 cells used in this study (LGC Standards GmbH, Wesel, Germany; ATCC no. CRL-12445) form a monolayer while growing in vitro if serum containing medium is used.
The culture medium was prepared dissolving 12 g Dulbecco's Modified Eagle's medium/Ham's Nutrient Mixture F12 (DMEM/F12, SAFC Biosciences, Irvine, UK) in 800 ml ultrapure water during stirring for 30 min and supplemented with 4 g/L glucose (Carl Roth, Karlsruhe, Germany) and 4 mM L-glutamine (Applichem, Darmstadt, Germany), followed by adding 2.44 g sodium bicarbonate (NaHCO3). The pH value was set to 7.2, and the medium was filled up to 1 L with ultrapure water. After sterile filtration of the medium (Sartolab P, 0.45 µm/0.22 µm, Sartorius, Göttingen, Germany), 100 ml sterile donor horse serum (biowest, Nuaillé, France) were added. The medium was stored at 4 °C.
Cells were grown on the 3D printed substrates placed in 6-well microtiter plates (Labsolute, Th. Geyer, Renningen, Germany) inside a safety cabinet Safe 2020 (Thermo Electron LED GmbH, Langenselbold, Germany).
5 ml medium with 1.9 × 105 cells were pipetted into each well. Cultivation was performed for 2 d (48 h) in a HERAcell 240i incubator (Thermo Electron LED GmbH, Langenselbold, Germany) at 37 °C and 5% CO2.
Images of the cells grown on the 3D printed substrates were taken with an inverted microscope Axiovert 40 CFL (Carl Zeiss, Göttingen, Germany) and a digital microscope VHX-600D (Keyence, Neu-Isenburg, Germany). Cell counting was performed using ImageJ (1.51j8 (National Institutes of Health, Bethesda, MD, USA). It should be mentioned that the aim of this study was to investigate whether cells adhered on the substrates, which is well visible with a relatively low resolution due to the shape of the cells [30]. A higher resolution, as given by a scanning electron microscope (SEM) or a confocal laser scanning microscope (CLSM), would reveal nicer images, but include the large problem of a bias due to the choice of the small areas of investigation [31], which is why similar investigations often show large-scale, low-magnification images [32]. Besides, since FDM printed samples are well-known to exhibit very low roughness in combination with a relatively high waviness [33], such images cannot reveal any further information about the sample surfaces.
After 2 d of cultivation, cell adhesion was determined. The medium was pipetted out of the wells, the wells were washed with PBS, and the residual cells were fixed on the substrates by adding 5 ml glyoxal solution [34]. The solution was prepared using 34 ml ultrapure water, 10 ml ethanol (abs., VWR BDH Prolabo, Langenfeld, Germany), 4 ml glyoxal (Roth, Karlsruhe Germany) and 0.38 ml acetic acid p.a. (VWR BDH Prolabo, Langenfeld, Germany) to control the pH value between 4 and 5. The so-prepared well-plates were stored for 30 min on ice followed by 30 min at room temperature, before the glyoxal solution was pipetted out of the wells. The wells were washed with phosphate buffered saline (PBS) buffer and afterwards twice with ultrapure water, before the samples were taken out of the wells and left for drying.
The samples were dyed with hematoxylin eosin (H&E), applying the H&E Fast Staining Kit (Roth, Karlsruhe, Germany), according to the protocol given by the manufacturer. The samples were firstly placed in H&E solution 1 (modified hematoxylin solution) for 6 min, washed with tap water and with 0.1% hydrochloric acid for 10 s each, and then blued for 6 min under floating tap water. The second part of the dyeing process was performed by placing them in H&E solution 2 (modified eosin yellow solution) for 30 s and washing them for 30 s with tap water again, before they were left for drying. All experiments were performed on three identical specimens.
Figure 2 shows the results of CHO cells cultivated on HT-PLA. The polymer was sterilized by autoclaving and SDS treatment, respectively. In addition, some specimens were treated with hydrochloric acid before addition of cells to provide additional positive charges on the polymer surface. Images were taken with different microscopes, comparing transmitted and reflected light, to find the optimum optical evaluation method.
Since a more temperature resistant PLA was used, autoclaving of the polymer was possible without deformation of the 3D printed structures. However, the material lost its transparency. Thus, microscopic images of the autoclaved polymer could be taken only with reflected light. Nevertheless, investigation with transmitted light was possible with SDS treated polymer samples. However, analysis with the inverted microscope displayed shadow structures due to the different filament layers, and focusing was difficult. It can be seen that CHO cells adhere only little to HT-PLA. Most of the cells display a round appearance, and only few cells show a flattened morphology (Figure 2, arrows), indicating a fully adhered cell. No differences in cell attachment regarding acid treatment can be seen. In addition, SDS treatment as a sterilization method did not harm the cells since the polymer samples were thoroughly washed with sterile water afterwards to remove all traces of the surfactant.
Autoclaving of HD-glass led to melting of the polymer. In addition, the material became opaque so that it could not be analyzed with the inverted microscope. Nevertheless, cell adhesion on these samples was also tested: cells attached well to this material as can be seen in Figure 3a,b. Again, it was possible to sterilize HD-glass by incubation with SDS solution, keeping the polymer's transparency. On this polymer, the CHO cells adhered well: they displayed a flattened morphology, as can be seen especially well in the higher magnification in Figure 3f. In addition, cells adhered on acid treated polymer as well as on untreated material.
Figure 4 shows cells on SDS sterilized PETG, investigated by the Axiovert 40 CFL in transmitted light. Usually, transmitted light is used for examinations of cells growing in T-flasks, petri dishes or on other completely even substrates. Nevertheless, it can be seen that adherent CHO cells attach and spread quite well on PETG. The spindle-like morphology of numerous cells indicates cell motility. Again, no difference between acid-treated and acid-untreated PETG on cell attachment can be seen. In addition, cells spread well on even and rippled surfaces.
For a quantitative comparison between the different substrates, Figure 5 depicts the cells attached on the different substrates. It must be mentioned that counting did not differentiate between stretched, rigidly adhered cells and round, only slightly attached cells.
Some combinations—e.g. even autoclaved HT-PLA with acid treatment or even SDS treated HT-PLA without acid—seem to be advantageous. The large error bars, however, show that all apparent differences are not significant and will thus not be discussed further.
Combining these findings, it can be assumed that HT-PLA, HD-glass and PETG are non-toxic 3D printing polymers that can be used for the cultivation of mammalian cells. All materials can be sterilized by SDS treatment without losing the shape of the printed specimen. However, it must be paid attention to the removal of any traces of the surfactant. Some of the polymer samples were treated with acid to provide additional charges on the polymer surface to facilitate cell adhesion. However, this treatment did not seem to improve cell attachment. Nevertheless, it could be used for sterilization of the polymers without further SDS treatment in the future: removal of the acid can be easily achieved by rinsing with sterile buffer. This will be tested in further experiments. Cell adhesion was stronger on PETG and HD-glass (a PETG derivative) as compared to HT-PLA. On the other hand, HT-PLA has got the advantage that it can be autoclaved without deformation. Unfortunately, the material becomes opaque after this treatment, indicating some changes of the polymer structure. Cells attach only weakly on HT-PLA which is a disadvantage regarding a possible application for microcarrier cultures, since cells can easily detach even at small shear stress. On the other hand, this might be an advantage when the cells themselves need to be harvested. Indeed, preliminary trypsinization experiments showed that cells cultured on HT-PLA detached quite easily in the presence of the enzyme (data not shown). Microcarrier cultures on 3D-printed HD-glass or PETG probably need longer incubation times for a complete detachment of the cells which might lead to cell damage. This is especially critical when cultivating stem cells or delicate primary cells.
For the first time, three commercially available, often used FDM filaments were used for 3D printing substrates for investigation of CHO cell adhesion: HT-PLA, PETG and HD-glass. Optical investigations revealed that the cells showed the most advanced adhesion on PETG and the PETG-derivative HD-glass, suggesting this material for further experiments. Nevertheless, HT-PLA might be useful for the cultivation of microcarrier cultures since weak attachment might be advantageous if cells need to be harvested. This will be tested in the near future.
[1] |
Tegel H, Dannemeyer M, Kanje S, et al. (2020) High throughput generation of a resource of the human secretome in mammalian cells. New Biotechnol 58: 45-54. doi: 10.1016/j.nbt.2020.05.002
![]() |
[2] |
Wells E, Song LQ, Greer M, et al. (2020) Media supplementation for targeted manipulation of monoclonal antibody galactosylation and fucosylation. doi: 10.1002/bit.27496
![]() |
[3] |
Sun T, Kwo WC, Chua KJ, et al. (2020) Development of a proline-based selection system for reliable genetic engineering in Chinese hamster ovary cells. ACS Synth Biol 9: 1864-1872. doi: 10.1021/acssynbio.0c00221
![]() |
[4] |
Wang YX, Puthussery JV, Yu HR, et al. (2020) Synergistic and antagonistic interactions among organic and metallic components of the ambient particulate matter (PM) for the cytotoxicity measured by Chinese hamster ovary cells. Sci Total Environ 736: 139511. doi: 10.1016/j.scitotenv.2020.139511
![]() |
[5] | Musini A, Pokala B, Zahoorulah SMD, et al. (2020) Cytotoxicity of Salacia oblonga extracts against cancer and normal cells and isolation of bioactive compounds. Res J Biotechnol 15: 105-109. |
[6] |
Louie S, Lakkyreddy J, Castellano BM, et al. (2020) Insulin degrading enzyme (IDE) expressed by Chinese hamster ovary (CHO) cells is responsible for degradation of insulin in culture media. J Biotechnol 320: 44-49. doi: 10.1016/j.jbiotec.2020.04.016
![]() |
[7] |
YekrangSafakar A, Hamel KM, Mehrnezhad A, et al. (2020) Development of rolled scaffold for high-density adherent cell culture. Biomed Microdevices 22: 4. doi: 10.1007/s10544-019-0459-9
![]() |
[8] |
Chen A, Ting S, Seow J, et al. (2014) Considerations in designing systems for large scale production of human cardiomyocytes from pluripotent stem cells. Stem Cell Res Ther 5: 12. doi: 10.1186/scrt401
![]() |
[9] |
Jenkins MJ, Farid SS (2015) Human pluripotent stem cell-derived products: Advances towards robust, scalable and cost-effective manufacturing strategies. Biotechnol J 10: 83-95. doi: 10.1002/biot.201400348
![]() |
[10] |
YekrangSafakar A, Acun A, Choi J-W, et al. (2018) Hollow microcarriers for large-scale expansion of anchorage-dependent cells in a stirred bioreactor. Biotechnol Bioeng 115: 1717-1728. doi: 10.1002/bit.26601
![]() |
[11] |
Sousa MFQ, Silva MM, Roldao A, et al. (2015) Production of oncolytic adenovirus and human mesenchymal stem cells in a single-use, Vertical-Wheel bioreactor system: Impact of bioreactor design on performance of microcarrier-based cell culture processes. Biotechnol Prog 31: 1600-1612. doi: 10.1002/btpr.2158
![]() |
[12] |
Tharmalingam T, Sunley K, Spearman M, et al. (2011) Enhanced production of human recombinant proteins from CHO cells grown to high densities in macroporous microcarriers. Mol Biotechnol 49: 263-276. doi: 10.1007/s12033-011-9401-y
![]() |
[13] |
Hilal-Alnaqbi A, Hu AYC, Zhang ZB, et al. (2013) Growth, metabolic activity, and productivity of immobilized and freely suspended CHO cells in perfusion culture. Biotechnol Appl Biochem 60: 436-445. doi: 10.1002/bab.1103
![]() |
[14] |
Meuwly F, Papp F, Ruffieux PA, et al. (2006) Use of glucose consumption rate (GCR) as a tool to monitor and control animal cell production processes in packed-bed bioreactors. J Biotechnol 122: 122-129. doi: 10.1016/j.jbiotec.2005.08.005
![]() |
[15] |
Meuwly F, Loviat F, Ruffieux PA, et al. (2006) Oxygen supply for CHO cells immobilized on a packed-bed of Fibra-Cel(R) disks. Biotechnol Bioeng 93: 791-800. doi: 10.1002/bit.20766
![]() |
[16] |
Nikolay A, de Grooth J, Genzel Y, et al. (2020) Virus harvesting in perfusion culture: Choosing the right type of hollow fiber membrane. doi: 10.1002/bit.27470
![]() |
[17] |
Menshutina NV, Guseva EV, Safarov RR, et al. (2019) Modelling of hollow fiber membrane bioreactor for mammalian cell cultivation using computational hydrodynamics. Bioprocess Biosyst Eng 43: 549-567. doi: 10.1007/s00449-019-02249-9
![]() |
[18] |
Rosenzweig DH, Carelli E, Steffen T, et al. (2015) 3D-printed ABS and PLA scaffolds for cartilage and nucleus pulposus tissue regeneration. Int J Mol Sci 16: 15118-15135. doi: 10.3390/ijms160715118
![]() |
[19] |
Zhang P, Han F, Li Y, et al. (2016) Local delivery of controlled-release simvastatin to improve the biocompatibility of polyethylene terephthalate artificial ligaments for reconstruction of the anterior cruciate ligament. Int J Nanomed 11: 465-478. doi: 10.2147/IJN.S95032
![]() |
[20] |
Pérez P, Serrano JA, Olmo A (2020) 3D-printed sensors and actuators in cell culture and tissue engineering: framework and research challenges. Sensors 20: 5617. doi: 10.3390/s20195617
![]() |
[21] |
Rimington RP, Capel AJ, Christie SDR, et al. (2017) Biocompatible 3D printed polymers via fused deposition modelling direct C2C12 cellular phenotype in vitro. Lab Chip 17: 2982-2993. doi: 10.1039/C7LC00577F
![]() |
[22] | Proto-Pasta – HT-PLA – PLA Filament – Translucent. Available from: https://www.filamentworld.de/shop/special-filament/hochtemperatur-filament/iridescent-ice-filament-1-75mm-hochtemperatur-pla/. |
[23] |
Lennaertz A, Knowles S, Drugmand J-C, et al. (2013) Viral vector production in the integrity® iCELLis® single-use fixed-bed bioreactor, from bench-scale to industrial scale. BMC Proc 7: P59. doi: 10.1186/1753-6561-7-S6-P59
![]() |
[24] | Dohogne Y, Collignon F, Drugmand J-C, et al. (2019) scale-X™ bioreactor for viral vector production: Proof of concept for scalable HEK293 cell growth and adenovirus production. Univercell Application note. Available from: https://www.univercells.com/wp-content/uploads/2019/03/1118-Univercells-Note-4pages-WEB-vF.pdf. |
[25] |
Drugmand J-C, Michiels J-F, Aghatos SN, et al. (2007) Growth of mammalian and lepidopteram cells on BioNOC® II disks, a novel macroporous microcarrier. Cell Technology for Cell Products Heidelberg: Springer, 781-784. doi: 10.1007/978-1-4020-5476-1_143
![]() |
[26] |
Chen J, Dai S, Liu LY, et al. (2021) Photo-functionalized TiO2 nanotubes decorated with multifunctional Ag nanoparticles for enhanced vascular biocompatibility. Bioact Mater 6: 45-54. doi: 10.1016/j.bioactmat.2020.07.009
![]() |
[27] |
Narayanasamy S, Jayaprakash J (2020) Application of carbon-polymer based composite electrodes for microbial fuel cells. Rev Environ Sci Bio-Technol 19: 595-620. doi: 10.1007/s11157-020-09545-x
![]() |
[28] |
Wehlage D, Blattner H, Mamun A, et al. (2020) Cell growth on electrospun nanofiber mats from polyacrylonitrile (PAN) blends. AIMS Bioengineering 7: 43-54. doi: 10.3934/bioeng.2020004
![]() |
[29] |
Wehlage D, Blatter H, Sabantina L, et al. (2019) Sterilization of PAN/gelatin nanofibrous mats for cell growth. Tekstilec 62: 78-88. doi: 10.14502/Tekstilec2019.62.78-88
![]() |
[30] |
Jiang PL, Liang JH, Lin CJ (2013) Construction of micro-nano network structure on titanium surface for improving bioactivity. Appl Surf Sci 280: 373-380. doi: 10.1016/j.apsusc.2013.04.164
![]() |
[31] |
Wortmann M, Layland AS, Frese N, et al. (2020) On the reliability of highly magnified micrographs for structural analysis in materials science. Sci Rep 10: 14708. doi: 10.1038/s41598-020-71682-8
![]() |
[32] |
Chen XY, Cai KY, Lai M, et al. (2011) Mesenchymal stem cells differentiation on hierarchically micro/nano-structured titanium substrates. Adv Eng Mater 14: B216-B223. doi: 10.1002/adem.201180073
![]() |
[33] | Kozior T, Mamun A, Trabelsi M, et al. (2020) Quality of the surface texture and mechanical properties of FDM printed samples after thermal and chemical treatment. Strojniški vestnik J Mech Eng 66: 105-113. |
[34] |
Richter KN, Revelo NH, Seitz KJ, et al. (2018) Glyoxal as an alternative fixative to formaldehyde in immunostaining and super-resolution microscopy. EMBO J 37: 139-159. doi: 10.15252/embj.201695709
![]() |
HT-PLA | HD-glass | PETG | |
Even surface | (74 ± 9)° | (83 ± 2)° | (79 ± 4)° |
Rippled surface | (72 ± 6)° | (83 ± 5)° | (85 ± 5)° |