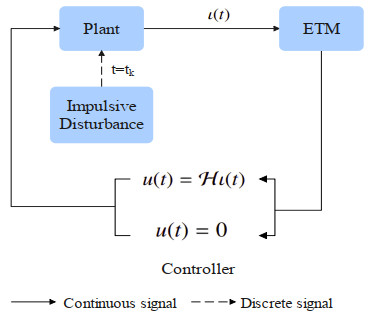
Traumatic brain injury (TBI) remains a significant public health concern, with current treatments primarily addressing acute symptoms while failing to mitigate secondary injuries that contribute to long-term neurological deficits. This article discusses emerging therapeutic strategies, including stem cell–based approaches, biomaterials, and exosome-based treatments, which show promise in promoting tissue repair, reducing inflammation, and enhancing neurological function. Despite these advancements, challenges such as immune rejection, scalability, and the absence of standardized clinical protocols persist, underscoring the need for further refinement and interdisciplinary collaboration across molecular biology, bioengineering, and clinical neuroscience. In particular, integrating regenerative strategies with advanced biomaterials may result in synergistic effects improving recovery outcomes. Additionally, this article explores the potential of novel materials, such as carbogenic nanozymes, and innovations in tissue engineering, including hydrogels and nanocarriers, to mitigate oxidative stress, preserve blood–brain barrier integrity, and modulate neuroinflammation. Furthermore, macrophage-based therapies, such as backpack–macrophage therapy and photobiomodulation (PBM) are emerging as promising interventions to address chronic TBI complications, including post-traumatic epilepsy and cognitive impairments. However, further research is needed to optimize treatment parameters and overcome barriers to clinical translation. Ultimately, the integration of these advanced therapeutic strategies, combined with a deeper understanding of neuroinflammatory and neurodegenerative processes, has the potential to revolutionize TBI treatment, offering improved recovery and quality of life for affected individuals.
Citation: Moawiah M Naffaa. Innovative therapeutic strategies for traumatic brain injury: integrating regenerative medicine, biomaterials, and neuroengineering[J]. AIMS Bioengineering, 2025, 12(1): 90-144. doi: 10.3934/bioeng.2025005
[1] | Tao Xie, Xing Xiong . Finite-time synchronization of fractional-order heterogeneous dynamical networks with impulsive interference via aperiodical intermittent control. AIMS Mathematics, 2025, 10(3): 6291-6317. doi: 10.3934/math.2025287 |
[2] | Huiling Li, Jin-E Zhang, Ailong Wu . Finite-time stabilization of nonlinear systems with partially known states via aperiodic intermittent control and event-triggered impulsive control. AIMS Mathematics, 2025, 10(2): 3269-3290. doi: 10.3934/math.2025152 |
[3] | Linni Li, Jin-E Zhang . Input-to-state stability of nonlinear systems with delayed impulse based on event-triggered impulse control. AIMS Mathematics, 2024, 9(10): 26446-26461. doi: 10.3934/math.20241287 |
[4] | Biwen Li, Yujie Liu . Quasi-synchronization of nonlinear systems with parameter mismatch and time-varying delays via event-triggered impulsive control. AIMS Mathematics, 2025, 10(2): 3759-3778. doi: 10.3934/math.2025174 |
[5] | Jiaojiao Li, Yingying Wang, Jianyu Zhang . Event-triggered sliding mode control for a class of uncertain switching systems. AIMS Mathematics, 2023, 8(12): 29424-29439. doi: 10.3934/math.20231506 |
[6] | Miao Xiao, Zhe Lin, Qian Jiang, Dingcheng Yang, Xiongfeng Deng . Neural network-based adaptive finite-time tracking control for multiple inputs uncertain nonlinear systems with positive odd integer powers and unknown multiple faults. AIMS Mathematics, 2025, 10(3): 4819-4841. doi: 10.3934/math.2025221 |
[7] | Biwen Li, Qiaoping Huang . Synchronization issue of uncertain time-delay systems based on flexible impulsive control. AIMS Mathematics, 2024, 9(10): 26538-26556. doi: 10.3934/math.20241291 |
[8] | Guijun Xing, Huatao Chen, Zahra S. Aghayan, Jingfei Jiang, Juan L. G. Guirao . Tracking control for a class of fractional order uncertain systems with time-delay based on composite nonlinear feedback control. AIMS Mathematics, 2024, 9(5): 13058-13076. doi: 10.3934/math.2024637 |
[9] | Li Liu, Yinfang Song, Hong Yu, Gang Zhang . Almost sure exponential synchronization of multilayer complex networks with Markovian switching via aperiodically intermittent discrete observa- tion noise. AIMS Mathematics, 2024, 9(10): 28828-28849. doi: 10.3934/math.20241399 |
[10] | Aziz Belmiloudi . Time-varying delays in electrophysiological wave propagation along cardiac tissue and minimax control problems associated with uncertain bidomain type models. AIMS Mathematics, 2019, 4(3): 928-983. doi: 10.3934/math.2019.3.928 |
Traumatic brain injury (TBI) remains a significant public health concern, with current treatments primarily addressing acute symptoms while failing to mitigate secondary injuries that contribute to long-term neurological deficits. This article discusses emerging therapeutic strategies, including stem cell–based approaches, biomaterials, and exosome-based treatments, which show promise in promoting tissue repair, reducing inflammation, and enhancing neurological function. Despite these advancements, challenges such as immune rejection, scalability, and the absence of standardized clinical protocols persist, underscoring the need for further refinement and interdisciplinary collaboration across molecular biology, bioengineering, and clinical neuroscience. In particular, integrating regenerative strategies with advanced biomaterials may result in synergistic effects improving recovery outcomes. Additionally, this article explores the potential of novel materials, such as carbogenic nanozymes, and innovations in tissue engineering, including hydrogels and nanocarriers, to mitigate oxidative stress, preserve blood–brain barrier integrity, and modulate neuroinflammation. Furthermore, macrophage-based therapies, such as backpack–macrophage therapy and photobiomodulation (PBM) are emerging as promising interventions to address chronic TBI complications, including post-traumatic epilepsy and cognitive impairments. However, further research is needed to optimize treatment parameters and overcome barriers to clinical translation. Ultimately, the integration of these advanced therapeutic strategies, combined with a deeper understanding of neuroinflammatory and neurodegenerative processes, has the potential to revolutionize TBI treatment, offering improved recovery and quality of life for affected individuals.
Impulsive systems are a class of dynamical systems characterized by sudden changes in state or input at discrete time points. Such dynamical properties of impulsive systems have generated widespread applications in fields such as electrical engineering [1], digital signal processing [2], and biomedical engineering [3]. In impulsive systems, impulses are typically categorized into stabilizing impulses and disturbance impulses. Stabilizing impulses represent the stabilizing effect and optimal performance achieved by the nonlinear system under specific impulsive control, positively influencing system stability (see, e.g., [4,5]). Conversely, disturbance impulses may occur when the nonlinear system is subjected to external perturbations, negatively affecting system stability and potentially leading to instability (see, e.g., [6,7]). To maintain system stability and performance, corresponding control strategies are required to suppress or compensate for such disturbance impulses.
In certain practical engineering applications, such as wind power management, vehicle control, and spacecraft trajectory adjustments, continuously feeding control signals into the plant system is not realistic. Compared to traditional continuous control methods, intermittent control (IC) allows for inputting control signals into the plant system only at specific control time intervals, making it more suitable for these actual applications. IC can be divided into periodic intermittent control (PIC) (see e.g., [8,9,10]) and aperiodic intermittent control (APIC) (see, e.g., [11,12,13]). PIC inputs control signals into the plant system at fixed control period and control width, while APIC is more flexible, allowing for dynamic adjustments of control strategies as needed. In [14], exponential stability of nonlinear systems under PIC was investigated, and stability criteria based on linear matrix inequalities (LMI) and scalar inequalities were proposed. Given that the control period and width in PIC are fixed and known, the derived conditions may be too conservative. Consequently, there is an urgent need to introduce the APIC to replace PIC. In [15], the stabilization problem of continuous systems achieving exponential input-to-state stability through APIC was studied. In [16], the concept of aperiodic time-triggered IC was introduced, and a lower conservative exponential stability theorem was formulated for the considered aperiodic time-triggered IC system through the construction of a hybrid time-dependent Lyapunov functional. In the light of these reporting-based works, the APIC has become a highly regarded area of interest.
In current research, traditional time-triggered control (TC) and event-triggered control (EC) employ different approaches for control system design. TC involves sending and executing control signals at regular intervals, which, although simple and convenient, may lead to resource wastage and performance degradation due to its fixed-time interval signal transmission. In contrast, EC significantly reduces resource consumption and enhances system performance by updating control signals under specific triggering conditions. Recently, EC has achieved significant progress in achieving the satisfied dynamic performances of control systems (see, e.g., [17,18,19,20]). However, EC is unnecessary and energy-consuming as the event generator and controller continue to keep on running when the control execution is not required. Hence, an event-triggered intermittent control (EIC) strategy combining IC with EC has emerged. This strategy operates within specific time periods, allowing for intermittent updating of control signals, thereby further reducing resource consumption. A dynamic EIC scheme with input delays was introduced to stabilize the delayed dynamical systems in [21]. The time-triggered APIC and event-triggered APIC (EAPIC) schemes were developed to achieve exponential stabilization of continuous-time dynamical systems in [22].
Within a finite-time control framework, two particular stability concepts are under scrutiny: asymptotic stability, where system trajectories converge to equilibrium states (see, e.g., [23,24]), and finite-time stability (FTS), where the system does not exceed a predefined threshold in finite-time. Building upon the FTS concept, finite-time contraction stability (FTCS) further emphasizes the "boundedness" and "contraction" nature of the system itself. Numerous theoretical results exist regarding FTS and FTCS (see, e.g., [25,26,27]). Additionally, some guidelines for FTS and FTCS are also presented for impulsive systems (see, e.g., [28,29,30]). In [31], some sufficient conditions of FTS/FTCS for nonlinear impulsive systems were constructed via the Lyapunov function method. In [32], FTS and FTCS of nonlinear systems subject to impulsive disturbances with parameter uncertainties were obtained by designing APIC. A further exploration about FTS for nonlinear systems involving impulsive disturbances under the EAPIC strategy faces a series of challenges. This includes how to build the relationship between the IC parameters and event-triggering mechanism (ETM) threshold under EAPIC, as well as whether the designing APIC can suppress the effects of impulsive disturbance. These issues constitute the focal points of further study. From the above discussion, the strengths of this paper can be concluded as below:
(1) Based on the Lyapunov method and LMI techniques, this paper combines EC and APIC to investigate the FTS and FTCS of uncertain systems with uncertain impulsive disturbance using EAPIC, where the impulsive instants are generated by the predesigned ETM.
(2) Four elements of uncertain systems–FTS/FTCS, APIC, and impulse disturbances–are combined simultaneously. Moreover, under EAPIC, the correlation among finite-time interval, impulsive disturbance, inspection period, and ETM threshold is revealed. Additionally, the adopted EAPIC can exclude the Zeno phenomenon.
(3) You et al. [32] apply time-triggered APIC to stabilize uncertain nonlinear systems with impulse disturbance. This method involves control actions at fixed time intervals, which can lead to unnecessary computations and communications. In contrast, the EAPIC considered in this paper triggers control based on the actual changes in the system state, thus being more universal and flexible.
Notations: R and R+ represent the sets of real and non-negative real numbers, respectively. Z+ stands for the set of positive integers. Rn denotes the n-dimensional real space with the Euclidean norm ||⋅||. Rn×m indicates the n×m dimensional real space. Y≥0 or Y≤0 means that matrix Y is a positive definite or negative definite symmetric matrix. YT and Y−1 are used to describe the transpose and inverse of Y separately. λmax(Y) and λmin(Y) represent the maximum and minimum eigenvalues of matrix Y, respectively. ∗ stands for a symmetric block within a symmetric matrix. I denotes the unit matrix possessing suitable dimension. C([−θ,0];Rn) is the family of continuous functions ψ:[−θ,0]→Rn. A locally Lipschitz function ν:Rn→R+ has the upper right-hand Dini derivative determined by
D+ν(ι)=lim supa→0+ν(ι+a)−ν(ι)a. |
The uncertain system is considered as follows:
{˙ι(t)=A(t)ι(t)+B(t)σ(ι(t−θ))+C(t)u(t),t≥0,ι(t)=ς(t),t∈[−θ,0], | (2.1) |
where ι(t)∈Rn is state vector, A(t),B(t)∈Rn×n, as well as C(t)∈Rn×m. Assume that A(t)= A+ΔA(t), B(t)=B+ΔB(t), and C(t)=C+ΔC(t), in which A,B, and C are known real matrices, whereas ΔA(t),ΔB(t), and ΔC(t) are uncertain unknown matrices. σ(ι(t))=(σ1(ι1(t)),σ2(ι2(t)),⋯,σn(ιn(t)))T∈Rn with σ(0)=0. θ denotes time delay. The APIC u(t)∈Rm is given as below
u(t)={Hι(t),tk≤t<tk+τk,0,tk+τk≤t<tk+1, | (2.2) |
in which H signifies the control strength. tk,τk respectively denote the start time and control width of each control period, which satisfy 0<τk<tk+1−tk. It can be noted that the controller (2.2) requires operation only on control intervals. That is, the absence of transmitting control input information during the rest interval implies that system (2.1) operates alternately between the open-loop control mode and the closed-loop control mode. Moreover, it is considered that the state of system (2.1) jumps in certain cases due to the activation of the aperiodic interval controller, namely, the generation of impulsive phenomena, and we regard them as disturbance impulses. Thus, system (2.1) with impulses involved can be formulated as
{˙ι(t)=A(t)ι(t)+B(t)σ(ι(t−θ))+C(t)u(t),t≠tk,t≥0,ι(t)=D(t)ι(t−),t=tk,ι(t)=ς(t),t∈[−θ,0], | (2.3) |
where D(t) represents the impulse gain matrix, which satisfies D(t)=D+ΔD(t). D is a known matrix, and ΔD(t) is an unknown matrix. Assume system (2.3) is right continuous. Put ι(t,0,ς) to indicate the solution of system (2.3) through (0,ς),ς∈Rn. The series {tk}+∞k=1 fulfills 0=t0<t1<⋯<tk<⋯ and limk→+∞=+∞.
It is assumed that the intermittent sequence {tk} is created through the ETM as follows:
tk+1={inf{t:t∈Γk(tk+τk,tk+ω]},ifΓk(tk+τk,tk+ω]≠∅,tk+ω,ifΓk(tk+τk,tk+ω]=∅, | (2.4) |
where Γk(ε,t]={t:t>ε+τk,U(ι(t))≥eζU(ι(ε+τk))}, eζ,ζ>0 denotes the threshold value, ω>0 stands for the inspection period, which is generally devised as a constant large enough, as well as U(⋅) indicates the Lyapunov function with respect to the state of the system (2.3). Figures 1 and 2 describe the EAPIC loop and framework of EAPIC, respectively.
The following describes some assumptions, definitions, and lemmas.
Assumption 2.1 ([29]). Suppose there are positive constants ϖj, which make
|σj(β1)−σj(β2)|≤ϖj|β1−β2|,β1,β2∈R,j=1,2,…,n, |
hold.
Assumption 2.2 ([32]). The parameter uncertainties ΔA(t),ΔB(t),ΔC(t), and ΔD(t) satisfy
(ΔA(t),ΔB(t),ΔC(t))=NK1(t)(M1,M2,M3),ΔD(t)=FK2(t)M4, |
where N,F,M1,M2,M3, and M4 are known matrices as well as Ki(t) is an uncertain matrix-valued function of suitable dimension fulfilling
KTi(t)Ki(t)≤I,i=1,2. |
Definition 2.1. For specified constants T,δ1,δ2,π,ρ with 0<ρ<δ1<δ2 and π∈(0,T), along with an arbitrary trajectory ι(t), the existence of a controller making ||ι(0)||≤δ1 implies that ||ι(t)||≤δ2 for t∈[0,T], then system (2.3) is called FTS with respect to (w.r.t) (T,δ1,δ2). Moreover, for t∈[T−π,T], if ||ι(t)||≤ρ, then system (2.3) is called FTCS w.r.t (T,δ1,δ2,ρ,π).
Remark 2.1. According to [33], FTS depicts the "boundedness" of the described systems. In contrast, FTCS demonstrates the "boundedness" and "contraction" of the described systems simultaneously. In particular, to attain the "contraction" feature, FTCS requires the additional condition that the reasoned systems can remain in a comparatively smaller boundary than the initial boundary until the end time is implemented. Figure 3 visually depicts the FTS and FTCS, which also consider the possible impact on the system of impulsive disturbance.
Lemma 2.1 ([34]). For matrices D,S,F,M, and K(t) with appropriate dimensions and S>0,KTK(t)≤I, scalars κ>0 and S−κFFT>0, it follows that
(D+FK(t)M)TS−1(D+FK(t)M)≤DT(S−κFFT)−1D+κ−1MTM. |
Lemma 2.2 ([35]). For a symmetric and positive matrix Q, constant ϱ>0, one has
2eTf≤ϱeTQe+ϱ−1fTQ−1f,e,f∈Rn. |
Lemma 2.3 ([36]). The N and M are matrices with proper dimensions. Let K(t) meet KTK(t)≤I. For any γ>0, then
NK(t)M+MTKT(t)NT≤γNNT+γ−1MTM. |
Lemma 2.4 ([29]). Let ι∈C([χ−θ,∞);[0,∞)) satisfy
˙ι(t)≤−cι(t)+dmax{ιt},t≥χ. |
If c>d>0, then
ι(t)≤[max{ιχ}]exp(−λ(t−χ)),t≥χ, |
in which max{ιt}=supt−θ≤ϕ≤tι(ϕ), and λ is the smallest positive real root in the following equation
c−λ−deλθ=0. |
Lemma 2.5 ([37]). For t∈(−θ,+∞), if function ι≥0 meets
˙ι(t)≤a1ι(t)+a2ι(t−θ),a1,a2>0,t≥0, |
it follows that
ι(t)≤||ι(0)||exp((a1+a2)t). |
In this section, sufficient conditions for FTS and FTCS of system (2.3) are obtained through EAPIC.
Theorem 3.1. Suppose that Assumptions 2.1 and 2.2 hold, there exists symmetric and positive matrix P, positive constants λ,δ1,δ2,T,ρ,π,μ1,μ2,γ1,γ2,γ3,γ4,α1,α2,η1,η2,ϑ, where ρ<δ1<δ2,π∈(0,T),μ1≤μ2, and ϑ≥1 to satisfy
(A)μ1I≤P≤μ2I;
(B)(−ϑPDTPMT4∗−P+γ4PFFTP0∗∗−γ4I)<0;
(C)(˜ψ1PB∗−α1I+γ−12MT2M2)<0, where
˜ψ1=PA+ATP+PCH+HTCTP+(γ1+γ2+γ3)PNNTP+γ−11MT1M1+γ−13HTMT3M3H+η1P; |
(D)(˜ψ2PB∗−α2I+γ−12MT2M2)<0, where
˜ψ2=PA+ATP+(γ1+γ2)PNNTP+γ−11MT1M1−η2P; |
(E)ζλ≤ˉτ≤τk≤ω−ζη2+h2;
(F)n(0,t)lnϑ−λ(∑n(0,t)i=0τi)+(n(0,t)+1)ζ≤ln(μ1δ22)−ln(μ2δ21),t∈[0,T],
in which λ is the smallest positive solution of the formula η1−λ−h1eλθ=0 and η1>h1. ˉτ=lim inf∑k−1i=0τik denotes the minimum average control width. n(0,t) represents the control period count on (0,T],T≠tk. Thus, the Zeno phenomenon is ruled out, and system (2.3) is FTS w.r.t (T,δ1,δ2) under APIC (2.2). Besides, for t∈[T−π,T], if the following relation.
(G)n(0,t)lnϑ−λ(∑n(0,t)i=0τi)+(n(0,t)+1)ζ≤ln(μ1ρ2)−ln(μ2δ21) holds, then system (2.3) is FTCS w.r.t (T,δ1,δ2,ρ,π) under APIC (2.2).
Proof. For 0<||ι(0)||≤δ1, assume that ι(t)=ι(t,0,ς) is a solution of system (2.3) via (0,ς). Consider the Lyapunov function as follows:
U(ι(t))=ιT(t)Pι(t). |
From (B) in Theorem 3.1, it follows that
−ϑP+DT(P−1−γ4FFT)−1D+γ−14MT4M4≤0. |
For t=tk, by using Lemma 2.1, we obtain
U(ι(tk))=ιT(tk)Pι(tk)=ιT(t−k)DT(tk)PD(tk)ι(t−k)=ιT(t−k)(D+ΔD(tk))TP(D+ΔD(tk))ι(t−k)=ιT(t−k)(D+FK2(t)M4)TP(D+FK2(t)M4)ι(t−k)≤ιT(t−k)[DT(P−1−γ4FFT)−1D+γ−14MT4M4]ι(t−k)≤ϑU(ι(t−k)). | (3.1) |
For t≠tk, when t∈[tk,tk+τk), we obtain
D+U(ι(t))=2ιT(t)P˙ι(t)=2ιT(t)P((A(t)+C(t)H)ι(t)+B(t)σ(ι(t−θ)))=2ιT(t)P((A+ΔA(t)+CH+ΔC(t)H)ι(t)+(B+ΔB(t))σ(ι(t−θ)))=ιT(t)(PA+ATP+PCH+HTCTP)ι(t)+2ιT(t)PΔA(t)ι(t)+2ιT(t)PΔC(t)Hι(t)+2ιT(t)PBσ(ι(t−θ))+2ιT(t)PΔB(t)σ(ι(t−θ)). | (3.2) |
Next, together with Assumption 2.2, Lemma 2.2, and Lemma 2.3 to obtain
2ιT(t)PΔA(t)ι(t)=2ιT(t)PNK1(t)M1ι(t)=ιT(t)(PNK1(t)M1+MT1KT1(t)NTPT)ι(t)≤ιT(t)(γ1PNNTP+γ−11MT1M1)ι(t), | (3.3) |
2ιT(t)PΔB(t)σ(ι(t−θ))=2ιT(t)PNK1(t)M2σ(ι(t−θ))≤ιT(t)γ2PNNTPι(t)+σT(ι(t−θ))γ−12MT2M2σ(ι(t−θ)), | (3.4) |
2ιT(t)PΔC(t)Hι(t)=2ιT(t)PNK1(t)M3Hι(t)=ιT(t)(PNK1(t)M3H+HTMT3KT1(t)NTPT)ι(t)≤ιT(t)(γ3PNNTP+γ−13HTMT3M3H)ι(t). | (3.5) |
Bringing (3.3)–(3.5) into (3.2), we have, according to (C) in Theorem 3.1, that
D+U(ι(t))≤ιT(t)[PA+ATP+PCH+HTCTP+(γ1+γ2+γ3)PNNTP+γ−11MT1M1+γ−13HTMT1M1H]ι(t)+2ιT(t)PBσ(ι(t−θ))+σT(ι(t−θ))γ−12MT2M2σ(ι(t−θ))≤(ιT(t)σT(ι(t−θ)))(˜ψ1PB∗−α1I+γ−12MT2M2)(ι(t)σ(ι(t−θ)))−η1ιT(t)Pι(t)+α1σT(ι(t−θ)σ(ι(t−θ)≤−η1U(ι(t))+h1U(ι(t−θ)), |
where h1=α1ϖσλmax(P−1) with ϖσ=max{ϖ21,ϖ22,…,ϖ2n}. For t∈[tk,tk+τk), by Lemma 2.4, one has
U(ι(t))≤U(ι(tk))exp(−λ(t−tk)). | (3.6) |
Similarly, for t∈[tk+τk,tk+1), it follows that
D+U(ι(t))≤ιT(t)[PA+ATP+(γ1+γ2)PNNTP+γ−11MT1M1]ι(t)+2ιT(t)PBσ(ι(t−θ))+σT(ι(t−θ))γ−12MT2M2σ(ι(t−θ))≤(ιT(t)σT(ι(t−θ)))(˜ψ2PB∗−α2I+γ−12MT2M2)(ι(t)σ(ι(t−θ)))+η2ιT(t)Pι(t)+α2σT(ι(t−θ))σ(ι(t−θ))≤η2U(ι(t))+h2U(ι(t−θ)), |
where h2=α2ϖσλmax(P−1). Based on Lemma 2.5, when t∈[tk+τk,tk+1), we obtain
U(ι(t))≤U(ι(tk+τk))exp((η2+h2)(t−tk−τk)). | (3.7) |
Thus,
U(ι(tk+1))≤U(ι(tk+τk))exp((η2+h2)(tk+1−tk−τk)). | (3.8) |
If Γk(tk+τk,tk+ω]≠∅, then tk+1−tk≤ω and only when the event occurrence function to be zero will trigger the next event, namely
U(ι(tk+1))=eζU(ι(tk+τk)). | (3.9) |
Consequently, together with (3.8) and (3.9), we derive
τk+ζη2+h2≤tk+1−tk≤ω. | (3.10) |
If Γk(tk+τk,tk+ω]=∅, then tk+1−tk=ω, (3.10) still stands. Because ζη2+h2>0, combining (3.10) with (E) in Theorem 3.1, it follows that
0<(1λ+1η2+h2)ζ≤tk+1−tk≤ω. | (3.11) |
Therefore, the Zeno behavior of system (2.3) is excluded.
For t∈[tk+τk,tk+1), by ETM (2.4), we obtain
U(ι(t))≤eζU(ι(tk+τk)). | (3.12) |
When t∈[0,τ0), by (3.6), it is obtained that
U(ι(t))≤U(ι(0))exp(−λt) |
and
U(ι(τ0))≤U(ι(0))exp(−λτ0). |
When t∈[τ0,t1), based on (3.12), one obtains
U(ι(t))≤eζU(ι(τ0))≤U(ι(0))exp(ζ−λτ0), |
and
U(ι(t−1))≤U(ι(0))exp(ζ−λτ0). |
When t∈[t1,t1+τ1), it follows from (3.1) and (3.6) that
U(ι(t))≤U(ι(t1))exp(−λ(t−t1))≤ϑU(ι(t−1))exp(−λ(t−t1))≤ϑU(ι(0))exp(ζ−λ(τ0+t−t1)), |
and
U(ι(t1+τ1))≤ϑU(ι(0))exp(ζ−λ(τ0+τ1)). |
When t∈[t1+τ1,t2), we obtain
U(ι(t))≤eζU(ι(t1+τ1))≤ϑU(ι(0))exp(2ζ−λ(τ0+τ1)), |
and
U(ι(t−2))≤ϑU(ι(0))exp(2ζ−λ(τ0+τ1)). |
When t∈[t2,t2+τ2), one has
U(ι(t))≤U(ι(t2))exp(−λ(t−t2))≤ϑU(ι(t−2))exp(−λ(t−t2))≤ϑ2U(ι(0))exp(2ζ−λ(τ0+τ1+t−t2)), |
and
U(ι(t2+τ2))≤ϑ2U(ι(0))exp(2ζ−λ(τ0+τ1+t−t2)). |
When t∈[t2+τ2,t3), there holds
U(ι(t))≤eζU(ι(t2+τ2))≤ϑ2U(ι(0))exp(3ζ−λ(τ0+τ1+τ2)). |
By mathematical induction, when t∈[tk+τk,tk+1), one obtains
U(ι(t))≤ϑkU(ι(0))exp((k+1)ζ−λ(k∑i=0τi))=U(ι(0))exp(klnϑ−λ(k∑i=0τi)+(k+1)ζ), |
which means
U(ι(t))≤U(ι(0))exp(n(0,t)lnϑ−λ(n(0,t)∑i=0τi)+(n(0,t)+1)ζ),t∈[0,T]. | (3.13) |
Using (A) and (F) in Theorem 3.1, we derive
μ1||ι(t)||2≤U(ι(t))≤μ2||ι(0)||2U(ι(0))exp(n(0,t)lnϑ−λ(n(0,t)∑i=0τi)+(n(0,t)+1)ζ)≤μ2δ21exp(n(0,t)lnϑ−λ(n(0,t)∑i=0τi)+(n(0,t)+1)ζ)≤μ1δ22, |
which implies ||ι(t)||≤δ2, and hence, ||ι(t)||≤δ2 holds on [0,T]. So, system (2.3) can achieve FTS under APIC (2.2). When t∈[T−π,T], if (G) in Theorem 3.1 is satisfied, it follows from (3.13) that
μ1||ι(t)||2≤U(ι(t))≤μ2||ι(0)||2U(ι(0))exp(n(0,t)lnϑ−λ(n(0,t)∑i=0τi)+(n(0,t)+1)ζ)≤μ2δ21exp(n(0,t)lnϑ−λ(n(0,t)∑i=0τi)+(n(0,t)+1)ζ)≤μ1ρ2, |
which shows that ||ι(t)||≤ρ for all t∈[T−π,T]. Then, system (2.3) can achieve FTCS under APIC (2.2).
Remark 3.1. It is important to note that conditions (C) and (D) in Theorem 3.1 regulate the continuous dynamics so that (3.6) and (3.7) hold, where the parameters η1 and η2 imply the decay rate and potential growth for system (2.3), respectively. This means that system (2.3) shows a decreasing trend in the control intervals, which makes system (2.3) to some extent divergent in the resting intervals. Furthermore, ϑ≥1 in condition (B) of Theorem 3.1 suggests that impulses may have destabilizing effects.
As a matter of fact, when τk=τ,tk+1−tk−τ=c with τ,c>0, APIC (2.2) can be turned into PIC as follows:
u(t)={Hι(t),kJ≤t<kJ+τ,0,kJ+τ≤t<(k+1)J, | (3.14) |
where J>0 denotes the control period as well as 0<τ<J indicates the control width, then the following corollary is obvious.
Corollary 3.1. Suppose that Assumptions 2.1 and 2.2 hold, there exists symmetric and positive matrix P, positive constants λ,δ1,δ2,T,ρ,π,μ1,μ2,γ1,γ2,γ3,γ4,α1,α2,η1,η2,ϑ, where ρ<δ1<δ2,π∈(0,T),μ1≤μ2, and ϑ≥1 to satisfy (A)−(D) in Theorem 3.1, and for any t∈[0,T], if the following relation
n(0,t)(lnϑ+(η2+h2)c−λc)≤ln(μ1δ22)−ln(μ2δ21)−(η2+h2)c |
holds, then system (2.3) is FTS w.r.t (T,δ1,δ2) under PIC (3.14). Besides, for t∈[T−π,T], if
n(0,t)(lnϑ+(η2+h2)c−λc)≤ln(μ1ρ2)−ln(μ2δ21)−(η2+h2)c |
holds, then system (2.3) is FTCS w.r.t (T,δ1,δ2,ρ,π) under PIC (3.14).
Remark 3.2. It should be noted that (3.14), as a special case of (2.2), is time-triggered, meanwhile, both J and τ are fixed.
Next, when parameter uncertainties ΔA(t)=ΔB(t)=ΔC(t)=ΔD(t)=0, the uncertain system (2.3) is transformed into the following deterministic system:
{˙ι(t)=Aι(t)+Bσ(ι(t−τ))+Cu(t),t≠tk,t≥0,ι(t)=Dι(t−),t=tk,ι(t)=ς(t),t∈[−θ,0]. | (3.15) |
Corollary 3.2. Suppose that Assumption 2.1 holds and there exists symmetric positive matrices P, positive constants λ,δ1,δ2,T,ρ,π,μ1,μ2,α1,α2,η1,η2,ϑ, where ρ<δ1<δ2,π∈(0,T),μ1≤μ2, and ϑ≥1 such that (A) in Theorem 3.1 and
(B′)(−ϑPDTP∗−P)<0;
(C′)(˜ψ1PB∗−α1I)<0, where ˜ψ1=PA+ATP+PCH+HTCTP+η1P;
(D′)(˜ψ2PB∗−α2I)<0, where ˜ψ2=PA+ATP−η2P;
(E′)ζλ≤ˉτ≤τk≤ω−ζη2+h2;
hold, then system (3.15) is FTS w.r.t (T,δ1,δ2) under APIC (2.2) if (F) in Theorem 3.1 is true and the Zeno phenomenon is excluded. Furthermore, system (3.15) is FTCS w.r.t (T,δ1,δ2,ρ,π) under APIC (2.2) if (G) in Theorem 3.1 is satisfied when t∈[T−π,T].
The validity of the theoretical results will be verified by two numerical examples.
Example 4.1. Consider the uncertain system as follows:
˙ι(t)=A(t)ι(t)+B(t)σ(ι(t−θ)) | (4.1) |
on t≥0, where
A=(−0.700−0.8),B=(1.8002), |
σ(ι(t−θ))=(0.3(|0.85ι1(t)+2|−|0.85ι1(t)−2|)0.3(|0.85ι2(t−θ)+2|−|0.85ι2(t−θ)−2|)), |
θ=0.5,ΔA(t)=ΔB(t)=(0.1sin(0.5t)000.1sin(0.5t)). |
Consider the initial condition ι(0)=(1.05,0.6)T, parameters δ1=1.21,δ2=2, and T=9.5. Based on the state trajectories of system (4.1) shown in Figure 4, we know that system (4.1) is not FTS w.r.t (9.5, 1.21, 2).
When APIC u(t) and impulsive disturbance are accounted for, system (4.1) can be rewritten as
{˙ι(t)=A(t)ι(t)+B(t)σ(ι(t−τ))+C(t)u(t),t≠tk,t≥0,ι(t)=D(t)ι(t−),t=tk,ι(t)=ς(t),t∈[−θ,0], | (4.2) |
where
C=(0.10.1),D=(1.20.10.11.2),ΔC(t)=(0.1sin(0.5t)0), |
ΔD(t)=(0.1sin(0.5t)000.1sin(0.5t)) |
and
u(t)={Hι(t),tk≤t<tk+τk,0,tk+τk≤t<tk+1. | (4.3) |
Let ϖ1=ϖ2=0.51,N=F=I2,M1=M2=M4=0.1I2,M3=(0.1;0),Ki(t)=(sin(0.5t),0;0,sin(0.5t)) and KTi(t)Ki(t)≤I2(i=1,2), in which case Assumptions 2.1 and 2.2 hold.
Assume that μ1=5.2,μ2=5.5,ϑ=2,γ1=0.0425,γ2=0.043,γ3=0.0358, γ4=0.018,α1=1.8266,α2=2.3013,η1=0.3665,η2=1.1. Under conditions (A)−(D) of Theorem 3.1, it can be obtained by using the MATLAB toolbox
P=(5.35−0.0018−0.00185.35),H=(−1.864−1.864). |
Additionally, by calculating, we can obtain h1=0.0888,h2=0.1119, and λ=0.2651.
Design ω=5,ζ=0.1 as well as τk=3.5, then the intermittent sequence {tk} is decided by the following ETM
tk+1={inf{t:t∈Γk(tk+3.5,tk+5]},ifΓk(tk+3.5,tk+5]≠∅,tk+5,ifΓk(tk+3.5,tk+5]=∅, | (4.4) |
where
Γk(ε,t]={t:t>ε+3.5,U(ι(t))≥e0.1U(ι(ε+3.5))}, |
and U(ι(t))=ιT(t)Pι(t). Obviously, the condition (E) in Theorem 3.1 is satisfied. According to Figure 5, we can obtain the intermittent times as t1=3.6359,t2=7.4563. Furthermore, when t∈[0,9.5], we have that the intermittent sequences {tk} and control span τk meet
0.6931k−0.9279k+0.1(k+1)≤0.949,k=0,1,2. |
By Theorem 3.1, we can conclude that system (4.2) is FTS w.r.t (9.5, 1.21, 2) under APIC (4.3). Moreover, take π=2,ρ=1.15. When t∈[7.5,9.5], the intermittent sequences {tk} and control span τk satisfy
0.6931k−0.9279k+0.1(k+1)≤−0.1578,k=2. |
Based on Theorem 3.1, we can deduce that system (4.2) is FTCS w.r.t (9.5, 1.21, 2, 1.15, 2) under APIC (4.3). The relevant simulation results for ι(0)=(1.05,0.6)T are displayed in Figure 5, where Figure 5(a) portrays the trajectories of ι1,ι2; Figure 5(b, c) shows the trajectories of ||ι(t)|| and u(t), respectively.
Example 4.2. Let us consider the two degrees of freedom damped dual-mass spring system illustrated in Figure 6, which has the following mathematical expression:
{m1¨ι1(t)=σ1+u+k2(ι2−ι1)+c2(˙ι2−˙ι1)−k1ι1−c1˙ι1,m2¨ι2(t)=σ2−k2(ι2−ι1)−c2(˙ι2−˙ι1), | (4.5) |
where m1,m2∈R+ denote two masses, ι1,ι2∈R+ represent displacements of masses m1,m2, k1,k2∈R+ indicate elasticity coefficients of two springs, c1,c2∈R+ signify damping coefficients of two dampers, as well as u stands for the control input as shown in (2.2). Setting ˙ι1=ι3,˙ι2=ι4, and ι=(ι1,ι2,ι3,ι4)T, then we obtain
˙ι=(00100001−k1+k2m1k2m1−c1+c2m1c2m1k2m2−k2m2c2m2−c2m2)ι+(00000000001m100001m2)(00σ1σ2)+(001m10)u. | (4.6) |
In real applications, the damped dual-mass spring system may experience transient disturbances in the control mass m1 caused by input noise and uncertainty. We treat such transient disturbances as impulsive disturbances that induce an instantaneous change in velocity, namely, ι(tk)=Dι(t−k), where D=diag{1,1,d,1}. Moreover, the sequence of impulsive moments is given by ETM (4.4). Assume that σ1=sin(0.1ι1(t)),σ2=sin(0.1ι2(t−θ)), where θ=0.2. Let μ1=1,μ2=3,η1=0.6513,η2=1.961,ϑ=1.8, α1=1.6371,α2=1.5088,k1=2.5,k2=2, m1=3.5,m2=1.2,c1=0.5,c2=3,d=1.3. According to (A),(C′)−(D′) in Corollary 3.2, using the MATLAB toolbox, one has
P=(1.992−0.14150.7554−0.2287−0.14151.67−0.017540.56890.7554−0.017541.684−0.1489−0.22870.5689−0.14891.59),H=(−2.005−4.391−5.702−9.21). |
By further calculations, it follows that h1=0.0159,h2=0.0147, as well as λ=0.6333. Therefore, (E′) in Corollary 3.2 holds. As can be seen from Figure 7, the impulsive instants are t1=4.6267,t2=9.6267. Let T=6,δ1=2.17,δ2=4,ρ=1.85,π=1.2, and the initial value is ι0=(1.8,0.8,−0.1,−0.9). When t∈[0,6], we have that the impulsive sequences {tk} and control span τk meet
0.5789k−2.2165k+0.1(k+1)≤0.1245,k=0,1. |
From Corollary 3.2, we can deduce that under APIC (2.2), system (4.6) is FTS w.r.t (6, 2.17, 4). Moreover, when t∈[4.8,6], the impulsive sequences {tk} and control span τk satisfy
0.5789k−2.2165k+0.1(k+1)≤−1.4176,k=1. |
According to Corollary 3.2, we can infer that system (4.6) is FTCS w.r.t (6, 2.17, 4, 1.85, 1.2) under APIC (2.2). The associated simulation results are exhibited in Figure 7.
Remark 4.1. Without considering the control input u and impulsive disturbance, it can be seen from Figure 8 that system (4.6) is not FTCS w.r.t (6, 2.17, 4, 1.85, 1.2).
Due to traditional PIC methods, which execute control actions at fixed time intervals regardless of system state changes, inefficiencies may arise. To overcome these restrictions, this paper proposes the EAPIC method by combining APIC with EC. Through this approach, the FTS and FTCS of parameter-uncertain systems with impulsive disturbance are investigated. With the aid of Lyapunov methods and LMI techniques, criteria for FTS and FTCS of parameter uncertain systems are derived. Additionally, under EAPIC conditions, relationships among impulsive disturbance, intermittent control parameters, and ETM threshold are established. The sequence of impulsive instants is determined by predefined ETM, and the Zeno phenomenon is ruled out. In future work, we will focus on how to incorporate external disturbances, such as random noise, into the EAPIC system. We plan to adapt the existing event-triggered control strategy to enhance the robustness of the system to these disturbances and ensure that the control strategy remains effective under various disturbance conditions.
Tian Xu: Writing-original draft; Jin-E Zhang: Supervision, writing-review & editing. Both authors have read and approved the final version of the manuscript for publication.
The authors would like to thank the editors and referees for their very helpful comments and suggestions.
The authors declare that there are no conflicts of interest.
[1] |
Haarbauer-Krupa J, Pugh MJ, Prager EM, et al. (2021) Epidemiology of chronic effects of traumatic brain injury. J Neurotrauma 38: 3235-3247. https://doi.org/10.1089/neu.2021.0062 ![]() |
[2] |
Thurman DJ, Alverson C, Dunn KA, et al. (1999) Traumatic brain injury in the United States: a public health perspective. J Head Trauma Rehabil 14: 602-615. https://doi.org/10.1097/00001199-199912000-00009 ![]() |
[3] |
Wilson MH (2016) Traumatic brain injury: an underappreciated public health issue. Lancet Public Health 1: e44. https://doi.org/10.1016/S2468-2667(16)30022-6 ![]() |
[4] |
McKee AC, Daneshvar DH (2015) The neuropathology of traumatic brain injury. Handb Clin Neurol 127: 45-66. https://doi.org/10.1016/B978-0-444-52892-6.00004-0 ![]() |
[5] |
Maas AIR, Menon DK, Manley GT, et al. (2022) Traumatic brain injury: progress and challenges in prevention, clinical care, and research. Lancet Neurol 21: 1004-1060. https://doi.org/10.1016/S1474-4422(22)00309-X ![]() |
[6] |
Rauchman SH, Albert J, Pinkhasov A, et al. (2022) Mild-to-moderate traumatic brain injury: a review with focus on the visual system. Neurol Int 14: 453-470. https://doi.org/10.3390/neurolint14020038 ![]() |
[7] |
Theadom A, Parag V, Dowell T, et al. (2016) Persistent problems 1 year after mild traumatic brain injury: a longitudinal population study in New Zealand. Br J Gen Pract 66: e16-e23. https://doi.org/10.3399/bjgp16X683161 ![]() |
[8] |
Silverberg ND, Iaccarino MA, Panenka WJ, et al. (2020) Management of concussion and mild traumatic brain injury: a synthesis of practice guidelines. Arch Phys Med Rehabil 101: 382-393. https://doi.org/10.1016/j.apmr.2019.10.179 ![]() |
[9] |
Simon DW, McGeachy MJ, Bayir H, et al. (2017) The far-reaching scope of neuroinflammation after traumatic brain injury. Nat Rev Neurol 13: 572. https://doi.org/10.1038/nrneurol.2017.116 ![]() |
[10] |
Postolache TT, Wadhawan A, Can A, et al. (2020) Inflammation in traumatic brain injury. J Alzheimers Dis 74: 1-28. https://doi.org/10.3233/JAD-191150 ![]() |
[11] |
Rabinowitz AR, Levin HS (2014) Cognitive sequelae of traumatic brain injury. Psychiatr Clin North Am 37: 1-11. https://doi.org/10.1016/j.psc.2013.11.004 ![]() |
[12] |
Rauchman SH, Zubair A, Jacob B, et al. (2023) Traumatic brain injury: mechanisms, manifestations, and visual sequelae. Front Neurosci 17: 1090672. https://doi.org/10.3389/fnins.2023.1090672 ![]() |
[13] |
Freire MAM, Rocha GS, Bittencourt LO, et al. (2023) Cellular and molecular pathophysiology of traumatic brain injury: what have we learned so far?. Biology 12: 1139. https://doi.org/10.3390/biology12081139 ![]() |
[14] | Ng SY, Lee AYW (2019) Traumatic brain injuries: pathophysiology and potential therapeutic targets. Front Cell Neurosci 13: 528. https://doi.org/10.3389/fncel.2019.00528 |
[15] |
Bramlett HM, Dietrich WD (2015) Long-term consequences of traumatic brain injury: current status of potential mechanisms of injury and neurological outcomes. J Neurotrauma 32: 1834-1848. https://doi.org/10.1089/neu.2014.3352 ![]() |
[16] |
Andriessen TM, Jacobs B, Vos PE (2010) Clinical characteristics and pathophysiological mechanisms of focal and diffuse traumatic brain injury. J Cell Mol Med 14: 2381-2392. https://doi.org/10.1111/j.1582-4934.2010.01164.x ![]() |
[17] |
Aghili-Mehrizi S, Williams E, Yan S, et al. (2022) Secondary mechanisms of neurotrauma: a closer look at the evidence. Diseases 10: 30. https://doi.org/10.3390/diseases10020030 ![]() |
[18] | Krishnamurthy K, Laskowitz DT (2016) Cellular and molecular mechanisms of secondary neuronal injury following traumatic brain injury. Translational Research in Traumatic Brain Injury. Boca Raton (FL): . https://doi.org/10.1201/b18959 |
[19] |
Peggion C, Cali T, Brini M (2024) Mitochondria dysfunction and neuroinflammation in neurodegeneration: who comes first?. Antioxidants 13: 240. https://doi.org/10.3390/antiox13020240 ![]() |
[20] |
Bylicky MA, Mueller GP, Day RM (2018) Mechanisms of endogenous neuroprotective effects of astrocytes in brain injury. Oxid Med Cell Longev 2018: 6501031. https://doi.org/10.1155/2018/6501031 ![]() |
[21] |
Khatri N, Sumadhura B, Kumar S, et al. (2021) The complexity of secondary cascade consequent to traumatic brain injury: pathobiology and potential treatments. Curr Neuropharmacol 19: 1984-2011. https://doi.org/10.2174/1570159X19666210215123914 ![]() |
[22] |
Eyolfson E, Khan A, Mychasiuk R, et al. (2020) Microglia dynamics in adolescent traumatic brain injury. J Neuroinflammation 17: 326. https://doi.org/10.1186/s12974-020-01994-z ![]() |
[23] |
Bhatt M, Sharma M, Das B (2024) The role of inflammatory cascade and reactive astrogliosis in glial scar formation post-spinal cord injury. Cell Mol Neurobiol 44: 78. https://doi.org/10.1007/s10571-024-01519-9 ![]() |
[24] |
Cieri MB, Ramos AJ (2025) Astrocytes, reactive astrogliosis, and glial scar formation in traumatic brain injury. Neural Regen Res 20: 973-989. https://doi.org/10.4103/NRR.NRR-D-23-02091 ![]() |
[25] |
Hamby ME, Sofroniew MV (2010) Reactive astrocytes as therapeutic targets for CNS disorders. Neurotherapeutics 7: 494-506. https://doi.org/10.1016/j.nurt.2010.07.003 ![]() |
[26] |
Alhadidi QM, Bahader GA, Arvola O, et al. (2024) Astrocytes in functional recovery following central nervous system injuries. J Physiol 602: 3069-3096. https://doi.org/10.1113/JP284197 ![]() |
[27] |
He L, Zhang R, Yang M, et al. (2024) The role of astrocyte in neuroinflammation in traumatic brain injury. Biochim Biophys Acta Mol Basis Dis 1870: 166992. https://doi.org/10.1016/j.bbadis.2023.166992 ![]() |
[28] |
Becerra-Calixto A, Cardona-Gomez GP (2017) The role of astrocytes in neuroprotection after brain stroke: potential in cell therapy. Front Mol Neurosci 10: 88. https://doi.org/10.3389/fnmol.2017.00088 ![]() |
[29] |
Liu X, Zhang L, Cao Y, et al. (2022) Neuroinflammation of traumatic brain injury: Roles of extracellular vesicles. Front Immunol 13: 1088827. https://doi.org/10.3389/fimmu.2022.1088827 ![]() |
[30] |
Chen L, Deng H, Cui H, et al. (2018) Inflammatory responses and inflammation-associated diseases in organs. Oncotarget 9: 7204-7218. https://doi.org/10.18632/oncotarget.23208 ![]() |
[31] |
Jassam YN, Izzy S, Whalen M, et al. (2017) Neuroimmunology of traumatic brain injury: time for a paradigm shift. Neuron 95: 1246-1265. https://doi.org/10.1016/j.neuron.2017.07.010 ![]() |
[32] |
Balanca B, Desmurs L, Grelier J, et al. (2021) DAMPs and RAGE pathophysiology at the acute phase of brain injury: an overview. Int J Mol Sci 22: 2439. https://doi.org/10.3390/ijms22052439 ![]() |
[33] |
Caceres E, Olivella JC, Di Napoli M, et al. (2024) Immune response in traumatic brain injury. Curr Neurol Neurosci Rep 24: 593-609. https://doi.org/10.1007/s11910-024-01382-7 ![]() |
[34] |
McKee CA, Lukens JR (2016) Emerging roles for the immune system in traumatic brain injury. Front Immunol 7: 556. https://doi.org/10.3389/fimmu.2016.00556 ![]() |
[35] |
Donat CK, Scott G, Gentleman SM, et al. (2017) Microglial activation in traumatic brain injury. Front Aging Neurosci 9: 208. https://doi.org/10.3389/fnagi.2017.00208 ![]() |
[36] |
Czyzewski W, Mazurek M, Sakwa L, et al. (2024) Astroglial cells: emerging therapeutic targets in the management of traumatic brain injury. Cells 13: 148. https://doi.org/10.3390/cells13020148 ![]() |
[37] |
Karve IP, Taylor JM, Crack PJ (2016) The contribution of astrocytes and microglia to traumatic brain injury. Br J Pharmacol 173: 692-702. https://doi.org/10.1111/bph.13125 ![]() |
[38] |
Chou A, Krukowski K, Morganti JM, et al. (2018) Persistent infiltration and impaired response of peripherally-derived monocytes after traumatic brain injury in the aged brain. Int J Mol Sci 19: 1616. https://doi.org/10.3390/ijms19061616 ![]() |
[39] |
Webster KM, Sun M, Crack P, et al. (2017) Inflammation in epileptogenesis after traumatic brain injury. J Neuroinflammation 14: 10. https://doi.org/10.1186/s12974-016-0786-1 ![]() |
[40] |
Wangler LM, Godbout JP (2023) Microglia moonlighting after traumatic brain injury: aging and interferons influence chronic microglia reactivity. Trends Neurosci 46: 926-940. https://doi.org/10.1016/j.tins.2023.08.008 ![]() |
[41] |
Delage C, Taib T, Mamma C, et al. (2021) Traumatic brain injury: an age-dependent view of post-traumatic neuroinflammation and its treatment. Pharmaceutics 13: 1624. https://doi.org/10.3390/pharmaceutics13101624 ![]() |
[42] |
Vella MA, Crandall ML, Patel MB (2017) Acute management of traumatic brain injury. Surg Clin North Am 97: 1015-1030. https://doi.org/10.1016/j.suc.2017.06.003 ![]() |
[43] |
Magid-Bernstein J, Girard R, Polster S, et al. (2022) Cerebral hemorrhage: pathophysiology, treatment, and future directions. Circ Res 130: 1204-1229. https://doi.org/10.1161/CIRCRESAHA.121.319949 ![]() |
[44] |
Si Z, Wang X, Sun C, et al. (2019) Adipose-derived stem cells: sources, potency, and implications for regenerative therapies. Biomed Pharmacother 114: 108765. https://doi.org/10.1016/j.biopha.2019.108765 ![]() |
[45] |
Li C, Zhao H, Cheng L, et al. (2021) Allogeneic vs. autologous mesenchymal stem/stromal cells in their medication practice. Cell Biosci 11: 187. https://doi.org/10.1186/s13578-021-00698-y ![]() |
[46] |
Yousefpour P, Ni K, Irvine DJ (2023) Targeted modulation of immune cells and tissues using engineered biomaterials. Nat Rev Bioeng 1: 107-124. https://doi.org/10.1038/s44222-022-00016-2 ![]() |
[47] |
Proto JD, Doran AC, Gusarova G, et al. (2018) Regulatory T cells promote macrophage efferocytosis during inflammation resolution. Immunity 49: 666-677.E6. https://doi.org/10.1016/j.immuni.2018.07.015 ![]() |
[48] |
Roszkowski S (2024) Therapeutic potential of mesenchymal stem cell-derived exosomes for regenerative medicine applications. Clin Exp Med 24: 46. https://doi.org/10.1007/s10238-023-01282-z ![]() |
[49] |
Swindle MM, Makin A, Herron AJ, et al. (2012) Swine as models in biomedical research and toxicology testing. Vet Pathol 49: 344-356. https://doi.org/10.1177/0300985811402846 ![]() |
[50] |
Bonsack B, Heyck M, Kingsbury C, et al. (2020) Fast-tracking regenerative medicine for traumatic brain injury. Neural Regen Res 15: 1179-1190. https://doi.org/10.4103/1673-5374.270294 ![]() |
[51] |
Raspa A, Gelain F (2021) Mimicking extracellular matrix via engineered nanostructured biomaterials for neural repair. Curr Neuropharmacol 19: 2110-2124. https://doi.org/10.2174/1570159X18666201111111102 ![]() |
[52] |
Smandri A, Al-Masawa ME, Hwei NM, et al. (2024) ECM-derived biomaterials for regulating tissue multicellularity and maturation. iScience 27: 109141. https://doi.org/10.1016/j.isci.2024.109141 ![]() |
[53] |
Zhao X, Hu DA, Wu D, et al. (2021) Applications of biocompatible scaffold materials in stem cell-based cartilage tissue engineering. Front Bioeng Biotechnol 9: 603444. https://doi.org/10.3389/fbioe.2021.603444 ![]() |
[54] |
Zhao X, Li Q, Guo Z, et al. (2021) Constructing a cell microenvironment with biomaterial scaffolds for stem cell therapy. Stem Cell Res Ther 12: 583. https://doi.org/10.1186/s13287-021-02650-w ![]() |
[55] |
Kharbikar BN, Mohindra P, Desai TA (2022) Biomaterials to enhance stem cell transplantation. Cell Stem Cell 29: 692-721. https://doi.org/10.1016/j.stem.2022.04.002 ![]() |
[56] |
Lucke-Wold BP, Logsdon AF, Nguyen L, et al. (2018) Supplements, nutrition, and alternative therapies for the treatment of traumatic brain injury. Nutr Neurosci 21: 79-91. https://doi.org/10.1080/1028415X.2016.1236174 ![]() |
[57] |
Scheff SW, Ansari MA (2017) Natural Compounds as a therapeutic intervention following traumatic brain injury: the role of phytochemicals. J Neurotrauma 34: 1491-1510. https://doi.org/10.1089/neu.2016.4718 ![]() |
[58] |
Lazaridis C, Rusin CG, Robertson CS (2019) Secondary brain injury: predicting and preventing insults. Neuropharmacology 145: 145-152. https://doi.org/10.1016/j.neuropharm.2018.06.005 ![]() |
[59] |
Aqel S, Al-Thani N, Haider MZ, et al. (2023) Biomaterials in traumatic brain injury: perspectives and challenges. Biology 13: 21. https://doi.org/10.3390/biology13010021 ![]() |
[60] |
Ucar B (2021) Natural biomaterials in brain repair: a focus on collagen. Neurochem Int 146: 105033. https://doi.org/10.1016/j.neuint.2021.105033 ![]() |
[61] |
Kim BS, Kim JU, Lee J, et al. (2024) Decellularized brain extracellular matrix based NGF-releasing cryogel for brain tissue engineering in traumatic brain injury. J Control Release 368: 140-156. https://doi.org/10.1016/j.jconrel.2024.02.017 ![]() |
[62] |
Diaz MD, Kandell RM, Wu JR, et al. (2023) Infusible extracellular matrix biomaterial promotes vascular integrity and modulates the inflammatory response in acute traumatic brain injury. Adv Healthc Mater 12: e2300782. https://doi.org/10.1002/adhm.202300782 ![]() |
[63] | Xiong Y, Mahmood A, Chopp M (2010) Angiogenesis, neurogenesis and brain recovery of function following injury. Curr Opin Investig Drugs 11: 298-308. |
[64] |
Ying C, Zhang J, Zhang H, et al. (2023) Stem cells in central nervous system diseases: Promising therapeutic strategies. Exp Neurol 369: 114543. https://doi.org/10.1016/j.expneurol.2023.114543 ![]() |
[65] | Adugna DG, Aragie H, Kibret AA, et al. (2022) Therapeutic application of stem cells in the repair of traumatic brain injury. Stem Cells Cloning 15: 53-61. https://doi.org/10.2147/SCCAA.S369577 |
[66] |
de Kanter AJ, Jongsma KR, Verhaar MC, et al. (2023) The ethical implications of tissue engineering for regenerative purposes: a systematic review. Tissue Eng Part B Rev 29: 167-187. https://doi.org/10.1089/ten.teb.2022.0033 ![]() |
[67] |
Hoang DM, Pham PT, Bach TQ, et al. (2022) Stem cell-based therapy for human diseases. Signal Transduct Target Ther 7: 272. https://doi.org/10.1038/s41392-022-01134-4 ![]() |
[68] |
Tan F, Li X, Wang Z, et al. (2024) Clinical applications of stem cell-derived exosomes. Signal Transduct Target Ther 9: 17. https://doi.org/10.1038/s41392-023-01704-0 ![]() |
[69] | Moghassemi S, Dadashzadeh A, Sousa MJ, et al. (2024) Extracellular vesicles in nanomedicine and regenerative medicine: a review over the last decade. Bioact Mater 36: 126-156. https://doi.org/10.1016/j.bioactmat.2024.02.021 |
[70] |
Zhong L, Wang J, Wang P, et al. (2023) Neural stem cell-derived exosomes and regeneration: cell-free therapeutic strategies for traumatic brain injury. Stem Cell Res Ther 14: 198. https://doi.org/10.1186/s13287-023-03409-1 ![]() |
[71] |
Yang Z, Liang Z, Rao J, et al. (2023) Mesenchymal stem cell-derived extracellular vesicles therapy in traumatic central nervous system diseases: a systematic review and meta-analysis. Neural Regen Res 18: 2406-2412. https://doi.org/10.4103/1673-5374.371376 ![]() |
[72] |
Cui L, Luo W, Jiang W, et al. (2022) Human umbilical cord mesenchymal stem cell-derived exosomes promote neurological function recovery in rat after traumatic brain injury by inhibiting the activation of microglia and astrocyte. Regen Ther 21: 282-287. https://doi.org/10.1016/j.reth.2022.07.005 ![]() |
[73] |
Li J, Li X, Li X, et al. (2024) Local delivery of dual stem cell-derived exosomes using an electrospun nanofibrous platform for the treatment of traumatic brain injury. ACS Appl Mater Interfaces 16: 37497-37512. https://doi.org/10.1021/acsami.4c05004 ![]() |
[74] |
Shen WB, Plachez C, Tsymbalyuk O, et al. (2016) Cell-based therapy in TBI: magnetic retention of neural stem cells in vivo. Cell Transplant 25: 1085-1099. https://doi.org/10.3727/096368915X689550 ![]() |
[75] |
Syzdykbayev M, Kazymov M, Aubakirov M, et al. (2024) A modern approach to the treatment of traumatic brain injury. Medicines 11: 10. https://doi.org/10.3390/medicines11050010 ![]() |
[76] |
Margulies S, Anderson G, Atif F, et al. (2016) Combination therapies for traumatic brain injury: retrospective considerations. J Neurotrauma 33: 101-112. https://doi.org/10.1089/neu.2014.3855 ![]() |
[77] |
Hade MD, Suire CN, Suo Z (2021) Mesenchymal stem cell-derived exosomes: applications in regenerative medicine. Cells 10: 1959. https://doi.org/10.3390/cells10081959 ![]() |
[78] |
Karnas E, Dudek P, Zuba-Surma EK (2023) Stem cell-derived extracellular vesicles as new tools in regenerative medicine-Immunomodulatory role and future perspectives. Front Immunol 14: 1120175. https://doi.org/10.3389/fimmu.2023.1120175 ![]() |
[79] |
Pan Y, Wu W, Jiang X, et al. (2023) Mesenchymal stem cell-derived exosomes in cardiovascular and cerebrovascular diseases: From mechanisms to therapy. Biomed Pharmacother 163: 114817. https://doi.org/10.1016/j.biopha.2023.114817 ![]() |
[80] |
Huang D, Shen H, Xie F, et al. (2024) Role of mesenchymal stem cell-derived exosomes in the regeneration of different tissues. J Biol Eng 18: 36. https://doi.org/10.1186/s13036-024-00431-6 ![]() |
[81] |
Zhu S, Liu X, Lu X, et al. (2024) Biomaterials and tissue engineering in traumatic brain injury: novel perspectives on promoting neural regeneration. Neural Regen Res 19: 2157-2174. https://doi.org/10.4103/1673-5374.391179 ![]() |
[82] |
Hong IS (2022) Enhancing stem cell-based therapeutic potential by combining various bioengineering technologies. Front Cell Dev Biol 10: 901661. https://doi.org/10.3389/fcell.2022.901661 ![]() |
[83] |
Suzuki H, Imajo Y, Funaba M, et al. (2023) Current Concepts of Biomaterial Scaffolds and Regenerative Therapy for Spinal Cord Injury. Int J Mol Sci 24: 2528. https://doi.org/10.3390/ijms24032528 ![]() |
[84] |
Rouleau N, Murugan NJ, Kaplan DL (2023) Functional bioengineered models of the central nervous system. Nat Rev Bioeng 1: 252-270. https://doi.org/10.1038/s44222-023-00027-7 ![]() |
[85] |
Han F, Wang J, Ding L, et al. (2020) Tissue engineering and regenerative medicine: achievements, future, and sustainability in Asia. Front Bioeng Biotechnol 8: 83. https://doi.org/10.3389/fbioe.2020.00083 ![]() |
[86] |
Tam RY, Fuehrmann T, Mitrousis N, et al. (2014) Regenerative therapies for central nervous system diseases: a biomaterials approach. Neuropsychopharmacology 39: 169-188. https://doi.org/10.1038/npp.2013.237 ![]() |
[87] |
Lu P, Ruan D, Huang M, et al. (2024) Harnessing the potential of hydrogels for advanced therapeutic applications: current achievements and future directions. Signal Transduct Target Ther 9: 166. https://doi.org/10.1038/s41392-024-01852-x ![]() |
[88] |
Grimaudo MA, Krishnakumar GS, Giusto E, et al. (2022) Bioactive injectable hydrogels for on demand molecule/cell delivery and for tissue regeneration in the central nervous system. Acta Biomater 140: 88-101. https://doi.org/10.1016/j.actbio.2021.11.038 ![]() |
[89] |
Kornev VA, Grebenik EA, Solovieva AB, et al. (2018) Hydrogel-assisted neuroregeneration approaches towards brain injury therapy: a state-of-the-art review. Comput Struct Biotechnol J 16: 488-502. https://doi.org/10.1016/j.csbj.2018.10.011 ![]() |
[90] |
Jia Z, Zeng H, Ye X, et al. (2023) Hydrogel-based treatments for spinal cord injuries. Heliyon 9: e19933. https://doi.org/10.1016/j.heliyon.2023.e19933 ![]() |
[91] | Wang R, Zhang X, Feng K, et al. (2023) Nanotechnologies meeting natural sources: engineered lipoproteins for precise brain disease theranostics. Asian J Pharm Sci 18: 100857. https://doi.org/10.1016/j.ajps.2023.100857 |
[92] |
Zhang Y, Zhang H, Zhao F, et al. (2023) Mitochondrial-targeted and ROS-responsive nanocarrier via nose-to-brain pathway for ischemic stroke treatment. Acta Pharm Sin B 13: 5107-5120. https://doi.org/10.1016/j.apsb.2023.06.011 ![]() |
[93] |
Kamath AP, Nayak PG, John J, et al. (2024) Revolutionizing neurotherapeutics: nanocarriers unveiling the potential of phytochemicals in Alzheimer's disease. Neuropharmacology 259: 110096. https://doi.org/10.1016/j.neuropharm.2024.110096 ![]() |
[94] |
Wu D, Chen Q, Chen X, et al. (2023) The blood-brain barrier: structure, regulation, and drug delivery. Signal Transduct Target Ther 8: 217. https://doi.org/10.1038/s41392-023-01481-w ![]() |
[95] |
Pound P, Ritskes-Hoitinga M (2018) Is it possible to overcome issues of external validity in preclinical animal research? Why most animal models are bound to fail. J Transl Med 16: 304. https://doi.org/10.1186/s12967-018-1678-1 ![]() |
[96] |
Van Norman GA (2019) Limitations of animal studies for predicting toxicity in clinical trials: is it time to rethink our current approach?. JACC Basic Transl Sci 4: 845-854. https://doi.org/10.1016/j.jacbts.2019.10.008 ![]() |
[97] |
Sarma S, Deka DJ, Rajak P, et al. (2023) Potential injectable hydrogels as biomaterials for central nervous system injury: a narrative review. Ibrain 9: 402-420. https://doi.org/10.1002/ibra.12137 ![]() |
[98] |
Fesharaki-Zadeh A (2022) Oxidative stress in traumatic brain injury. Int J Mol Sci 23: 13000. https://doi.org/10.3390/ijms232113000 ![]() |
[99] |
Davis CK, Vemuganti R (2021) DNA damage and repair following traumatic brain injury. Neurobiol Dis 147: 105143. https://doi.org/10.1016/j.nbd.2020.105143 ![]() |
[100] |
Ismail H, Shakkour Z, Tabet M, et al. (2020) Traumatic brain injury: oxidative stress and novel anti-oxidants such as mitoquinone and edaravone. Antioxidants 9: 943. https://doi.org/10.3390/antiox9100943 ![]() |
[101] |
Thapak P, Gomez-Pinilla F (2024) The bioenergetics of traumatic brain injury and its long-term impact for brain plasticity and function. Pharmacol Res 208: 107389. https://doi.org/10.1016/j.phrs.2024.107389 ![]() |
[102] |
Simpson DSA, Oliver PL (2020) ROS generation in microglia: understanding oxidative stress and inflammation in neurodegenerative disease. Antioxidants 9: 743. https://doi.org/10.3390/antiox9080743 ![]() |
[103] |
Andres CMC, Perez de la Lastra JM, Juan CA, et al. (2022) The role of reactive species on innate immunity. Vaccines 10: 1735. https://doi.org/10.3390/vaccines10101735 ![]() |
[104] |
Dmytriv TR, Duve KV, Storey KB, et al. (2024) Vicious cycle of oxidative stress and neuroinflammation in pathophysiology of chronic vascular encephalopathy. Front Physiol 15: 1443604. https://doi.org/10.3389/fphys.2024.1443604 ![]() |
[105] |
Massaad CA, Klann E (2011) Reactive oxygen species in the regulation of synaptic plasticity and memory. Antioxid Redox Signal 14: 2013-2054. https://doi.org/10.1089/ars.2010.3208 ![]() |
[106] | Beckhauser TF, Francis-Oliveira J, De Pasquale R (2016) Reactive oxygen species: physiological and physiopathological effects on synaptic plasticity. J Exp Neurosci 10: 23-48. https://doi.org/10.4137/JEN.S39887 |
[107] |
Polyak E, Ostrovsky J, Peng M, et al. (2018) N-acetylcysteine and vitamin E rescue animal longevity and cellular oxidative stress in pre-clinical models of mitochondrial complex I disease. Mol Genet Metab 123: 449-462. https://doi.org/10.1016/j.ymgme.2018.02.013 ![]() |
[108] |
Kurutas EB (2016) The importance of antioxidants which play the role in cellular response against oxidative/nitrosative stress: current state. Nutr J 15: 71. https://doi.org/10.1186/s12937-016-0186-5 ![]() |
[109] |
Jomova K, Alomar SY, Alwasel SH, et al. (2024) Several lines of antioxidant defense against oxidative stress: antioxidant enzymes, nanomaterials with multiple enzyme-mimicking activities, and low-molecular-weight antioxidants. Arch Toxicol 98: 1323-1367. https://doi.org/10.1007/s00204-024-03696-4 ![]() |
[110] |
Pandey PK, Sharma AK, Gupta U (2016) Blood brain barrier: an overview on strategies in drug delivery, realistic in vitro modeling and in vivo live tracking. Tissue Barriers 4: e1129476. https://doi.org/10.1080/21688370.2015.1129476 ![]() |
[111] |
Teleanu RI, Preda MD, Niculescu AG, et al. (2022) Current strategies to enhance delivery of drugs across the blood-brain barrier. Pharmaceutics 14: 987. https://doi.org/10.3390/pharmaceutics14050987 ![]() |
[112] | Upadhyay RK (2014) Drug delivery systems, CNS protection, and the blood brain barrier. Biomed Res Int 2014: 869269. https://doi.org/10.1155/2014/869269 |
[113] |
Ji P, Xu Q, Li J, et al. (2024) Advances in nanoparticle-based therapeutics for ischemic stroke: Enhancing drug delivery and efficacy. Biomed Pharmacother 180: 117564. https://doi.org/10.1016/j.biopha.2024.117564 ![]() |
[114] |
Song YH, De R, Lee KT (2023) Emerging strategies to fabricate polymeric nanocarriers for enhanced drug delivery across blood-brain barrier: an overview. Adv Colloid Interface Sci 320: 103008. https://doi.org/10.1016/j.cis.2023.103008 ![]() |
[115] |
Nguyen TT, Dung Nguyen TT, Vo TK, et al. (2021) Nanotechnology-based drug delivery for central nervous system disorders. Biomed Pharmacother 143: 112117. https://doi.org/10.1016/j.biopha.2021.112117 ![]() |
[116] |
Xie J, Shen Z, Anraku Y, et al. (2019) Nanomaterial-based blood-brain-barrier (BBB) crossing strategies. Biomaterials 224: 119491. https://doi.org/10.1016/j.biomaterials.2019.119491 ![]() |
[117] |
Zou T, Jiang S, Yi B, et al. (2022) Gelatin methacrylate hydrogel loaded with brain-derived neurotrophic factor enhances small molecule-induced neurogenic differentiation of stem cells from apical papilla. J Biomed Mater Res A 110: 623-634. https://doi.org/10.1002/jbm.a.37315 ![]() |
[118] |
Cruz EM, Machado LS, Zamproni LN, et al. (2023) A gelatin methacrylate-based hydrogel as a potential bioink for 3D bioprinting and neuronal differentiation. Pharmaceutics 15: 627. https://doi.org/10.3390/pharmaceutics15020627 ![]() |
[119] |
Dai W, Wang H, Fang J, et al. (2018) Curcumin provides neuroprotection in model of traumatic brain injury via the Nrf2-ARE signaling pathway. Brain Res Bull 140: 65-71. https://doi.org/10.1016/j.brainresbull.2018.03.020 ![]() |
[120] |
Huang X, Ye Y, Zhang J, et al. (2022) Reactive oxygen species scavenging functional hydrogel delivers procyanidins for the treatment of traumatic brain injury in mice. ACS Appl Mater Interfaces 14: 33756-33767. https://doi.org/10.1021/acsami.2c04930 ![]() |
[121] |
Chen Y, Lin J, Yan W (2022) A prosperous application of hydrogels with extracellular vesicles release for traumatic brain injury. Front Neurol 13: 908468. https://doi.org/10.3389/fneur.2022.908468 ![]() |
[122] |
Aurand ER, Lampe KJ, Bjugstad KB (2012) Defining and designing polymers and hydrogels for neural tissue engineering. Neurosci Res 72: 199-213. https://doi.org/10.1016/j.neures.2011.12.005 ![]() |
[123] |
Politron-Zepeda GA, Fletes-Vargas G, Rodriguez-Rodriguez R (2024) Injectable hydrogels for nervous tissue repair-a brief review. Gels 10: 190. https://doi.org/10.3390/gels10030190 ![]() |
[124] |
Hasanzadeh E, Seifalian A, Mellati A, et al. (2023) Injectable hydrogels in central nervous system: Unique and novel platforms for promoting extracellular matrix remodeling and tissue engineering. Mater Today Bio 20: 100614. https://doi.org/10.1016/j.mtbio.2023.100614 ![]() |
[125] |
Li S, Xu J, Qian Y, et al. (2024) Hydrogel in the treatment of traumatic brain injury. Biomater Res 28: 0085. https://doi.org/10.34133/bmr.0085 ![]() |
[126] |
Lou S, Gong D, Yang M, et al. (2024) Curcumin improves neurogenesis in Alzheimer's disease mice via the upregulation of wnt/beta-catenin and BDNF. Int J Mol Sci 25: 5123. https://doi.org/10.3390/ijms25105123 ![]() |
[127] |
Qian F, Han Y, Han Z, et al. (2021) In situ implantable, post-trauma microenvironment-responsive, ROS depletion hydrogels for the treatment of traumatic brain injury. Biomaterials 270: 120675. https://doi.org/10.1016/j.biomaterials.2021.120675 ![]() |
[128] |
Jiang Y, Kang Y, Liu J, et al. (2022) Nanomaterials alleviating redox stress in neurological diseases: mechanisms and applications. J Nanobiotechnology 20: 265. https://doi.org/10.1186/s12951-022-01434-5 ![]() |
[129] |
Mu X, He H, Wang J, et al. (2019) Carbogenic nanozyme with ultrahigh reactive nitrogen species selectivity for traumatic brain injury. Nano Lett 19: 4527-4534. https://doi.org/10.1021/acs.nanolett.9b01333 ![]() |
[130] |
Wu J, Wang X, Wang Q, et al. (2019) Nanomaterials with enzyme-like characteristics (nanozymes): next-generation artificial enzymes (II). Chem Soc Rev 48: 1004-1076. https://doi.org/10.1039/C8CS00457A ![]() |
[131] |
Yang J, Zhang R, Zhao H, et al. (2022) Bioinspired copper single-atom nanozyme as a superoxide dismutase-like antioxidant for sepsis treatment. Exploration 2: 20210267. https://doi.org/10.1002/EXP.20210267 ![]() |
[132] |
Zhang Y, Zhang L, Wang M, et al. (2023) The applications of nanozymes in neurological diseases: from mechanism to design. Theranostics 13: 2492-2514. https://doi.org/10.7150/thno.83370 ![]() |
[133] |
Rempe RG, Hartz AMS, Bauer B (2016) Matrix metalloproteinases in the brain and blood-brain barrier: versatile breakers and makers. J Cereb Blood Flow Metab 36: 1481-1507. https://doi.org/10.1177/0271678X16655551 ![]() |
[134] |
Lakhan SE, Kirchgessner A, Tepper D, et al. (2018) Corrigendum: matrix metalloproteinases and blood-brain barrier disruption in acute ischemic stroke. Front Neurol 9: 202. https://doi.org/10.3389/fneur.2018.00202 ![]() |
[135] |
Ji Y, Gao Q, Ma Y, et al. (2023) An MMP-9 exclusive neutralizing antibody attenuates blood-brain barrier breakdown in mice with stroke and reduces stroke patient-derived MMP-9 activity. Pharmacol Res 190: 106720. https://doi.org/10.1016/j.phrs.2023.106720 ![]() |
[136] |
Zhu FD, Hu YJ, Yu L, et al. (2021) Nanoparticles: a hope for the treatment of inflammation in CNS. Front Pharmacol 12: 683935. https://doi.org/10.3389/fphar.2021.683935 ![]() |
[137] |
Zhang K, Tu M, Gao W, et al. (2019) Hollow prussian blue nanozymes drive neuroprotection against ischemic stroke via attenuating oxidative stress, counteracting inflammation, and suppressing cell apoptosis. Nano Lett 19: 2812-2823. https://doi.org/10.1021/acs.nanolett.8b04729 ![]() |
[138] |
Zhao H, Zhang R, Yan X, et al. (2021) Superoxide dismutase nanozymes: an emerging star for anti-oxidation. J Mater Chem B 9: 6939-6957. https://doi.org/10.1039/D1TB00720C ![]() |
[139] |
Zheng G, Yu W, Xu Z, et al. (2024) Neuroimmune modulating and energy supporting nanozyme-mimic scaffold synergistically promotes axon regeneration after spinal cord injury. J Nanobiotechnology 22: 399. https://doi.org/10.1186/s12951-024-02594-2 ![]() |
[140] |
Tian R, Xu J, Luo Q, et al. (2020) Rational design and biological application of antioxidant nanozymes. Front Chem 8: 831. https://doi.org/10.3389/fchem.2020.00831 ![]() |
[141] |
Gao W, He J, Chen L, et al. (2023) Deciphering the catalytic mechanism of superoxide dismutase activity of carbon dot nanozyme. Nat Commun 14: 160. https://doi.org/10.1038/s41467-023-35828-2 ![]() |
[142] |
Zhang W, Sigdel G, Mintz KJ, et al. (2021) Carbon dots: a future blood-brain barrier penetrating nanomedicine and drug nanocarrier. Int J Nanomedicine 16: 5003-5016. https://doi.org/10.2147/IJN.S318732 ![]() |
[143] |
Seven ES, Seven YB, Zhou Y, et al. (2021) Crossing the blood-brain barrier with carbon dots: uptake mechanism and in vivo cargo delivery. Nanoscale Adv 3: 3942-3953. https://doi.org/10.1039/D1NA00145K ![]() |
[144] |
Liu Y, Li J, Guo H, et al. (2024) Nanomaterials for stroke diagnosis and treatment. iScience 27: 111112. https://doi.org/10.1016/j.isci.2024.111112 ![]() |
[145] |
Wu S, FitzGerald KT, Giordano J (2018) On the viability and potential value of stem cells for repair and treatment of central neurotrauma: overview and speculations. Front Neurol 9: 602. https://doi.org/10.3389/fneur.2018.00602 ![]() |
[146] |
Moeinabadi-Bidgoli K, Babajani A, Yazdanpanah G, et al. (2021) Translational insights into stem cell preconditioning: from molecular mechanisms to preclinical applications. Biomed Pharmacother 142: 112026. https://doi.org/10.1016/j.biopha.2021.112026 ![]() |
[147] |
Mousaei Ghasroldasht M, Seok J, Park HS, et al. (2022) Stem cell therapy: from idea to clinical practice. Int J Mol Sci 23: 2850. https://doi.org/10.3390/ijms23052850 ![]() |
[148] | Aazmi A, Zhang D, Mazzaglia C, et al. (2024) Biofabrication methods for reconstructing extracellular matrix mimetics. Bioact Mater 31: 475-496. https://doi.org/10.1016/j.bioactmat.2023.08.018 |
[149] |
Ansari MJ, Rajendran RR, Mohanto S, et al. (2022) Poly(N-isopropylacrylamide)-based hydrogels for biomedical applications: a review of the state-of-the-art. Gels 8: 454. https://doi.org/10.3390/gels8070454 ![]() |
[150] |
Bashir MH, Korany NS, Farag DBE, et al. (2023) Polymeric nanocomposite hydrogel scaffolds in craniofacial bone regeneration: a comprehensive review. Biomolecules 13: 205. https://doi.org/10.3390/biom13020205 ![]() |
[151] |
Omidian H, Chowdhury SD, Cubeddu LX (2024) Hydrogels for neural regeneration: exploring new horizons. Materials 17: 3472. https://doi.org/10.3390/ma17143472 ![]() |
[152] |
Wilson KL, Perez SCL, Naffaa MM, et al. (2022) Stoichiometric post-modification of hydrogel microparticles dictates neural stem cell fate in microporous annealed particle scaffolds. Adv Mater 34: e2201921. https://doi.org/10.1002/adma.202201921 ![]() |
[153] |
Bellotti E, Schilling AL, Little SR, et al. (2021) Injectable thermoresponsive hydrogels as drug delivery system for the treatment of central nervous system disorders: a review. J Control Release 329: 16-35. https://doi.org/10.1016/j.jconrel.2020.11.049 ![]() |
[154] |
Fan R, Cheng Y, Wang R, et al. (2022) Thermosensitive hydrogels and advances in their application in disease therapy. Polymers 14: 2379. https://doi.org/10.3390/polym14122379 ![]() |
[155] |
Zhang K, Xue K, Loh XJ (2021) Thermo-responsive hydrogels: from recent progress to biomedical applications. Gels 7: 77. https://doi.org/10.3390/gels7030077 ![]() |
[156] |
Cao H, Duan L, Zhang Y, et al. (2021) Current hydrogel advances in physicochemical and biological response-driven biomedical application diversity. Signal Transduct Target Ther 6: 426. https://doi.org/10.1038/s41392-021-00830-x ![]() |
[157] |
Rana MM, De la Hoz Siegler H (2024) Evolution of hybrid hydrogels: next-generation biomaterials for drug delivery and tissue engineering. Gels 10: 216. https://doi.org/10.3390/gels10040216 ![]() |
[158] |
Vasile C, Pamfil D, Stoleru E, et al. (2020) New developments in medical applications of hybrid hydrogels containing natural polymers. Molecules 25: 1539. https://doi.org/10.3390/molecules25071539 ![]() |
[159] |
Tripathi AS, Zaki MEA, Al-Hussain SA, et al. (2023) Material matters: exploring the interplay between natural biomaterials and host immune system. Front Immunol 14: 1269960. https://doi.org/10.3389/fimmu.2023.1269960 ![]() |
[160] |
Brovold M, Almeida JI, Pla-Palacin I, et al. (2018) Naturally-derived biomaterials for tissue engineering applications. Adv Exp Med Biol 1077: 421-449. https://doi.org/10.1007/978-981-13-0947-2_23 ![]() |
[161] | Liu S, Yu JM, Gan YC, et al. (2023) Biomimetic natural biomaterials for tissue engineering and regenerative medicine: new biosynthesis methods, recent advances, and emerging applications. Mil Med Res 10: 16. https://doi.org/10.1186/s40779-023-00448-w |
[162] |
Revete A, Aparicio A, Cisterna BA, et al. (2022) Advancements in the use of hydrogels for regenerative medicine: properties and biomedical applications. Int J Biomater 2022: 3606765. https://doi.org/10.1155/2022/3606765 ![]() |
[163] | Assuncao-Silva RC, Gomes ED, Sousa N, et al. (2015) Hydrogels and cell based therapies in spinal cord injury regeneration. Stem Cells Int 2015: 948040. https://doi.org/10.1155/2015/948040 |
[164] |
Li Z, Fan Z, Xu Y, et al. (2016) pH-sensitive and thermosensitive hydrogels as stem-cell carriers for cardiac therapy. ACS Appl Mater Interfaces 8: 10752-10760. https://doi.org/10.1021/acsami.6b01374 ![]() |
[165] |
Xu X, Liu Y, Fu W, et al. (2020) Poly(N-isopropylacrylamide)-based thermoresponsive composite hydrogels for biomedical applications. Polymers 12: 580. https://doi.org/10.3390/polym12030580 ![]() |
[166] |
Nagase K (2021) Thermoresponsive interfaces obtained using poly(N-isopropylacrylamide)-based copolymer for bioseparation and tissue engineering applications. Adv Colloid Interface Sci 295: 102487. https://doi.org/10.1016/j.cis.2021.102487 ![]() |
[167] |
Li Y, Yang L, Hu F, et al. (2022) Novel thermosensitive hydrogel promotes spinal cord repair by regulating mitochondrial function. ACS Appl Mater Interfaces 14: 25155-25172. https://doi.org/10.1021/acsami.2c04341 ![]() |
[168] |
Hu B, Gao J, Lu Y, et al. (2023) Applications of degradable hydrogels in novel approaches to disease treatment and new modes of drug delivery. Pharmaceutics 15: 2370. https://doi.org/10.3390/pharmaceutics15102370 ![]() |
[169] |
Bertsch P, Diba M, Mooney DJ, et al. (2023) Self-healing injectable hydrogels for tissue regeneration. Chem Rev 123: 834-873. https://doi.org/10.1021/acs.chemrev.2c00179 ![]() |
[170] |
Thang NH, Chien TB, Cuong DX (2023) Polymer-based hydrogels applied in drug delivery: an overview. Gels 9: 523. https://doi.org/10.3390/gels9070523 ![]() |
[171] |
Zoller K, To D, Bernkop-Schnurch A (2025) Biomedical applications of functional hydrogels: Innovative developments, relevant clinical trials and advanced products. Biomaterials 312: 122718. https://doi.org/10.1016/j.biomaterials.2024.122718 ![]() |
[172] |
Xue Y, Baig R, Dong Y (2022) Recent advances of biomaterials in stem cell therapies. Nanotechnology 33: 132501. https://doi.org/10.1088/1361-6528/ac4520 ![]() |
[173] |
Huang X, He D, Pan Z, et al. (2021) Reactive-oxygen-species-scavenging nanomaterials for resolving inflammation. Mater Today Bio 11: 100124. https://doi.org/10.1016/j.mtbio.2021.100124 ![]() |
[174] |
Pai V, Singh BN, Singh AK (2024) Insights into advances and applications of biomaterials for nerve tissue injuries and neurodegenerative disorders. Macromol Biosci 24: e2400150. https://doi.org/10.1002/mabi.202400150 ![]() |
[175] |
Zhang Y, Wu D, Zhou C, et al. (2024) Engineered extracellular vesicles for tissue repair and regeneration. Burns Trauma 12: tkae062. https://doi.org/10.1093/burnst/tkae062 ![]() |
[176] |
Liu J, Han X, Zhang T, et al. (2023) Reactive oxygen species (ROS) scavenging biomaterials for anti-inflammatory diseases: from mechanism to therapy. J Hematol Oncol 16: 116. https://doi.org/10.1186/s13045-023-01512-7 ![]() |
[177] |
Shafiq M, Chen Y, Hashim R, et al. (2021) Reactive oxygen species-based biomaterials for regenerative medicine and tissue engineering applications. Front Bioeng Biotechnol 9: 821288. https://doi.org/10.3389/fbioe.2021.821288 ![]() |
[178] |
Krishani M, Shin WY, Suhaimi H, et al. (2023) Development of scaffolds from bio-based natural materials for tissue regeneration applications: a review. Gels 9: 100. https://doi.org/10.3390/gels9020100 ![]() |
[179] |
Calderone A, Latella D, Cardile D, et al. (2024) The role of neuroinflammation in shaping neuroplasticity and recovery outcomes following traumatic brain injury: a systematic review. Int J Mol Sci 25: 11708. https://doi.org/10.3390/ijms252111708 ![]() |
[180] |
Wang J, Lv C, Wei X, et al. (2024) Molecular mechanisms and therapeutic strategies for ferroptosis and cuproptosis in ischemic stroke. Brain Behav Immun Health 40: 100837. https://doi.org/10.1016/j.bbih.2024.100837 ![]() |
[181] |
Kaur H, Gogoi B, Sharma I, et al. (2024) Hydrogels as a potential biomaterial for multimodal therapeutic applications. Mol Pharm 21: 4827-4848. https://doi.org/10.1021/acs.molpharmaceut.4c00595 ![]() |
[182] |
Chen X, Huang X, Liu C, et al. (2022) Surface-fill H(2)S-releasing silk fibroin hydrogel for brain repair through the repression of neuronal pyroptosis. Acta Biomater 154: 259-274. https://doi.org/10.1016/j.actbio.2022.11.021 ![]() |
[183] |
Rodkin S, Nwosu C, Sannikov A, et al. (2023) The role of hydrogen sulfide in regulation of cell death following neurotrauma and related neurodegenerative and psychiatric diseases. Int J Mol Sci 24: 10742. https://doi.org/10.3390/ijms241310742 ![]() |
[184] |
Sun D, Liu K, Li Y, et al. (2022) Intrinsically bioactive manganese-eumelanin nanocomposites mediated antioxidation and anti-neuroinflammation for targeted theranostics of traumatic brain injury. Adv Healthc Mater 11: e2200517. https://doi.org/10.1002/adhm.202200517 ![]() |
[185] |
Liu Y, Shang W, Liu H, et al. (2022) Biomimetic manganese-eumelanin nanocomposites for combined hyperthermia-immunotherapy against prostate cancer. J Nanobiotechnology 20: 48. https://doi.org/10.1186/s12951-022-01248-5 ![]() |
[186] |
Gao F, Liang W, Chen Q, et al. (2024) A curcumin-decorated nanozyme with ROS scavenging and anti-inflammatory properties for neuroprotection. Nanomaterials 14: 389. https://doi.org/10.3390/nano14050389 ![]() |
[187] |
Bertossi R, Kurz JE, McGuire T, et al. (2024) Intravenous immunomodulatory nanoparticles prevent secondary damage after traumatic brain injury. J Neurotrauma 42: 94-106. https://doi.org/10.1089/neu.2024.0218 ![]() |
[188] |
Abou-El-Hassan H, Bernstock JD, Chalif JI, et al. (2023) Elucidating the neuroimmunology of traumatic brain injury: methodological approaches to unravel intercellular communication and function. Front Cell Neurosci 17: 1322325. https://doi.org/10.3389/fncel.2023.1322325 ![]() |
[189] |
Sun S, Shen J, Jiang J, et al. (2023) Targeting ferroptosis opens new avenues for the development of novel therapeutics. Signal Transduct Target Ther 8: 372. https://doi.org/10.1038/s41392-023-01606-1 ![]() |
[190] |
Wei Z, Yu H, Zhao H, et al. (2024) Broadening horizons: ferroptosis as a new target for traumatic brain injury. Burns Trauma 12: tkad051. https://doi.org/10.1093/burnst/tkad051 ![]() |
[191] | Kumar J, Patel T, Sugandh F, et al. (2023) Innovative approaches and therapies to enhance neuroplasticity and promote recovery in patients with neurological disorders: a narrative review. Cureus 15: e41914. https://doi.org/10.7759/cureus.41914 |
[192] |
Zhao LR, Willing A (2018) Enhancing endogenous capacity to repair a stroke-damaged brain: an evolving field for stroke research. Prog Neurobiol 163-164: 5-26. https://doi.org/10.1016/j.pneurobio.2018.01.004 ![]() |
[193] |
Rust R, Nih LR, Liberale L, et al. (2024) Brain repair mechanisms after cell therapy for stroke. Brain 147: 3286-3305. https://doi.org/10.1093/brain/awae204 ![]() |
[194] |
Massa SM, Yang T, Xie Y, et al. (2010) Small molecule BDNF mimetics activate TrkB signaling and prevent neuronal degeneration in rodents. J Clin Invest 120: 1774-1785. https://doi.org/10.1172/JCI41356 ![]() |
[195] |
Wurzelmann M, Romeika J, Sun D (2017) Therapeutic potential of brain-derived neurotrophic factor (BDNF) and a small molecular mimics of BDNF for traumatic brain injury. Neural Regen Res 12: 7-12. https://doi.org/10.4103/1673-5374.198964 ![]() |
[196] |
Atkinson E, Dickman R (2023) Growth factors and their peptide mimetics for treatment of traumatic brain injury. Bioorg Med Chem 90: 117368. https://doi.org/10.1016/j.bmc.2023.117368 ![]() |
[197] |
Mishchenko TA, Klimenko MO, Kuznetsova AI, et al. (2022) 3D-printed hyaluronic acid hydrogel scaffolds impregnated with neurotrophic factors (BDNF, GDNF) for post-traumatic brain tissue reconstruction. Front Bioeng Biotechnol 10: 895406. https://doi.org/10.3389/fbioe.2022.895406 ![]() |
[198] |
Huang WH, Ding SL, Zhao XY, et al. (2023) Collagen for neural tissue engineering: materials, strategies, and challenges. Mater Today Bio 20: 100639. https://doi.org/10.1016/j.mtbio.2023.100639 ![]() |
[199] |
Zhang J, Liu X, Ma K, et al. (2021) Collagen/heparin scaffold combined with vascular endothelial growth factor promotes the repair of neurological function in rats with traumatic brain injury. Biomater Sci 9: 745-764. https://doi.org/10.1039/C9BM01446B ![]() |
[200] | Ma X, Agas A, Siddiqui Z, et al. (2020) Angiogenic peptide hydrogels for treatment of traumatic brain injury. Bioact Mater 5: 124-132. https://doi.org/10.1016/j.bioactmat.2020.01.005 |
[201] |
Binetruy-Tournaire R, Demangel C, Malavaud B, et al. (2000) Identification of a peptide blocking vascular endothelial growth factor (VEGF)-mediated angiogenesis. EMBO J 19: 1525-1533. https://doi.org/10.1093/emboj/19.7.1525 ![]() |
[202] |
Ma S, Zhou J, Huang T, et al. (2021) Sodium alginate/collagen/stromal cell-derived factor-1 neural scaffold loaded with BMSCs promotes neurological function recovery after traumatic brain injury. Acta Biomater 131: 185-197. https://doi.org/10.1016/j.actbio.2021.06.038 ![]() |
[203] |
Ling L, Hou J, Liu D, et al. (2022) Important role of the SDF-1/CXCR4 axis in the homing of systemically transplanted human amnion-derived mesenchymal stem cells (hAD-MSCs) to ovaries in rats with chemotherapy-induced premature ovarian insufficiency (POI). Stem Cell Res Ther 13: 79. https://doi.org/10.1186/s13287-022-02759-6 ![]() |
[204] |
Zhang J, Cheng T, Chen Y, et al. (2020) A chitosan-based thermosensitive scaffold loaded with bone marrow-derived mesenchymal stem cells promotes motor function recovery in spinal cord injured mice. Biomed Mater 15: 035020. https://doi.org/10.1088/1748-605X/ab785f ![]() |
[205] |
Bjorklund GR, Anderson TR, Stabenfeldt SE (2021) Recent advances in stem cell therapies to address neuroinflammation, stem cell survival, and the need for rehabilitative therapies to treat traumatic brain injuries. Int J Mol Sci 22: 1978. https://doi.org/10.3390/ijms22041978 ![]() |
[206] |
Liu X, Wu C, Zhang Y, et al. (2023) Hyaluronan-based hydrogel integrating exosomes for traumatic brain injury repair by promoting angiogenesis and neurogenesis. Carbohydr Polym 306: 120578. https://doi.org/10.1016/j.carbpol.2023.120578 ![]() |
[207] |
Li M, Jiang Y, Hou Q, et al. (2022) Potential pre-activation strategies for improving therapeutic efficacy of mesenchymal stem cells: current status and future prospects. Stem Cell Res Ther 13: 146. https://doi.org/10.1186/s13287-022-02822-2 ![]() |
[208] | Xiu G, Li X, Yin Y, et al. (2020) SDF-1/CXCR4 augments the therapeutic effect of bone marrow mesenchymal stem cells in the treatment of lipopolysaccharide-induced liver injury by promoting their migration through PI3K/Akt signaling pathway. Cell Transplant 29: 0963689720929992. https://doi.org/10.1177/0963689720929992 |
[209] |
Petit I, Jin D, Rafii S (2007) The SDF-1-CXCR4 signaling pathway: a molecular hub modulating neo-angiogenesis. Trends Immunol 28: 299-307. https://doi.org/10.1016/j.it.2007.05.007 ![]() |
[210] |
Cheng X, Wang H, Zhang X, et al. (2017) The role of SDF-1/CXCR4/CXCR7 in neuronal regeneration after cerebral ischemia. Front Neurosci 11: 590. https://doi.org/10.3389/fnins.2017.00590 ![]() |
[211] |
Li X, Duan L, Kong M, et al. (2022) Applications and mechanisms of stimuli-responsive hydrogels in traumatic brain injury. Gels 8: 482. https://doi.org/10.3390/gels8080482 ![]() |
[212] |
Sun Z, Zhu D, Zhao H, et al. (2023) Recent advance in bioactive hydrogels for repairing spinal cord injury: material design, biofunctional regulation, and applications. J Nanobiotechnology 21: 238. https://doi.org/10.1186/s12951-023-01996-y ![]() |
[213] |
Deng H, Wang J, An R (2023) Hyaluronic acid-based hydrogels: as an exosome delivery system in bone regeneration. Front Pharmacol 14: 1131001. https://doi.org/10.3389/fphar.2023.1131001 ![]() |
[214] |
Hu JC, Zheng CX, Sui BD, et al. (2022) Mesenchymal stem cell-derived exosomes: a novel and potential remedy for cutaneous wound healing and regeneration. World J Stem Cells 14: 318-329. https://doi.org/10.4252/wjsc.v14.i5.318 ![]() |
[215] |
Kou M, Huang L, Yang J, et al. (2022) Mesenchymal stem cell-derived extracellular vesicles for immunomodulation and regeneration: a next generation therapeutic tool?. Cell Death Dis 13: 580. https://doi.org/10.1038/s41419-022-05034-x ![]() |
[216] | Essola JM, Zhang M, Yang H, et al. (2024) Exosome regulation of immune response mechanism: Pros and cons in immunotherapy. Bioact Mater 32: 124-146. https://doi.org/10.1016/j.bioactmat.2023.09.018 |
[217] |
Zou Y, Liao L, Dai J, et al. (2023) Mesenchymal stem cell-derived extracellular vesicles/exosome: a promising therapeutic strategy for intracerebral hemorrhage. Regen Ther 22: 181-190. https://doi.org/10.1016/j.reth.2023.01.006 ![]() |
[218] | Fan MH, Pi JK, Zou CY, et al. (2024) Hydrogel-exosome system in tissue engineering: a promising therapeutic strategy. Bioact Mater 38: 1-30. https://doi.org/10.1016/j.bioactmat.2024.04.007 |
[219] |
Oyarce K, Cepeda MY, Lagos R, et al. (2022) Neuroprotective and neurotoxic effects of glial-derived exosomes. Front Cell Neurosci 16: 920686. https://doi.org/10.3389/fncel.2022.920686 ![]() |
[220] |
Zhao S, Sheng S, Wang Y, et al. (2021) Astrocyte-derived extracellular vesicles: a double-edged sword in central nervous system disorders. Neurosci Biobehav Rev 125: 148-159. https://doi.org/10.1016/j.neubiorev.2021.02.027 ![]() |
[221] |
Wan T, Huang Y, Gao X, et al. (2022) Microglia polarization: a novel target of exosome for stroke treatment. Front Cell Dev Biol 10: 842320. https://doi.org/10.3389/fcell.2022.842320 ![]() |
[222] | Lee S, Choi E, Cha MJ, et al. (2015) Cell adhesion and long-term survival of transplanted mesenchymal stem cells: a prerequisite for cell therapy. Oxid Med Cell Longev 2015: 632902. https://doi.org/10.1155/2015/632902 |
[223] |
Alagesan S, Brady J, Byrnes D, et al. (2022) Enhancement strategies for mesenchymal stem cells and related therapies. Stem Cell Res Ther 13: 75. https://doi.org/10.1186/s13287-022-02747-w ![]() |
[224] |
Khan NA, Asim M, El-Menyar A, et al. (2022) The evolving role of extracellular vesicles (exosomes) as biomarkers in traumatic brain injury: clinical perspectives and therapeutic implications. Front Aging Neurosci 14: 933434. https://doi.org/10.3389/fnagi.2022.933434 ![]() |
[225] |
Chen Y, Li J, Ma B, et al. (2020) MSC-derived exosomes promote recovery from traumatic brain injury via microglia/macrophages in rat. Aging 12: 18274-18296. https://doi.org/10.18632/aging.103692 ![]() |
[226] |
Selvam S, Midhun BT, Bhowmick T, et al. (2023) Bioprinting of exosomes: prospects and challenges for clinical applications. Int J Bioprint 9: 690. https://doi.org/10.18063/ijb.690 ![]() |
[227] |
Li Q, Yu H, Zhao F, et al. (2023) 3D printing of microenvironment-specific bioinspired and exosome-reinforced hydrogel scaffolds for efficient cartilage and subchondral bone regeneration. Adv Sci 10: e2303650. https://doi.org/10.1002/advs.202303650 ![]() |
[228] | Chen C, Chang ZH, Yao B, et al. (2024) 3D printing of interferon gamma-preconditioned NSC-derived exosomes/collagen/chitosan biological scaffolds for neurological recovery after TBI. Bioact Mater 39: 375-391. https://doi.org/10.1016/j.bioactmat.2024.05.026 |
[229] |
Xiong Y, Mahmood A, Chopp M (2013) Animal models of traumatic brain injury. Nat Rev Neurosci 14: 128-142. https://doi.org/10.1038/nrn3407 ![]() |
[230] |
Zhao Q, Zhang J, Li H, et al. (2023) Models of traumatic brain injury-highlights and drawbacks. Front Neurol 14: 1151660. https://doi.org/10.3389/fneur.2023.1151660 ![]() |
[231] |
Fesharaki-Zadeh A, Datta D (2024) An overview of preclinical models of traumatic brain injury (TBI): relevance to pathophysiological mechanisms. Front Cell Neurosci 18: 1371213. https://doi.org/10.3389/fncel.2024.1371213 ![]() |
[232] |
Marklund N, Hillered L (2011) Animal modelling of traumatic brain injury in preclinical drug development: where do we go from here?. Br J Pharmacol 164: 1207-1229. https://doi.org/10.1111/j.1476-5381.2010.01163.x ![]() |
[233] |
Kinder HA, Baker EW, West FD (2019) The pig as a preclinical traumatic brain injury model: current models, functional outcome measures, and translational detection strategies. Neural Regen Res 14: 413-424. https://doi.org/10.4103/1673-5374.245334 ![]() |
[234] |
Netzley AH, Pelled G (2023) The pig as a translational animal model for biobehavioral and neurotrauma research. Biomedicines 11: 2165. https://doi.org/10.3390/biomedicines11082165 ![]() |
[235] |
O'Donnell JC, Petrov D (2024) Porcine models of neurotrauma and neurological disorders. Biomedicines 12: 245. https://doi.org/10.3390/books978-3-7258-0506-8 ![]() |
[236] |
Xiong Y, Mahmood A, Chopp M (2018) Current understanding of neuroinflammation after traumatic brain injury and cell-based therapeutic opportunities. Chin J Traumatol 21: 137-151. https://doi.org/10.1016/j.cjtee.2018.02.003 ![]() |
[237] |
El Baassiri MG, Raouf Z, Badin S, et al. (2024) Dysregulated brain-gut axis in the setting of traumatic brain injury: review of mechanisms and anti-inflammatory pharmacotherapies. J Neuroinflammation 21: 124. https://doi.org/10.1186/s12974-024-03118-3 ![]() |
[238] |
Kapate N, Liao R, Sodemann RL, et al. (2024) Backpack-mediated anti-inflammatory macrophage cell therapy for the treatment of traumatic brain injury. PNAS Nexus 3: pgad434. https://doi.org/10.1093/pnasnexus/pgad434 ![]() |
[239] |
Klyachko NL, Polak R, Haney MJ, et al. (2017) Macrophages with cellular backpacks for targeted drug delivery to the brain. Biomaterials 140: 79-87. https://doi.org/10.1016/j.biomaterials.2017.06.017 ![]() |
[240] |
Nong J, Glassman PM, Myerson JW, et al. (2023) Targeted nanocarriers Co-opting pulmonary intravascular leukocytes for drug delivery to the injured brain. ACS Nano 17: 13121-13136. https://doi.org/10.1021/acsnano.2c08275 ![]() |
[241] |
Qu Y, Chu B, Li J, et al. (2024) Macrophage-biomimetic nanoplatform-based therapy for inflammation-associated diseases. Small Methods 8: e2301178. https://doi.org/10.1002/smtd.202301178 ![]() |
[242] |
Shields CWt, Evans MA, Wang LL, et al. (2020) Cellular backpacks for macrophage immunotherapy. Sci Adv 6: eaaz6579. https://doi.org/10.1126/sciadv.aaz6579 ![]() |
[243] |
Su Y, Gao J, Kaur P, et al. (2020) Neutrophils and macrophages as targets for development of nanotherapeutics in inflammatory diseases. Pharmaceutics 12: 1222. https://doi.org/10.3390/pharmaceutics12121222 ![]() |
[244] |
Savchenko IV, Zlotnikov ID, Kudryashova EV (2023) Biomimetic systems involving macrophages and their potential for targeted drug delivery. Biomimetics 8: 543. https://doi.org/10.20944/preprints202308.0872.v1 ![]() |
[245] |
Kapate N, Dunne M, Kumbhojkar N, et al. (2023) A backpack-based myeloid cell therapy for multiple sclerosis. Proc Natl Acad Sci USA 120: e2221535120. https://doi.org/10.1073/pnas.2221535120 ![]() |
[246] |
Vink R (2018) Large animal models of traumatic brain injury. J Neurosci Res 96: 527-535. https://doi.org/10.1002/jnr.24079 ![]() |
[247] |
Sorby-Adams AJ, Vink R, Turner RJ (2018) Large animal models of stroke and traumatic brain injury as translational tools. Am J Physiol Regul Integr Comp Physiol 315: R165-R190. https://doi.org/10.1152/ajpregu.00163.2017 ![]() |
[248] |
Smith DH, Kochanek PM, Rosi S, et al. (2021) Roadmap for advancing pre-clinical science in traumatic brain injury. J Neurotrauma 38: 3204-3221. https://doi.org/10.1089/neu.2021.0094 ![]() |
[249] |
Hamblin MR (2018) Photobiomodulation for traumatic brain injury and stroke. J Neurosci Res 96: 731-743. https://doi.org/10.1002/jnr.24190 ![]() |
[250] |
Nairuz T, Sangwoo C, Lee JH (2024) Photobiomodulation therapy on brain: pioneering an innovative approach to revolutionize cognitive dynamics. Cells 13: 966. https://doi.org/10.3390/cells13110966 ![]() |
[251] |
Hamblin MR (2018) Mechanisms and mitochondrial redox signaling in photobiomodulation. Photochem Photobiol 94: 199-212. https://doi.org/10.1111/php.12864 ![]() |
[252] |
Cardoso FDS, Barrett DW, Wade Z, et al. (2022) Photobiomodulation of cytochrome c oxidase by chronic transcranial laser in young and aged brains. Front Neurosci 16: 818005. https://doi.org/10.3389/fnins.2022.818005 ![]() |
[253] |
Cheng G, Kong RH, Zhang LM, et al. (2012) Mitochondria in traumatic brain injury and mitochondrial-targeted multipotential therapeutic strategies. Br J Pharmacol 167: 699-719. https://doi.org/10.1111/j.1476-5381.2012.02025.x ![]() |
[254] |
Lim L (2024) Traumatic brain injury recovery with photobiomodulation: cellular mechanisms, clinical evidence, and future potential. Cells 13: 385. https://doi.org/10.3390/cells13050385 ![]() |
[255] |
Yang J, Jiang H, Fu Q, et al. (2023) Blue light photobiomodulation induced apoptosis by increasing ROS level and regulating SOCS3 and PTEN/PI3K/AKT pathway in osteosarcoma cells. J Photochem Photobiol B 249: 112814. https://doi.org/10.1016/j.jphotobiol.2023.112814 ![]() |
[256] |
Stevens AR, Hadis M, Milward M, et al. (2023) Photobiomodulation in acute traumatic brain injury: a systematic review and meta-analysis. J Neurotrauma 40: 210-227. https://doi.org/10.1089/neu.2022.0140 ![]() |
[257] |
Stevens AR, Hadis M, Phillips A, et al. (2024) Implantable and transcutaneous photobiomodulation promote neuroregeneration and recovery of lost function after spinal cord injury. Bioeng Transl Med 9: e10674. https://doi.org/10.1002/btm2.10674 ![]() |
[258] |
Farazi N, Salehi-Pourmehr H, Farajdokht F, et al. (2024) Photobiomodulation combination therapy as a new insight in neurological disorders: a comprehensive systematic review. BMC Neurol 24: 101. https://doi.org/10.1186/s12883-024-03593-4 ![]() |
[259] |
Hennessy M, Hamblin MR (2017) Photobiomodulation and the brain: a new paradigm. J Opt 19: 013003. https://doi.org/10.1088/2040-8986/19/1/013003 ![]() |
[260] |
Cardoso FDS, Salehpour F, Coimbra NC, et al. (2022) Photobiomodulation for the treatment of neuroinflammation: a systematic review of controlled laboratory animal studies. Front Neurosci 16: 1006031. https://doi.org/10.3389/fnins.2022.1006031 ![]() |
[261] |
Rodriguez-Fernandez L, Zorzo C, Arias JL (2024) Photobiomodulation in the aging brain: a systematic review from animal models to humans. Geroscience 46: 6583-6623. https://doi.org/10.1007/s11357-024-01231-y ![]() |
[262] |
Li S, Wong TWL, Ng SSM (2024) Potential and challenges of transcranial photobiomodulation for the treatment of stroke. CNS Neurosci Ther 30: e70142. https://doi.org/10.1111/cns.70142 ![]() |
[263] |
Shen Q, Guo H, Yan Y (2024) Photobiomodulation for neurodegenerative diseases: a scoping review. Int J Mol Sci 25: 1625. https://doi.org/10.3390/ijms25031625 ![]() |
[264] | Morries LD, Cassano P, Henderson TA (2015) Treatments for traumatic brain injury with emphasis on transcranial near-infrared laser phototherapy. Neuropsychiatr Dis Treat 11: 2159-2175. https://doi.org/10.2147/NDT.S65809 |
[265] |
Chao LL, Barlow C, Karimpoor M, et al. (2020) Changes in brain function and structure after self-administered home photobiomodulation treatment in a concussion case. Front Neurol 11: 952. https://doi.org/10.3389/fneur.2020.00952 ![]() |
[266] |
Wu Q, Xuan W, Ando T, et al. (2012) Low-level laser therapy for closed-head traumatic brain injury in mice: effect of different wavelengths. Lasers Surg Med 44: 218-226. https://doi.org/10.1002/lsm.22003 ![]() |
[267] |
Huang YY, Gupta A, Vecchio D, et al. (2012) Transcranial low level laser (light) therapy for traumatic brain injury. J Biophotonics 5: 827-837. https://doi.org/10.1002/jbio.201200077 ![]() |
[268] |
Hamblin MR (2016) Shining light on the head: photobiomodulation for brain disorders. BBA Clin 6: 113-124. https://doi.org/10.1016/j.bbacli.2016.09.002 ![]() |
[269] |
Thunshelle C, Hamblin MR (2016) Transcranial low-level laser (light) therapy for brain injury. Photomed Laser Surg 34: 587-598. https://doi.org/10.1089/pho.2015.4051 ![]() |
[270] | Lin H, Li D, Zhu J, et al. (2024) Transcranial photobiomodulation for brain diseases: review of animal and human studies including mechanisms and emerging trends. Neurophotonics 11: 010601. https://doi.org/10.1117/1.NPh.11.1.010601 |
[271] |
Hassan MP, Abdollahifar MA, Aliaghaei A, et al. (2021) Photobiomodulation therapy improved functional recovery and overexpression of interleukins-10 after contusion spinal cord injury in rats. J Chem Neuroanat 117: 102010. https://doi.org/10.1016/j.jchemneu.2021.102010 ![]() |
[272] |
Davies DJ, Hadis M, Di Pietro V, et al. (2022) Photobiomodulation reduces hippocampal apoptotic cell death and produces a Raman spectroscopic “signature”. PLoS One 17: e0264533. https://doi.org/10.1371/journal.pone.0264533 ![]() |
[273] |
Serrage H, Heiskanen V, Palin WM, et al. (2019) Under the spotlight: mechanisms of photobiomodulation concentrating on blue and green light. Photochem Photobiol Sci 18: 1877-1909. https://doi.org/10.1039/c9pp00089e ![]() |
[274] |
Dompe C, Moncrieff L, Matys J, et al. (2020) Photobiomodulation-underlying mechanism and clinical applications. J Clin Med 9: 1724. https://doi.org/10.3390/jcm9061724 ![]() |
[275] | Blivet G, Meunier J, Roman FJ, et al. (2018) Neuroprotective effect of a new photobiomodulation technique against Abeta(25–35) peptide-induced toxicity in mice: novel hypothesis for therapeutic approach of Alzheimer's disease suggested. Alzheimers Dement 4: 54-63. https://doi.org/10.1016/j.trci.2017.12.003 |
[276] |
Deshetty UM, Periyasamy P (2023) Potential biomarkers in experimental animal models for traumatic brain injury. J Clin Med 12: 3923. https://doi.org/10.3390/jcm12123923 ![]() |
[277] |
Peterson TC, Maass WR, Anderson JR, et al. (2015) A behavioral and histological comparison of fluid percussion injury and controlled cortical impact injury to the rat sensorimotor cortex. Behav Brain Res 294: 254-263. https://doi.org/10.1016/j.bbr.2015.08.007 ![]() |
[278] |
Osier ND, Dixon CE (2016) The controlled cortical impact model: applications, considerations for researchers, and future directions. Front Neurol 7: 134. https://doi.org/10.3389/fneur.2016.00134 ![]() |
[279] |
Wahab RA, Neuberger EJ, Lyeth BG, et al. (2015) Fluid percussion injury device for the precise control of injury parameters. J Neurosci Methods 248: 16-26. https://doi.org/10.1016/j.jneumeth.2015.03.010 ![]() |
[280] | Mesfin FB, Gupta N, Hays Shapshak A, et al. (2025) Diffuse Axonal Injury. Treasure Island (FL): StatPearls. |
[281] | Su E, Bell M (2016) Diffuse axonal injury. Translational Research in Traumatic Brain Injury. Boca Raton (FL): . https://doi.org/10.1201/b18959-4 |
[282] |
Smith DH, Hicks R, Povlishock JT (2013) Therapy development for diffuse axonal injury. J Neurotrauma 30: 307-323. https://doi.org/10.1089/neu.2012.2825 ![]() |
[283] |
Corrigan JD, Hammond FM, Sander AM, et al. (2025) Model of care for chronic brain injury. Arch Phys Med Rehabil 106: 145-149. https://doi.org/10.1016/j.apmr.2024.08.001 ![]() |
[284] |
Albayram O, Albayram S, Mannix R (2020) Chronic traumatic encephalopathy-a blueprint for the bridge between neurological and psychiatric disorders. Transl Psychiatry 10: 424. https://doi.org/10.1038/s41398-020-01111-x ![]() |
[285] | Turner RC, Lucke-Wold BP, Logsdon AF, et al. (2015) Modeling chronic traumatic encephalopathy: the way forward for future discovery. Front Neurol 6: 223. https://doi.org/10.3389/fneur.2015.00223 |
[286] |
Lucke-Wold BP, Turner RC, Logsdon AF, et al. (2014) Linking traumatic brain injury to chronic traumatic encephalopathy: identification of potential mechanisms leading to neurofibrillary tangle development. J Neurotrauma 31: 1129-1138. https://doi.org/10.1089/neu.2013.3303 ![]() |
[287] |
Seplovich G, Bouchi Y, de Rivero Vaccari JP, et al. (2025) Inflammasome links traumatic brain injury, chronic traumatic encephalopathy, and Alzheimer's disease. Neural Regen Res 20: 1644-1664. https://doi.org/10.4103/NRR.NRR-D-24-00107 ![]() |
[288] |
Jarrahi A, Braun M, Ahluwalia M, et al. (2020) Revisiting traumatic brain injury: from molecular mechanisms to therapeutic interventions. Biomedicines 8: 389. https://doi.org/10.3390/biomedicines8100389 ![]() |
[289] | Failla MD, Wagner AK (2015) Models of posttraumatic brain injury neurorehabilitation. Brain Neurotrauma: Molecular, Neuropsychological, and Rehabilitation Aspects. Boca Raton (FL): . |
[290] |
Balakin E, Yurku K, Fomina T, et al. (2024) A systematic review of traumatic brain injury in modern rodent models: current status and future prospects. Biology 13: 813. https://doi.org/10.3390/biology13100813 ![]() |
[291] |
Ali MA, Bhuiyan MH (2021) Types of biomaterials useful in brain repair. Neurochem Int 146: 105034. https://doi.org/10.1016/j.neuint.2021.105034 ![]() |
[292] |
Hacene S, Le Friec A, Desmoulin F, et al. (2022) Present and future avenues of cell-based therapy for brain injury: the enteric nervous system as a potential cell source. Brain Pathol 32: e13105. https://doi.org/10.1111/bpa.13105 ![]() |
[293] |
Rahimi Darehbagh R, Seyedoshohadaei SA, Ramezani R, et al. (2024) Stem cell therapies for neurological disorders: current progress, challenges, and future perspectives. Eur J Med Res 29: 386. https://doi.org/10.1186/s40001-024-01987-1 ![]() |
[294] |
Haus DL, Lopez-Velazquez L, Gold EM, et al. (2016) Transplantation of human neural stem cells restores cognition in an immunodeficient rodent model of traumatic brain injury. Exp Neurol 281: 1-16. https://doi.org/10.1016/j.expneurol.2016.04.008 ![]() |
[295] |
Mattingly J, Li Y, Bihl JC, et al. (2021) The promise of exosome applications in treating central nervous system diseases. CNS Neurosci Ther 27: 1437-1445. https://doi.org/10.1111/cns.13743 ![]() |
[296] |
Willing AE, Das M, Howell M, et al. (2020) Potential of mesenchymal stem cells alone, or in combination, to treat traumatic brain injury. CNS Neurosci Ther 26: 616-627. https://doi.org/10.1111/cns.13300 ![]() |
[297] |
Ojeda-Hernandez DD, Hernandez-Sapiens MA, Reza-Zaldivar EE, et al. (2022) Exosomes and biomaterials: in search of a new therapeutic strategy for multiple sclerosis. Life 12: 1417. https://doi.org/10.3390/life12091417 ![]() |
[298] |
Nelson DW, Gilbert RJ (2021) Extracellular matrix-mimetic hydrogels for treating neural tissue injury: a focus on fibrin, hyaluronic acid, and elastin-like polypeptide hydrogels. Adv Healthc Mater 10: e2101329. https://doi.org/10.1002/adhm.202101329 ![]() |
[299] | Zheng Y, Wu G, Chen L, et al. (2021) Neuro-regenerative imidazole-functionalized GelMA hydrogel loaded with hAMSC and SDF-1alpha promote stem cell differentiation and repair focal brain injury. Bioact Mater 6: 627-637. https://doi.org/10.1016/j.bioactmat.2020.08.026 |
[300] |
Da Silva K, Kumar P, van Vuuren SF, et al. (2021) Three-dimensional printability of an ECM-based gelatin methacryloyl (GelMA) biomaterial for potential neuroregeneration. ACS Omega 6: 21368-21383. https://doi.org/10.1021/acsomega.1c01903 ![]() |
[301] |
Yan B, Zhou J, Yan F, et al. (2025) Unlocking the potential of photobiomodulation therapy for brain neurovascular coupling: The biological effects and medical applications. J Cereb Blood Flow Metab 2025: 0271678X241311695. ![]() |
[302] |
Wu H, Zheng J, Xu S, et al. (2021) Mer regulates microglial/macrophage M1/M2 polarization and alleviates neuroinflammation following traumatic brain injury. J Neuroinflammation 18: 2. https://doi.org/10.1186/s12974-020-02041-7 ![]() |