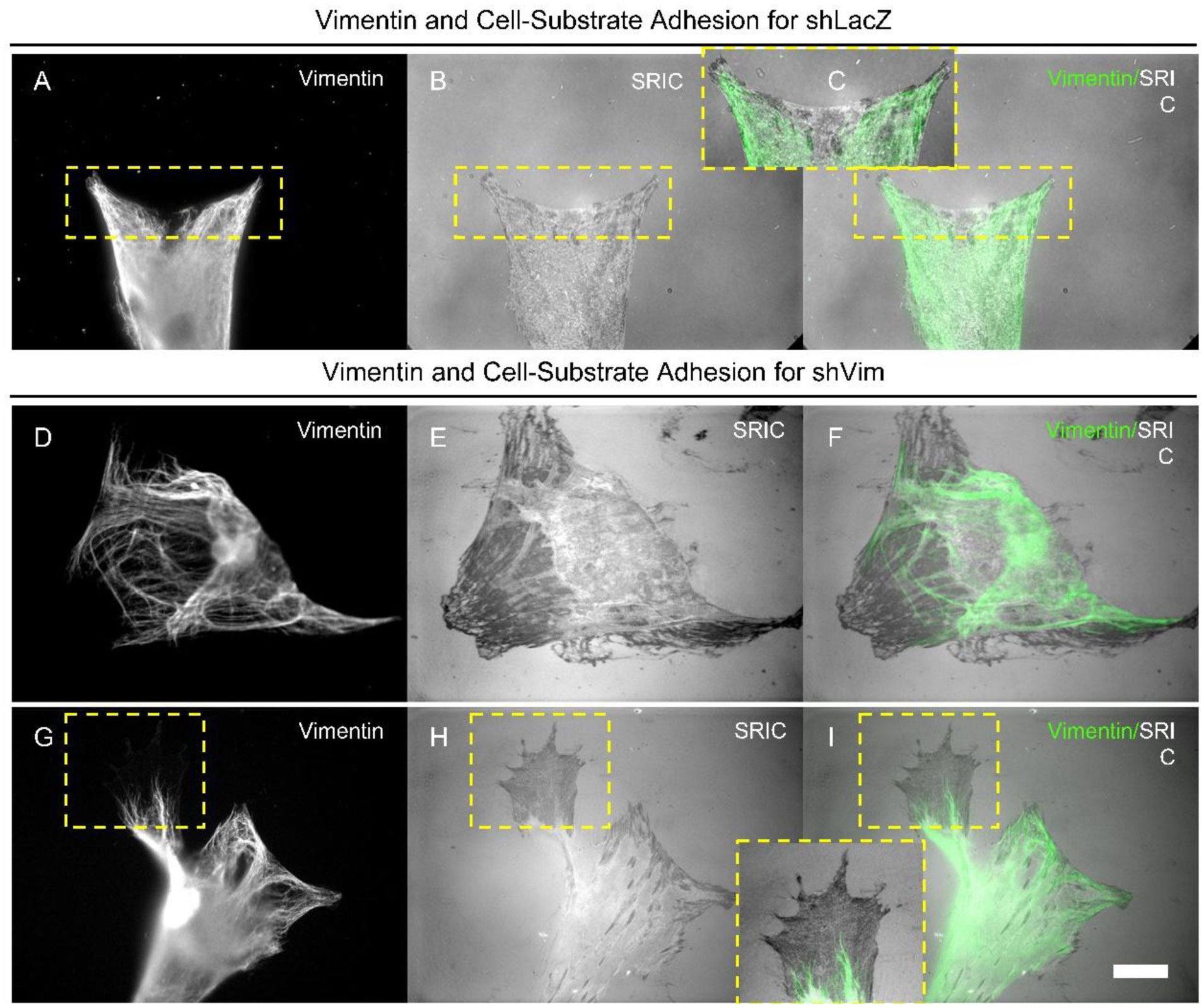
Citation: Poonam Sharma, Shalise Burch, Tejasvi Peesay, Susan M. Hamilla, Adam H. Hsieh, Carlos Luna Lopez. Downregulation of vimentin intermediate filaments affect human mesenchymal stem cell adhesion and formation of cellular projections[J]. AIMS Bioengineering, 2020, 7(4): 272-288. doi: 10.3934/bioeng.2020023
[1] | Daniel Pelaez, John H. Michel, Herman S. Cheung . Growth on elastic silicone substrate elicits a partial myogenic response in periodontal ligament derived stem cells. AIMS Bioengineering, 2016, 3(4): 515-527. doi: 10.3934/bioeng.2016.4.515 |
[2] | Carlos M. Carballosa, Jordan M. Greenberg, Herman S. Cheung . Expression and function of nicotinic acetylcholine receptors in stem cells. AIMS Bioengineering, 2016, 3(3): 245-263. doi: 10.3934/bioeng.2016.3.245 |
[3] | Shaoyung Chen, Hsinyi Mao, Pinhua Tu, Naichen Cheng, Jiashing Yu . Fabrication of decellularized adipose tissue/alginate composite microspheres with pASCs encapsulation for tissue engineering. AIMS Bioengineering, 2017, 4(3): 351-365. doi: 10.3934/bioeng.2017.3.351 |
[4] | Ana Meireles, Ana L. Gonçalves, Inês B. Gomes, Lúcia Chaves Simões, Manuel Simões . Methods to study microbial adhesion on abiotic surfaces. AIMS Bioengineering, 2015, 2(4): 297-309. doi: 10.3934/bioeng.2015.4.297 |
[5] | Mark Weingarten, Nathan Akhavan, Joshua Hanau, Yakov Peter . A Place to Call Home: Bioengineering Pluripotential Stem Cell Cultures. AIMS Bioengineering, 2015, 2(2): 15-28. doi: 10.3934/bioeng.2015.2.15 |
[6] | Daria Wehlage, Hannah Blattner, Al Mamun, Ines Kutzli, Elise Diestelhorst, Anke Rattenholl, Frank Gudermann, Dirk Lütkemeyer, Andrea Ehrmann . Cell growth on electrospun nanofiber mats from polyacrylonitrile (PAN) blends. AIMS Bioengineering, 2020, 7(1): 43-54. doi: 10.3934/bioeng.2020004 |
[7] | Yihan Zhang . Manufacture of complex heart tissues: technological advancements and future directions. AIMS Bioengineering, 2021, 8(1): 73-92. doi: 10.3934/bioeng.2021008 |
[8] | Jan Barekzai, Jonas Friedrich, Maduwuike Okpara, Laura Refflinghaus, Dustin Eckhardt, Peter Czermak, Denise Salzig . Dynamic expansion of mesenchymal stem/stromal cells in a stirred tank bioreactor promotes the release of potent extracellular vesicles. AIMS Bioengineering, 2023, 10(3): 240-264. doi: 10.3934/bioeng.2023016 |
[9] | Sven Sölmann, Anke Rattenholl, Hannah Blattner, Guido Ehrmann, Frank Gudermann, Dirk Lütkemeyer, Andrea Ehrmann . Mammalian cell adhesion on different 3D printed polymers with varying sterilization methods and acidic treatment. AIMS Bioengineering, 2021, 8(1): 25-35. doi: 10.3934/bioeng.2021004 |
[10] | Daniele D’Arrigo, Marta Bottagisio, Silvia Lopa, Matteo Moretti, Arianna B. Lovati . Tissue engineering approaches to develop decellularized tendon matrices functionalized with progenitor cells cultured under undifferentiated and tenogenic conditions. AIMS Bioengineering, 2017, 4(4): 431-445. doi: 10.3934/bioeng.2017.4.431 |
Mesenchymal stem cells (MSCs) have been widely explored for a variety of therapeutic applications, including musculoskeletal disorders such as osteoarthritis [1] and degenerative disc disease [2]. The use of MSCs as a cell source for these conditions, among others, can be attributed to the combined advantages of diverse differentiation potential, immunomodulation properties, paracrine effects, and homing capability [3]. Migration and adhesion of MSCs to a damaged tissue's extracellular matrix are essential for MSCs to carry out their therapeutic function. Their capacity to migrate to diseased or damaged tissues has been investigated in a number of models [4]–[6], and further understanding of their homing potential has come from studies focusing on MSCs' chemotactic response to chemokines or growth factors [4],[6]–[8]. In addition to migration, MSC adhesion and spreading have been found to be particularly important for phenotypic changes. For instance, changes to cell shape [9], size [10], substrate stiffness [11], and composition [12],[13] have been shown to be capable of tuning MSC differentiation.
As with most other cell types, migration and adhesion of MSCs are largely governed by the cytoskeleton, which consists of actin microfilaments, microtubules, and intermediate filaments (IFs), primarily vimentin. The roles of actin and microtubules in migration and adhesion, in concert with a complex collection of supporting proteins, have been well described. Actin microfilaments form structures required for migration at the leading edge of the cell called lamellipodia. The development and stability of these structures is mediated by the formation and separation of focal adhesions or integrin clustering at the leading and trailing edges of the cells [14]. It is thought that microtubules help to polarize cells for migration through extension into the lamellipodia and trafficking of vesicles containing adhesion molecules to the leading edge of the cell [14]. Similarly, actin has also been found to be required for the formation of cellular projections for migration while microtubules are needed for cellular cytoplasmic projection elongation [15]. Comparatively, significantly less is known about the related function of vimentin IFs in these processes.
There is increasing evidence that vimentin IFs are involved in cellular adhesion and spreading. Vimentin deficiency results in slower adhesion by cancer cells [16],[17]. Further, vimentin IFs are known to interact with numerous integrin subunits [18]–[24], and potentially regulate, focal adhesions [16],[19],[25]–[28]. These interactions with adhesion proteins appear to be associated with the activity of cytoskeletal linkers plectin and filamin A [21]–[23],[29]. However, the relationship between cellular adhesion, spreading, and vimentin IFs in MSCs has not been fully explored.
Vimentin IFs also appear to be involved in cellular migration. While changes in vimentin IFs have been found to correlate with changes in the migration of MSCs [30], the mechanisms by which vimentin is involved in migration has been more extensively investigated in cancer cells and fibroblasts. Increased vimentin expression has been designated as a key marker in the epithelial to mesenchymal transition and is associated with increased tumor cell migration, metastasis, and poor prognosis [17],[25],[28],[31]. Down regulation or absence of vimentin in carcinoma cells, fibroblasts, and epithelial has been shown to impair cell migration [17],[26],[28],[32]–[34] and alter the migration of lymphocytes and leukocytes through endothelial cells [35],[36]. Vimentin IFs further appear to be involved in modulating cellular structures involved in migration. Long cellular cytoplasmic projections during migration through matrix pores have been found to require vimentin IFs for elongation [15]. Similarly, pseudopodia of leukocytes interacting with their target endothelial cells were shown to require vimentin reorganization [35]. While it is apparent that vimentin IFs are involved in cellular migration, the mechanism by which they act in MSCs remains to be clarified.
In this study we sought to investigate the role of vimentin IFs in adhesion and protrusion formation in MSCs. Specifically, we examined the relationship among MSC adhesion, cellular projection formation, and vimentin IFs using lentiviral shRNA-mediated RNA interference (RNAi) to determine the effect of decreased vimentin expression on these behaviors. Our results indicate that vimentin IFs are necessary for the cellular structural integrity required for forming cellular protrusions and normal cell-substrate adhesion.
Population doubling level (PDL) 9 bone marrow derived human mesenchymal stem cells (hMSCs) (RoosterBio; Frederick, MD) were expanded using RoosterBio Enriched Basal media supplemented with GTX Booster (RoosterBio) per manufacturer instructions. PDL 13-18 hMSCs were used for all experiments. Subsequent subculture for lentiviral transduction and experimentation was completed using hMSC growth media: high glucose DMEM containing 4 mM L-Glutamine (Gibco) supplemented with 10% fetal bovine serum (FBS) (Gibco), 100 U/ml Penicillin Streptomycin (Gibco), 1% MEM non-essential amino acids (Gibco), and 4mM L-Glutamine (Gibco). Complete media exchange was completed every 2–3 days and the cells were maintained at 5% CO2 and at 37 °C.
shRNA lentivirus was designed and generated as previously described [37]. Briefly, a 52-nucleotide shRNA sense-loop-antisense.
(5′-AAAAGGCAGAAGAATGGTACAAATTGGATCCAATTTGTACCATTCTTCTGCC-3′) sequence was designed and selected from human vimentin [Gen Bank: NM_003380] mRNA using the shRNA Designer through Biosettia, Inc. After annealing, double stranded oligonucleotides were ligated per manufacturer instructions into an inducible lentiviral RNAi vector conveying resistance to blasticidin and containing a TetO-H1 promoter. RNA interference in this inducible system occurs only in the presence of doxycycline. The pLV-RNAi kit and pLV-Pack Packaging mix (Biosettia) were used to generate the shRNA constructs and package into replication-deficient lentivirus using HEK 293FT cells and Lipofectamine 2000. A shRNA lentiviral vector targeting the LacZ gene (5′-GCAGTTATCTGGAAGATCAGGTTGGATCCAACCTGATCTTCCAGATAACTGC-3′) was used as a control (Biosettia). Three days post-transfection, virus-containing supernatants were collected and stored at −80 °C until use.
hMSCs were transduced with the shVim- or shLacZ- lentiviral particles for 24 hrs at a multiplicity of infection (MOI) of 15. The shLacZ-transduced hMSCs served as our non-targeting control for all experiments, as previously described [37]. Transduction was completed in the presence of 6 µg/ml Hexadimethrine bromide (Polybrene) (Sigma) to assist with transduction efficiency. Titered viral concentrations for a MOI of 15 were determined using a Quanti-IT PicoGreen Assay (Invitrogen). Two days after transduction, 12 µg/ml Blasticidin was used to select for pure populations for 4 days. Subsequently, shVim-hMSCs and shLacZ-hMSCs were cultured in the presence of 1 µg/ml doxycycline to induce RNA interference (RNAi). Cells were cultured for 14–18 days on tissue culture polystyrene before being harvested to be assayed. The success of this knockdown in hMSCs has been previously described as a 40-60% decrease in vimentin expression [37]. For data analysis we only chose cells with vimentin knockdown, we did this by verifying with vimentin immunofluorescence.
5 × 105 PDL ~ 15–17 hMSCs (RoosterBio) were transfected with 6 µg EGFP-Vimentin DNA plasmid according to the Amaxa™ Optimized Protocol provided by the LONZA Nucleofector Kit for hMSCs. On day 3, pure populations were selected using 500 µg/mL geneticin for 1 day. On Day 7, cells were harvested to be assayed.
Surface reflective interference contrast microscopy (SRIC) was used to detect surface-to-surface interference between light rays reflected from the substrate/medium interface and those from the medium/cell membrane interface. The intensity of the light is a measure of the proximity of the cell membrane to the glass surface, so the membrane closest to the surface appears darker and those further away appear brighter. Therefore, SRIC is an optimal method when evaluating cellular attachment, adhesion, and spreading behavior. Cells were fixed in 4% paraformaldehyde prior to imaging and stained for vimentin. To visualize vimentin, cells were labelled with a rabbit IgG anti-human vimentin primary antibody (ThermoFisher) followed by a biotinylated (anti-rabbit IgG) secondary antibody (vector) and fluorescein-labelled streptavidin (Vector). For image capture using SRIC and to visualize vimentin, we used a Nikon Eclipse TE2000-E microscope.
To visualize vimentin and analyze focal adhesion staining, cells fixed with 4% paraformaldehyde and permeabilized using 0.1% Triton-X-100. Cells were then labelled with a rabbit IgG anti-human vimentin primary antibody (ThermoFisher) followed by a biotinylated (anti-rabbit IgG) secondary antibody (Vector) and fluorescein-labelled streptavidin (Vector). To dual stain for vimentin and vinculin, after vimentin staining, cells were then labelled with a mouse IgG anti-human vinculin primary antibody followed by an Alexa Fluor 594-labelled goat anti-mouse IgG secondary antibody. To dual stain for vimentin and F-actin, after vimentin staining, Alexa Fluor 594 Phalloidin was used to label F-actin. DAPI was used to stain cellular nuclei. Fluorescent imaging was completed using a Nikon Eclipse TE2000-E microscope. ImageJ was used for the quantification of the average vinculin adhesion area. All images were converted to binary images to isolate individual vinculin adhesions, and the particle analyzer in ImageJ was used to detect all adhesions greater than 0.5 µm2 to prevent the quantification of background noise. The average vinculin adhesion area was recorded for each individual cell.
25 mm diameter glass coverslips (VWR) or 35 mm diameter (14 mm diameter glass) glass bottomed dishes (Matek) were sterilized using UV light for 30 min and then coated with 100 µg/ml human fibronectin for 30 min (Corning). EGFP-vimentin hMSCs were seeded onto glass-bottomed dishes while shVim- and shLacZ- hMSCs were seeded onto the glass coverslips. Chemical disruption of actin microfilaments and inhibition of cellular contractility was completed using 0.4 µM cytochalasin-D (Sigma) treatment, respectively. Chemical treatments were conducted at 37 °C and 5% CO2. After the designated treatment time, the cells were fixed with 4% paraformaldehyde. To visualize vimentin with disrupted cytoskeletal elements in shVim- and shLacZ-hMSCs were then permeabilized using 0.1% Triton-X-100. Subsequently, cells were labelled with a rabbit IgG anti-human vimentin primary antibody (ThermoFisher) followed by a biotinylated (anti-rabbit IgG) secondary antibody (Vector) and fluorescein-labelled streptavidin (Vector). Fluorescent imaging was completed using a Nikon Eclipse TE2000-E microscope.
Chemical disruption of cytoskeletal elements and time lapse microscopy of EGFP-vimentin hMSCs was completed after 4 days of culture. After adding the chemicals directly to the cells, fluorescence images were taken every 2 min for 1.5 hours using an Olympus IX81 microscope with an environmental chamber to maintain the environment at 5% CO2 and 37 °C.
Tissue culture treated polyester transwell inserts with 3.0 µm pores were coated with 100 µg/mL fibronectin for 30 min at room temperature. The 3.0 µm pores restricts movement of whole cell bodies while allowing hMSCs to form cellular projections. shVim- and shLacZ-hMSCs were seeded onto transwell inserts for 20 min. The bottom chamber was filled growth media containing SDF-1 (ThermoFisher) (200 ng/ml) Cells were incubated at 37°C and 5% CO2 for 24 hr, after which the cells were fixed with 4% paraformaldehyde and permeabilized using 0.1% Triton-X-100. To visualize protrusion formation, cells were then labelled with a rabbit IgG anti-human vimentin primary antibody (ThermoFisher) followed by a biotinylated (anti-rabbit IgG) secondary antibody (vector) and fluorescein-labelled streptavidin (Vector). Alexa Fluor 594-labelled Phalloidin was used to stain F-Actin. Fluorescence images were taken of the protrusions using a Nikon Eclipse TE2000-E microscope. 15–30 fields of view were taken for only the bottom face of each transwell insert and the number, length, and average area of protrusions were analyzed on ImageJ or Fiji software.
To determine significant differences in focal adhesion area between shVim- and shLacZ-hMSCs, Student's t-test (alpha = 0.05) was used. To determine significant differences between the average number, length, and area of protrusions on by shVim- and shLacZ-hMSCs, a Student's t-test (alpha = 0.05) was also used. In all experiments involving fluorescence (SRIC, vinculin, vimentin, actin, transwell and retraction assays) we analyzed a minimum number of 20 cells from 3 independent experiments per experimental group (shLacZ, shVim).
First, we evaluated the relationship between the cell-substrate contact and the vimentin network (Figure 1). Surface reflective interference contrast microscopy (SRIC) was used to evaluate the proximity between the cell membrane and the underlying substrate. Areas with closer contact between the cell membrane and the substrate appear darker in the resulting micrograph, due to a greater degree of reflective interference, whereas white/gray areas indicate that the cell membrane and the glass substrate are farther apart. In the same cells, immunofluorescence of vimentin enabled us to localize the distribution of vimentin IFs. Superimposing the SRIC and vimentin immunofluorescence images enabled us to characterize the relationship between cell adhesion and vimentin.
As expected, vimentin knockdown cells (shVim-hMSCs) exhibited diminished immunofluorescence intensity, but its effect was most striking at the edges of cells (Figure 1D, G). In addition, shVim-hMSCs exhibited larger darkened areas on SRIC images, indicating that a larger proportion of these cells were flattened against the substrate. In contrast, shLacZ cells did not have these large darkened areas (Figure 1A–C). More importantly, the areas that lacked vimentin fluorescence (Figure 1F, I) coincided with these darkened areas. Our results clearly indicate that within a cell the absence of vimentin directly corresponds to areas of cell-substrate contact.
Noting the changes in vimentin IF organization, we then sought to determine whether there are any differences in actin microfilament organization between shVim- and shLacZ-hMSCs. In shLacZ-hMSCs, both vimentin and actin filaments were observed along the cell edge (Figure 2A). When comparing the actin fluorescence staining with SRIC, it appeared that the darker areas of enhanced cell-substrate contact appeared in areas where actin filaments terminated. In shVim-hMSCs, the protrusions and edges of the cell were largely absent of vimentin, but appeared to have thicker bundles of actin filaments, compared to the shLacZ-hMSCs (Figure 2B, C). When we divided the actin fluorescence between Ashvim/AshlacZ (Figure 2D, patterned column) we found that the value was higher than 1, indicating that there is more actin in shVim cells than shLacZ cells. When we compared the ratio of actin to vimentin fluorescence between shVim and shLacZ-hMSCs (Figure 2D, black and white column bars respectively), we found that at the cell edges shVim-hMSCs expressed more actin than vimentin (Figure 2D), as indicated by the black column (Ashvim/Vshvim) being higher than white column (AshlacZ/VshlacZ).
The termination of actin in the darker areas in SRIC prompted us to look at vinculin staining in those areas. We calculated the average area of the focal adhesions by taking a binary image of the fluorescence images using ImageJ (Figure 2E–G, n = 20 cells per experimental group). At first glance, the areas of vinculin immunopositivity seemed similar between shVim and shLacZ cells. However, they were statistically larger in shVim-hMSCs than in shLacZ-hMSCs (Figure 2E, H, I), and shVim cells also exhibited greater variation in the values of vinculin areas.
Additionally, darkened areas correspond almost precisely with vinculin fluorescence expression (Figure 2H, I), indicating that regions of close cell-substrate are correlated with vinculin stained focal adhesions. In the case of shVim-hMSCs, while the dark areas still match some of the vinculin staining, the amount of dark area is increased beyond vinculin (Figure 2I). This indicates that the reduction of a vimentin network increases regions of close cell-substrate proximity.
Due to actin's apparent role in the adhesion of shVim-hMSCs to the substrate, we decided to inhibit actin polymerization using cytochalasin D (Figure 3). In shLacZ-hMSCs, upon treatment of cytochalasin D, the cell body retracted, but did not completely round up (Figure 3A–G). As the cell body retracted, some small cytoplasmic protrusions remained in place (Figure 3B, D, E, G). Using live-cell imaging of EGFP-Vimentin expressing cells, we observed that these cytoplasmic protrusions contained vimentin (Figure 3C, D). When we treated shVim-hMSCs with cytochalasin D, the cell bodies retracted into a rounded morphology and no cytoplasmic protrusions were left remaining in place (Figure 3F, H), compared to the shLacZ-hMSCs where these projections were still observed. This effect was observed in all 3 different independent experiments with shVim and shLacZ cells. These results indicate that vimentin plays a role in the stability of the cell during retraction.
To gain a better understanding of the role of vimentin in protrusion formation, we used a transwell assay to evaluate the differences between the control shLacZ-hMSCs and vimentin-deficient shVim-hMSCs (Figure 4A). SDF-1 was used as a chemoattractant, which resulted in the formation of cellular protrusions as the cells tried to reach the bottom portion of the well (Figure 4A). One major difference between shVim- and shLacZ-hMSCs was the lack of protrusions visibly extending from the bottom of the transwell insert in vimentin knockdown cells. In shVim-hMSCs short, actin-rich, cytoplasmic extensions could be observed having migrated shortly beyond the transwell pores (Figure 4B). ShVim-hMSCs did not form the long cellular projections (or protrusions) rich in vimentin that were observed in the shLacZ-hMSCs (Figure 4C). In shLacZ cells, z-projections revealed that these protrusions extending from the shLacZ-hMSCs contained both actin and vimentin (Figure 4D–F). Vimentin appeared to be centrally located within the cells and was surrounded by cortical actin on the outside.
To quantify the differences in protrusion formation between shLacZ- and shVim-hMSCs, we determined the average number of protrusions per viewing frame (Figure 4G–I). Significantly more shLacZ-hMSCs formed long cellular projections compared to shVim-hMSCs. Because we knocked down the expression of vimentin rather than ablated it, vimentin positive projections could still be observed in shVim-hMSCs. There was no difference observed in the length of each protrusion (data not shown), demonstrating that vimentin-deficient cells can still grow cell projections if they retain enough vimentin to form these protrusions.
In this study, we sought to clarify vimentin's role in MSC-substrate interactions by using lentiviral-based RNAi to decrease expression of vimentin intermediate filaments. Our results show that vimentin is needed to control cell-substrate adhesion. Without vimentin, regions of the cell are found in closer proximity to the substrate. Vimentin also played a role in the formation of protrusions through a transwell thus, indicating that vimentin networks provide a structural element to control the cell membrane.
Vimentin immunofluorescence images show that control shLacZ-hMSCs have vimentin IFs present throughout the cell. Further, SRIC micrographs show a vimentin network that is interspersed with darkened areas corresponding to focal adhesions within the shLacZ-hMSCs. Comparatively, shVim-hMSCs lack vimentin near the cell edges, and possess larger darkened areas where the cell membrane is closer to the substrate surface. These dark regions correspond to areas of the cell without vimentin (Figure 1), suggesting that with decreased vimentin the MSC cell membrane is closer to the substrate surface and may have increased regions of surface adhesion.
Interestingly, we observed that the regions in shVim cells in which vimentin was absent, had an increase expression of actin (Figure 2). Suggesting that actin filaments try to compensate for the absence of vimentin. Another similar effect was observed when we forced the cells to retract (Figure 3). The disruption of F-actin in GFP-vimentin hMSCs and shLacZ-hMSCs revealed vimentin-positive extensions remaining when the cell body retracted. The cellular extensions were not active pseudopodia, but remnants after the cell body retracted. In shVim-hMSCs these remnants were not existent, and the cells immediately retracted into a sphere. This experiment provides further evidence of the importance of vimentin network and the potential connection between actin and vimentin. In fact, the interplay between actin and vimentin has been studied to understand cell morphogenesis [38]. In this paper, researchers found that specific actin stress fibers interact with vimentin networks via plectin. In this study it was also shown that disruption of actin produced changes in the vimentin network, and vice versa.
Plectin also has been found to couple intermediate filaments to focal adhesions [39],[40]. In our experiments, the immunofluorescence for focal adhesions, as visualized through vinculin, showed co-localization with the darkened areas visualized with SRIC. In control cells, the area of SRIC dark regions and the area of vinculin fluorescence was almost identical. However, in shVim cells we found that while vinculin fluorescence was still contained within the dark areas, these dark areas were much larger. Investigation of other focal adhesion proteins (integrins), may help clarify if there are more proteins in this area or if the cell membrane simply has collapsed into the substrate creating the increase in dark areas in SRIC. In fact, a study in endothelial cells has found several proteins that were recognized in what they call vimentin associated matrix adhesions (VMAs) [18], this includes vinculin, integrin and the interaction of this adhesions with vimentin is mediated by plectin. Thus, these proteins could be potential targets for future studies.
In our study we found even in control cells that vinculin fluorescence had a large variation in terms of size (area). Still, shVim cells showed vinculin focal adhesions that were statistically larger than shLacZ cells (control). A study in endothelial cells subjected to flow, found that RNA vimentin inhibition resulted in smaller focal adhesions. Interestingly, they also found that integrin focal adhesions associate with vimentin bundles [19]. While our study found larger focal adhesion, they had a different physical condition (flow vs no flow), different cell type and focal adhesion protein. Another study proposed a model in which fibroblasts' vimentin network is connected to integrins via synemin and vinculin or plectin [41]. In this study [41] they found that fibroblasts from mice with a R113C mutation that resulted in disrupted vimentin did not result in changes in vinculin. It could be possible that some of the changes related to vimentin and cell adhesion occur in the proteins connecting the intermediate filament to integrins or vinculin. For example, Synemin might connect vimentin with vinculin [42] and could be a potential target for future studies.
So far, our studies explored a relatively static system in which cells did not move or migrate. While the retraction assay explored the “leftover” protrusions, our transwell assay explore the formation of new protrusions via chemotactic signals. We observed that shvim-hMSCs lacked vimentin-positive cellular projections, which shows that vimentin was also necessary to actively form cell projections. Our results showed that shLacZ-hMSCs' extensions contained both vimentin and F-actin (Figure 4). Closer to the transwell pore and the origin of the extension, vimentin appears to be in the interior of these protrusions with actin primarily located in the outer regions. Closer to the protrusion end, vimentin and actin appear more interspersed. It is possible that in addition to potentially playing a structural role, vimentin may be involved in initial contact and adhesion. Comparatively, microtubules have been found to be needed for protrusion elongation [15] and microtentacles are dependent on a microtubule network [43]. However, the study of microtubules and other structures are outside of the scope of this paper.
It is well established that vimentin deficiency impairs cellular migration in a variety of cell types including fibroblasts, leukocytes, astrocytes, and numerous cancer cell types [16],[17],[26],[28],[32]–[34]. Increasingly, studies have shown that vimentin is necessary for the formation of filopodial structures during adhesion and early spreading on 2D surfaces [22]. Similarly, vimentin has been found to be needed for other types of cellular extensions as well such as for early neurite extension in hippocampal neurons [44] and lamellipodia formation for epithelial lens repair [34]. Our results support this assertion that vimentin IFs may be necessary for the formation of protrusions in MSCs.
The importance of vimentin in the formation of cellular protrusions has also been studied in cancer cells. Invadopodia formation and extension in breast cancer and colorectal cancer cells lines both required vimentin for elongation [15]. Similarly, actin-independent tubulin microtentacles on detached breast carcinoma cells were found to contain vimentin [43]. Disruption of vimentin with phosphatase inhibitors in these cells as well as the use of vimentin negative cancer cells resulted in a decrease in the number of microtentacles. It has been proposed that vimentin provides stability for the formation of longer microtentacles, or cellular extensions, compared to the shorter, less stable vimentin-negative microtentacles of less invasive cancer cells [43].
Our study is primarily limited by the use of RNAi to decrease vimentin networks rather than to ablate all vimentin expression. Specifically, MSCs completely lacking a vimentin network may yield larger effects in protrusion formation and extension [43]. In addition, we chose to examine the formation of cellular protrusions using small pored transwell inserts. Examining transmigration through a variety of pore sizes as well as alternative culture and test conditions such as confined migration scenarios [45], gel degradation experiments [15], and transmigration through cell layers [46] may reveal more intricacies about vimentin IF's role in protrusive structures for MSC migration. We are also limited by the size and optical constraints of our system to observe more intricate details in the architecture of the vimentin network and its relationship with the actin cytoskeleton. Changes in pore size using microfabrication, or perhaps cell micropatterning could be used to create cellular projections of different lengths and widths that could be used to evaluate the role and architecture of vimentin in a variety of cellular projections. The use of electron microscopy and super resolution fluorescence microscopy could be used to better observe the architecture of protrusions from shVim and shLacZ cells. Further investigation into the relationship between vimentin, F-actin, and microtubules will help to clarify the formation, extension, and maintenance of these protrusive structures.
This study shines light on the importance of vimentin to maintain cellular morphology, cell-substrate distance and create protrusions trough chemotaxis. Both the capacities to adhere and form pseudopodia are critical cellular functions, and greater understanding of the mechanisms involved in these behaviors in MSCs is increasingly relevant as MSCs continue to be investigated for therapeutic purposes.
[1] |
Jo CH, Lee YG, Shin WH, et al. (2014) Intra-articular injection of mesenchymal stem cells for the treatment of osteoarthritis of the knee: a proof-of-concept clinical trial. Stem Cells 32: 1254-1266. doi: 10.1002/stem.1634
![]() |
[2] |
Orozco L, Soler R, Morera C, et al. (2011) Intervertebral disc repair by autologous Mesenchymal bone marrow cells: a pilot study. Transplantation 92: 822-828. doi: 10.1097/TP.0b013e3182298a15
![]() |
[3] |
Squillaro T, Peluso G, Galderisi U (2016) Clinical trials with mesenchymal stem cells: an update. Cell Transplant 25: 829-848. doi: 10.3727/096368915X689622
![]() |
[4] | Kang SK, Shin IS, Ko MS, et al. (2012) Journey of mesenchymal stem cells for homing: strategies to enhance efficacy and safety of stem cell Therapy. Stem Cells Int 2012: e342968. |
[5] |
Chapel A, Bertho JM, Bensidhoum M, et al. (2003) Mesenchymal stem cells home to injured tissues when co-infused with hematopoietic cells to treat a radiation-induced multi-organ failure syndrome. J Gene Med 5: 1028-1038. doi: 10.1002/jgm.452
![]() |
[6] |
Sasaki M, Abe R, Fujita Y, et al. (2008) Mesenchymal stem cells are recruited into wounded skin and contribute to wound repair by transdifferentiation into multiple skin cell type. J Immunol 180: 2581-2587. doi: 10.4049/jimmunol.180.4.2581
![]() |
[7] |
Wynn RF, Hart CA, Corradi-Perini C, et al. (2004) A small proportion of mesenchymal stem cells strongly expresses functionally active CXCR4 receptor capable of promoting migration to bone marrow. Blood 104: 2643-2645. doi: 10.1182/blood-2004-02-0526
![]() |
[8] |
Son B-R, Marquez-Curtis LA, Kucia M, et al. (2006) Migration of bone marrow and cord blood mesenchymal stem cells in vitro is regulated by stromal-derived factor-1-CXCR4 and hepatocyte growth factor-c-met axes and involves matrix metalloproteinases. Stem Cells 24: 1254-1264. doi: 10.1634/stemcells.2005-0271
![]() |
[9] |
McBeath R, Pirone DM, Nelson CM, et al. (2004) Cell shape, cytoskeletal tension, and RhoA regulate stem cell lineage commitment. Dev Cell 6: 483-495. doi: 10.1016/S1534-5807(04)00075-9
![]() |
[10] |
Kilian KA, Bugarija B, Lahn BT, et al. (2010) Geometric cues for directing the differentiation of mesenchymal stem cells. Proc Natl Acad Sci 107: 4872-4877. doi: 10.1073/pnas.0903269107
![]() |
[11] |
Engler AJ, Sen S, Sweeney HL, et al. (2006) Matrix elasticity directs stem cell lineage specification. Cell 126: 677-689. doi: 10.1016/j.cell.2006.06.044
![]() |
[12] |
Curran JM, Chen R, Hunt JA (2006) The guidance of human mesenchymal stem cell differentiation in vitro by controlled modifications to the cell substrate. Biomaterials 27: 4783-4793. doi: 10.1016/j.biomaterials.2006.05.001
![]() |
[13] |
Salasznyk RM, Williams WA, Boskey A, et al. (2004) Adhesion to vitronectin and collagen I promotes osteogenic differentiation of human mesenchymal stem cells. J Biomed Biotechnol 2004: 24-34. doi: 10.1155/S1110724304306017
![]() |
[14] |
Ridley AJ, Schwartz MA, Burridge K, et al. (2003) Cell migration: integrating signals from front to back. Science 302: 1704-1709. doi: 10.1126/science.1092053
![]() |
[15] |
Schoumacher M, Goldman RD, Louvard D, et al. (2010) Actin, microtubules, and vimentin intermediate filaments cooperate for elongation of invadopodia. J Cell Biol 189: 541-556. doi: 10.1083/jcb.200909113
![]() |
[16] |
Havel LS, Kline ER, Salgueiro AM, et al. (2015) Vimentin regulates lung cancer cell adhesion through a VAV2–Rac1 pathway to control focal adhesion kinase activity. Oncogene 34: 1979-1990. doi: 10.1038/onc.2014.123
![]() |
[17] |
McInroy L, Määttä A (2007) Down-regulation of vimentin expression inhibits carcinoma cell migration and adhesion. Biochem Biophys Res Commun 360: 109-114. doi: 10.1016/j.bbrc.2007.06.036
![]() |
[18] |
Gonzales M, Weksler B, Tsuruta D, et al. (2001) Structure and function of a vimentin-associated matrix adhesion in endothelial cells. Mol Biol Cell 12: 85-100. doi: 10.1091/mbc.12.1.85
![]() |
[19] |
Tsuruta D (2003) The vimentin cytoskeleton regulates focal contact size and adhesion of endothelial cells subjected to shear stress. J Cell Sci 116: 4977-4984. doi: 10.1242/jcs.00823
![]() |
[20] |
Kreis S, Schönfeld H-J, Melchior C, et al. (2005) The intermediate filament protein vimentin binds specifically to a recombinant integrin α2/β1 cytoplasmic tail complex and co-localizes with native α2/β1 in endothelial cell focal adhesions. Exp Cell Res 305: 110-121. doi: 10.1016/j.yexcr.2004.12.023
![]() |
[21] |
Kim H, Nakamura F, Lee W, et al. (2010) Regulation of cell adhesion to collagen via β1 integrins is dependent on interactions of filamin A with vimentin and protein kinase C epsilon. Exp Cell Res 316: 1829-1844. doi: 10.1016/j.yexcr.2010.02.007
![]() |
[22] |
Kim H, Nakamura F, Lee W, et al. (2010) Filamin A is required for vimentin-mediated cell adhesion and spreading. Am J Physiol-Cell Physiol 298: C221-C236. doi: 10.1152/ajpcell.00323.2009
![]() |
[23] |
Bhattacharya R, Gonzalez AM, DeBiase PJ, et al. (2009) Recruitment of vimentin to the cell surface by β3 integrin and plectin mediates adhesion strength. J Cell Sci 122: 1390-1400. doi: 10.1242/jcs.043042
![]() |
[24] |
Homan SM, Martinez R, Benware A, et al. (2002) Regulation of the Association of α6β4 with vimentin intermediate filaments in endothelial cells. Exp Cell Res 281: 107-114. doi: 10.1006/excr.2002.5643
![]() |
[25] |
Liu C-Y, Lin H-H, Tang M-J, et al. (2015) Vimentin contributes to epithelial-mesenchymal transition cancer cell mechanics by mediating cytoskeletal organization and focal adhesion maturation. Oncotarget 6: 15966-15983. doi: 10.18632/oncotarget.3862
![]() |
[26] | Eckes B, Dogic D, Colucci-Guyon E, et al. (1998) Impaired mechanical stability, migration and contractile capacity in vimentin-deficient fibroblasts. J Cell Sci 111: 1897-1907. |
[27] |
Bershadsky AD, Tint IS, Svitkina TM (1987) Association of intermediate filaments with vinculin-containing adhesion plaques of fibroblasts. Cell Motil Cytoskeleton 8: 274-283. doi: 10.1002/cm.970080308
![]() |
[28] |
Mendez MG, Kojima S-I, Goldman RD (2010) Vimentin induces changes in cell shape, motility, and adhesion during the epithelial to mesenchymal transition. FASEB J 24: 1838-1851. doi: 10.1096/fj.09-151639
![]() |
[29] |
Burgstaller G, Gregor M, Winter L, et al. (2010) Keeping the vimentin network under control: cell–matrix adhesion–associated plectin 1f affects cell shape and polarity of fibroblasts. Mol Biol Cell 21: 3362-3375. doi: 10.1091/mbc.e10-02-0094
![]() |
[30] |
Huang L, Niu C, Willard B, et al. (2015) Proteomic analysis of porcine mesenchymal stem cells derived from bone marrow and umbilical cord: implication of the proteins involved in the higher migration capability of bone marrow mesenchymal stem cells. Stem Cell Res Ther 6: 7. doi: 10.1186/scrt541
![]() |
[31] |
Satelli A, Li S (2011) Vimentin in cancer and its potential as a molecular target for cancer therapy. Cell Mol Life Sci 68: 3033-3046. doi: 10.1007/s00018-011-0735-1
![]() |
[32] |
Zhao Y, Yan Q, Long X, et al. (2008) Vimentin affects the mobility and invasiveness of prostate cancer cells. Cell Biochem Funct 26: 571-577. doi: 10.1002/cbf.1478
![]() |
[33] |
Helfand BT, Mendez MG, Murthy SNP, et al. (2011) Vimentin organization modulates the formation of lamellipodia. Mol Biol Cell 22: 1274-1289. doi: 10.1091/mbc.e10-08-0699
![]() |
[34] |
Menko AS, Bleaken BM, Libowitz AA, et al. (2014) A central role for vimentin in regulating repair function during healing of the lens epithelium. Mol Biol Cell 25: 776-790. doi: 10.1091/mbc.e12-12-0900
![]() |
[35] |
Nieminen M, Henttinen T, Merinen M, et al. (2006) Vimentin function in lymphocyte adhesion and transcellular migration. Nat Cell Biol 8: 156-162. doi: 10.1038/ncb1355
![]() |
[36] |
Barberis L, Pasquali C, Bertschy-Meier D, et al. (2009) Leukocyte transmigration is modulated by chemokine-mediated PI3Kγ-dependent phosphorylation of vimentin. Eur J Immunol 39: 1136-1146. doi: 10.1002/eji.200838884
![]() |
[37] |
Sharma P, Bolten ZT, Wagner DR, et al. (2017) Deformability of human mesenchymal stem cells is dependent on vimentin intermediate filaments. Ann Biomed Eng 45: 1365-1374. doi: 10.1007/s10439-016-1787-z
![]() |
[38] |
Jiu Y, Lehtimäki J, Tojkander S, et al. (2015) Bidirectional interplay between vimentin intermediate filaments and contractile actin stress fibers. Cell Rep 11: 1511-1518. doi: 10.1016/j.celrep.2015.05.008
![]() |
[39] |
Burgstaller G, Gregor M, Winter L, et al. (2010) Keeping the vimentin network under control: cell–matrix adhesion–associated plectin 1f affects cell shape and polarity of fibroblasts. Mol Biol Cell 21: 3362-3375. doi: 10.1091/mbc.e10-02-0094
![]() |
[40] |
Gregor M, Osmanagic-Myers S, Burgstaller G, et al. (2014) Mechanosensing through focal adhesion-anchored intermediate filaments. FASEB J 28: 715-729. doi: 10.1096/fj.13-231829
![]() |
[41] |
Bornheim R, Müller M, Reuter U, et al. (2008) A dominant vimentin mutant upregulates Hsp70 and the activity of the ubiquitin-proteasome system, and causes posterior cataracts in transgenic mice. J Cell Sci 121: 3737-3746. doi: 10.1242/jcs.030312
![]() |
[42] |
Uyama N, Zhao L, Rossen EV, et al. (2006) Hepatic stellate cells express synemin, a protein bridging intermediate filaments to focal adhesions. Gut 55: 1276-1289. doi: 10.1136/gut.2005.078865
![]() |
[43] |
Whipple RA, Balzer EM, Cho EH, et al. (2008) Vimentin filaments support extension of tubulin-based microtentacles in detached breast tumor cells. Cancer Res 68: 5678-5688. doi: 10.1158/0008-5472.CAN-07-6589
![]() |
[44] |
Boyne LJ, Fischer I, Shea TB (1996) Role of vimentin in early stages of neuritogenesis in cultured hippocampal neurons. Int J Dev Neurosci 14: 739-748. doi: 10.1016/S0736-5748(96)00053-6
![]() |
[45] |
Paul CD, Hung W-C, Wirtz D, et al. (2016) Engineered models of confined cell migration. Annu Rev Biomed Eng 18: 159-180. doi: 10.1146/annurev-bioeng-071114-040654
![]() |
[46] |
Smith H, Whittall C, Weksler B, et al. (2011) Chemokines stimulate bidirectional migration of human mesenchymal stem cells across bone marrow endothelial cells. Stem Cells Dev 21: 476-486. doi: 10.1089/scd.2011.0025
![]() |
1. | Mahmood Dehghani-Ashkezari, Fateme Montazeri, Ehsan Farashahi-Yazd, Fatemeh Hajizadeh-Tafti, Jalal Golzadeh, Seyed Mehdi Hoseini, Behrouz Aflatoonian, Immunomodulatory Potential of Human Testicular Tissue-Derived Mesenchymal Stem/Stromal Cells over the Lifespan, 2024, 18, 1990-519X, 390, 10.1134/S1990519X24700329 |