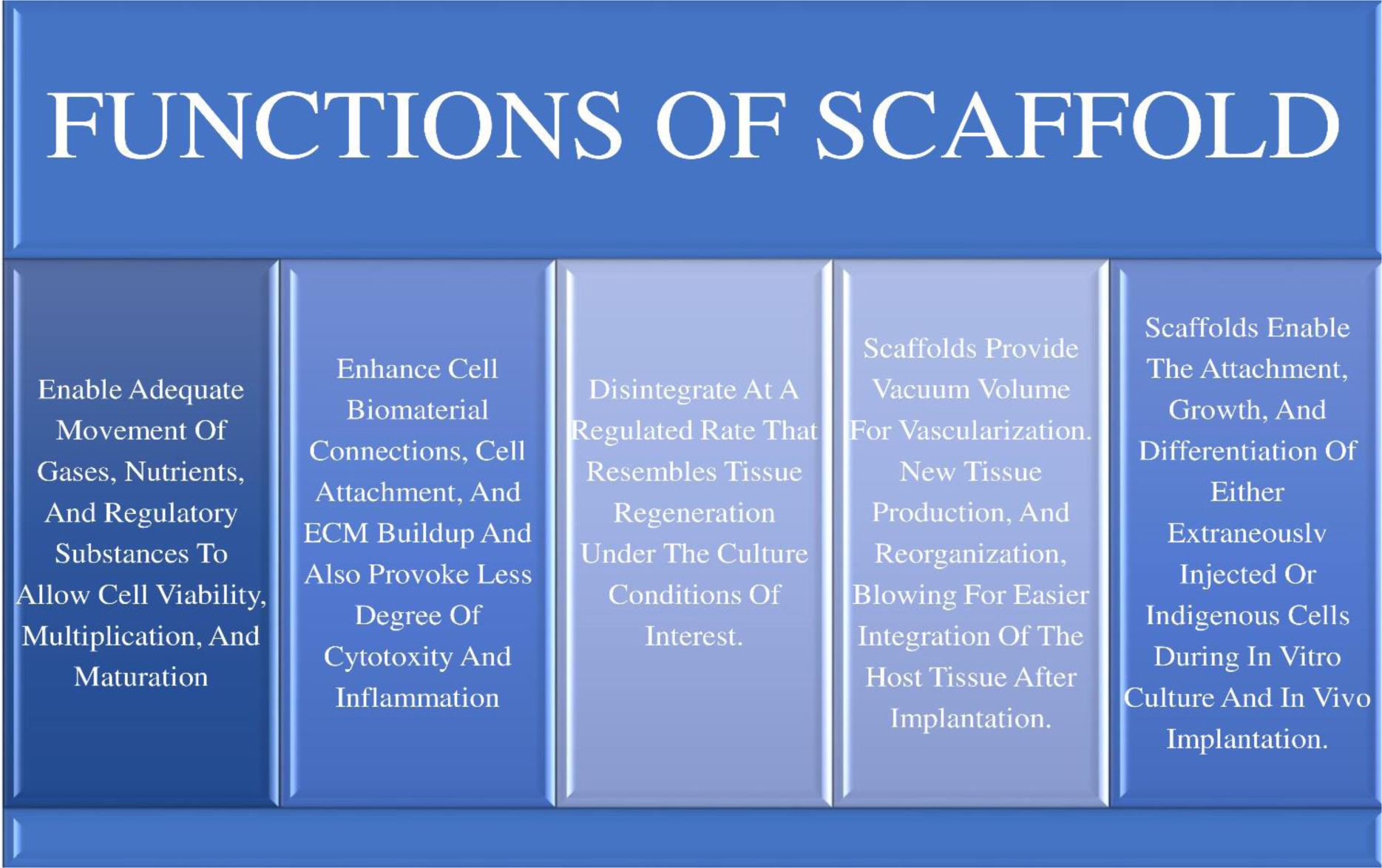
Craniofacial tissue-engineered techniques have significantly improved over the past 20 years as a result of developments in engineering and in material science. The regeneration of the craniofacial tissue is frequently complicated due to the craniofacial region's complexity, which includes bone, cartilage, soft tissue, and neurovascular bundles. It is now possible to construct tissues in the lab using scaffolds, cells, and physiologically active chemicals. For bone repair/augmentation, the biomaterials are classified into natural like “collagen, fibrin, alginate, silk, hyaluronate, chitosan” and synthetic like “polyethyleneglycol, poly-e-caprolactone, polyglycolic acid” and some bioceramics “tricalcium phosphate, hydroxyapatite, biphasic calcium phosphate, and the bioactive glasses” along with metals certain (Titanium and Zirconia ) and as this is part of advanced tissue engineering in dentistry there are some bioactive restorative materials like mineral trioxide aggregate and biodentine. The newer advanced techniques like 3D printed templates present a framework for achieving the three pillars of tissue engineering: healing, rebuilding and rejuvenation. The field of tissue engineering has recently become interested in 3D printing, also known as “Additive Manufacturing”, which is a ground-breaking technique that allows for the printing of patient-specific scaffolds, medical devices, multiscale, biomimetic/intricate cytoarchitecture/function-structure hierarchies and multicellular tissues in complex microenvironments. Biopolymers use is dependent on meeting the criteria for various scaffolds, including mechanical integrity, thermal stability, chemical composition, along with biological properties. Researchers have developed a revolutionary 4D bioprinting technique using cell traction forces and they are used to develop intricate dynamic structures, smart medical devices, or complex human organs.
Citation: Tanishka Taori, Anjali Borle, Shefali Maheshwari, Amit Reche. An insight into the biomaterials used in craniofacial tissue engineering inclusive of regenerative dentistry[J]. AIMS Bioengineering, 2023, 10(2): 153-174. doi: 10.3934/bioeng.2023011
[1] | Amit Aherwar, Amit K Singh, Amar Patnaik . Current and future biocompatibility aspects of biomaterials for hip prosthesis. AIMS Bioengineering, 2016, 3(1): 23-43. doi: 10.3934/bioeng.2016.1.23 |
[2] | Jéssica de Oliveira Rossi, Gabriel Tognon Rossi, Maria Eduarda Côrtes Camargo, Rogerio Leone Buchaim, Daniela Vieira Buchaim . Effects of the association between hydroxyapatite and photobiomodulation on bone regeneration. AIMS Bioengineering, 2023, 10(4): 466-490. doi: 10.3934/bioeng.2023027 |
[3] | Shaoyung Chen, Hsinyi Mao, Pinhua Tu, Naichen Cheng, Jiashing Yu . Fabrication of decellularized adipose tissue/alginate composite microspheres with pASCs encapsulation for tissue engineering. AIMS Bioengineering, 2017, 4(3): 351-365. doi: 10.3934/bioeng.2017.3.351 |
[4] | José Luis Calvo-Guirado . New biomaterials for bone augmentation in complicated cases. AIMS Bioengineering, 2023, 10(3): 362-363. doi: 10.3934/bioeng.2023021 |
[5] | Moawiah M Naffaa . Innovative therapeutic strategies for traumatic brain injury: integrating regenerative medicine, biomaterials, and neuroengineering. AIMS Bioengineering, 2025, 12(1): 90-144. doi: 10.3934/bioeng.2025005 |
[6] | P. Mora-Raimundo, M. Manzano, M. Vallet-Regí . Nanoparticles for the treatment of osteoporosis. AIMS Bioengineering, 2017, 4(2): 259-274. doi: 10.3934/bioeng.2017.2.259 |
[7] | Fabrizio Belleggia . Hard and soft tissue augmentation of vertical ridge defects with the “hard top double membrane technique”: introduction of a new technique and a case report. AIMS Bioengineering, 2022, 9(1): 26-43. doi: 10.3934/bioeng.2022003 |
[8] | Izgen Karakaya, Nuran Ulusoy . Basics of dentin-pulp tissue engineering. AIMS Bioengineering, 2018, 5(3): 162-178. doi: 10.3934/bioeng.2018.3.162 |
[9] | Pornchai Kittivarakarn, Matthew Penna, Zenith Acosta, Daniel Pelaez, Ramon Montero, Fotios M. Andreopoulos, Herman S. Cheung . Cardiomyotic induction and proliferation of dental stem cells on electrospun scaffolds. AIMS Bioengineering, 2016, 3(2): 139-155. doi: 10.3934/bioeng.2016.2.139 |
[10] | Maria Júlia Bento Martins Parreira, Bruna Trazzi Pagani, Matheus Bento Medeiros Moscatel, Daniela Vieira Buchaim, Carlos Henrique Bertoni Reis, Beatriz Flávia de Moraes Trazzi, Acácio Fuziy, Rogerio Leone Buchaim . Effects of systemic administration of the retinoid Isotretinoin on bone tissue: A narrative literature review. AIMS Bioengineering, 2024, 11(2): 212-240. doi: 10.3934/bioeng.2024012 |
Craniofacial tissue-engineered techniques have significantly improved over the past 20 years as a result of developments in engineering and in material science. The regeneration of the craniofacial tissue is frequently complicated due to the craniofacial region's complexity, which includes bone, cartilage, soft tissue, and neurovascular bundles. It is now possible to construct tissues in the lab using scaffolds, cells, and physiologically active chemicals. For bone repair/augmentation, the biomaterials are classified into natural like “collagen, fibrin, alginate, silk, hyaluronate, chitosan” and synthetic like “polyethyleneglycol, poly-e-caprolactone, polyglycolic acid” and some bioceramics “tricalcium phosphate, hydroxyapatite, biphasic calcium phosphate, and the bioactive glasses” along with metals certain (Titanium and Zirconia ) and as this is part of advanced tissue engineering in dentistry there are some bioactive restorative materials like mineral trioxide aggregate and biodentine. The newer advanced techniques like 3D printed templates present a framework for achieving the three pillars of tissue engineering: healing, rebuilding and rejuvenation. The field of tissue engineering has recently become interested in 3D printing, also known as “Additive Manufacturing”, which is a ground-breaking technique that allows for the printing of patient-specific scaffolds, medical devices, multiscale, biomimetic/intricate cytoarchitecture/function-structure hierarchies and multicellular tissues in complex microenvironments. Biopolymers use is dependent on meeting the criteria for various scaffolds, including mechanical integrity, thermal stability, chemical composition, along with biological properties. Researchers have developed a revolutionary 4D bioprinting technique using cell traction forces and they are used to develop intricate dynamic structures, smart medical devices, or complex human organs.
Three-Dimensional printing;
computer-aided design;
additive manufacturing;
three-dimensional printing;
solid freeform fabrication;
tissue engineering;
collagen, chitosan and tricalcium phosphate;
Poly Lactic-co Glycolic acid;
glycosaminoglycan;
Calcium Phosphate;
Hydroxyapatite;
Mesenchymal Stem Cell;
Chitosan;
Tricalcium Phosphate;
Bone Morphogenetic protein;
Silk Fibroin;
phosphate-buffered saline;
hexafluoroisopropano;
natural fibers reinforced composites;
Cellulose nanocrystal;
Nano fibrillated Cellulose;
Bacterial nanocellulose;
Polyethyleneglycol;
Poly-E-Caprolactone;
Polyglycolic Acid;
Bicalcium Phosphate;
Titanium;
Aluminium;
Vanadium;
Polyetherketoneketone;
High entrophy alloys;
Laser cladding;
Laser-aided additive manufacturing;
Laser-cladded high-entropy alloy coatings;
Bioactive glass;
Silicon Dioxide;
Sodium Dioxide;
Calcium Oxide;
Phosphorus Pentaoxide;
polyetheretherketone;
polyaryletherketone;
Bone tissue regeneration;
Regenerative Medicine;
Shape Memory Polymers;
Shape Memory Alloys;
Polyvinyl Alcohol
The craniofacial skeleton is intricate and enables a variety of functions. The ability to regenerate craniofacial structures necessitates thorough understanding of the underlying developmental processes and remodeling characteristics [1]. The bioengineering trio, which includes cells, scaffolds and growth-stimulating chemicals, is the most essential element [2]. Scaffolds are biocompatible and biodegradable, 3D porous biomaterials act as spacers, creating a foundation to connect with cells, develop, proliferate as well as migrate and diversify while also acting as a temporary support structure. Various traditional fabrication techniques, such as particle leaching, thermal electrospinning, melt moulding, phase separation, solvent casting, gas foaming, along with freeze-drying, are frequently used for fabricating scaffolds. By eliminating the shortcomings of traditional manufacturing techniques and enabling the fabrication of high-quality scaffolds through layer-by-layer stacking assisted by computer-aided design (CAD), additive manufacturing (AM), as three-dimensional printing (3DP), has been used in the modern world. Without the need of a specific mold or equipment, composite scaffolds with uniform thickness are manufactured utilizing a rapid prototyping technique, which is rarely referred to as solid freeform fabrication (SFF) [3]. They are also the transport system for cells, allowing them to stay in place and be distributed in the area where extracellular development is required [4].
A good scaffold should be bioactive, with a removal percentage that reflects the rate at which new tissues repair [5],[6]. The form and size of the implant, chemical reactivity, the technique speed and decomposition products of breakdown and the material's potential to stimulate cell-material interactions are all elements that contribute to cell activation by scaffolds [7]. Figure 1 encapsulates some functions of a scaffold.
Bioactivity, physical resiliency and stability, quality of transplantable bone, osseointegration, integrity, governed degradation, together with tissue inadequate interstitial fluid flow are all prerequisites for tissue regeneration constructs for cartilage along with bone repair in addition to maintenance. The finest scaffold for tissue engineering (TE) should replicate extracellular matrix [8]. Scaffolds are used to supply biofactors, such as molecules, genes, enzymes, proteins and peptides to the construct in order to create bone, blood vessel creation and development. The improved distribution of bio factors necessitates closely interconnected scaffolds and permits cellular dispersion, vascularization inside scaffolds, whereas obtaining structural stability similar to bone tissue necessitates slightly porous scaffolds [9]. Scaffolds must have a high diffusion of water and binding ability and in their capacity to take in inflammatory mediators and cellular components, protect them and gradually release good growth factors and inflammatory mediators to create the perfect conditions for bone repair [10]. Figure 2 and 3 incorporates desirable characteristics of scaffold for successful treatment of bone graft and the requirements of scaffolds, respectively. For decades numerous synthetic and natural biomaterials for scaffolds have been proposed for various tissue recreation/repair (Figure 4). The natural biomaterials can be of human and animal-derived (Figure 5). Synthetic polymers pose a major hazard to the environment because they produce trash that accumulates and causes plastic particles to be discovered everywhere in the biosphere, including inside living things. This encourages scientists to turn to using naturally occurring materials, which don't have any issues with waste disposal [11].
Essentially, a composite is a material that is composed of at least two different materials. Inserting natural fibres (NFs) is a widespread approach for a range of purposes, particularly in the context of sustainable materials, especially when doing so as a reinforcement in composite materials. The advantages of natural fibre reinforced polymer composites (NFRPCs), which include low weight, biodegradability, affordability, environmental acceptability, commercial availability, recyclable materials made from renewable resources, make them sustainable polymers. NFs are produced by three major types: animal based, mineral based and natural lignocellulosic fiber. Because cellulose, hemicellulose and lignin play a significant role in the structure of NFRPCs, they are more environmentally friendly than typical composites. NFRPCs are superior to synthetic fibre reinforced composites because they are abundant in nature, durable, have higher mechanical and physical qualities due to chemical treatment, can be used in ballistic applications and have little environmental effects [12].
These biomaterials originate from microorganisms, animals and plants. Bio-actuators, transmission of genes, controlled medication delivery, tissue regeneration and other applications in medicine all use this polymer (polysaccharides and proteins), which has a wide range of functions. Based on their origins and production processes, bio-based polymeric materials are divided into three distinct classes. The first category contains natural polymers such as proteins, lipids, polysaccharides, Chitosan, silk fibroin (SF), collagen, cellulose, gelatin, hemicellulose, alginate, hyaluronic acid (HA), fibrin and alginate that are derived from plants or animals [13]. Natural biopolymers have a few limitations despite having strong bioactivity, biocompatibility and biodegradability, which include poor mechanical qualities, high water solubility, potential immunogenicity, as well as denaturation during processing [14].
They are among the core parts of bone which lay out strength and structural stability to the tissue, accounting for 89 percent of the organic matrix and 32 percent of the inorganic content [15]. To adjust the physicochemical with the mechanical characteristics of the scaffold for the final application its routinely changed/cross-linked/coupled with another elements (polymers/ceramics) [16].
Real collagen has several benefits like high swelling capacity/cytocompatibility/less inflammatory response rate and the ability to produce fibres from aqueous preparations that are comparable to those seen in natural tissues [17]. Bioengineering scaffolds built on collagen, chitosan and tricalcium phosphate (CCTP) were investigated. A “Poly Lactic-co Glycolic acid (PLGA)” was placed in the CCTP template scaffold to increase its regeneration capabilities. In absence of the absence of neovascularization in the “Cobalt ion-incorporated bioactive glass/glycosaminoglycan (GAG)” template for creating bone substitute were produced, which improves vascular endothelial growth factor production [18]. When inserted ectopically in rabbits, these 3 discrete Calcium phosphates-collagen-hydroxyapitite scaffolds displayed good tensile strength as well as faster and larger bone production than regular Calcium phosphate scaffolds [19].
Fibrin's biocompatibility, and intrinsic bioactivity promotes cell migration, proliferation, as well as matrix synthesis by the incorporation of platelet-derived growth factor (PDGF) with biomolecules which have piqued the interest which used for bone augmentation. Initiators like fibrinogen and thrombin are emanate from patients' blood [20]. Fibrin can be employed to improve the behavior of cells when they are exposed to other natural polymers. Confluence of mesenchymal stem cell adhesion, volume, multiplication, maturation and calcification all enhanced significantly when fibrin was added to CS/nano—Tricalcium phosphate composites [21]. The use of fibrin in conjunction with osteoconductive ceramics which permits for insertion of growth factors, medicines and metallic particles, might lead to the creation of multimodal templates for bone substitute rejuvenation [22].
They are brown seaweed-derived water gel made up of “1,4-linked b-D-mannuronic acid together with a-L-guluronic acid”. There are gels as a result of guluronic acid blocks binding to other G blocks through cations, mainly calcium ions [23]. They are available in the form of hydrogels, microspheres and microcapsules.
A freeze-drying process was used to create 2 types of polymer biological templates, alginate alongside Chitosan and CS along with alginate including fucoidan, both of which are classified as bone transplant substitutes [24]. The mechanical strength, oxygenation, cell migration, adhesion, proliferation and structural stability of CS alginate hybrid scaffolds were enhanced, and they were demonstrated to encourage new bone growth and fast vascularisation [25]. An elevated permeability composite scaffold made of alginate and collagen, and hydroxyapatite, solid free from fabricated polycaprolactone, bone morphogenetic protein peptide was employed to rebuild bone tissue without the usage of a chemical cross-linking agent [26].
Silk fibroin (SF) is a fibrous protein and a natural biomaterial is a viable material for a biological and medicinal manipulation due to its outstanding bioactivity, harmless, diversified physical properties and cell attachment and proliferation capabilities. Silk, an organic polypeptide with excellent biological features, can be used as a scaffold for biomedical applications, either alone or in conjunction with other materials. Because SF films and fibers have a high potential to facilitate cell adhesion and proliferation, they are used as bone substitutes [27],[28]. Magnetic scaffolds based on SF/CS revealed ideal features, including saturation magnetization behaviour and reduced phosphate-buffered saline (PBS) absorption as well as degradation, and may thus be employed for creating implantable bone substitute [29].
Silk when mixed or based on hexafluoroisopropanol (HFIP) degrades more slowly and promotes pulp tissue development based on water. Tooth stem cells sown on HFIP silk scaffolds, formed mineralized tissue, showing that these scaffolds are beneficial in the creation of osteodentine [30].
They area nonsulfated GAG found in connective tissue's extracellular matrix acts as biological template for tissue regeneration and also have advantages like biocompatibility, structural durability, proliferation, anti-inflammatory capacity [31] and interact with CD4 receptors to promote wound healing and chondrogenesis.
“CS, an untwisted polymer and polysaccharide produced by deacetylation which is incomplete of chitin is found in the exoskeletons of crustaceans”. A little amount of emotion is evoked by CS. Fibrous encapsulate and a reaction to a foreign body. CS is particularly appealing as scaffold material as it encourages adherence and growth of osteoblast cells including the development of bone marrow [32]. Pure CS is a second hand for the proliferation of osteoblast, osteogenic cells, mesenchymal stem cells, matrix formation and mineralization, osteoconductivity. Additionally, it may be mixed with various substances, including polymers and ceramics, to create composite scaffolds with better mechanical and biological characteristics. Because various polymers and bioactive substances may be combined with CS matrices to produce materials with superior physicochemical and biological characteristics [33]. As these composites have specific physical, biochemical, and tensile qualities helpful for bone substitute, CS coupled with bioceramics play a major role in bone repair. There is improved mechanical strength when CS is combined with nanohydroxyapatite [34],[35].
Cellulose is a renewable, biodegradable, ubiquitous and natural bio-based polysaccaride polymer that is created by plants (including bamboo, wood, bast and cotton), algae, tunicates and bacteria [11],[33]. It has a total biomass production of 1.5 × 1012 tonnes annually. Due to their non-toxicity and eco-friendliness, they are employed as sustainable materials in numerous new disciplines like biomedicine, medication transport, motor vehicle, electronic devices and structural engineering sectors. Cellulose keeps up the cell shape in contrast, a secondary component called hemicellulose serves as a medium for connecting cellulose and lignin [36].
Cellulose-based natural fibers reinforced composites (NFRCs) also known as “green composites”—are made by incorporating different NFs with biodegradable resins along with starch as a polymer matrix to enhance the mechanical properties of the green composites. They are used in hand lay-up, extrusion, resin transfer moulding, and injection moulding techniques, along with compression molding. However, there are several significant disadvantages, including a higher absorption of moisture, lower mechanical qualities, incompatibility with the matrix, poor wetting characteristics, as well as low resistance to heat which influences their mechanical properties [36].
Nanocellulose is produced when bacteria, plant cell walls, or cotton liners are mechanically, enzymatically, or chemically separated from the nanoscaled cellulose fibrils and nanocrystals that result. They are classified into Cellulose nanocrystal (CNC), Nano fibrillated Cellulose (NFC) and Bacterial nanocellulose (BNC). High elastic modulus (110–220 GPa), tensile strength (7.5–7.7 GPa), large specific surface area, ease of surface functionalization, adjustable crystallinity, high degree of polymerization, along with excellent chemical resistance are nano cellulose exceptional qualities [36].
In the second group, bio-based monomers are used to create synthetic polymers utilising various ring-opening polymerization or condensation techniques Polyethyleneglycol (PEG), Polycaprolactone (PCL), Polyglycolic Acid (PGA), Hydroxyapatite (HA), Tricalcium Phosphate and Hydroxyapatite Biphasic Ceramics (Biphasic Calcium Phosphate) polylactic acid (PLA), are some of these polymers [37].
PEG oligomers are made of ethylene oxide. PEG are synthetic and offers the advantages of nontoxicity, non immune, resistance to protein absorption, cell adhesion proteolytic degradation, a second hand for growth factor binding, biocompatibility, low immunogenicity and in vivo degradation. Due to its resistance to epithelial and peptide adsorption, it is less likely to be recognized by the immune response and rejected and is also used in medical applications, surface modification, drug delivery and bioconjugation [38].
A member of the poly (-hydroxyl esters) family, poly-e-caprolactone (PCL) is a semicrystalline material with good mechanical qualities. Its nearly new which is handed down as biodegradable polyester for medical implementation in view of the fact of its biocompatibility and biodegradability, slower degradation kinematics, resistant to hydrolysis, proliferation and differentiation status of encapsulated cells, maintain structural integrity and the flexibility [39].
A cyclic glycolide is used as the starting material for the ring-opening polymerization process that produces polyglycolic acid (PGA), a hard, thermoplastic aliphatic polyester. Because lactic and glycolic acids, the breakdown products of PGA, are biological compounds, PGA is favored in medicinal applications. Although PGA possesses excellent characteristics, its biological uses have been constrained by its slow disintegration, low solubility in organic solvents [40].
The physical morphology, degree of crystallinity and pore of natural and synthetic HA are different, both types of HA share chemical properties with bone that contribute to their osteoconductive properties. Sr-HA, Mg-HA and Si-HA resorbs slowly, have improved mechanical and biological properties for bone repair and augmentation along with coating of implants, and also have improved sinterability and enhances densification [41].
The more stable phase HA as well as the more soluble phase TCP are optimally balanced in the biphasic calcium phosphate (BCP) idea. Best bone conductive characteristics appeared to be found in BCP compounds with around 60 percent of HA and 40 percent of TCP. However, more recently, the osteoconductive effects of BCP products with greater -TCP ratios (BCP 60/40 and BCP 20/80) have been investigated [42]. The advantages of BCP is osteogenic property, biological stability, alternative to autograft, bioactive concept, direct osseointegration and superior property over either of the components. Figure 6 demonstrates the flowchart showing the process of bone regeneration.
In dentistry and orthopaedics, titanium is frequently used. “There are 4 grades of commercially pure titanium (cpTi) (Grades 1-4) and two grades of titanium (Ti) alloys (Grades V and VI)—Ti6-Al-4V and Ti-6Al-4V-extra low interstitial alloys”—contigent on the different concentrations of O2, N2, H2, Fe and C that affect the material's physical and mechanical properties [43]. The most used cpTi grades for making metal-ceramic fixed dental prostheses are graded I and II. Ti-6Al-4V alloy is employed in situations where strong mechanical properties are enforced, such as in bone screws and plates [43].
The titanium dental implant is anchored in the bone by osseointegration, a direct structural and functional link. Implant-tissue interaction and osseointegration are influenced by surface chemistry and topography and also inveigled by wettability, plastic strain, the existence of particulates, the thickness of the titanium oxide layer, the presence of metal as well as nonmetal composites [44].
The mechanical characteristics of the titanium foam are similar to those of human cancellous bone at low relative solidity of around 0.20–0.30 [45].
In the beginning, this material, “which is tooth-colored, was created as endosseous implants for bone repair to largely substitute the diminished aesthetic effects of titanium's dense grey color, which are visible through the peri-implant mucosa”. Unfavorable soft tissue issues include anterior maxillary and mandibular incisor replacements, gum disease and thin mucosal biotype. Yttrium oxide or a little quantity of aluminum oxide can be added to zirconia dioxide to boost its hardness [46].
Currently, zirconia is the popular tooth color and the esthetic restorative material, while Polyetherethylketone (PEEK) is utilized as a zirconium substitute in dentistry. Due to the three times greater antagonist wear than PEEK, the failure rates of zirconia were generally higher. nevertheless, zirconia offered the least displacement and had the most higher color stability compared to PEEK crowns. The PEEK crowns were a good substitute for zirconia in crown building because to its minimal abrasion, superior stress modulation through plastic deformation, and high colour stability [47].
Modern materials known as high entropy alloys (HEAs) have exceptional mechanical, physical and chemical properties that are unsurpassed by those of traditional alloys. Configurational entropy Sconf > 1.5R and at least four elements are required for HEAs. These alloys are made up of four or more elements in equiatomic or non-equiatomic fractions, ranging from 5 to 35 for each element, and include cobalt-chromium alloys, copper alloys, aluminium alloys, Nickel alloys and Titanium alloys. Additionally included in HEAs are alkaline soil elements, transition elements, basic elements, refractory elements, nonmetals and metalloids. The three primary types of HEAs are ceramic-based, refractory metal-based, as well as 3d-transition metal-based. Laser welding and LC technology are the ancestors of Laser-aided additive manufacturing (LAAM) technologies. Without using any part-specific tools that result in non-equilibrium microstructures and aberrant mechanical characteristics, these procedures build complex prototypes incrementally layer by layer [48].
Solid mixing, liquid mixing and gas mixing are the three main passages that make up HEAs production processes. The liquid mixing method, which includes arc melting, inductive melting, laser cladding (LC), laser surface alloying, laser surface remelting and thermal spraying, is the main method for creating HEAs [49].
Due to its high density of energy, rapid consolidation, little thermal effect on the substrate, low dissolution, improved metallurgical bonding, minimal clad geometry distortion, low number of crack openings, flexibility when used in fully automated mode, along with potential to create claddings alongside nonequilibrium microstructures or better surface qualities like corrosion, oxidation, as well as etching, modern, multidisciplinary, non-linear technology referred to as laser cladding (LC) is used to alter surfaces. Three categories of LC-HEA coating exist: composite coating, ceramic coating, as well as mettalic coating. Laser-cladded high-entropy alloy coatings contain transitional elements such Al, Cr, Co, Mn, Cu, Ni, Fe andand Ti. In LC-HEA ceramic-based coatings, HEA materials are mixed with oxygen, boron, or other negatively-charged elements in order to offer ionic or covalent bonding. These claddings comprise elements having a high affinity for borides, carbides, or nitrides (Al, Cr, Ti, Nb and Zr). Pharmaceutical delivery, oral implants and cardiovascular implants among LC HEAs' medical uses [50].
Knowing that the host commonly rejects inert metal and plastic materials used in amputation cases, Larry L. Hench set out to find a graft material that was compatible wit.h the human body. This material was found to be a glass that precipitated hydroxyapatite in aqueous solutions and could adhere to both hard and soft tissues without being rejected. Originally, BAG was composed 45% SiO2, 24.5% Na2O, 24.5% CaO and 6% P2O5. Class A BAGs are mainly comprised of 40–52% SiO2, 10–50% CaO and 10–35% Na2O. Most class B glasses have a silica concentration of greater than 60% by weight, making them bioinert. Since they are optically transparent, amorphous solids with uneven atom structures and brittle materials, such as glass, are also known as supercooled liquids [51].
Application of bioactive glasses are in Implant dentistry, In maxillofacial surgery, Dental Adhesives, In Periodontics, Bone regeneration, Enamel mineralization, Dentin Hypersensitivity, Bone regeneration, Pulp capping, RCT, and Restorative Materials, etc [51]. There are some other bioactive resorative materials like Minereal Trioxide Aggregate and Biodentine some of the properties of these biomaterials are shown in Figure 7 and some applications of Minereal Trioxide aggregate are shown in Figure 8.
The most well-known members of the polyaryletherketone (PAEK) family are polyetheretherketone (PEEK) and PEKK. Methacrylate-free thermoplastic high-performance materials belong to the PEEK family. The physical, mechanical and chemical properties of the PEKK materials are suited for various applications, specially in the biomedical field. In dentistry, they are employed as a prosthetic material, an implant biomaterial and as abutments, for the creation of removable partial prostheses and for the attachments, endodontic post and core, endo crowns, etc.
Although PEKK is frequently used in prosthodontics and oral implantology, long-term monitoring is required because the PEKK framework has not yet shown long-term results [52].
Graphene, a promising biocompatible Scaffold, is a single or can be few layers of sp2-hybridized carbon atoms, and is a 3D porous scaffold that was initially separated from graphite. Surface functionalization, concentration, size, along with shape are some of the variables that affect the cytotoxicity as well as the biocompatibility of materials belonging to graphene family. Due to its increased safety for biomedical applications, the graphene family of materials on the nanoscale were thus presented. Graphene can open up a large surface area in the substrate for cell spreading and even osteogenic differentiation. For example, 3D graphene foams applied as hMSC growth substrates showed they could keep stem cells viable and encourage osteogenic advancement [53].
Calcium Phosphate-Based Materials and the Graphene Family were used in orthopedic or maxillofacial surgery, it has long been employed as synthetic bone grafts to fill in regions where there are bone defects. Graphene along with chitosan as well as collagen were used as scaffolds in BTR. Graphene is employed in guided bone membrane and the drug delivery system [53]. Table 9. Shows various biomaterials used for tissue engineering and scaffolds used for regeneration.
Orign | Advantages | Disadvantages | Polymers | Applications |
Natural polymers | Biocompatible, biodegradable, improved cellular interaction, hydrophilicity, antibacterial effect | Lack of bioactivity, rapid degradation rate, low mechanical strength | Collagen, Fibrin, Silk, Chitosan, Alignate and Hyaluronan, Cellulose | Skin, Cartilage, Vessels, Heart, etc. Tissue scaffold, Drugs Delivery, etc. |
Synthetic polymers | Mechanical strength can be processed, biocompatibility, favorable barrier function | Slow degration rate. Hydrophobic, low cell affinity, poor cellular response, Acidic byproduct | Polyethylenegycol, Poly-E-Caprolactone, Polyglycolic acid, Hydroxyapatite, Tricalcium phosphate | Skin, Cartilage, Tendon, Bladder, Liver tissue scaffold, Drug delivery, etc |
Metals | Biocompatible, High barrier, dense, too strong, Ductile | May corrode, surgical removal required, expensive | Titanium, Zirconia, Stainless steel | Dental restoration, load bearing bone, implants, etc. |
Composite | Biocompatible, biodegradable, good in ceel adhesion | Low density, high cost, shape cannot be changed easily | PCL/gelatin, PCL/chitosan, PCL/gelatin/chitosan, Collagen/chitosan | Cartilage, Skin, Nerve, Bone, Blood vessel, tissue scaffold and Drug delivery, etc. |
Despite significant improvements in tissue engineering and regenerative medicine (RM) approaches, there are still many difficulties that need to be overcome before successful translation into widespread clinical applications can be accomplished. The successful vascularization of TE constructions continues to be a significant limiting factor for TE of huge volumes or complete organs, even if many potential tissue engineering procedures are built on a foundation of diverse cell types, growth hormones and scaffolds. The successful implementation of future vascularization technologies will determine whether TE principles can be applied in clinical settings because sufficient perfusion is a key determinant for the development along with the host integration of TE constructions [54].
The oncologic safety of the materials utilized in TE procedures, like GFs, cell types andand scaffolds, needs to be thoroughly examined in subsequent studies as yet another constraint. Another restriction of TE/RM is the inability to recreate tissues or organs functionally. Since skin substitutes are devoid of dermal appendages like sebaceous glands, hair follicles and neurovascular systems, they are unable to replicate fully functional skin layers. To convert functional TE applications, significant improvement in all TE fields is still required [54].
The conclusions that can be derived from this literature are that there are just a few minor variances in study technique with regard to cell types, various scaffolds and defect features. To find cell types, scaffolds and growth factors that are very successful for particular therapeutic settings, future comparison studies that are more uniformed are required [54].
Since 4D printing is still a young technology, there are a number of issues that must be resolved before it can be extensively used. The requirement to create novel materials and methods that can be applied to the 4D printing process is one of the main obstacles. The ethical and legal ramifications of 4D printing also raise a number of unresolved difficulties, including considerations of intellectual property and liability [55].
The 3D printing of biomaterials and nanotechnology are recent technological developments which are particularly beneficial for craniofacial reconstruction [54]. The benefits of 3D printing include the ability to control the internal or external 3D architecture of scaffold systems, the ease with which scaffolds can be created to precisely match patient compliance as well as requirements, being able to make scaffolds out of a wide range of substances, and the capability to predefine scaffold architecture in order to control cell behaviour and mechanical response [56].The more well-known 3D printing modalities for TE applications are generally regarded as extrusion printing, inkjet printing, laser printing and to a lesser extent lithography printing (which has similarities to the laser 3D printing modality). Compared to other printers, extrusion 3D printers/bioprinters are the most useful for research on bone replacement since they quickly allow the building of larger-scale structures required for clinically meaningful tissue topologies. Depending on the materials and hardware features, extrusion 3D printers can also be set up to discharge various substances with proven osteoinductive capacities, such as CaP injectable pastes, ceramic basesand hydrogels [56].
Hi-tech A cutting-edge technique called 4D bioprinting produces customised pieces of complex products by processing digital medical images and demonstrates exceptional automation control. The overall objective of 4D printing is to produce products that are more adaptable, efficient and versatile than their 3D printed counterparts and that may be able to solve a variety of challenging issues across numerous industries [55]. By building and developing 3D cell-laden dynamical structures, this technology is advancing and opening up new research directions in the biomedical sectors. The future of the biomedical sector is smart polymers and 4D bioprinting technologies. Shape memory, self-assembly, self-actuating, self-sensing and self-healing are five examples of smart polymers that can be found in 4D printed objects. 4D printing technology depicts different aspect of smart materials like shape memory polymers (SMPs), shape memory alloys (SMAs), shape memory composites, hydrogels, liquid crystals, Magnetorheological fluids, photoresponsive materials, thermoplastic elastomers, Thermochromic materials, piezoelectric materials and self healing polymers [57],[58].
SMAs are one of the smart materials used in 4D printing the most frequently. SMAs are a family of intelligent materials that may change shape when heated or cooled and can return to its original shape when the temperature changes again. These alloys are made of a combination of metals that have the unique capacity to retain their shape, including copper, zinc, aluminium, nickel, titanium, and more [55].
Shape-changing smart materials, SMPs, are capable of altering their structure in response to environmental factors like pressure, temperature, light and pH. The shape memory effect, which is a property of these polymers, allows for the restoration of their original shape following deformation [55].
4D printing also employs materials that exploit piezoelectricity. Piezoelectric materials are a class of smart materials that can generate an electrical current in response to mechanical stress and may also alter shape in response to an electrical current. These substances, like quartz or barium titanate, are made of crystals or ceramics with particular crystal structures [55].
A class of smart material known as a hydrogel is made up of a web of polymer chains that have a high-water absorption and retention capacity. PEG, PVA, as well as alginate are types of the natural and synthetic polymers that can be used to create these materials. Depending on their structure and content, hydrogels can have a wide variety of mechanical qualities, from soft and flexible to stiff and brittle [55].
These smart materals are also used in biomedical products such as cardiac patches, stents, orthopaedic implants, cardiovascular implants, cardiac TE, cartilage TE, neural TE, actuators, skin dressings, biosensors, scaffolds, as well as splints. The potential application of 4D printing in tissue engineering is for vascular, nerve and cartilage repairing and reconstruction as well as for various soft and hard tissue regeneration and their applications in dentistry is for smart orthodontics, dental scaffolds and smart dental implants [57].
The future research directions that need to be pursued are as follows: The development of innovative multi-stimuli materials, the need for empirical modelling, the enhancement of the biocompatibility, interlinking of the vascular tissues, and the commercialization of 4D bioprinting technology are all factors [14].
The fields of molecular biology, polymer chemistry, molecular genetics, along with materials science, robotics, and mechanical engineering will work together in an interdisciplinary manner to make further advancements [54].
By using 3D constructions that restore both shape and function, TE has introduced new discoveries with innovative therapies that entail the regeneration and replacement of tissues and organs. Utilising the patient's own cells, biomaterials, biomolecules and exogenous structures makes this possible.
According to Thrivikraman et al., biomaterials which are employed as biocompatible scaffolds which permit the migration along with proliferation, and the differentiation of both internal or exterior cells are used for the production of new bone tissue [56]. According to Mijiritsky et al., biomaterials are also important for creating a 3D substrate on which cells can multiply, which needs necessary growth factors (GF) for each type of cell [59].
Building a dental scaffold is challenging, say Shakya et al., because it must fit into a 3D anatomical defect and remain temporarily load-free until the anticipated bone neoformation takes place [60].
Most often, the best scaffold is developed by amalgamating various biomaterials. The goal is to seek the combination of all its essential features, or as an alternative, it is enough to choose one of them [56],[60].
Since the introduction of the idea of tissue engineering, cranial and facial rehabilitation has advanced significantly. Numerous substances have indeed been investigated as frameworks to encourage the renewal of maxillofacial tissue, including metals and ceramics, natural and synthetic polymers and even their mixtures. Medical science, the creation of fresh foundations, manufacturing methods and evaluation methodologies are just a few of the directions this discipline is moving. It is difficult to regenerate orofacial components since it is necessary to recreate the physiological genesis of numerous regions and interactions. The discovery of polymers that support quick self-renewal, the establishment of optimizing the process parameters that yield templates with specific architectural designs, and indeed the transport of medicinal compounds like antibiotics and growth hormones should all be the reference for future study.
[1] |
KTevlin R, McArdle A, Atashroo D, et al. (2014) Biomaterials for craniofacial bone engineering. J Dent Res 93: 1187-1195. https://doi.org/10.1177/0022034514547271 ![]() |
[2] |
Chan BP, Leong KW (2008) Scaffolding in tissue engineering: general approaches and tissue-specific considerations. Eur Spine J 17: 467-479. https://doi.org/10.1007/s00586-008-0745-3 ![]() |
[3] |
Arif ZU, Khalid MY, Noroozi R, et al. (2022) Recent advances in 3D-printed polylactide and polycaprolactone-based biomaterials for tissue engineering applications. Int J Biol Macromo 218: 930-968. https://doi.org/10.1016/j.ijbiomac.2022.07.140 ![]() |
[4] |
Hsu EL, Ghodasra JH, Ashtekar A, et al. (2013) A comparative evaluation of factors influencing osteoinductivity among scaffolds designed for bone regeneration. Tissue Eng Part A 19: 1764-1772. https://doi.org/10.1089/ten.tea.2012.0711 ![]() |
[5] |
Keane TJ, Badylak SF (2014) Biomaterials for tissue engineering applications. Semin Pediatr Surg 23: 112-118. https://doi.org/10.1053/j.sempedsurg.2014.06.010 ![]() |
[6] |
Jafari M, Paknejad Z, Rad MR, et al. (2017) Polymeric scaffolds in tissue engineering: a literature review. J Biomed Mater Res Part B: Appl Biomater 105: 431-459. https://doi.org/10.1002/jbm.b.33547 ![]() |
[7] |
Schulte M, Schultheiss M, Hartwig E, et al. (2000) Vertebral body replacement with a bioglass-polyurethane composite in spine metastases–clinical, radiological and biomechanical results. Eur Spine J 9: 437-444. https://doi.org/10.1007/s005860000162 ![]() |
[8] |
Elisseeff J, Puleo C, Yang F, et al. (2005) Advances in skeletal tissue engineering with hydrogels. Orthod Craniofac Res 8: 150-161. https://doi.org/10.1111/j.1601-6343.2005.00335.x ![]() |
[9] |
Arvidson K, Abdallah BM, Applegate LA, et al. (2011) Bone regeneration and stem cells. J Cell Mol Med 15: 718-746. https://doi.org/10.1111/j.1582-4934.2010. 01224.x ![]() |
[10] |
Moshiri A, Oryan A (2012) Role of tissue engineering in tendon reconstructive surgery and regenerative medicine: current concepts, approaches and concerns. Hard Tissue 1: 11. https://doi.org/10.13172/2050-2303-1-2-291 ![]() |
[11] |
Khalid MY, Arif ZU (2022) Novel biopolymer-based sustainable composites for food packaging applications: a narrative review. Food Packag Shelf Life 33: 100892. https://doi.org/10.1016/j.fpsl.2022.100892 ![]() |
[12] |
Khalid MY, Al Rashid A, Arif ZU, et al. (2021) Natural fiber reinforced composites: Sustainable materials for emerging applications. Results Eng 11: 100263. https://doi.org/10.1016/j.rineng.2021.100263 ![]() |
[13] |
Arif ZU, Khalid MY, Sheikh MF, et al. (2022) Biopolymeric sustainable materials and their emerging applications. J Environ Chem Eng 10: 108159. https://doi.org/10.1016/j.jece.2022.108159 ![]() |
[14] |
Arif ZU, Khalid MY, Noroozi R, et al. (2023) Additive manufacturing of sustainable biomaterials for biomedical applications. Asian J Pharm Sci 2023: 100812. https://doi.org/10.1016/j.ajps.2023.100812 ![]() |
[15] |
Kang BJ, Kim Y, Lee SH, et al. (2013) Collagen I gel promotes homogenous osteogenic differentiation of adipose tissue-derived mesenchymal stem cells in serum-derived albumin scaffold. J Biomater Sci Polym Ed 24: 1233-1243. https://doi.org/10.1080/09205063.2012.745717 ![]() |
[16] |
Ferreira AM, Gentile P, Chiono V, et al. (2012) Collagen for bone tissue engineering. Acta Biomater 8: 3191-3200. https://doi.org/10.1016/j.actbio.2012.06.014 ![]() |
[17] |
Kadler K (2004) Matrix loading: assembly of extracellular matrix collagen fibrils during embryogenesis. Birth Defects Res C 72: 1-11. https://doi.org/10.1002/bdrc.20002 ![]() |
[18] |
Quinlan E, Thompson EM, Matsiko A, et al. (2015) Functionalization of a collagen-hydroxyapatite scaffold with osteostatin to facilitate enhanced bone regeneration. Adv Healthcare Mater 4: 2649-2656. https://doi.org/10.1002/adhm.201500439 ![]() |
[19] |
Zhou C, Ye X, Fan Y, et al. (2014) Biomimetic fabrication of a three-level hierarchical calcium phosphate/collagen/hydroxyapatite scaffold for bone tissue engineering. Biofabrication 6: 035013. https://doi.org/10.1088/1758-5082/6/3/035013 ![]() |
[20] |
Noori A, Ashrafi SJ, Vaez-Ghaemi R, et al. (2017) A review of fibrin and fibrin composites for bone tissue engineering. Int J Nanomed 12: 4937. https://doi.org/10.2147/IJN.S124671 ![]() |
[21] | Siddiqui N, Pramanik K (2015) Development of fibrin conjugated chitosan/nano β-TCP composite scaffolds with improved cell supportive property for bone tissue regeneration. J Appl Polym Sci 132: 41534. https://doi.org/10.1002/app.41534 |
[22] |
Kim BS, Sung HM, You HK, et al. (2014) Effects of fibrinogen concentration on fibrin glue and bone powder scaffolds in bone regeneration. J Biosci Bioeng 118: 469-475. https://doi.org/10.1016/j.jbiosc.2014.03.014 ![]() |
[23] | Wong M (2004) Alginates in tissue engineering. Biopolymer Methods in Tissue Engineering. Methods in Molecular Biology™. Switzerland: Humana Press 77-86. https://doi.org/10.1385/1-59259-428-X:77 |
[24] |
Venkatesan J, Bhatnagar I, Kim SK (2014) Chitosan-alginate biocomposite containing fucoidan for bone tissue engineering. Marine Drugs 12: 300-316. https://doi.org/10.3390/md12010300 ![]() |
[25] |
Valente JFA, Valente TAM, Alves P, et al. (2012) Alginate based scaffolds for bone tissue engineering. Mater Sci Engi C 32: 2596-2603. 10.1016/j.msec.2012.08.001 ![]() |
[26] |
Kim M, Jung WK, Kim G (2013) Bio-composites composed of a solid free-form fabricated polycaprolactone and alginate-releasing bone morphogenic protein and bone formation peptide for bone tissue regeneration. Bioprocess Biosyst Eng 36: 1725-1734. https://doi.org/10.1007/s00449-013-0947-x ![]() |
[27] |
Pina S, Rebelo R, Correlo VM, et al. (2018) Bioceramics for osteochondral tissue engineering and regeneration. Osteochondral Tissue Engineering. Advances in Experimental Medicine and Biology. Cham: Springer 53-75. https://doi.org/10.1007/978-3-319-76711-6_3 ![]() |
[28] |
Li DW, He J, He FL, et al. (2018) Silk fibroin/chitosan thin film promotes osteogenic and adipogenic differentiation of rat bone marrow-derived mesenchymal stem cells. J Biomater Appl 32: 1164-1173. https://doi.org/10.1177/0885328218757767 ![]() |
[29] |
Aliramaji S, Zamanian A, Mozafari M (2017) Super-paramagnetic responsive silk fibroin/chitosan/magnetite scaffolds with tunable pore structures for bone tissue engineering applications. Mater Sci Eng C 70: 736-744. https://doi.org/10.1016/j.msec.2016.09.039 ![]() |
[30] |
Zhang W, Ahluwalia IP, Literman R, et al. (2011) Human dental pulp progenitor cell behavior on aqueous and hexafluoroisopropanol based silk scaffolds. J Biomed Mater Res A 97: 414-422. https://doi.org/10.1002/jbm.a.33062 ![]() |
[31] |
Collins MN, Birkinshaw C (2013) Hyaluronic acid-based scaffolds for tissue engineering—a review. Carbohydr Polym 92: 1262-1279. https://doi.org/10.1016/j.carbpol.2012.10.028 ![]() |
[32] |
Seol YJ, Lee JY, Park YJ, et al. (2004) Chitosan sponges as tissue engineering scaffolds for bone formation. Biotechnol Lett 26: 1037-1041. https://doi.org/10.1023/B:BILE.0000032962.79531.fd ![]() |
[33] |
Balagangadharan K, Dhivya S, Selvamurugan N (2017) Chitosan based nanofibers in bone tissue engineering. Int J Biol Macromol 104: 1372-1382. https://doi.org/10.1016/j.ijbiomac.2016.12.046 ![]() |
[34] |
Shalumon KT, Sowmya S, Sathish D, et al. (2013) Effect of incorporation of nanoscale bioactive glass and hydroxyapatite in PCL/chitosan nanofibers for bone and periodontal tissue engineering. J Biomed Nanotechnol 9: 430-440. https://doi.org/10.1166/jbn.2013.1559 ![]() |
[35] |
Wang F, Zhang YC, Zhou H, et al. (2014) Evaluation of in vitro and in vivo osteogenic differentiation of nano-hydroxyapatite/chitosan/poly (lactide-co-glycolide) scaffolds with human umbilical cord mesenchymal stem cells. J Biomed Mater Res A 102: 760-768. https://doi.org/10.1002/jbm.a.34747 ![]() |
[36] |
Khalid MY, Al Rashid A, Arif ZU, et al. (2021) Recent advances in nanocellulose-based different biomaterials: types, properties, and emerging applications. J Mater Res Technol 14: 2601-2623. https://doi.org/10.1016/j.jmrt.2021.07.128 ![]() |
[37] |
Arif ZU, Khalid MY, Sheikh MF, et al. (2022) Biopolymeric sustainable materials and their emerging applications. J Environ Chem Eng 10: 108159. https://doi.org/10.1016/j.jece.2022.108159 ![]() |
[38] |
Burdick JA, Anseth KS (2002) Photoencapsulation of osteoblasts in injectable RGD-modified PEG hydrogels for bone tissue engineering. Biomaterials 23: 4315-4323. https://doi.org/10.1016/s0142-9612(02)00176-x ![]() |
[39] |
Lin WJ, Flanagan DR, Linhardt RJ (1999) A novel fabrication of poly (ϵ-caprolactone) microspheres from blends of poly (ϵ-caprolactone) and poly (ethylene glycol) s. Polymer 40: 1731-1735. https://doi.org/10.1016/S0032-3861(98)00378-4 ![]() |
[40] |
Nair LS, Laurencin CT (2007) Biodegradable polymers as biomaterials. Prog Polym Sci 32: 762-798. https://doi.org/10.1016/j.progpolymsci.2007.05.017 ![]() |
[41] |
Ramesh N, Moratti SC, Dias GJ (2018) Hydroxyapatite–polymer biocomposites for bone regeneration: A review of current trends. J Biomed Mater Res Part B 106: 2046-2057. https://doi.org/10.1002/jbm.b.33950 ![]() |
[42] |
Yang C, Unursaikhan O, Lee JS, et al. (2014) Osteoconductivity and biodegradation of synthetic bone substitutes with different tricalcium phosphate contents in rabbits. J Biomed Mater Res Part B 102: 80-88. https://doi.org/10.1002/jbm.b.32984 ![]() |
[43] |
Özcan M, Hämmerle C (2012) Titanium as a reconstruction and implant material in dentistry: advantages and pitfalls. Materials 5: 1528-1545. https://doi.org/10.3390/ma5091528 ![]() |
[44] | Anil S, Anand PS, Alghamdi H, et al. (2011) Dental implant surface enhancement and osseointegration. Implant Dentistry—A Rapidly Evolving Practice. Europe: InTech 83-108. https://doi.org/10.5772/16475 |
[45] |
Wen CE, Yamada Y, Shimojima K, et al. (2002) Novel titanium foam for bone tissue engineering. J Mater Res 17: 2633-2639. https://doi.org/10.1557/JMR.2002.0382 ![]() |
[46] |
Özkurt Z, Kazazoğlu E (2011) Zirconia dental implants: a literature review. J Oral Implantol 37: 367-376. https://doi.org/10.1563/AAID-JOI-D-09-00079 ![]() |
[47] |
Abhay SS, Ganapathy D, Veeraiyan DN, et al. (2021) Wear resistance, color stability and displacement resistance of milled PEEK crowns compared to zirconia crowns under stimulated chewing and high-performance aging. Polymers 13: 3761. https://doi.org/10.3390/polym13213761 ![]() |
[48] |
Arif ZU, Khalid MY, ur Rehman E (2022) Laser-aided additive manufacturing of high entropy alloys: processes, properties, and emerging applications. J Manuf Process 78: 131-171. https://doi.org/10.1016/j.jmapro.2022.04.014 ![]() |
[49] |
Arif ZU, Khalid MY, Al Rashid A, et al. (2022) Laser deposition of high-entropy alloys: a comprehensive review. Opt Laser Technol 145: 107447. https://doi.org/10.1016/j.optlastec.2021.107447 ![]() |
[50] |
Arif ZU, Khalid MY, ur Rehman E, et al. (2021) A review on laser cladding of high-entropy alloys, their recent trends and potential applications. J Manuf Process 68: 225-273. https://doi.org/10.1016/j.jmapro.2021.06.041 ![]() |
[51] |
Skallevold HE, Rokaya D, Khurshid Z, et al. (2019) Bioactive glass applications in dentistry. Int J Mol Sci 20: 5960. https://doi.org/10.3390/ijms20235960 ![]() |
[52] |
Alqurashi H, Khurshid Z, Syed AUY, et al. (2021) Polyetherketoneketone (PEKK): An emerging biomaterial for oral implants and dental prostheses. J Adv Res 28: 87-95. https://doi.org/10.1016/j.jare.2020.09.004 ![]() |
[53] |
Cheng X, Wan Q, Pei X (2018) Graphene family materials in bone tissue regeneration: perspectives and challenges. Nanoscale Res Lett 13: 289. https://doi.org/10.1186/s11671-018-2694-z ![]() |
[54] |
Borrelli MR, Hu MS, Longaker MT, et al. (2020) Tissue engineering and regenerative medicine in craniofacial reconstruction and facial aesthetics. J Craniofac Surg 31: 15-27. https://doi.org/10.1097/SCS.0000000000005840 ![]() |
[55] |
Khalid MY, Arif ZU, Noroozi R, et al. (2022) 4D printing of shape memory polymer composites: A review on fabrication techniques, applications, and future perspectives. J Manuf Process 81: 759-797. https://doi.org/10.1016/j.jmapro.2022.07.035 ![]() |
[56] | Thrivikraman G, Athirasala A, Twohig C, et al. (2017) Biomaterials for craniofacial bone regeneration. Dent Clin 61: 835-856. https://doi.org/10.1016/j.cden.2017.06.003 |
[57] |
Khalid MY, Arif ZU, Ahmed W (2022) 4D printing: technological and manufacturing renaissance. Macromol Mater Eng 307: 2200003. https://doi.org/10.1002/mame.202200003 ![]() |
[58] |
Arif ZU, Khalid M Y, Zolfagharian A, et al. 4D bioprinting of smart polymers for biomedical applications: Recent progress, challenges, and future perspectives. React Funct Polym 179: 105374. https://doi.org/10.1016/j.reactfunctpolym.2022.105374 ![]() |
[59] |
Mijiritsky E, Ferroni L, Gardin C, et al. (2017) Porcine bone scaffolds adsorb growth factors secreted by MSCs and improve bone tissue repair. Materials 10: 1054. https://doi.org/10.3390/ma10091054 ![]() |
[60] |
Shakya AK, Kandalam U (2017) Three-dimensional macroporous materials for tissue engineering of craniofacial bone. Brit J Oral Max Surg 55: 875-891. https://doi.org/10.1016/j.bjoms.2017.09.007 ![]() |
Orign | Advantages | Disadvantages | Polymers | Applications |
Natural polymers | Biocompatible, biodegradable, improved cellular interaction, hydrophilicity, antibacterial effect | Lack of bioactivity, rapid degradation rate, low mechanical strength | Collagen, Fibrin, Silk, Chitosan, Alignate and Hyaluronan, Cellulose | Skin, Cartilage, Vessels, Heart, etc. Tissue scaffold, Drugs Delivery, etc. |
Synthetic polymers | Mechanical strength can be processed, biocompatibility, favorable barrier function | Slow degration rate. Hydrophobic, low cell affinity, poor cellular response, Acidic byproduct | Polyethylenegycol, Poly-E-Caprolactone, Polyglycolic acid, Hydroxyapatite, Tricalcium phosphate | Skin, Cartilage, Tendon, Bladder, Liver tissue scaffold, Drug delivery, etc |
Metals | Biocompatible, High barrier, dense, too strong, Ductile | May corrode, surgical removal required, expensive | Titanium, Zirconia, Stainless steel | Dental restoration, load bearing bone, implants, etc. |
Composite | Biocompatible, biodegradable, good in ceel adhesion | Low density, high cost, shape cannot be changed easily | PCL/gelatin, PCL/chitosan, PCL/gelatin/chitosan, Collagen/chitosan | Cartilage, Skin, Nerve, Bone, Blood vessel, tissue scaffold and Drug delivery, etc. |
Orign | Advantages | Disadvantages | Polymers | Applications |
Natural polymers | Biocompatible, biodegradable, improved cellular interaction, hydrophilicity, antibacterial effect | Lack of bioactivity, rapid degradation rate, low mechanical strength | Collagen, Fibrin, Silk, Chitosan, Alignate and Hyaluronan, Cellulose | Skin, Cartilage, Vessels, Heart, etc. Tissue scaffold, Drugs Delivery, etc. |
Synthetic polymers | Mechanical strength can be processed, biocompatibility, favorable barrier function | Slow degration rate. Hydrophobic, low cell affinity, poor cellular response, Acidic byproduct | Polyethylenegycol, Poly-E-Caprolactone, Polyglycolic acid, Hydroxyapatite, Tricalcium phosphate | Skin, Cartilage, Tendon, Bladder, Liver tissue scaffold, Drug delivery, etc |
Metals | Biocompatible, High barrier, dense, too strong, Ductile | May corrode, surgical removal required, expensive | Titanium, Zirconia, Stainless steel | Dental restoration, load bearing bone, implants, etc. |
Composite | Biocompatible, biodegradable, good in ceel adhesion | Low density, high cost, shape cannot be changed easily | PCL/gelatin, PCL/chitosan, PCL/gelatin/chitosan, Collagen/chitosan | Cartilage, Skin, Nerve, Bone, Blood vessel, tissue scaffold and Drug delivery, etc. |