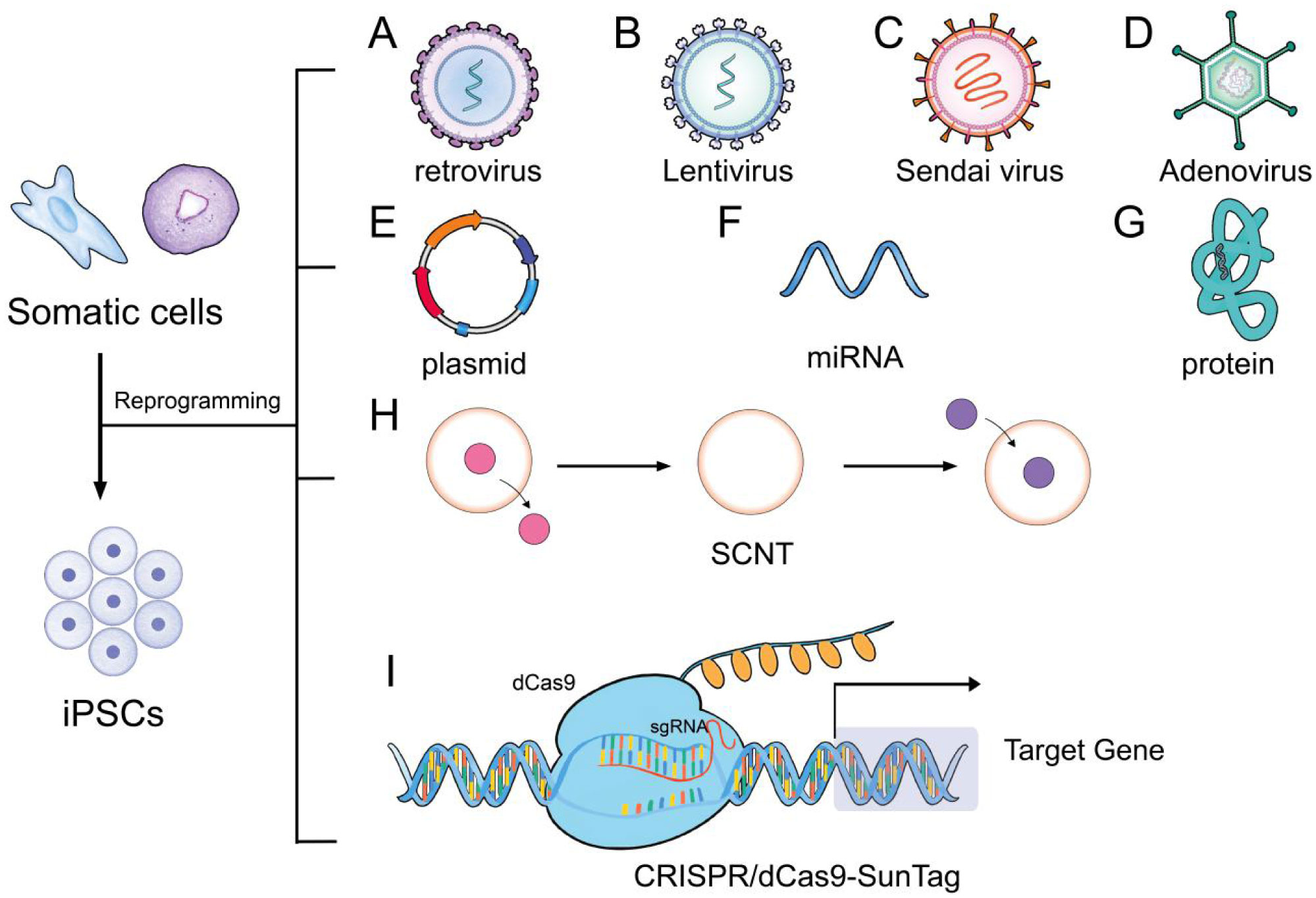
Citation: Yihan Zhang. Manufacture of complex heart tissues: technological advancements and future directions[J]. AIMS Bioengineering, 2021, 8(1): 73-92. doi: 10.3934/bioeng.2021008
[1] | Tanishka Taori, Anjali Borle, Shefali Maheshwari, Amit Reche . An insight into the biomaterials used in craniofacial tissue engineering inclusive of regenerative dentistry. AIMS Bioengineering, 2023, 10(2): 153-174. doi: 10.3934/bioeng.2023011 |
[2] | Matthew Hogan, Glauco Souza, Ravi Birla . Assembly of a functional 3D primary cardiac construct using magnetic levitation. AIMS Bioengineering, 2016, 3(3): 277-288. doi: 10.3934/bioeng.2016.3.277 |
[3] | Joseph M. Chambers, Robert A. McKee, Bridgette E. Drummond, Rebecca A. Wingert . Evolving technology: creating kidney organoids from stem cells. AIMS Bioengineering, 2016, 3(3): 305-318. doi: 10.3934/bioeng.2016.3.305 |
[4] | Izgen Karakaya, Nuran Ulusoy . Basics of dentin-pulp tissue engineering. AIMS Bioengineering, 2018, 5(3): 162-178. doi: 10.3934/bioeng.2018.3.162 |
[5] | Sven Sölmann, Anke Rattenholl, Hannah Blattner, Guido Ehrmann, Frank Gudermann, Dirk Lütkemeyer, Andrea Ehrmann . Mammalian cell adhesion on different 3D printed polymers with varying sterilization methods and acidic treatment. AIMS Bioengineering, 2021, 8(1): 25-35. doi: 10.3934/bioeng.2021004 |
[6] | Stephen W. Sawyer, Kairui Zhang, Jason A. Horton, Pranav Soman . Perfusion-based co-culture model system for bone tissue engineering. AIMS Bioengineering, 2020, 7(2): 91-105. doi: 10.3934/bioeng.2020009 |
[7] | Vincent DEPLAIGNE, Gael Y. ROCHEFORT . Bone tissue engineering at a glance. AIMS Bioengineering, 2022, 9(1): 22-25. doi: 10.3934/bioeng.2022002 |
[8] | Jennifer L. Wood, Wuxing Liu, Caixian Tang, Ashley E. Franks . Microorganisms in heavy metal bioremediation: strategies for applying microbial-community engineering to remediate soils. AIMS Bioengineering, 2016, 3(2): 211-229. doi: 10.3934/bioeng.2016.2.211 |
[9] | Ana Letícia Braz, Ifty Ahmed . Manufacturing processes for polymeric micro and nanoparticles and their biomedical applications. AIMS Bioengineering, 2017, 4(1): 46-72. doi: 10.3934/bioeng.2017.1.46 |
[10] | Pornchai Kittivarakarn, Matthew Penna, Zenith Acosta, Daniel Pelaez, Ramon Montero, Fotios M. Andreopoulos, Herman S. Cheung . Cardiomyotic induction and proliferation of dental stem cells on electrospun scaffolds. AIMS Bioengineering, 2016, 3(2): 139-155. doi: 10.3934/bioeng.2016.2.139 |
Cardiovascular diseases (CVD) are one of the major diseases that threaten human life. According to recent statistics, nearly 17.9 million people die from CVD each year worldwide [1].
Although heart organ transplantation can significantly reduce the mortality of patients, due to insufficient supply of organs matching the patient, patients often need to experience a long and painful wait before surgery, and are also subject to postoperative problems such as immune rejection and surgical complications that require long-term or lifetime immunosuppressive treatment [2].
Based on the culture of functional iPSCs, research on manufacturing functional heart tissue through 3D bioprinting is bringing a potential revolution in the field of heart transplantation [3]. The acquisition of specific iPSCs is achieved by reprogramming the patient's somatic cells. These iPSCs have unlimited proliferation capacity and can differentiate into any type of cells [4]. Engineered bioink mixed with differentiated cells and biocompatible materials can be used to construct complex engineered tissues. Since the production of bioink is based on the patient's own cells, this technology can potentially solve the problems of donor shortage and immune rejection. In this review, we explore different strategies for reprogramming and cell differentiation, including the use of the recent CRISPRa strategy.
The manufacture of complex heart tissue relies on advances in printing equipment, materials, computer simulations, and cytology. This review compares the advantages and disadvantages of different printing strategies and materials. Manufacturing microvascularized heart tissue has been a difficult task in heart tissue engineering. Here we present the current direct and indirect vascularization strategies and discuss potential strategies to produce microvascularized heart tissue in the future.
Induced pluripotent stem cells (iPSC) are reprogrammed cells that have similar functions to embryonic stem cells, such as the ability to self-renew and differentiate into multiple types of cells [4]. Differentiating during embryonic development, cells show differences in function and phenotype. The cause of these differences is the expression of specific genes. With the establishment of this specific expression, the cell state will be stabilized through cooperative transcription and epigenetic mechanisms, and this specificity will be passed on during cell division [5].
In the 2006 groundbreaking study of Yamanaka et al. [6], researchers use somatic cells to overexpress 4 transcription factors to obtain iPSCs. To honor the author's work, these four transcription factors are named Yamanaka factors: Oct3/4, Sox2, Klf4 and c-Myc.
Oct3/4 is a homeodomain transcription factor, helping pluripotent stem cells to maintain and differentiate [6]. Sox2 plays a vital role in controlling the expression of Oct3/4. C-Myc is a proto-oncogene related to the causes of various cancers. It recruits chromatin-modifying proteins, leading to extensive transcriptional activation. c-Myc can be replaced by L-Myc, which has lower transformation activity. Klf4 is an oncoprotein or tumor suppressor, a downstream target of leukemia inhibitory factor, and can activate Sox2. These four transcription factors work together to convert somatic cells into iPSCs [7].
This review mainly focuses on the following reprogramming methods: virus reprogramming, non-virus reprogramming, somatic cell nuclear transfer (SCNT), and CRISPRa-based reprogramming.
Retrovirus (Figure 1A) and lentivirus (Figure 1B) are integrative viruses. Using integrated virus, the gene delivery system is relatively efficient, but the introduction of new genes brings potential toxicity and tumorigenicity [8]. Somers et al. [9] have developed a single resectable lentiviral stem cell cassette. This technology uses Cre/loxP to remove the introduced reprogramming gene. However, this technology also has certain drawbacks. After excision, there are still about 200 bp of inactive viral LTR remaining in the host genome.
Sendai virus and adenovirus are non-integrative viruses. Sendai virus (Figure 1C) is an RNA virus that does not enter the nucleus. It can produce a large amount of protein and is suitable for almost all cell types. However, it takes about 10 generations of Sendai virus infection to produce toxicity-free iPSC [10]. Adenovirus (Figure 1D) is non-enveloped and has an icosahedral nucleocapsid containing a double-stranded DNA genome [11]. Therefore, it can infect dividing and non-dividing cells, and has a wide range of cell tropism. However, its reprogramming efficiency is only 0.001–0.0001% in mice, and only 0.0002% in human cells [12].
Plasmid vectors (Figure 1E) are one of the most basic methods of foreign gene expression, because compared with linear DNA, plasmid transfection is less susceptible to degradation by exonucleases [13]. Plasmid transfection steps are simple, but due to the transient expression of transcription factors, the recombination efficiency is significantly lower than that of integrated viral vectors. At the same time, plasmid transfection requires multiple rounds of transfection, resulting in stress to cells.
The use of microRNAs (miRNAs) (Figure 1F) is another non-viral reprogramming method. Subramanyam et al. [14] showed that miR-302s and miR-372 promote reprogramming of somatic cells by acting on multiple downstream pathways. Bioactive proteins (Figure 1G) that can cross the plasma membrane have also been used to generate footprint-free iPSCs with great potential. However, low efficiency and technical difficulty make such technologies difficult to put into clinical use [15]–[18].
PiggyBac transposon is a mobile genetic element that efficiently transposes between vectors and chromosomes through a ‘cut-and-paste’ mechanism [19]. Through transposase, it can be integrated into the TTAA site of the chromosome, and when the transposase is re-expressed, it can be excised from the genome without a trace, generating safe and clean iPSC without genetic modification.
SCNT (Figure 1H), also called Oocyte reprogramming, has relatively high efficiency in several species including mouse [20]. Although this technology has many advantages, it is still a target for its ethical concerns. At the same time, during SCNT, the cell nucleus undergoes epigenetic structural modifications, which usually manifests as a higher degree of DNA methylation and abnormal modification of histones [21]. To control the epigenetic modification in the SCNT process is difficult, since the best epigenetic regulation depends on the interaction between DNA methylation and histone modification. Therefore, different kinds of chromatin remodeling agents, such as histone deacetylase inhibitor (HDACi), are often used to improve SCNT [22].
Since the efficiency of homologous recombination in iPSCs is low, CRISPR-based targeted genome editing, which was first identified as a bacterial immune system against invading pathogens, may promote the reprogramming process by inserting targeted genes that activate reprogramming factors. Moreover, dCas9-SunTag (Figure 1I) is a type of CRISPR activation (CRISPRa) system, that can target the silenced chromatin locus with high precision and promote downstream gene transcription [23]. Research done by Weltner et al. [24] demonstrated that CRISPR activation of endogenous Oct4 successfully reprogrammed neuroepithelial stem cells (NSCs) to iPSCs. Liu P et al. [25] reported that dCas9-SunTag-VP64 activation of endogenous Oct4 or Sox2 can trigger mouse embryonic fibroblasts to iPSCs. Besides, CRISPR can also be used to correct the error genes from reprogramming or the genes causing heart disease [26].
Cardiomyocytes (CMs) are the main cell source to generate engineered cardiac tissues. During embryonic development, pluripotent epiblasts cells undergo a series of sequential steps to finally differentiate into CMs and other cardiac cells. Under the regulation of multiple growth factors and signal pathways, pluripotent stem cells (PSCs) experience three transitional cell states: mesoderm, cardiogenic mesoderm, and cardiac progenitors [27].
Therefore, in theory, in vitro environment, precise simulation of in vivo events, such as the sequence of growth factors and the regulation of metabolism by small molecules, can lead to the efficient production of healthy CMs [28]. In order to generate cardiac mesoderm-like cells, which express markers such as T Brachyury and Emoes, and to activate WNT/β-catenin pathway, growth factors, including bone morphogenic protein 2/4 (BMP2/4), Activin A, Nodal, basic fibroblast growth factor (bFGF) and WNT3A, should be added in a specific sequence [29]. Early cardiac specification is identified by expression of mesoderm posterior 1 (MESP1). Dickkopf-1 (Dkk-1) is then required to inhibit Endogenously WNT/β-catenin pathway, helping improve the differentiation efficiency [30]. WNT/β-catenin pathway promotes the transition of PSCs to primitive-streak-like cells in the early process, but inhibits the transition of PSs to stable mesoderm.
The use of small molecules (SM) to regulate specific signaling pathways provides a solution for the stable production of CMs in large quantities [28]. For example, CHIR99021, targeting GSK3α and GSK3β, serves as WNT/β-catenin pathway activator, DMH-1 and SB431542 as BMP inhibitor, controlling TGF-β signaling. Compared with clinical grade biological agents, the use of small molecules is more accurate and cost-effective because the production of clinical-grade biological agents such as recombinant growth factors, usually requires complex biological processes, and may have improper biological activity due to differences between batches [31]. As such, purity of product and biological activity need to be thoroughly assessed, thereby increasing the overall production cost.
There are currently 3 main strategies for inducing PSCs into CMs: embryoid body (EB) formation, co-culture, and adherent monolayer culture [32].
EBs are multi-cell agammaegates formed spontaneously from stem cells under suspension culture conditions [32]. This multicellular three-dimensional structure greatly improves the contact and communication between cells, and at the same time promotes the exchange of nutrients. Furthermore, EBs can be grown in 96 or 384-well plates, which greatly improves production efficiency [33]. Brickman et. al studied in detail the in vitro formation of EBs [34]. They observed that at the very beginning of the formation of agammaegates of cells, internal cells are affected by Fgf signaling, and primitive endoderm (PrE) is formed around the agammaegates on Day 2. A Frs2α- and Grb2-dependent Fgf-Mek-Erk signaling is necessary in PrE differentiation. Deficiency of Grb2 will lead to extracellular regulated protein kinases (Erk) cascade instead of PrE, and cavity structure will fail to form. The Wnt response gradually expands to encompass the entire EB on Day 2 and concomitant with this Nodal-response can be observed on Day 3. At late stage, cavitation forms a yolk sac-like structure on Day 7. Interestingly, In the culture environment with low serum content or rich in Activin, endoderm is more likely to form. As such, to promote the formation of mesoderm, a medium rich in fetal bovine serum is often used. At the same time, addition of ascorbic acid, Vitamin C, is recommended to improve the efficiency of differentiation [35],[36]. In the process of culturing cardiomyocytes, a medium rich in type IV collagenase and trypsin-EDTA is normally used to promote differentiation [37]. Although the more three-dimensional culture conditions brought about by this technology cannot be surpassed by other technologies, the cavity structure formed is not conducive to the direct use of bioprinting. Separation methods such as FACS or immunological methods must be implemented to obtain pure CMs. Tohyama et al. [38] noticed strong biochemical differences in glucose and lactate metabolism between cardiomyocytes and non-cardiomyocytes, and used glucose starvation to obtain purified cardiomyocytes.
Co-culture is a means of co-cultivating iPSCs with other cells to promote differentiation. Mummery et al. [39] first used visceral endoderm-like cell line (END-2) derived from mouse P19 embryonal carcinoma cells co-cultured with hESCs. This technology can only produce beating cells containing 2–3% CMs at the earliest, but with the addition of serum, insulin and a p38 protein kinase inhibitor, the yield of CMs can increase up to 20% [40]. END-2 could consume the insulin in the medium and promote the secretion of prostaglandin 2 (PGI2). Compared with EBs, co-culture is more cost-effective, but significantly increases the labor and time consumed.
In adherent monolayer culture, iPSCs are not only similar in morphology to monolayer epithelial cells, but they also share identical biological behavior [29]. Similar to epithelial cells, the death of iPSCs can be avoided when specific signaling pathways are manipulated. For example, inhibition of Rho-associated kinase (ROCK) pathway by the small molecule Y-27632, markedly diminishes dissociation-induced apoptosis in iPSCs and enables the culture of individualized iPSCs in adherent or suspension culture systems [30]. Compared with the other two strategies, monolayer culture is easier to control, since there are no complex diffusion barriers in a flat culture environment.
iPSC-derived CMs still face some limitations. Firstly, the number of CMs in the heart is not static, and the cellular composition may change in patients with heart disease. At the same time, CMs account for 25%–30% of the total number of heart cells, which means that other cells need to be produced to complete the diversity of cells when using bioprinting technology. Second, there will be a small number of iPSCs that carry partial or complete mutations, which means there will still be genomic instability [29]. At the same time, genetic heterogeneity cannot be completely avoided. iPSC-derived CMs still show flaws in the similarity to adult CMs [30]. For example, adult CMs usually have a rod-like structure, while iPSC-derived CMs usually appear as irregular polygons and are smaller in size. Adult CMs showed a more organized sarcomere structure, while iPSC-derived CMs did not. iPSC-derived CMs often exhibit a mononuclear structure, while some adult CMs have a binuclear structure.
The structure and function of newly differentiated CMs are closer to the cardiomyocytes of human infants than those of adults. The following methods are typically used to promote maturity: (1) stimulate with electrical or mechanical impulse; (2) co-culture with human-derived-CMs; (3) Physical, chemical, electrical, biological factors [32]. The following properties are normally used to assess maturity: (1) expression patterns of mRNAs, genes, proteins; (2) Ca2+ transients. (3) electrophysiology; (4) metabolic ability; (5) structure maturation such as T-tubule formation.
Interestingly, studies have shown that by adding a combination of transcription factors [41], such as the three cardiac development transcription factors Gata4, Mef2c and Tbx5 [42] or the combination of the four miRNAs miR-1, miR-133, miR-208 and miR-499 [43] FBs can be directly reprogrammed into CMs. Although this technology named trans-differentiation still faces many challenges, it offers exciting possibilities [44]–[47].
3D bioprinting is a recent method used in tissue engineering to produce tissue or organ substitutes. 3D bioprinting uses computer-aided printers to accurately deposit cells in viscous biological materials in a specific spatial arrangement. The usual steps of 3D bioprinting are 2D imaging scanning, computer assisted design (CAD) 3D modeling, generation of printable files layer by layer, calculation of the nozzle path at each layer, injection of biological ink and cells into the printer, and printing [48].
Depending on the physiological and structural requirements for different tissues, different bioprinting strategies are used. The existing bioprinting strategies mainly include inkjet-based bioprinting, laser-assisted bioprinting (LAB), extrusion bioprinting, stereolithography (SLA) bioprinting, digital light processing (DLP) bioprinting and magnetic bioprinting.
Inkjet bioprinting (Figure 2A) is a printing method used to deliver discrete droplets to a predetermined location. Inkjet bioprinting is mainly divided into thermal inkjet and acoustic inkjet according to different drivers. Thermal inkjet printer function by electrically heating the print head to produce pulses of pressure that force droplets from the nozzle [49]. The high temperature generally reaches 200–300 °C, but due to the short heating time, the overall bioink will only rise by 4–10 °C, suggesting that this warming has minimal effect on cell structure and function [50]. When printing with thermal inkjet, what still needs to be noted is the survival rate of cells exposed to heat and mechanical pressure, poor droplet positioning, nonuniform droplet size, and clog of nozzle. Another method is acoustic inkjet, in which piezoelectric crystal creates an acoustic wave inside the print head to break the liquid into droplets at regular intervals [51]. Using voltage may cause changes in the shape of the cells. At the same time, studies have shown that potential damage of the cell membrane and lysis may exist under 15–25kHZ used by piezoelectric inkjet bioprinters [49]. When using the inkjet method, the printing material must be liquid to produce droplets. The main advantages of this type of method are low cost, high resolution, high speed, and biocompatibility.
Extrusion bioprinting (Figure 2B) is the most widely used bioprinting technology. In this method, the printer that extrudes bioink through one or more nozzles. The nozzle can be driven by pneumatic, piston and screw forces [48]. Zhang YS et al. [52] assembled a microfluidic nozzle that allows two types of bioink. Extrusion bioprinting is suitable for a wide range of material viscosities. The viscosity of the material ranges from 30 to > 6 × 107 mPa/s [53]. However, the material source is not limited to liquids — other sources such as multicellular spheres can be used [54]. The printing material made with this kind of multicellular spheres allows very high cell density. However, the cell viability obtained by extrusion bioprinting is less than inkjet printing, and this decrease in viability may be caused by shear force applied during extrusion. Moreover, when viscosity of bioink is high, it will result in difficulty to squeeze out of the nozzle.
Laser-assisted bioprinting (LAB) (Figure 2C) is composed of a pulsed laser beam, focusing system, and the ribbon. LAB functions using laser pulses on the absorbing layer of the ribbon to generate a high-pressure bubble that propels cell-containing materials toward the collector substrate [55]. The main advantage of this technology is to print different living cells and biological materials with precision and micron-level resolution, while bringing a nozzle-free, no clogging system that enables a various range of viscosity and has negligible effects on cell viability. The resolution of LAB is limited by laser fluence, the surface tension, wettability of the substrate thickness and viscosity of the biological layer [56]. However, the individual ribbon usually needs to be replaced for different cell and material types, which may bring additional time and work when it comes to printing operations with multiple materials or complex structures [57]. Some of these challenges can be overcome by using cell recognition scanning technology to enable the laser beam to select a single cell in each pulse. Another issue to be addressed is that metallic residues have been found in the final printed structure, due to vaporization of the metallic laser-absorbing layer during printing [58].
Stereolithography (SLA), first introduced in 1980, is a typical light-assisted direct printing method [59]. In this process, ultraviolet light or laser is guided to the path of the photopolymerizable liquid polymer in a desired pattern. Exposure to the laser beam will photo-cure the pattern on the path drawn on the liquid polymer, and then connect it to the lower layer, allowing printing layer by layer to form a 3D structure [60]. This bioprinting system integrates a light source, a liquid photopolymer reservoir and a three-axis motion platform. Depending on the choice of laser source, SLA can have single-photon or two-photon absorption. Two-photon SLA has a higher printing resolution than single-photon, because the two-photon absorption photopolymerization occurs in a more precise area [61]. As an automation technology, SLA provides control over the manufacturing of structures ranging in size from a few hundred microns to a few millimeters [62].
Digital light processing (DLP) bioprinting technology (Figure 3C) comes from the image projection technology developed by Texas Instruments in the 1980s [63]. DLP utilizes projection light to polymerize materials to obtain pre-designed patterns. The precision projection light, reflected by a digital micromirror device of million pixels, ensures this technology both high resolution and printing speed [64]. At the same time, compared with extrusion printing, DLP has no nozzle-induced high temperature, high pressure and shear stress that could potentially harm cell viability, providing a relatively mild environment. Recently, Kelly et al. [65] invented a method called computed axial lithography (CAL) (Figure 2D), in which a transparent container filled with materials rotates to let patterned illumination from multiple directions deliver the calculated 3D exposure dose to the photosensitive material. 3D geometric shapes can be formed in the material in less than 1 minute after washing away the uncured material.
Magnetic bioprinting, a more recent type of non-contact bioprinting technology, uses magnetic materials to encapsulate cells, enabling scaffold-free cell magnetic levitation culture under a controlled magnetic field [66]. This technology brings new opportunities for complex cell assembly and 3D cell culture [67]. Tseng H et al. [68] used magnetic 3D bioprinting to study the contraction of circular vascular smooth muscle tissue, thereby assessing vascular activity in vitro, proving that this technology supports high-throughput screening. Although this technology can provide precise spatial control, the manufacturable spatial geometry is limited based on existing technology [69], usually a sphere or a ring.
As a natural biological material, hydrogel is widely used in bioprinting technology. The hydrogel is designed to mimic the composition of the extracellular matrix (ECM) and provide structural support for cells to promote cell proliferation, migration, and adhesion. At the same time, it has good degradation capabilities. The ideal hydrogel should maintain the same rate of degradation as the rate at which cells produce extracellular matrix. In order to make the properties of the hydrogel meet specific physical, chemical or biochemical requirements, several natural or synthetic polymers are usually mixed as additives for hydrogels [70]. The accuracy of 3D bioprinting of cell-filled polymer materials largely depends on the polymer concentration, viscoelasticity, gelation speed, shear thinning behavior, hydrophilicity and crosslinking properties.
Natural polymers are widely found in animal, plant, and microbial tissues as the main component of extracellular matrix (ECM). At present, commonly used natural polymers for 3D organ bioprinting include alginate, nanocellulose, collagen, gelatin, among others.
Alginate is currently the main plant source used for cardiac printing research. Alginate is a polymer composed of β-D-Mannuronic acid (M) and α-l-guluronic acid (G). The M:G ratio will eventually affect the physiochemical properties of alginate [71]. With a higher concentration of M, alginate shows higher flexibility, whereas with a higher G content, it shows higher rigidity and gelation. Alginate will actively crosslink when added to the culture solution, and this crosslink will be promoted when the photo-initiator is present. Alginate solutions will crosslink instantly when exposed to Ca2+. Although the crosslinking takes place very quickly, the post-crosslinked structure is not stable enough and easy to fall apart. The solution to this can be a barium chloride solution [72]. At the same time, the alginate cross-linked hydrogel has a restricted cell viscosity. Because it has a tendency to increase the loss modulus and decrease the storage modulus, it is usually more prone to plastic deformation, which means that when using extrusion printers, the extrusion pressure requirements increase with the increase in viscosity [73]. However, the increase in pressure will lead to a decrease in cell viability and also affect structural stability. Considering the flow rate, the reduction in viscosity caused by the increase in temperature or pressure, and the compatibility with cells, the best printing temperature for this hydrogel is around 37 °C. Cells can be stored at 0–30 °C under the encapsulation of alginate [74].
Nanocellulose is another widely used candidate. Despite the wide range of cellulose sources and low cost, the production of nanocellulose requires intensive processing and improvement processes. Nanocellulose exhibits the characteristics of a non-Newtonian fluid and exhibits shear thinning behavior, [75] that is, the pressure increases and the viscosity decreases. Due to the lack of cellulase, enzyme that hydrolyses cellulose, in the human body, nanocellulose cannot be degraded together with hydrogel. This non-degradable material has not yet been widely tested, but its long-term presence in the body may affect the risk of delayed immunogenicity.
Collagen is also a very strong candidate material for biomanufacturing. It is abundant in the extracellular matrix, provides mechanical strength, realizes the structural organization of cells and tissue compartments, and acts as a reservoir for cell adhesion and signaling molecules [76]. However, 3D bioprinting of composite scaffolds with natural unmodified form of collagen is difficult, because its gelation is usually accompanied by heat-driven self-assembly that is difficult to control [77]. In order to improve this, frequently used methods include chemical modification of collagen into ultraviolet (UV) cross-linkable forms, adjustment of pH, temperature and collagen concentration to control gelation and printing fidelity, or its denaturation to gelatin to make it reversible. Recently, Lee et al. [78] developed a method that uses rapid changes in pH to drive the self-assembly of collagen in a buffer support material, thereby using collagen that is not chemically modified as bioink.
Gelatin is a partially hydrolyzed collagen, derived from different animal tissues. Gelatin is a typical heat-sensitive natural polymer, and has excellent water solubility, biocompatibility, biodegradability, and 3D printability. When the gelatin hydrogel is implanted in the body, there is no inflammation or other adverse reactions [79]. The liquefaction temperature of gelatin is approximately 28 °C, suggesting a wide range of cell durability. Various gelatin-based composite bioinks have been explored, such as gelatin/alginate, gelatin/fibrin, etc. Using the properties of different cross-linking materials, such as heat sensitivity, photosensitivity, ionic or biological cross-linking, composite gelatin is usually designed as a two-step crosslinking strategy [80]. To note, gelatin methacrylamide (GelMA) has been widely used in LAB and DLP technology as a photopolymerizable biological ink in recent years [81],[82].
Synthetic polymers are artificial polymers produced through chemical reactions. Compared with natural polymers, synthetic polymers have better mechanical properties but limited cell compatibility [83]. For most biodegradable synthetic polymer solutions, the curing temperature is usually −20 to −40 °C, while melting temperature is usually 100–200 °C [84]. Therefore, most synthetic polymers have been used as support or sacrificial structures without direct contact with living cells. Commonly used synthetic polymers are PLGA, poly glycolic acid (PGA), poly hydroxypropyl methacrylamide (PHPMA), PU, PCL, PLA, and poly methyl methacrylate (PMMA). One with the most potential is PLGA. PLGA is a synthetic copolymer of lactic acid and glycolic acid. It can be hydrolyzed in the water by breaking the ester linkages [85]. The final degradation products of PLGA are acidic monomers, such as lactic acid and glycolic acid. It has been shown that the time required to degrade PLGA is related to the ratio of monomers, which can be reflected in the molecular composition. Compared with lactide-based polymers, the higher the content of glycolide units, the shorter the time required for degradation [86].
Cardiac cell sheets are engineered patches that could be used to help prevent heart failure and dilatation after acute myocardial infarction or to restore function to failing hearts. The purpose of these patches is to promote cell delivery, retention, and differentiation, offer mechanical support, conduct electrical signals, and even provide mechanical force for contractions.
Cell sheets are produced by separating monolayers of cells that grow in confluence from the culture surface. Then, functional tissues can be manufactured by layering the obtained cell sheets without scaffolds or complicated operations [87]. Research on new technologies has resulted in improvements to cell sheet technology. Homma et al. [88] developed a temperature-sensitive culture dish that uses temperature changes to obtain shed cell sheets, avoiding the use of trypsin. After the researchers performed unidirectional mechanical stretching on the harvested cell sheet, the cardiomyocytes in the cell sheet showed a unidirectional alignment. After being transplanted, the cell sheet was observed to be penetrated by the patient's original blood vessel, suggesting the future possibility of microvascular formation. Wang et al. [89] found that inhibition of Notch signaling can promote the production of synchronized contraction of cardiomyocytes.
Sacrificial materials or DLP technology can be used to design the micro-patterning of the cell sheet. Redd et al. [90] printed patterned vascular grafts by implanting human embryonic stem cell-derived endothelial cells (hESC-ECs) into patterned microchannels (Figure 3B) surrounding collagen matrix. After being transplanted into mice with myocardial infarction, the patterned graft survived and showed perfusion. Shimizu et al. [91] used water-soluble sacrificial polyvinyl alcohol (PVA) to produce a vascular mold with high biocompatibility. In this mold, a stretchable blood vessel network with a diameter of 300µm can be produced. Ma et al. [92] used a DLP-based 3D bioprinting system (Figure 3C) to embed hiPSC-derived cells and support them into a 3D miniature hexagonal hydrogel structure, thereby gradually.
The premise of making a complete engineered organ is to build a scaffold that can seed and grow cells. Scaffolds can usually be made of synthetic and natural polymers or derived from decellularized tissue. Decellularization uses chemicals, enzymes or physical methods to remove cells in heterogeneous or homogeneous organs, and then reseeds the resulting tissues or organs with the recipient's cells [93]. In 2008, Taylor and colleagues [94] successfully completed perfusion decellularization of the entire heart in a rat model for the first time. The main advantage of the decellularized scaffold is that it retains the macroscopic and microscopic structural integrity of the original tissue while ensuring low immune rejection. Still, this method still has certain drawbacks. For example, the size and structure of the organs produced depends largely on the decellularized scaffolds. Moreover, like traditional organ transplantation, suitable pre-scaffolds are not always sufficient since this kind of scaffolds may always be made from the organs of the deceased.
To make the engineered tissue have appropriate local mechanical and physiological specificity, a mixed formula of a variety of different cells and materials is usually used [95]. Hockaday et al. [96] produced a printed valve as a one-way channel for blood flow. The leaflets are highly compliant to ensure effective and flexible opening, while the sinus root wall is significantly more rigid, helping to maintain an open lumen under high hemodynamic load. Recently, Lee et al. [78] used collagen as the structural component, combined with the high-density cell bioink, to print a model of the left ventricle. According to sarcomeric α-actinin–positive myofibrils immunofluorescence staining, ventricles were synchronized with the tightly connected hESC-CM dense layer of striated muscle.
In cardiac tissue engineering, the major difficulty is the printing of microvascular networks. Francoise et al. [97] used multicellular spheres and hydrogel crosslinked with agarose as bioink. By printing layer by layer, the inner cavity and outline composed of hydrogel are constructed, so that the printed structure of the multicellular spheres form a preset shape. After the multicellular spheres fuse into a continuous tissue, the hydrogel that serves as a support and shape structure is removed (Figure 3A). By changing the print volume of the central hydrogel, the inner diameter of the vascular structure can be controlled. Interestingly, using CD31 staining, it can be observed that human aortic endothelial cell (HAEC) spontaneously agammaegated to the periphery of the tissue within three days, with ECM protein beginning to be produced at the periphery of the cell, mimicking vascular physiology. Lee et al. [78] used collagen to create a suspension culture system, in which the blood vessels designed according to a space-filling branching network 3D Voronoi lattice model can ensure the unobstructed blood of about 100 µm in diameter.
However, due to the small inner diameter of the capillaries, it is not easy to generate the engineered capillaries directly by printer. Indirect strategies were devised to take advantage of the spontaneous formation of blood vessels. In an early research, Madden and colleagues [98] found that cardiomyocytes occupy pores in porous scaffold, while non-cardiomyocytes occupy other positions. They use a serum-free culture environment to kill non-cardiomyocytes, the empty left by dead cells becoming channels, the hollow microchannel network could then become perfusable microvessels. It has been shown that the matrix encapsulated with endothelial cells tends to promote vasculogenesis. Zhang et al. [99] used composite bioink encapsulating human umbilical vein endothelial cells (HUVECs) to print microfiber scaffolds. The HUVECs migrate to the periphery of the microfibers to form a layer of confluent endothelium (Figure 3D). The cardiomyocytes are seeded into the gaps of the endothelialized scaffold to mimic a natural structure. Cui H et al. [100] evaluated engineered small-diameter vasculature with smooth muscle and endothelium, showing feasible vascular permeability and biocompatibility [101],[102].
Another indirect strategy utilizes angiogenesis, which usually refers to the sprouting of capillaries from pre-existing blood vessels [103],[104]. First, endothelial progenitor cells (EPC) differentiate into mature ECs. These cells proliferate in the original avascular area and create the first primitive vascular network. Then, ECs release matrix metalloproteinases (MMP) to degrade the surrounding extracellular matrix. Cells migrate into the newly formed gap and germinate into new blood vessels. Inui A et al. [105] used decellularized porcine small intestine to co-culture with non-vascular human heart cell sheets (Figure 3E). The formation of capillaries was observed after 6 days of culture. In order to promote the formation of blood vessels, vascular endothelial growth factor (VEGF) or fibronectin can be added to the bioink. The main disadvantage of the indirect strategy is that the randomness of spontaneous blood vessel formation is difficult to precisely control and the whole process is slow. At the same time, this strategy cannot guarantee the formation of a blood-circulating network.
In this review, we see encouraging progress in tissue engineering for cardiac repair. The realization of these advances comes from the intersection of multiple disciplines. Due to excellent ability to self-renew and to differentiate into other cells, iPSCs are the most widely used source to produce CMs currently. In the past years, it is often necessary to express Yamanaka factors by integrating viruses or introducing proteins. Although the use of PiggyBac can reduce the potential risks of introducing new genes, the low efficiency of reprogramming is still the main reason hindering mass production of CM. The development of SCNT technology and CRISPRa/dCas9-SunTag technology has greatly improved the efficiency of somatic cell reprogramming to pluripotency, and because there is no gene insertion, the iPSC obtained after reprogramming has less biological toxicity. It is worth mentioning that in the epigenetic study of the transition from somatic cells to pluripotency, the cell type traversed a variety of boundary states [5], suggesting that the continued expression of Yamanaka factors to promote the transition from the transition state to pluripotency is a feasible solution to improve the efficiency of reprogramming.
Simulating the in vivo environment, such as adding growth factors and small molecules in order to simulate the metabolic environment in the body, can improve the specific differentiation of iPSC to CM. In the past research, the small-scale production of CM has realized efficient differentiation. However, mass production still faces lower efficiency. At the same time, since the differentiated cells are not pure CM, different separation methods are used such as glucose starvation and flow cytometry.
The exciting development of transdifferentiation technology makes it possible to directly transform somatic cells into CMs in vitro, although this technology is still a long way from being widely and efficiently used. We expect that future research will use new technology such as CRISPRa to improve the efficiency of transdifferentiation. The future maturity and development of this technology will bring a new array of opportunities to tissue engineering.
After transplantation, cell sheet can gradually achieve synchronization with the heart. Multilayer cell sheets obtained by specific culture methods [106] are currently the most promising technology for clinical applications. Maturity of this technology will help more patients with heart damage.
The development of 3D bioprinting provides the possibility of using iPSC-CMs to make transplantable organ replacements. While advances in material chemistry and mechanical engineering continue to increase the speed and resolution of 3D printing, the cost of printing is also decreasing. DLP technology and sacrificial material technology allow researchers to design complex micro-patterns and the recently invented CAL technology will further simplify the manufacture of complex tissues.
Cardiac ECM is customarily regarded as an inert web of fibrous proteins, providing structural support and synergizing myocyte contraction from the cellular to the organ level. But more and more studies have suggested that ECM plays a much broader foundational role by directing cell survival, migration, proliferation, anoikis, apoptosis, and inflammatory responses [107]. At the same time, it is worth mentioning that the abundant proteases in cardiac ECM play a vital role in the maintenance and repair of heart function. For example, matrix metalloproteinase 9 (MMP9) is in close connection with neovascularization [108]. This suggests that the simulation of specific extracellular matrix is indispensable in tissue engineering.
The creation of capillaries presents the main challenge in cardiac tissue engineering. In past research, a variety of strategies for printing blood vessels have been suggested. The success of direct printing depends on engineering materials and device resolution, but it still remains challenging to print capillaries. Indirect blood vessel formation depends on the migration of endothelial cells. In the presence of pre-existing blood vessels, this spontaneous process can be accelerated. However, the spontaneous process is random and cannot guarantee the formation of a recirculating vascular network.
The capillaries are spread throughout the heart, and their role is to provide oxygen to cells and exchange metabolites. The limiting energy supply diameter of blood vessels is 200 µm [103]. The author believes that this suggests the possibility of constructing a relatively large diameter blood vessel network equivalent to a capillary network, although it may bring about changes in mechanical properties. To truly successfully print a complete functional heart, there are still many difficulties that need to be overcome, for example, billions of cells are needed for the production of large tissues [109]. At the same time, a complete heart contains a variety of cell types, and different functional local cellular organizations have different cell distributions, which means that only-CM-based printing is not sufficient.
Despite the current challenges, we also believe that research and breakthroughs in molecular, cellular, and multicellular bioengineering will deepen our understanding of tissue engineering and look forward to its application in biomedicine.
[1] | Cardiovascular diseases (CVDs), World Health Organization Available from: https://www.who.int/health-topics/cardiovascular-diseases. |
[2] |
Fuchs M, Schibilsky D, Zeh W, et al. (2019) Does the heart transplant have a future? Eur J Cardio-thorac 55: i38-i48. doi: 10.1093/ejcts/ezz107
![]() |
[3] |
Qasim M, Haq F, Kang M, et al. (2019) 3D printing approaches for cardiac tissue engineering and role of immune modulation in tissue regeneration. Int J Nanomed 14: 1311-1333. doi: 10.2147/IJN.S189587
![]() |
[4] |
Takahashi K, Yamanaka S (2006) Induction of pluripotent stem cells from mouse embryonic and adult fibroblast cultures by defined factors. Cell 126: 663-676. doi: 10.1016/j.cell.2006.07.024
![]() |
[5] |
Smith Z, Sindhu C, Meissner A (2016) Molecular features of cellular reprogramming and development. Nat Rev Mol Cell Biol 17: 139-154. doi: 10.1038/nrm.2016.6
![]() |
[6] |
Okita K, Ichisaka T, Yamanaka S (2007) Generation of germline-competent induced pluripotent stem cells. Nature 448: 313-317. doi: 10.1038/nature05934
![]() |
[7] |
Yoshida Y, Yamanaka S (2017) Induced pluripotent stem cells 10 years later. Circ Res 120: 1958-1968. doi: 10.1161/CIRCRESAHA.117.311080
![]() |
[8] |
Maherali N, Sridharan R, Xie W, et al. (2007) Directly reprogrammed fibroblasts show global epigenetic remodeling and widespread tissue contribution. Cell Stem Cell 1: 55-70. doi: 10.1016/j.stem.2007.05.014
![]() |
[9] |
Somers A, Jean JC, Sommer CA, et al. (2010) Generation of transgene-free lung disease-specific human induced pluripotent stem cells using a single excisable lentiviral stem cell cassette. Stem cells 28: 1728-1740. doi: 10.1002/stem.495
![]() |
[10] | Fusaki N, Ban H, Nishiyama A, et al. (2009) Efficient induction of transgene-free human pluripotent stem cells using a vector based on Sendai virus, an RNA virus that does not integrate into the host genome. P Jpn Acad B Phys 85: 348-362. |
[11] |
Carey BW, Markoulaki S, Hanna J, et al. (2009) Reprogramming of murine and human somatic cells using a single polycistronic vector. Proc Natl Acad Sci USA 106: 157-162. doi: 10.1073/pnas.0811426106
![]() |
[12] |
Stadtfeld M, Nagaya M, Utikal J, et al. (2008) Induced pluripotent stem cells generated without viral integration. Science 322: 945-949. doi: 10.1126/science.1162494
![]() |
[13] |
McLenachan S, Sarsero J, Ioannou P (2007) Flow-cytometric analysis of mouse embryonic stem cell lipofection using small and large DNA constructs. Genomics 89: 708-720. doi: 10.1016/j.ygeno.2007.02.006
![]() |
[14] |
Subramanyam D, Lamouille S, Judson R, et al. (2011) Multiple targets of miR-302 and miR-372 promote reprogramming of human fibroblasts to induced pluripotent stem cells. Nat Biotechnol 29: 443-448. doi: 10.1038/nbt.1862
![]() |
[15] |
Kim D, Kim CH, Moon JI, et al. (2009) Generation of human induced pluripotent stem cells by direct delivery of reprogramming proteins. Cell Stem Cell 4: 472-476. doi: 10.1016/j.stem.2009.05.005
![]() |
[16] |
Warren L, Manos PD, Ahfeldt T, et al. (2010) Highly efficient reprogramming to pluripotency and directed differentiation of human cells with synthetic modified mRNA. Cell Stem Cell 7: 618-630. doi: 10.1016/j.stem.2010.08.012
![]() |
[17] |
Malik N, Rao MS (2013) A review of the methods for human iPSC derivation. Pluripotent Stem Cells: Methods and Protocols Totowa: Humana Press, 23-33. doi: 10.1007/978-1-62703-348-0_3
![]() |
[18] |
Hong H, Takahashi K, Ichisaka T, et al. (2009) Suppression of induced pluripotent stem cell generation by the p53–p21 pathway. Nature 460: 1132-1135. doi: 10.1038/nature08235
![]() |
[19] |
Zhao S, Jiang E, Chen S, et al. (2016) PiggyBac transposon vectors: the tools of the human gene encoding. Transl Lung Cancer Res 5: 120-125. doi: 10.21037/tcr.2016.04.02
![]() |
[20] |
Al Abbar A, Ngai SC, Nograles N, et al. (2020) Induced pluripotent stem cells: Reprogramming platforms and applications in cell replacement therapy. Biores Open Access 9: 121-136. doi: 10.1089/biores.2019.0046
![]() |
[21] |
Gouveia C, Huyser C, Egli D, et al. (2020) Lessons learned from somatic cell nuclear transfer. Int J Mol Sci 21: 2314. doi: 10.3390/ijms21072314
![]() |
[22] |
Tsuji Y, Kato Y, Tsunoda Y (2009) The developmental potential of mouse somatic cell nuclear-transferred oocytes treated with trichostatin A and 5-aza-2′-deoxycytidine. Zygote 17: 109-115. doi: 10.1017/S0967199408005133
![]() |
[23] |
Polstein LR, Perez-Pinera P, Kocak DD, et al. (2015) Genome-wide specificity of DNA binding, gene regulation, and chromatin remodeling by TALE- and CRISPR/Cas9-based transcriptional activators. Genome Res 25: 1158-1169. doi: 10.1101/gr.179044.114
![]() |
[24] |
Weltner J, Balboa D, Katayama S, et al. (2018) Human pluripotent reprogramming with CRISPR activators. Nat Commun 9: 2643. doi: 10.1038/s41467-018-05067-x
![]() |
[25] |
Liu P, Chen M, Liu Y, et al. (2017) CRISPR-based chromatin remodeling of the endogenous Oct4 or Sox2 locus enables reprogramming to pluripotency. Cell Stem Cell 22: 252-261.e4. doi: 10.1016/j.stem.2017.12.001
![]() |
[26] |
Ben JR, Shemer Y, Binah O (2018) Genome editing in induced pluripotent stem cells using CRISPR/Cas9. Stem Cell Rev Rep 14: 323-336. doi: 10.1007/s12015-018-9811-3
![]() |
[27] |
Brodehl A, Ebbinghaus H, Deutsch MA, et al. (2019) Human induced pluripotent stem-cell-derived cardiomyocytes as models for genetic cardiomyopathies. Int J Mol Sci 20: 4381. doi: 10.3390/ijms20184381
![]() |
[28] |
Kempf H, Zweigerdt R (2017) Scalable cardiac differentiation of pluripotent stem cells using specific growth factors and small molecules. Engineering and Application of Pluripotent Stem Cells Cham: Springer, 39-69. doi: 10.1007/10_2017_30
![]() |
[29] |
Wu S, Cheng CM, Lanz RB, et al. (2013) Atrial identity is determined by a COUP-TFII regulatory network. Dev Cell 25: 417-426. doi: 10.1016/j.devcel.2013.04.017
![]() |
[30] |
Prowse AB, Timmins NE, Yau TM, et al. (2014) Transforming the promise of pluripotent stem cell-derived cardiomyocytes to a therapy: challenges and solutions for clinical trials. Can J Cardiol 30: 1335-1349. doi: 10.1016/j.cjca.2014.08.005
![]() |
[31] |
Evans SM, Yelon D, Conlon FL, et al. (2010) Myocardial lineage development. Circ Res 107: 1428-1444. doi: 10.1161/CIRCRESAHA.110.227405
![]() |
[32] | Mahmood T, Nasser A, Hossein B (2015) Human cardiomyocyte generation from pluripotent stem cells: A state-of-art. Life Sciences 145: 98-113. |
[33] |
Guo NN, Liu LP, Zheng YW, et al. (2020) Inducing human induced pluripotent stem cell differentiation through embryoid bodies: A practical and stable approach. J Stem Cells 12: 25-34. doi: 10.4252/wjsc.v12.i1.25
![]() |
[34] |
Brickman JM, Serup P (2017) Properties of embryoid bodies. WIREs Dev Biol 6: e259. doi: 10.1002/wdev.259
![]() |
[35] |
Yang L, Soonpaa MH, Adler ED, et al. (2008) Human cardiovascular progenitor cells develop from a KDR+ embryonic-stem-cell-derived population. Nature 453: 524-528. doi: 10.1038/nature06894
![]() |
[36] |
Kattman J, Witty AD, Gagliardi M, et al. (2011) Stage-specific optimization of activin/nodal and BMP signaling promotes cardiac differentiation of mouse and human pluripotent stem cell lines. Cell Stem Cell 8: 228-240. doi: 10.1016/j.stem.2010.12.008
![]() |
[37] |
Besser RR, Ishahak M, Mayo V, et al. (2018) Engineered microenvironments for maturation of stem cell derived cardiac myocytes. Theranostics 8: 124-140. doi: 10.7150/thno.19441
![]() |
[38] |
Tohyama S, Hattori F, Sano M, et al. (2012) Distinct metabolic flow enables large-scale purification of mouse and human pluripotent stem cell-derived cardiomyocytes. Cell Stem Cell 12: 127-137. doi: 10.1016/j.stem.2012.09.013
![]() |
[39] |
Mummery CL, Zhang J, Elliott DA, et al. (2012) Differentiation of human embryonic stem cells and induced pluripotent stem cells to cardiomyocytes: a methods overview. Circ Res 111: 344-358. doi: 10.1161/CIRCRESAHA.110.227512
![]() |
[40] |
Graichen R, Xu X, Braam SR, et al. (2008) Enhanced cardiomyogenesis of human embryonic stem cells by a small molecular inhibitor of p38 MAPK. Differentiation 76: 357-370. doi: 10.1111/j.1432-0436.2007.00236.x
![]() |
[41] |
Vlahos CJ, Matter WF, Hui KY, et al. (1994) A specific inhibitor of phosphatidylinositol3-kinase, 2-(4-morpholinyl)-8-phenyl-4H-1-benzopyran-4-one (LY294002). J Biol Chem 269: 5241-5248. doi: 10.1016/S0021-9258(17)37680-9
![]() |
[42] |
Ieda M, Fu JD, Delgado P, et al. (2010) Direct reprogramming of fibroblasts into functional cardiomyocytes by defined factors. Cell 142: 375-386. doi: 10.1016/j.cell.2010.07.002
![]() |
[43] |
Jayawardena TM, Egemnazarov B, Finch EA, et al. (2012) MicroRNA-mediated in vitro and in vivo direct reprogramming of cardiac fibroblasts to cardiomyocytes. Circ Res 110: 1465-1473. doi: 10.1161/CIRCRESAHA.112.269035
![]() |
[44] |
Burridge PW, Matsa E, Shukla P, et al. (2014) Chemically defined generation of human cardiomyocytes. Nat Methods 11: 855-860. doi: 10.1038/nmeth.2999
![]() |
[45] |
Srivastava D, DeWitt N (2016) In vivo cellular reprogramming: the next generation. Cell 166: 1386-1396. doi: 10.1016/j.cell.2016.08.055
![]() |
[46] |
Chen Y, Yang Z, Zhao ZA, et al. (2017) Direct reprogramming of fibroblasts into cardiomyocytes. Stem Cell Res Ther 8: 118. doi: 10.1186/s13287-017-0569-3
![]() |
[47] |
Wang J, Jiang X, Zhao L, et al. (2019) Lineage reprogramming of fibroblasts into induced cardiac progenitor cells by CRISPR/Cas9-based transcriptional activators. Acta Pharm Sin B 10: 313-326. doi: 10.1016/j.apsb.2019.09.003
![]() |
[48] |
Murphy SV, Atala A (2014) 3D bioprinting of tissues and organs. Nat Biotechnol 32: 773-785. doi: 10.1038/nbt.2958
![]() |
[49] |
Cui X, Boland T, DLima DD, et al. (2012) Thermal inkjet printing in tissue engineering and regenerative medicine. Recent Pat Drug Deliv Formul 6: 149-155. doi: 10.2174/187221112800672949
![]() |
[50] |
Cui X, Dean D, Ruggeri ZM, et al. (2010) Cell damage evaluation of thermal inkjet printed Chinese hamster ovary cells. Biotechnol Bioeng 106: 963-969. doi: 10.1002/bit.22762
![]() |
[51] |
Fang Y, Frampton JP, Raghavan S, et al. (2012) Rapid generation of multiplexed cell cocultures using acoustic droplet ejection followed by aqueous two-phase exclusion patterning. Tissue Eng Part C-Me 18: 647-657. doi: 10.1089/ten.tec.2011.0709
![]() |
[52] |
Jones N (2012) Science in three dimensions: the print revolution. Nature 487: 22-23. doi: 10.1038/487022a
![]() |
[53] |
Mironov V, Visconti RP, Kasyanov V, et al. (2008) Organ printing: tissue spheroids as building blocks. Biomaterials 30: 2164-2174. doi: 10.1016/j.biomaterials.2008.12.084
![]() |
[54] |
Zhang YS, Pi Q, van Genderen AM Microfluidic bioprinting for engineering vascularized tissues and organoids (2017) . doi: 10.3791/55957
![]() |
[55] |
Bohandy J, Kim B, Adrian F (1986) Metal deposition from a supported metal film using an excimer laser. J Appl Phys 60: 1538-1539. doi: 10.1063/1.337287
![]() |
[56] |
Guillemot F, Souquet A, Catros S, et al. (2010) Laser-assisted cell printing: principle, physical parameters versus cell fate and perspectives in tissue engineering. Nanomedicine 5: 507-515. doi: 10.2217/nnm.10.14
![]() |
[57] |
Guillotin B, Souquet A, Catros S, et al. (2010) Laser assisted bioprinting of engineered tissue with high cell density and microscale organization. Biomaterials 31: 7250-7256. doi: 10.1016/j.biomaterials.2010.05.055
![]() |
[58] |
Kattamis NT, Purnick PE, Weiss R, et al. (2007) Thick film laser induced forward transfer for deposition of thermally and mechanically sensitive materials. Appl Phys Lett 91: 171120-171123. doi: 10.1063/1.2799877
![]() |
[59] |
Gauvin R, Chen YC, Lee JW, et al. (2012) Microfabrication of complex porous tissue engineering scaffolds using 3D projection stereolithography. Biomaterials 33: 3824-3834. doi: 10.1016/j.biomaterials.2012.01.048
![]() |
[60] |
Alonzo M, AnilKumar S, Roman B, et al. (2019) 3D Bioprinting of cardiac tissue and cardiac stem cell therapy. Transl Res 211: 64-83. doi: 10.1016/j.trsl.2019.04.004
![]() |
[61] |
Bishop ES, Mostafa S, Pakvasa M, et al. (2017) 3-D bioprinting technologies in tissue engineering and regenerative medicine: Current and future trends. Genes Dis 4: 185-195. doi: 10.1016/j.gendis.2017.10.002
![]() |
[62] |
Morris VB, Nimbalkar S, Younesi M, et al. (2017) Mechanical properties, cytocompatibility and manufacturability of chitosan: PEGDA hybrid-gel scaffolds by stereolithography. Ann Biomed Eng 45: 286-296. doi: 10.1007/s10439-016-1643-1
![]() |
[63] |
Lu Y, Mapili G, Suhali G, et al. (2006) A digital micro-mirror device-based system for the microfabrication of complex, spatially patterned tissue engineering scaffolds. J Biomed Mater Res A 77: 396-405. doi: 10.1002/jbm.a.30601
![]() |
[64] |
Zhang J, Hu Q, Wang S, et al. (2019) Digital light processing based three-dimensional printing for medical applications. Int J Bioprint 6: 242. doi: 10.18063/ijb.v6i1.242
![]() |
[65] |
Kelly BE, Bhattacharya I, Heidari H, et al. (2019) Volumetric additive manufacturing via tomographic reconstruction. Science 363: 1075-1079. doi: 10.1126/science.aau7114
![]() |
[66] |
Türker E, Demirçak N, Arslan YA (2018) Scaffold-free three-dimensional cell culturing using magnetic levitation. Biomater Sci 6: 1745-1753. doi: 10.1039/C8BM00122G
![]() |
[67] |
Matai I, Kaur G, Seyedsalehi A, et al. (2019) Progress in 3D bioprinting technology for tissue/organ regenerative engineering. Biomaterials 226: 119536. doi: 10.1016/j.biomaterials.2019.119536
![]() |
[68] |
Tseng H, Gage JA, Haisler WL, et al. (2016) A high-throughput in vitro ring assay for vasoactivity using magnetic 3D bioprinting. Sci Rep 6: 30640. doi: 10.1038/srep30640
![]() |
[69] |
Bowser DA, Moore MJ (2019) Biofabrication of neural microphysiological systems using magnetic spheroid bioprinting. Biofabrication 12: 015002. doi: 10.1088/1758-5090/ab41b4
![]() |
[70] |
Wang X (2019) Advanced polymers for three-dimensional (3D) organ bioprinting. Micromachines 10: 814. doi: 10.3390/mi10120814
![]() |
[71] |
Axpe E, Oyen ML (2016) Applications of alginate-based bioinks in 3D bioprinting. Int J Mol Sci 17: E1976. doi: 10.3390/ijms17121976
![]() |
[72] |
Bajpai SK, Sharma S (2004) Investigation of swelling/degradation behaviour of alginate beads crosslinked with Ca2+ and Ba2+ ions. React Funct Polym 59: 129-140. doi: 10.1016/j.reactfunctpolym.2004.01.002
![]() |
[73] |
Gao T, Gillispie GJ, Copus JS, et al. (2018) Optimization of gelatin–alginate composite bioink printability using rheological parameters: a systematic approach. Biofabrication 10: 034106. doi: 10.1088/1758-5090/aacdc7
![]() |
[74] |
Giuseppe MD, Law N, Webb BA, et al. (2018) Mechanical behaviour of alginate-gelatin hydrogels for 3D bioprinting. J Mech Behav Biomed Mater 79: 150-157. doi: 10.1016/j.jmbbm.2017.12.018
![]() |
[75] |
Markstedt K, Mantas A, Tournier I, et al. (2015) 3D bioprinting human chondrocytes with nanocellulose-alginate bioink for cartilage tissue engineering applications. Biomacromolecules 16: 1489-1496. doi: 10.1021/acs.biomac.5b00188
![]() |
[76] |
Frantz C, Stewart KM, Weaver VM (2010) The extracellular matrix at a glance. J Cell Sci 123: 4195-4200. doi: 10.1242/jcs.023820
![]() |
[77] |
Drzewiecki KE, Parmar AS, Gaudet ID, et al. (2014) Methacrylation induces rapid, temperature-dependent, reversible self-assembly of type-I collagen. Langmuir 30: 11204-11211. doi: 10.1021/la502418s
![]() |
[78] |
Lee A, Hudson AR, Shiwarski DJ, et al. (2019) 3D bioprinting of collagen to rebuild components of the human heart. Science 365: 482-487. doi: 10.1126/science.aav9051
![]() |
[79] |
Wang X, Yu X, Yan Y, et al. (2008) Liver tissue responses to gelatin and gelatin/chitosan gels. J Biomed Mater Res A 87: 62-68. doi: 10.1002/jbm.a.31712
![]() |
[80] |
Skardal A, Zhang J, McCoard L, et al. (2010) Photocrosslinkable hyaluronan-gelatin hydrogels for two-step bioprinting. Tissue Eng Part A 16: 2675-2685. doi: 10.1089/ten.tea.2009.0798
![]() |
[81] |
Zhu H, Yang H, Ma Y, et al. (2020) Spatiotemporally controlled photoresponsive hydrogels: design and predictive modeling from processing through application. Adv Funct Mater 30: 2000639. doi: 10.1002/adfm.202000639
![]() |
[82] |
Xiao S, Zhao T, Wang J, et al. (2019) Gelatin methacrylate (GelMA)-based hydrogels for cell transplantation: an effective strategy for tissue engineering. Stem Cell Rev Rep 15: 664-679. doi: 10.1007/s12015-019-09893-4
![]() |
[83] |
Hoffman AS (2002) Hydrogels for biomedical applications. Adv Drug Deliv Rev 54: 3-12. doi: 10.1016/S0169-409X(01)00239-3
![]() |
[84] |
Jungst T, Smolan W, Schacht K, et al. (2016) Strategies and molecular design criteria for 3D printable hydrogels. Chem Rev 116: 1496-1539. doi: 10.1021/acs.chemrev.5b00303
![]() |
[85] |
Astete CE, Sabliov CM (2006) Synthesis and characterization of PLGA nanoparticles. J Biomater Sci Polym Ed 17: 247-289. doi: 10.1163/156856206775997322
![]() |
[86] |
Samadi N, Abbadessa A, Di Stefano A, et al. (2013) The effect of lauryl capping group on protein release and degradation of poly (D, L-lactic-co-glycolic acid) particles. J Control Release 172: 436-443. doi: 10.1016/j.jconrel.2013.05.034
![]() |
[87] |
Mazzola M, Pasquale E (2020) Toward cardiac regeneration: Combination of pluripotent stem cell-based therapies and bioengineering strategies. Front Bioeng Biotechnol 8: 455. doi: 10.3389/fbioe.2020.00455
![]() |
[88] |
Homma J, Shimizu S, Sekine H, et al. (2020) A novel method to align cells in a cardiac tissue-like construct fabricated by cell sheet-based tissue engineering. J Tissue Eng Regen Med 14: 944-954. doi: 10.1002/term.3074
![]() |
[89] |
Wang Z, Lee SJ, Cheng HJ, et al. (2018) 3D bioprinted functional and contractile cardiac tissue constructs. Acta Biomater 70: 48-56. doi: 10.1016/j.actbio.2018.02.007
![]() |
[90] |
Redd MA, Zeinstra N, Qin W, et al. (2019) Patterned human microvascular grafts enable rapid vascularization and increase perfusion in infarcted rat hearts. Nat Commun 10: 584. doi: 10.1038/s41467-019-08388-7
![]() |
[91] |
Shimizu A, Goh WH, Itai S, et al. (2020) ECM-based microchannel for culturing in vitro vascular tissues with simultaneous perfusion and stretch. Lab Chip 20: 1917-1927. doi: 10.1039/D0LC00254B
![]() |
[92] |
Ma X, Qu X, Zhu W, et al. (2016) Deterministically patterned biomimetic human iPSC-derived hepatic model via rapid 3D bioprinting. Proc Natl Acad Sci 113: 2206-2211. doi: 10.1073/pnas.1524510113
![]() |
[93] |
Taylor DA, Sampaio LC, Ferdous Z, et al. (2018) Decellularized matrices in regenerative medicine. Acta Biomater 74: 74-89. doi: 10.1016/j.actbio.2018.04.044
![]() |
[94] |
Ott HC, Matthiesen TS, Goh SK, et al. (2008) Perfusion-decellularized matrix: using nature's platform to engineer a bioartificial heart. Nat Med 14: 213-219. doi: 10.1038/nm1684
![]() |
[95] |
Jovic TH, Kungwengwe G, Mills AC, et al. (2019) Plant-derived biomaterials: A review of 3D bioprinting and biomedical applications. Front Mech Eng 5: 19. doi: 10.3389/fmech.2019.00019
![]() |
[96] |
Marga F, Jakab K, Khatiwala C, et al. (2012) Toward engineering functional organ modules by additive manufacturing. Biofabrication 4: 022001. doi: 10.1088/1758-5082/4/2/022001
![]() |
[97] |
Hockaday LA, Kang KH, Colangelo NW, et al. (2012) Rapid 3D printing of anatomically accurate and mechanically heterogeneous aortic valve hydrogel scaffolds. Biofabrication 4: 035005. doi: 10.1088/1758-5082/4/3/035005
![]() |
[98] |
Madden LR, Mortisen DJ, Sussman EM, et al. (2010) Proangiogenic scaffolds as functional templates for cardiac tissue engineering. Proc Natl Acad Sci 107: 15211-15216. doi: 10.1073/pnas.1006442107
![]() |
[99] |
Zhang YS, Arneri A, Bersini S, et al. (2016) Bioprinting 3D microfibrous scaffolds for engineering endothelialized myocardium and heart-on-a-chip. Biomaterials 110: 45-59. doi: 10.1016/j.biomaterials.2016.09.003
![]() |
[100] |
Hann SY, Cui H, Esworthy T, et al. (2019) Recent advances in 3D printing: vascular network for tissue and organ regeneration. Transl Res 211: 46-63. doi: 10.1016/j.trsl.2019.04.002
![]() |
[101] |
Duan B, Hockaday LA, Kang KH, et al. (2013) 3D bioprinting of heterogeneous aortic valve conduits with alginate/gelatin hydrogels. J Biomed Mater Res A 101: 1255-1264. doi: 10.1002/jbm.a.34420
![]() |
[102] |
Grigoryan B, Paulsen SJ, Corbett DC, et al. (2019) Multivascular networks and functional intravascular topologies within biocompatible hydrogels. Science 364: 458-464. doi: 10.1126/science.aav9750
![]() |
[103] |
Esther C, Claudia K, Petra J (2011) Vascularization is the key challenge in tissue engineering. Adv Drug Deliv Rev 63: 300-311. doi: 10.1016/j.addr.2011.03.004
![]() |
[104] |
Kobayashi J, Akiyama Y, Yamato M, et al. (2018) Design of temperature-responsive cell culture surfaces for cell sheet-based regenerative therapy and 3D tissue fabrication. Adv Exp Med Biol 1078: 371-393. doi: 10.1007/978-981-13-0950-2_19
![]() |
[105] |
Inui A, Sekine H, Sano K, et al. (2019) Generation of a large-scale vascular bed for the in vitro creation of three-dimensional cardiac tissue. Regen Ther 11: 316-323. doi: 10.1016/j.reth.2019.10.001
![]() |
[106] |
Masuda S, Shimizu T (2015) Three-dimensional cardiac tissue fabrication based on cell sheet technology. Adv Drug Deliv Rev 96: 103-109. doi: 10.1016/j.addr.2015.05.002
![]() |
[107] |
Daley MC, Fenn SL, Black LD (2018) Applications of cardiac extracellular matrix in tissue engineering and regenerative medicine. Cardiac Extracellular Matrix Cham: Springer, 59-83. doi: 10.1007/978-3-319-97421-7_4
![]() |
[108] |
Seignez C, Phillipson M (2017) The multitasking neutrophils and their involvement in angiogenesis. Curr Opin Hematol 24: 3-8. doi: 10.1097/MOH.0000000000000300
![]() |
[109] |
Miller JS (2014) The billion cells construct: will three-dimensional printing get us there? PLoS Biol 12: e1001882. doi: 10.1371/journal.pbio.1001882
![]() |