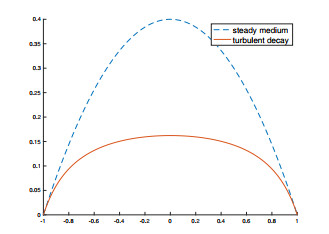
In this paper, we investigated the dynamics of a pair of VDP (Van der Pol) oscillators with direct-indirect coupling, which is described by five first-order differential equations. The system presented three types of equilibria including HSS (homogeneous steady state), IHSS (inhomogeneous steady state) and NPSS (no-pattern steady state). Employing the corresponding characteristic equations of the linearized system, we obtained the necessary conditions for the pitchfork and Hopf bifurcations of the equilibria. Further, we illustrated one-dimensional bifurcation and phase diagrams to verify theoretical results. The results show that the system exhibited two types of oscillation quenching, i.e., amplitude death (AD) for HSS equilibria and oscillation death (OD) for IHSS equilibria. In some special regions of the parameters, the system proposed multiple types of stable coexistence including HSS and IHSS equilibria, periodic orbits or quasi-periodic oscillations.
Citation: Xiaojun Huang, Zigen Song, Jian Xu. Amplitude death, oscillation death, and stable coexistence in a pair of VDP oscillators with direct–indirect coupling[J]. Electronic Research Archive, 2023, 31(11): 6964-6981. doi: 10.3934/era.2023353
[1] | Camilla Nobili . The role of boundary conditions in scaling laws for turbulent heat transport. Mathematics in Engineering, 2023, 5(1): 1-41. doi: 10.3934/mine.2023013 |
[2] | Giuseppe Maria Coclite, Lorenzo di Ruvo . On the initial-boundary value problem for a Kuramoto-Sinelshchikov type equation. Mathematics in Engineering, 2021, 3(4): 1-43. doi: 10.3934/mine.2021036 |
[3] | Gabriel B. Apolinário, Laurent Chevillard . Space-time statistics of a linear dynamical energy cascade model. Mathematics in Engineering, 2023, 5(2): 1-23. doi: 10.3934/mine.2023025 |
[4] | Antonio Vitolo . Singular elliptic equations with directional diffusion. Mathematics in Engineering, 2021, 3(3): 1-16. doi: 10.3934/mine.2021027 |
[5] | Anne-Charline Chalmin, Jean-Michel Roquejoffre . Improved bounds for reaction-diffusion propagation driven by a line of nonlocal diffusion. Mathematics in Engineering, 2021, 3(1): 1-16. doi: 10.3934/mine.2021006 |
[6] | Anoumou Attiogbe, Mouhamed Moustapha Fall, El Hadji Abdoulaye Thiam . Nonlocal diffusion of smooth sets. Mathematics in Engineering, 2022, 4(2): 1-22. doi: 10.3934/mine.2022009 |
[7] | Youchan Kim, Seungjin Ryu, Pilsoo Shin . Approximation of elliptic and parabolic equations with Dirichlet boundary conditions. Mathematics in Engineering, 2023, 5(4): 1-43. doi: 10.3934/mine.2023079 |
[8] | Massimiliano Giona, Luigi Pucci . Hyperbolic heat/mass transport and stochastic modelling - Three simple problems. Mathematics in Engineering, 2019, 1(2): 224-251. doi: 10.3934/mine.2019.2.224 |
[9] | Mouhamed Moustapha Fall, Veronica Felli, Alberto Ferrero, Alassane Niang . Asymptotic expansions and unique continuation at Dirichlet-Neumann boundary junctions for planar elliptic equations. Mathematics in Engineering, 2019, 1(1): 84-117. doi: 10.3934/Mine.2018.1.84 |
[10] | Marguerite Champion, Miguel A. Fernández, Céline Grandmont, Fabien Vergnet, Marina Vidrascu . On the analysis of a mechanically consistent model of fluid-structure-contact interaction. Mathematics in Engineering, 2024, 6(3): 425-467. doi: 10.3934/mine.2024018 |
In this paper, we investigated the dynamics of a pair of VDP (Van der Pol) oscillators with direct-indirect coupling, which is described by five first-order differential equations. The system presented three types of equilibria including HSS (homogeneous steady state), IHSS (inhomogeneous steady state) and NPSS (no-pattern steady state). Employing the corresponding characteristic equations of the linearized system, we obtained the necessary conditions for the pitchfork and Hopf bifurcations of the equilibria. Further, we illustrated one-dimensional bifurcation and phase diagrams to verify theoretical results. The results show that the system exhibited two types of oscillation quenching, i.e., amplitude death (AD) for HSS equilibria and oscillation death (OD) for IHSS equilibria. In some special regions of the parameters, the system proposed multiple types of stable coexistence including HSS and IHSS equilibria, periodic orbits or quasi-periodic oscillations.
In the last four years, a new understanding of heat diffusion in a turbulant fluid modeled by white noise has been developed. The equation for the heat diffusion and transport, with a heat source q, is
∂tθ+u⋅∇θ=κΔθ+q | (1.1) |
where θ=θ(t,x) is the temperature, κ is the diffusion constant and u=u(t,x) is the velocity field of the fluid. By turbulent fluid modeled by white noise we mean the case when, instead of considering true equations of motion of the fluid (which should also include the effect of the temperature on the motion), we assume that u is a random field, Gaussian and white in time, with covariance structure give a priori (hence the temperature is a passive scalar). In this paper we choose the following description for u:
u(t,x)=∑k∈Kσk(x)dWktdt | (1.2) |
where σk are vector fields and Wkt are independent Brownian motions on a filtered probability space (Ω,F,(Ft)t≥0,P); for simplicity, assume K is a finite set, but the case of a countable set can be studied without troubles at the price of additional summability assumptions. As expained in a number of classical or more modern works [4,5,19,20,30,34,35], which extend to SPDE the remarkable principle of Wong-Zakai [36], the correct interpretation of Eq (1.1) when u has the form (1.2) is the Stratonovich equation
dθ+∑k∈Kuk⋅∇θ∘dWkt=(κΔθ+q)dt | (1.3) |
or equivalently the Itô equation with corrector Lθ given by the second order differential operator (2.1) below:
dθ+∑k∈Kuk⋅∇θdWkt=(κΔθ+Lθ+q)dt. | (1.4) |
This is the equation we shall investigate below.
Diffusion in a white noise velocity field is a classical subject, see for instance [6,15,17,24,25,28,33]. The new approach mentioned at the beginning of the introduction started with [16], and was interpreted initially as a scaling limit, for a suitable parametrization of the coefficients σk(x) of the noise, such that in the limit the solution of Eq (1.4) converges (in a suitable topology) to the solution of the deterministic parabolic equation
∂tΘ=(κΔ+L)Θ+q | (1.5) |
where for simplicity of exposition we assume that the source q is deterministic. Assuming that also the initial temperature θ0 is deterministic, the solution Θ is the average of θ:
Θ(t,x)=E[θ(t,x)] |
where E denotes the mathematical expectation on (Ω,F,P). That the mean temperature Θ(t,x) has enhanced dissipative properties (due to L) was obviously well known, see for instance [28] Chapter 4, but the fact that in a suitable scaling limit the solution θ(t,x) was close to its average Θ(t,x) is a new information provided by [16]. Later on this result was perfectioned into quantitative estimates on the difference θ−Θ, in [11] and [12]; the present note is a continuation of these works. Let us mention the very important fact that both the scaling limit framework of [16] and the quantitative estimates extend to nonlinear problems, like the Navier-Stokes equations and others, as well as Wong-Zakai type results which motivate the Stratonovich operation, see [9,10,12,13,14,21,22,23,26,27].
As already said, the present work is a continuation of [11,12]. The main novelty, beside the fact that we work in an infinite 2D channel, is the presence of a heat source q, neglected in previous works. This detail has an important consequence, not investigated before: that the deterministic Eq (1.5) has a unique non trivial stationary solution Θst and it becomes interesting to understand whether the solution θ of the stochastic problem (1.4) is close to Θst, for large times. One of our main results, Theorem 7 below, gives sufficient conditions on the noise to have that θ is close to Θst.
In Section 2 we define precisely the problem and state the main results, including the numerical ones. In Section 3 we prove the well posedness of the equations and in Section 4 we prove the main result on the link between θ(t,x) and Θst.
Remark 1. We only focused our attention on an infinite 2d channel, to avoid the potential confusion of mixing different set-ups. However, all the results can be extended to Rd×(−1,1) and Td×(−1,1) (Td being the torus in dimension d), for both d=1,2, without any change or addition of stronger assumptions on the coefficients σk, the heat source q and the initial condition θ0. To this purpose two key remarks are the validity of Poincaré inequality in these domains as well as the embedding of W2,2 into L∞.
Consider the 2D domain D=R×(−1,1), namely an infinite channel. We write the coordinates using the notation
x=(x1,z)∈D |
because the global notation x appears very often but also the vertical coordinate z will play a basic role. Let Z be a separable Hilbert space, denote by L2(Ft0,Z) the space of square integrable random variables with values in Z, measurable with respect to Ft0. Moreover, denote by CF([0,T];Z) the space of continuous adapted processes (Xt)t∈[0,T] with values in Z such that
E[supt∈[0,T]‖Xt‖2Z]<∞ |
and by L2F(0,T;Z) the space of progressively measurable processes (Xt)t∈[0,T] with values in Z such that
E[∫T0‖Xt‖2Zdt]<∞. |
Denote by L2(D) and Wk,2(D) the usual Lebesgue and Sobolev spaces and by Wk,20(D) the closure in Wk,2(D) of smooth compact support functions. Set H=L2(D), V=W1,20(D), D(A)=W2,2(D)∩V. We denote by ⟨⋅,⋅⟩ and ‖⋅‖ the inner product and the norm in H respectively.
Assume that K is a finite set and σk∈(D(A)∩C∞b(D))2, divσk=0, k∈K (less is sufficient but we do not stress this level of generality). Define the matrix-valued function
Q(x,y)=∑k∈Kσk(x)⊗σk(y). |
If we denote by W(t,x) the vector valued random field
W(t,x)=∑k∈Kσk(x)Wkt |
(the velocity field u given by (1.2) is the distributional time derivative of W) then we see that Q(x,y) is the space-covarance of W(1,x):
Q(x,y)=E[W(1,x)⊗W(1,y)]. |
The matrix-function Q(x,x) is elliptic:
d∑i,j=1Qij(x,x)ξiξj=E[|W(t,x)⋅ξ|2]≥0 |
for all ξ=(ξ1,...,ξd)∈Rd. Consider the divergence form elliptic operator L defined as
(Lθ)(x)=12d∑i,j=1∂i(Qij(x,x)∂jθ(x)) (∗) |
for θ∈W2,2(D). Define the linear operator A:D(A)⊂H→H as
Aθ=(κΔ+L)θ |
It is the infinitesimal generator of an analytic semigroup, see Section 3 and [31], that we denote by etA, t≥0. Moreover, we denote by Vα the Hilbert space D((−A)α2), see Section 3.
Definition 2. Given θ0∈L2(F0,H) and q∈L2(0,T;H), a stochastic process
θ∈CF([0,T];H)∩L2F(0,T;V) |
is a mild solution of equation (1.4) if the following identity holds
θ(t)=etAθ0+∫t0e(t−s)Aq(s)ds−∑k∈K∫t0e(t−s)Aσk⋅∇θ(s)dWks |
for every t∈[0,T], P-a.s.
Definition 3. Let α∈R. Problem (1.4) is well posed in Vα, if for every θ0∈L2(F0,Vα) and q∈L2(0,T;Vα) there exists a unique θ mild solution of Eq (1.4) in CF([0,T];Vα)∩L2F(0,T;Vα+1). Moreover θ depends continuously on θ0 and q.
Theorem 4. Equation (1.4) is well posed in H in the sense of definition 3.
Theorem 5. Equation (1.4) is well posed in Vα for 0≤α≤2 in the sense of definition 3.
Moreover, if we assume only θ0∈L2(F0,H) and q∈L2(0,T;Vα) for some 0≤α≤2, then for every ϵ∈(0,T) we have θ|[ϵ,T]∈CF(ϵ,T;Vα)∩L2F(ϵ,T;Vα+1) and this restriction depends continuously on θ0 and q.
It is possible to get stronger regularity results adding further assumputions on the coefficients σk, see [8] for similar results in bounded domains. We do not stress these assumptions because in the following sections we need just the estimate guaranteed by the following corollary.
Corollary 6. If θ0∈L2(F0;D(A)), q(t)≡q∈D(A), then
supt∈[0,T]E[‖θ(t)‖2∞]≤C(‖q‖2D(A)+‖u0‖2D(A)) |
for some C independent from T.
In this section we state our main result about the behavior of θ(t) for large times. Assume that q is independent of time and introduce the stationary solution of Eq (1.5):
Θst:=−A−1q. |
Define ϵQ≥0 as the smallest number such that
∫∫v(x)TQ(x,y)v(y)dxdy≤ϵQ∫|v(x)|2dx | (2.1) |
for all v∈L2(D,Rd). Call C∞(θ0,q)>0 a constant such that
supt≥0E‖θ(t)‖2∞≤C∞(θ0,q). |
Theorem 7. For every ϕ∈H,
limsupt→∞ E[⟨θ(t)−Θst,ϕ⟩2]≤ϵQκ‖ϕ‖2C∞(θ0,q). |
The theorem is proved in Section 4 below. The existence of a constant C∞(θ0,q) is provided by Corollary 6 above. In order to be of interest for applications, this theorem requires two conditions:
1). that ϵQ is small.
2). that Θst is significantly affected by the noise.
In this section we discuss the first problem, the size of ϵQ. In the next section we give numerical simulations which show the great difference between the prensence or absence of noise in the shape of Θst.
Proposition 8. Assume that the family of coefficients (σk(⋅))k∈K has the following approximate orthogonality property: there exists a finite number M∈N and a partition K=K1∪...∪KM such that
⟨σk,σk′⟩=0 for all k,k′∈Ki |
for all i=1,...,M. Then
ϵQ≤Msupk∈K‖σk‖2. |
Proof.
∫∫v(x)TQ(x,y)v(y)dxdy=∑k∈K⟨σk,v⟩2=M∑i=1∑k∈Ki‖σk‖2⟨σk‖σk‖,v⟩2≤M(supk∈K‖σk‖2)‖v‖2. |
The approximate orthogonality property imposed in the previous proposition is a consequence, in examples, of the fact that the supports of elements of Ki are disjoint, for all i=1,...,M. Therefore the approximation between θ(t) and Θst is good if the coefficients σk have sufficiently disjoint supports and have sufficiently small size ‖σk‖2.
These conditions are compatible with a strong modification of the profile Θst, with respect to the case of the parabolic profile given by the solution of κΔθ=−q. For other domains, in [11], a theoretical investigation of the difference is made; the theoretical result requires strong conditions; for instance the cardinality of K must be very large and a finite but not small M is required: certain supports have to overlap so that the noise acts everywhere. In the present work we show numerically, in the next section, that Θst differs significantly from the parabolic profile even for relatively modest sets K and for M=1.
As announced in the previous section, the purpose of this numerical section is to show that the presence of the correction Lθ, due to the noise, in the deterministic Eq (1.5), modifies the asymptotic profile, even when the noise is weak in intensity, as described in the previous section, in order to have a small constant ϵQ.
We explain here this fact in two ways. The first one is theoretical, based on a very ideal noise. The second one is numerical.
In this subsection we suspend the requirement that q,Θ have to decay at infinity and accept a geometrically simpler case, although not strictly covered by the previous theory. We assume that the function q(x) is equal to a constant q, and both the stationary solution Θst(x) and Q(x,x) depend only on the vertical direction z∈[−1,1] and they are symmetric with respect to z=0; and smooth. The equation
div((κI+12Q(x,x))∇Θst(x))=−q(x) |
becomes
∂z((κ+Q22(z))∂zΘst(z))=−q. |
It gives us
(κ+Q22(z))∂zΘst(z)=−qz |
without constants, since both sides of the identity should vanish at z=0 (the function Θst is symmetric with respect to z=0 and smooth, hence ∂zΘst(0)=0). Therefore we have to solve
∂zΘst(z)=−qzκ+Q22(z)Θst(1)=0. |
The solution of the previous equation is
Θst(z)=−∫z−1qsκ+Q22(s)ds. |
Without noise the solution is
ΘQ=0st(z)=qκ1−z22=q2κ−q2κz2 |
so the curvature qκ is large (for κ small) and also the maximum is large:
maxΘQ=0st=q2κ. |
Assume
c2σ21[−1+δ,1−δ]≤Q22(z)≤c2σ2 |
with large σ2 and small δ. Then
qκ+c2σ21−z22≤Θst(z)≤−∫z−1qsκ+c1σ21[−1+δ,1−δ](s)ds. |
If z∈[−1,−1+δ] we have
Θst(z)≤qκ1−z22 |
like in the case without noise but, for z∈[−1+δ,0] we have
Θst(z)≤qκ1−(1−δ)22+qκ+c1σ2(1−δ)2−z22=C(κ,q,δ,σ2)−qκ+c1σ2z22. |
The curvature qκ+c1σ2 is much smaller than qκ and the maximum
maxΘst(z)=C(κ,q,δ,σ2)≥qκ+c1σ2(1−δ)22 |
is very small for large σ2 and small δ.
Figure 1 illustrates the modification of profile, from the standard parabolic one of free diffusion in a steady medium, to the case of turbulent decay. The reduction in heat content can be dramatic, due to turbulence, creating a fundamental engineering problem.
The purpose of this subsection is the numerical simulation of the effects of an operator L, based on the idea of vortex structures, to the solution of the problem
(κΔ+L)Θst=−q. |
More details on the construction of this operator L can be found in [11]. In this subsection we continue to suspend the requirement that q,Θ have to decay at infinity and accept that the function q(x) is equal to a constant q.
Recalling that
(Lθ)(x)=12d∑i,j=1∂i(∑k∈Kσk(x)⊗σk(x)∂jθ(x)), |
the σk's are chosen in order to be a rescaled and shifted version of a vector field w which satisfies several conditions:
1). w is smooth and divw=0;
2). w has compact support contained in ¯B(0,1);
3). w is close to 12πx⊥|x|2 near x=0.
The first two properties are useful in order to have that the σk's model the velocity of an incompressible fluid at rest. The third one is close to our idea of vortex structures. In particular, for r>0 and {xk}k∈K⊆R2|K| fixed, then
σk(x)=Γr−1w(x−xkr), |
where Γ is another parameter larger than 0. It remains to describe how to choose w. We construct it as w=∇⊥ψ so that it is divergence free. It remains to fix ψ compactly supported in ¯B(0,1) such that it is close to log|x|2π near x=0.
ψ(x)=∫R2ψ0(x−y)fϵ(y) dy |
where fϵ is a mollifier with support in B(0,ϵ) and ψ0 is a C∞(R2∖{0}) radial function such that
ψ0(x)=log|x|2π for |x|≤13 and ψ0(x)=0 for |x|>23. |
For numerical reasons we consider the problem in the bounded domain
˜D=(tan(−1.54),tan(1.54))×(−0.1,0.1). |
In order to have that the σk's model a fluid at rest, we can take
r≤maxk∈Kd(∂˜D,xk) and ϵ<16. |
These are the real constraints on the parameters of our numerical simulation. The other parameters Γ, K, {xk}k∈K can be chosen more arbitrarily in order to have satisfactory results. In fact, even if we do not examine the other constraints described in [11] the profile changes considerably in the region where the vortex structures have an impact.
The centers of the vortex structures {xk} have been chosen on a grid equally spaced in both directions. In particular we have chosen to take 10 points in the x1 direction between −0.5 and 0.5 and 3 points in the z direction between −0.05 and 0.05. Moreover, we take r=0.04, ϵ=0.1 and Γ=0.02√2. The other parameters of the problem are κ=0.05 and q≡1. In this way the impact of the operator L is related to a small portion of the domain ˜D and we can completely appreciate how it changes the profile of the solution.
Figures 2 and 3 illustrate the modification of the profile, from the standard parabolic one of free diffusion in a steady medium, to the case of turbulent decay. Even if we use just a really reduced number of vortices we can observe a significant decay modification of the profile due to turbulence.
The following abstract results are taken from [8]. The regularity theory of these equations has been recently raised and improved by [2,3,29], where the reader may find additional results.
Let H be a separable Hilbert space, A:D(A)⊆H→H the infinitesimal generator of a strongly continuous semigroup of negative type. Under these assumptions the family
Vα:=D((−A)α2) |
forms a Hilbert scale with inner product ⟨⋅,⋅⟩Vα and norm ‖⋅‖Vα, see [32]. We note that
A∈L(Vα+2,Vα) ∀α∈R, |
are linear bounded operators. For α>0 we mean the restriction of A to Vα+2 and for α<0 there exists a unique linear bounded extension of A from Vα+2 and Vα. Moreover, ∀α∈R, A generates an analytic semigroup of negative type in Vα denoted by eAt∈L(Vα), t≥0.
Consider the stochastic evolution equation
{du(t)=(Au(t)+q(t))dt+∑Nk=1Bku(t)dWkt t∈[t0,T]u(t0)=u0, | (3.1) |
interpreted in mild sense
u(t)=eAtu0+∫tt0eA(t−s)q(s) ds+N∑k=1∫tt0eA(t−s)Bku(s)dWks. | (3.2) |
Definition 9. Let α∈R, Bk∈L(Vα+1,Vα), problem (3.1) is well posed in Vα, if for every u0∈L2(Ft0,Vα) and q∈L2(t0,T;Vα) there exists a unique u mild solution of Eq (3.2) in CF([0,T];Vα)∩L2F(0,T;Vα+1). Moreover u depends continuously on u0 and q.
Theorem 10. Let α∈R be fixed. Let Bk∈L(Vα+1,Vα) such that
12N∑k=1‖Bku‖2Vα≤−η⟨Au,u⟩Vα+λ‖u‖2Vα, u∈Vα+2 |
and
N∑k=1‖Bku‖2Vα≤c‖u‖2Vα+1, u∈Vα+1 |
for some constants η∈(0,1) λ≥0 and c>0. Then Eq (3.1) is well posed in Vα. Moreover
‖u‖2CF([0,T];Vα)+‖u‖2L2F(0,T;Vα+1)≤C(‖ϕ‖2CF([0,T];Vα)+‖ϕ‖2L2F(0,T;Vα+1)) |
for ϕ(t)=eAtu0+∫tt0eA(t−s)q(s) ds and some constant c>0 independent of u0 and q.
Theorem 11. Let α<β be given real numbers. If Eq (3.1) is well posed in Vα and Vβ, then it is well posed in Vγ for all γ∈[α,β]. Moreover, for every u0∈L2F(Vα), q∈L2(t0,T;Vβ) and ϵ∈(t0,T), then u|[ϵ,T]∈CF(ϵ,T;Vβ)∩L2F(ϵ,T;Vβ+1) and depends continuously from u0 and q.
Theorem 12. Fixed α∈R, if the assumptions of theorem 10 hold true and
● Bk∈L(Vα+3,Vα+2);
● Lk:=ABk−BkA∈L(Vα+3,Vα) and
N∑k=1‖Lku‖2Vα≤c2‖u‖2Vα+2, u∈Vα+3 |
for some c2>0
then
12N∑k=1‖Bku‖2Vα+2≤−˜η⟨Au,u⟩Vα+2+˜λ‖u‖2Vα+2, u∈Vα+4 |
N∑k=1‖Bku‖2Vα+2≤c‖u‖2Vα+3, u∈Vα+3 |
for some ˜η∈(0,1), ˜λ≥0 and c>0. In particular Eq (3.1) is well posed in Vα+2.
Let A, H, V, D(A) and D as described in Section 2.1. In particular A is an elliptic operator. In fact ∀x∈D and ξ∈R2
⟨ξ,(κ+12Q(x,x))ξ⟩R2=∑k∈K⟨ξ,(κI+12σk(x)σk(x)t)ξ⟩R2≥κ|ξ|2 . |
Moreover from the boundedness of D in the second direction the Poincaré inequality holds, namely
∃Cp>0: ‖u‖2V≤Cp‖u‖2 ∀u∈V. |
For the operator A the following results hold, see for example [1,7,18].
Proposition 13. -A is self-adjoint.
Proposition 14. A is the infinitesimal generator of an analytic semigroup of negative type.
Under these assumptions, as described in Section 3.1, the family
Vα:=D((−A)α2) |
form a Hilbert scale with inner product ⟨⋅,⋅⟩Vα and norm ‖⋅‖Vα. We note that
A∈L(Vα+2,Vα) ∀α∈R, |
are linear bounder operators. For α>0 we mean the restriction of A to Vα+2 and for α<0 there exists a unique linear bounded extension of A from Vα+2 and Vα. Moreover, ∀α∈R, A generates an analytic semigroup of negative type in Vα denoted by eAt∈L(Vα), t≥0.
Proposition 15.
● D((−A)θ)=H2θ(D) if θ∈(0,14);
● D((−A)θ)={u∈H2θ(D): u|∂D=0} if θ∈(14,1).
In particular, H=V0, V=V1, D(A)=V2.
Equation (1.4) can be rewritten as
{dθ(t,x)=(Aθ(t,x)+q(t,x))dt+∑k∈KBkθ(t,x)dWkt (t,x)∈[0,T]×Dθ(t,(x1,±1))=0 x1∈R, t∈[0,T]θ(0,x)=θ0(x) x∈D, | (3.3) |
where Bku:=−∑2j=1σjk∂u∂xj. Bk∈L(V1,H) without any further assumption on {σk}k∈K. The linearity is obvious, the continuity follows from the boundedness of σk.
Definition 16. Given θ0∈L2(F0,H) and q∈L2(0,T;H), we say that a stochastic process θ is a weak solution of Eq (1.4) if
θ∈CF([0,T];H)∩L2F(0,T;V) |
and for every ϕ∈D(A), we have
⟨θ(t),ϕ⟩=⟨θ0,ϕ⟩+∫t0⟨θ(s),Aϕ⟩ ds+∫t0⟨q(s),ϕ⟩+−∑k∈K∫t0⟨θ(s),Bkϕ⟩ dWks |
for every t∈[0,T], P−a.s.
Proposition 17. θ is a weak solution of problem (1.4) if and only if is a mild solution of problem (1.4).
Proof. Let θ(t) be a weak solution and ϕ(t)∈C1([0,T];H)∩C([0,T];D(A)). Let, moreover, π={0=t0<t1<⋯<Tn=T} be a partition of [0,T]. Thus, using the identity
⟨θ(ti+1),ϕ(ti+1)⟩−⟨θ(ti+1),ϕ(ti)⟩=∫ti+1ti⟨θ(ti+1),∂sϕ(s)⟩ ds, |
we get
⟨θ(ti+1),ϕ(ti+1)⟩=⟨θ(ti),ϕ(ti)⟩+∫ti+1ti⟨θ(s),Aϕ(ti)⟩ ds+∫ti+1ti⟨q(s),ϕ(ti)⟩ ds+∫ti+1ti⟨θ(ti+1),∂sϕ(s)⟩ ds−∑k∈K∫ti+1ti⟨θ(s),Bkϕ(ti)⟩ dWks. |
It implies
⟨θ(T),ϕ(T)⟩=⟨θ0,ϕ(0)⟩+∫T0⟨θ(s),Aϕ(s−π(s))⟩ ds+∫T0⟨θ(s+π(s)),∂sϕ(s)⟩ ds+∫t0⟨q(s),ϕ(s−π(s))⟩ ds−∑k∈K∫T0⟨θ(s),Bkϕ(s−π(s))⟩ dWks, |
where s−π(s)=ti if s∈[ti,ti+1] and s+π(s)=ti+1 if s∈[ti,ti+1]. Taking the limit over a sequence of partitions πN with size going to zero, we get
⟨θ(T),ϕ(T)⟩=⟨θ0,ϕ(0)⟩+∫T0⟨θ(s),Aϕ(s)⟩ ds+∫T0⟨θ(s),∂sϕ(s)⟩ ds+∫T0⟨q(s),ϕ(s)⟩ ds−∑k∈K∫T0⟨θ(s),Bkϕ(s)⟩ dWks |
(thanks to the regularity of θ, ϕ, q, dominated convergence theorem and Itô isometry). The argument applies to a generic t∈[0,T], hence we have
⟨θ(t),ϕ(t)⟩=⟨θ0,ϕ(0)⟩+∫t0⟨θ(s),Aϕ(s)⟩ ds+∫t0⟨θ(s),∂sϕ(s)⟩ ds+∫t0⟨q(s),ϕ(s)⟩ ds−∑k∈K∫t0⟨θ(s),Bkϕ(s)⟩ dWks. |
For such value of t, take the function ϕt(s)=e(t−s)Aψ with ψ∈D(A). This function is of class ϕt∈C1([0,t];H)∩C([0,t];D(A)). Hence from previous identity we get
⟨θ(t),ψ⟩=⟨θ0,etAψ⟩+∫t0⟨θ(s),Ae(t−s)Aψ⟩ ds−∫t0⟨θ(s),Ae(t−s)Aψ⟩ ds+∫t0⟨q(s),e(t−s)Aψ⟩ ds−∑k∈K∫t0⟨θ(s),Bke(t−s)Aψ⟩ dWks=⟨θ0,etAψ⟩+∫t0⟨q(s),e(t−s)Aψ⟩ ds−∑k∈K∫t0⟨θ(s),Bke(t−s)Aψ⟩ dWks. |
Recalling that Bkv=−σk⋅∇v, div(σk)=0, integrating by parts and using the fact that A is selfadjoint we get
⟨θ(t),ψ⟩=⟨etAθ0,ψ⟩+∑k∈K∫t0⟨e(t−s)ABkθ(s),ψ⟩ dWks+∫t0⟨e(t−s)Aq(s),ψ⟩ ds. |
By the arbitrarity of ψ we get that θ is a mild solution, namely
θ(t)=etAθ0+∫t0e(t−s)Aq(s) ds+∑k∈K∫t0e(t−s)ABkθ(s)dWks. |
Let now θ(t) be a mild solution and ϕ∈D(A). Doing the scalar product between θ(t) and ϕ we get
⟨θ(t),ϕ⟩=⟨etAθ0,ϕ⟩+∫t0⟨e(t−s)Aq(s),ϕ⟩ ds+∑k∈K∫t0⟨e(t−s)ABkθ(s),ϕ⟩ dWks. |
Let us analyze the quantity ⟨etAθ0,ϕ⟩. Using the fact that A is selfadjoint and integrating by parts backwards we get
⟨etAθ0,ϕ⟩=⟨θ0,etAϕ⟩=⟨θ0,ϕ⟩+∫t0⟨θ0,AesAϕ⟩ ds. |
Now thanks to the regularity of ϕ∈D(A) and the fact that A is selfadjoint, exploiting the definition of mild solution we get
∫t0⟨θ0,AesAϕ⟩ds=∫t0⟨esAθ0,Aϕ⟩ ds=∫t0⟨θ(s),Aϕ⟩ ds−∫t0ds∫s0 du⟨e(s−u)Aq(u),Aϕ⟩−∑k∈K∫t0ds∫s0⟨e(s−u)ABkθ(u),Aϕ⟩ dWku. |
Let us note that
∫Tt dxE[∫T0|⟨e(x−t)ABkθ(t),Aϕ⟩|2 dt]12≤∫Ttdx E[∫T0‖Bkθ(t)‖2‖Aϕ‖2 dt]12≤C‖Aϕ‖‖θ‖L2F(0,T;V)<+∞. |
Thus we can apply the stochastic Fubini theorem to the stochastic integrals and, exploiting arguments analogous to the previous ones, we get
−∫t0 ds∫s0⟨e(s−u)ABkθ(u),Aϕ⟩ dWku=−∫t0dWku∫tu⟨e(s−u)ABkθ(u),Aϕ⟩ ds=−∫t0dWku[⟨Bkθ(u),e(s−u)Aϕ⟩]s=ts=u=−∫t0dWku⟨e(t−u)ABkθ(u),ϕ⟩ −∫t0dWku⟨θ(u),Bkϕ⟩. |
Applying Fubini theorem to −∫t0ds∫s0 du⟨e(s−u)Aq(u),Aϕ⟩ we get
−∫t0 ds∫s0⟨e(s−u)Aq(u),Aϕ⟩ du=−∫t0du∫tu⟨e(s−u)Aq(u),Aϕ⟩ ds=−∫t0du[⟨q(u),e(s−u)Aϕ⟩]s=ts=u=−∫t0du⟨e(t−u)Aq(u),ϕ⟩+∫t0du⟨q(u),ϕ⟩. |
Putting together all these relations we get the weak formulation.
Remark 18. From the weak formulation we can obtain easily the Itô formula
‖θ(t)‖2−‖θ(0)‖2=−2∑k∈K∫t0 dWks⟨θ(s),σk⋅∇θ(s)⟩+2∫t0⟨θ(s),q(s)⟩ ds−2∫t0⟨(−A)12θ(s),(−A)12θ(s)⟩ ds+∑k∈K∫t0‖σk⋅∇θ(s)‖2 ds. |
Thanks to the results of Section 3.2 we know that A is the infinitesimal generator of an analytic semigroup of negative type, hence we can apply the abstract results of Section 3.1.
Thanks to theorem 10 it is enough to show that there exist η∈(0,1),λ≥0, c>0 such that:
1). 12∑k∈K‖∑2j=1σjk∂u∂xj‖2≤−η⟨Au,u⟩+λ‖u‖2 ∀u∈D(A).
2). ∑k∈K‖∑2j=1σjk∂u∂xj‖2≤c‖u‖2V ∀u∈V.
1). Calling M:=‖Q‖L∞(D), the first inequality holds taking λ≥0, η∈[M2κ+M,1). In fact
−η<Au,u>+λ‖u‖2=ηκ∫D|∇u|2 dx+λ∫D|u|2 dx+η2∫D∇u⋅Q∇u dx |
and
12∑k∈K‖2∑j=1σjk∂u∂xj‖2=12∑k∈K∫D∇u⋅σkσk⋅∇u dx=12∫D∇u⋅Q∇u dx. |
Under previous assumptions on λ and η
12∑k∈K‖2∑j=1σjk∂u∂xj‖2+η⟨Au,u⟩−λ‖u‖2=−ηκ∫D|∇u|2 dx−λ∫D|u|2 dx+1−η2∫D∇u⋅Q∇u dx≤−ηκ∫D|∇u|2 dx+M(1−η)2∫D|∇u|2 dx≤0. |
In particular, if we choose η=M2κ+M and λ=0 we get
12∑k∈K‖2∑j=1σjk∂u∂xj‖2≤−M2κ+M⟨Au,u⟩ ∀u∈D(A) |
2). The second inequality is satisfied taking c=M:=‖Q‖L∞(D). In fact, as above,
∑k∈K‖2∑j=1σjk∂u∂xj‖2=∫D∇u⋅Q∇u dx≤M‖u‖2V. |
The assumptions of theorem 10 are satisfied for α=0. In particular, Eq (1.4) is well posed in H and the thesis follows.
Remark 19. As a corollary one gets existence and uniqueness of the weak solution in the sense of definition 16.
1). Theorem 5. Since Theorem 4 was proved verifying the assumptions of Theorem 10, we can exploit a bootstrapping procedure thanks to Theorem 11 and 12. Regardless of the other hypotheses, if Bk∈L(V3,D(A)) then
Lku=(ABk−BkA)u= |
2∑i,j,l=1κ∂i,iσlk∂lu+2κ∂iσlk∂i,lu+12(∂iQi,j∂jσlk∂lu+Qi,j∂i,jσlk∂lu+2Qi,j∂iσlk∂j,lu−∂i,lQi,jσlk∂ju−∂lQi,jσlk∂i,ju). |
In particular, if u∈V3 thanks to the regularity of σk, then
∑k∈K‖Lku‖2≤C∑j,l(‖∂2u∂xj∂xl‖2+‖∂u∂xj‖2)≤C‖u‖2D(A). |
Moreover, thanks to the assumptions on σk, Bk∈L(V3,D(A)). The linearity is obvious. If u∈V3, then Bku∈D(A) which means in particular that Bku|{x2=±1}=0. In fact
Bku|{x2=±1}=σ2k∂u∂x2|{x2=±1}=0. |
The continuity follows from the boundedness of the derivatives of σk and by the equivalence between the norm of H3(D) and V3 for u∈V3. Then we get the first part of the thesis applying Theorem 12 and Theorem 11. The second part follows by the first one and Theorem 11.
2). Corollary 6. Under these assumptions
θ∈L2F(0,T;V3)∩CF([0,T];D(A)), |
thus from the Itô formula described in Remark 18, with starting time t0=t and ending time t+h we get
‖θ(t+h)‖2−‖θ(t)‖2=−2∑k∈K∫t+ht dWks⟨θ(s),σk⋅∇θ(s)⟩+∑k∈K∫t+ht‖σk⋅∇θ(s)‖2 ds+2∫t+ht⟨θ(s),q⟩ ds+2∫t+ht⟨θ(s),Aθ(s)⟩ ds. |
Looking carefully at the proof of Theorem 4 we know that ∃η∈(0,1) such that
12∑k∈K‖σk⋅∇u‖2≤−η⟨Au,u⟩ ∀u∈D(A). |
Thus, taking the expected value and exploiting this relation, Young and Poincaré inequalities we get
E[‖θ(t+h)‖2]=E[‖θ(t)‖2]+2E[∫t+ht⟨θ(s),q⟩ ds] +2E[∫t+ht⟨θ(s),Aθ(s)⟩ ds] +∑k∈KE[∫t+ht‖σk⋅∇θ(s)‖2 ds]≤E[‖θ(t)‖2]+2(1−η)E[∫t+ht⟨θ(s),Aθ(s)⟩ ds] +2E[∫t+ht‖θ(s)‖‖q‖ ds]≤E[‖θ(t)‖2]−2(1−η)κE[∫t+ht‖∇θ(s)‖2 ds] +(1−η)κCpE[∫t+ht‖θ(s)‖2 ds]+Cp4(1−λ)κh‖q‖2≤E[‖θ(t)‖2]−2(1−η)κCpE[∫t+ht‖θ(s)‖2 ds] +(1−η)κCpE[∫t+ht‖θ(s)‖2 ds]+Cp4(1−λ)κh‖q‖2, |
namely there exist C1, C2 depending on η, κ and Cp such that
E[‖θ(t+h)‖2]≤E[‖θ(t)‖2]−C1∫t+htE[‖θ(s)‖2] ds+C2h‖q‖2. | (3.4) |
From Eq (3.4), exploiting the arbitrariness of t and h and the regularity of θ, we can apply Gronwall's lemma in differential form proving that
E[‖θ(t)‖2]≤‖θ0‖2+C2C1‖q‖2. |
Moreover we can apply the second part of Theorem 5 with parameters
t0=t, T=t+2. From the regularity of θ we get that
θ(t0)∈L2(Ft0,D(A)), |
thus thanks to previous inequality
E[‖θ(t+1)‖2D(A)]≤C(E[‖θ(t)‖2]+2‖q‖2D(A))≤C(‖θ0‖2+‖q‖2D(A)). |
From the arbitrariness of t
supt∈[1,T]E[‖θ(t)‖2D(A)]≤C(‖θ0‖2D(A)+‖q‖2D(A)). |
It remains to show that
supt∈[0,1]E[‖θ(t)‖2D(A)]≤C(‖θ0‖2D(A)+‖q‖2D(A)). |
This inequality can be obtained directly from the well-posedness in D(A) and we omit the details. Lastly by Sobolev embedding theorem, recalling that
D(A)↪L∞(D) |
we get the thesis.
Recall the identity
θ(t)=etAθ0+∫t0e(t−s)Aq(s)ds−∑k∈K∫t0e(t−s)Aσk⋅∇θ(s)dWks. |
Set
Θ(t)=etAθ0+∫t0e(t−s)Aq(s)ds. |
Then
θ(t)−Θ(t)=−∑k∈K∫t0e(t−s)Aσk⋅∇θ(s)dWks. |
If ϕ∈H,
⟨θ(t)−Θ(t),ϕ⟩=∑k∈K∫t0⟨θ(s),σk⋅∇θe(t−s)Aϕ⟩dWks. |
Then (here we take advantage of the cancellations of Itô integrals)
E[⟨θ(t)−Θ(t),ϕ⟩2]=∑k∈KE∫t0⟨θ(s),σk⋅∇e(t−s)Aϕ⟩2ds. |
Write ϕt,s:=e(t−s)Aϕ. Then
∑k∈K⟨θ(s),σk⋅∇ϕt,s⟩2=∑k∈K∫∫θ(s,x)θ(s,y)σk(x)⋅∇ϕt,s(x)σk(y)⋅∇ϕt,s(y)dxdy=∫∫θ(s,y)∇ϕt,s(y)TQ(x,y)∇ϕt,s(x)θ(s,x)dxdy≤−ϵQk‖θ(s)‖2∞⟨Ae(t−s)Aϕ,e(t−s)Aϕ⟩. |
Therefore
E[⟨θ(t)−Θ(t),ϕ⟩2]≤ϵQkC∞(θ0,q)∫t0⟨(−A)e(t−s)Aϕ,e(t−s)Aϕ⟩ds=ϵQkC∞(θ0,q)∫t0dds‖e(t−s)Aϕ‖2ds≤ϵQkC∞(θ0,q)‖ϕ‖2. |
Now we use the fact that
limt→∞⟨Θ(t)−Θst,ϕ⟩=0. |
Indeed,
Θ(t)−Θst=etA(θ0+A−1q). |
For every ϵ>0, from the inequality (a+b)2≤(1+ϵ)a2+(1+4ϵ)b2 we have
E[⟨θ(t)−Θst,ϕ⟩2]≤(1+ϵ)E[⟨θ(t)−Θ(t),ϕ⟩2]+(1+4ϵ)E[⟨Θ(t)−Θst,ϕ⟩2]. |
This implies the result of the theorem.
The authors declare no conflict of interest.
[1] | A. Pikovsky, M. Rosenblum, J. Kurths, Synchronization: A Universal Concept in Nonlinear Sciences, Cambridge Nonlinear Science Series, Cambridge University Press, 2010. https://doi.org/10.1017/CBO9780511755743 |
[2] | K. Kaneko, Theory and applications of coupled map lattices, in Nonlinear Science: Theory and Applications, Wiley–Blackwell, 1993. Available from: https://cir.nii.ac.jp/crid/1573105973923422464. |
[3] |
E. Ott, K. Wiesenfeld, Chaos in Dynamical Systems, Phys. Today, 47 (1994). https://doi.org/10.1063/1.2808369 doi: 10.1063/1.2808369
![]() |
[4] |
G. Saxena, A. Prasad, R. Ramaswamy, Amplitude death: the emergence of stationarity in coupled nonlinear systems, Phys. Rep., 521 (2012), 205–228. https://doi.org/10.1016/j.physrep.2012.09.003 doi: 10.1016/j.physrep.2012.09.003
![]() |
[5] |
P. Kumar, A. Prasad, R. Ghosh, Stable phase-locking of an external-cavity diode laser subjected to external optical injection, J. Phys. B: At. Mol. Opt. Phys., 41 (2008), 135402. https://doi.org/10.1088/0953-4075/41/13/135402 doi: 10.1088/0953-4075/41/13/135402
![]() |
[6] |
B. Gallego, P. Cessi, Decadal variability of two oceans and an atmosphere, J. Clim., 14 (2001), 2815–2832. https://doi.org/10.1175/1520-0442(2001)014<2815:DVOTOA>2.0.CO;2 doi: 10.1175/1520-0442(2001)014<2815:DVOTOA>2.0.CO;2
![]() |
[7] |
H. Zhang, D. Xu, C. Lu, E. Qi, J. Hu, Y. Wu, Amplitude death of a multi-module floating airport, Nonlinear Dyn., 79 (2015), 2385–2394. https://doi.org/10.1007/s11071-014-1819-x doi: 10.1007/s11071-014-1819-x
![]() |
[8] |
T. Banerjee, D. Biswas, Amplitude death and synchronized states in nonlinear time-delay systems coupled through mean-field diffusion, Chaos, 23 (2013), 043101. https://doi.org/10.1063/1.4823599 doi: 10.1063/1.4823599
![]() |
[9] |
D. Ghosh, T. Banerjee, Transitions among the diverse oscillation quenching states induced by the interplay of direct and indirect coupling, Phys. Rev. E: Stat. Nonlinear Soft Matter Phys., 90 (2014), 062908. https://doi.org/10.1103/PhysRevE.90.062908 doi: 10.1103/PhysRevE.90.062908
![]() |
[10] |
G. B. Ermentrout, N. Kopell, Oscillator death in systems of coupled neural oscillators, SIAM J. Appl. Math., 50 (1990), 125–146. https://doi.org/10.1137/0150009 doi: 10.1137/0150009
![]() |
[11] |
A. Koseska, E. Volkov, J. Kurths, Oscillation quenching mechanisms: amplitude vs. oscillation death, Phys. Rep., 531 (2013), 173–199. https://doi.org/10.1016/j.physrep.2013.06.001 doi: 10.1016/j.physrep.2013.06.001
![]() |
[12] |
R. Curtu, Singular Hopf bifurcations and mixed-mode oscillations in a two-cell inhibitory neural network, Physica D, 239 (2010), 504–514. https://doi.org/10.1016/j.physd.2009.12.010 doi: 10.1016/j.physd.2009.12.010
![]() |
[13] |
A. Koseska, E. Volkov, J. Kurths, Parameter mismatches and oscillation death in coupled oscillators, Chaos, 20 (2010), 023132. https://doi.org/10.1063/1.3456937 doi: 10.1063/1.3456937
![]() |
[14] |
N. Suzuki, C. Furusawa, K. Kaneko, Oscillatory protein expression dynamics endows stem cells with robust differentiation potential, PLoS One, 6 (2011), e27232. https://doi.org/10.1371/journal.pone.0027232 doi: 10.1371/journal.pone.0027232
![]() |
[15] |
D. Biswas, N. Hui, T. Banerjee, Amplitude death in intrinsic time-delayed chaotic oscillators with direct–indirect coupling: the existence of death islands, Nonlinear Dyn., 88 (2017), 2783–2795. https://doi.org/10.1007/s11071-017-3411-7 doi: 10.1007/s11071-017-3411-7
![]() |
[16] | A. H. Nayfeh, D. T. Mook, Nonlinear Oscillations, John Wiley & Sons, 2008. |
[17] |
A. Anees, Z. Ahmed, A technique for designing substitution box based on van der pol oscillator, Wireless Pers. Commun., 82 (2015), 1497–1503. https://doi.org/10.1007/s11277-015-2295-4 doi: 10.1007/s11277-015-2295-4
![]() |
[18] |
G. Juárez, M. Ramírez-Trocherie, Á. Báez, A. Lobato, E. Iglesias-Rodríguez, P. Padilla, et al., Hopf bifurcation for a fractional van der Pol oscillator and applications to aerodynamics: implications in flutter, J. Eng. Math., 139 (2023), 1–15. https://doi.org/10.1007/s10665-023-10258-7 doi: 10.1007/s10665-023-10258-7
![]() |
[19] |
S. Dutta, N. R. Cooper, Critical response of a quantum van der Pol oscillator, Phys. Rev. Lett., 123 (2019), 250401. https://doi.org/10.1103/PhysRevLett.123.250401 doi: 10.1103/PhysRevLett.123.250401
![]() |
[20] |
S. Wirkus, R. Rand, The dynamics of two coupled van der Pol oscillators with delay coupling, Nonlinear Dyn., 30 (2002), 205–221. https://doi.org/10.1023/A:1020536525009 doi: 10.1023/A:1020536525009
![]() |
[21] |
E. Camacho, R. Rand, H. Howland, Dynamics of two van der Pol oscillators coupled via a bath, Int. J. Solids Struct., 41 (2004), 2133–2143. https://doi.org/10.1016/j.ijsolstr.2003.11.035 doi: 10.1016/j.ijsolstr.2003.11.035
![]() |
[22] | K. Konishi, Experimental evidence for amplitude death induced by dynamic coupling: van der Pol oscillators, in 2004 IEEE International Symposium on Circuits and Systems (ISCAS), 4 (2004), 792–795. https://doi.org/10.1109/ISCAS.2004.1329123 |
[23] |
T. Endo, S. Mori, Mode analysis of a ring of a large number of mutually coupled van der Pol oscillators, IEEE Trans. Circuits Syst., 25 (1978), 7–18. https://doi.org/10.1109/TCS.1978.1084380 doi: 10.1109/TCS.1978.1084380
![]() |
[24] |
V. Resmi, G. Ambika, R. E. Amritkar, General mechanism for amplitude death in coupled systems, Phys. Rev. E: Stat. Nonlinear Soft Matter Phys., 84 (2011), 046212. https://doi.org/10.1103/PhysRevE.84.046212 doi: 10.1103/PhysRevE.84.046212
![]() |
[25] |
D. Ghosh, T. Banerjee Mixed-mode oscillation suppression states in coupled oscillators, Phys. Rev. E: Stat. Nonlinear Soft Matter Phys., 92 (2015), 052913. https://doi.org/10.1103/PhysRevE.92.052913 doi: 10.1103/PhysRevE.92.052913
![]() |
[26] | C. O. Weiss, R. Vilaseca, Dynamics of lasers, NASA STI/Recon Tech. Rep. A, 92 (1991), 39875. Available from: https://ui.adsabs.harvard.edu/abs/1991STIA...9239875W/abstract. |
[27] | K. A. Robbins, A new approach to subcritical instability and turbulent transitions in a simple dynamo, in Mathematical Proceedings of the Cambridge Philosophical Society, Cambridge University Press, 82 (1997), 309–325. https://doi/org/10.1017/S0305004100053950 |
[28] | B. Ermentrout, XPPAUT 5.0-the Differential Equations Tool, University of Pittsburgh, Pittsburgh, 2001. |
[29] |
E. X. DeJesus, C. Kaufman, Routh-Hurwitz criterion in the examination of eigenvalues of a system of nonlinear ordinary differential equations, Phys. Rev. A: At. Mol. Opt. Phys., 35 (1987), 5288. https://doi.org/10.1103/PhysRevA.35.5288 doi: 10.1103/PhysRevA.35.5288
![]() |
[30] |
G. Saxena, A. Prasad, R. Ramaswamy, Amplitude death: the emergence of stationarity in coupled nonlinear systems, Phys. Rep., 521 (2012), 205–228. https://doi.org/10.1016/j.physrep.2012.09.003 doi: 10.1016/j.physrep.2012.09.003
![]() |
[31] |
A. Sharma, M. D. Shrimali Amplitude death with mean-field diffusion, Phys. Rev. E: Stat. Nonlinear Soft Matter Phys., 85 (2012), 057204. https://doi.org/10.1103/PhysRevE.85.057204 doi: 10.1103/PhysRevE.85.057204
![]() |
[32] |
A. Sharma, K. Suresh, K. Thamilmaran, A. Prasad, M. D. Shrimali, Effect of parameter mismatch and time delay interaction on density-induced amplitude death in coupled nonlinear oscillators, Nonlinear Dyn., 76 (2014), 1797–1806. https://doi.org/10.1007/s11071-014-1247-y doi: 10.1007/s11071-014-1247-y
![]() |
[33] |
T. Banerjee, D. Ghosh, Experimental observation of a transition from amplitude to oscillation death in coupled oscillators, Phys. Rev. E: Stat. Nonlinear Soft Matter Phys., 89 (2014), 062902. https://doi.org/10.1103/PhysRevE.89.062902 doi: 10.1103/PhysRevE.89.062902
![]() |
[34] |
N. K. Kamal, P. R. Sharma, M. D. Shrimali, Suppression of oscillations in mean-field diffusion, Pramana, 84 (2015), 237–247. https://doi.org/10.1007/s12043-015-0929-4 doi: 10.1007/s12043-015-0929-4
![]() |
[35] |
A. Zakharova, I. Schneider, Y. N. Kyrychko, K. B. Blyuss, A. Koseska, B. Fiedler, et al., Time delay control of symmetry-breaking primary and secondary oscillation death, Europhys. Lett., 104 (2013), 50004. https://doi.org/10.1209/0295-5075/104/50004 doi: 10.1209/0295-5075/104/50004
![]() |
[36] |
D. V. R. Reddy, A. Sen, G. L. Johnston, Time delay induced death in coupled limit cycle oscillators, Phys. Rev. Lett., 80 (1998), 5019. https://doi.org/10.1103/PhysRevLett.80.5109 doi: 10.1103/PhysRevLett.80.5109
![]() |
[37] |
D. V. R. Reddy, A. Sen, G. L. Johnston, Experimental evidence of time-delay-induced death in coupled limit-cycle oscillators, Phys. Rev. Lett., 85 (2000), 3381. https://doi.org/10.1103/PhysRevLett.85.3381 doi: 10.1103/PhysRevLett.85.3381
![]() |
[38] |
F. M. Atay, Distributed delays facilitate amplitude death of coupled oscillators, Phys. Rev. Lett., 91 (2003), 094101. https://doi.org/10.1103/PhysRevLett.91.094101 doi: 10.1103/PhysRevLett.91.094101
![]() |
[39] |
W. Zou, D. V. Senthilkumar, A. Koseska, J. Kurths, Generalizing the transition from amplitude to oscillation death in coupled oscillators, Phys. Rev. E: Stat. Nonlinear Soft Matter Phys., 88 (2013), 050901. https://doi.org/10.1103/PhysRevE.88.050901 doi: 10.1103/PhysRevE.88.050901
![]() |
[40] |
R. Karnatak, R. Ramaswamy, A. Prasad, Amplitude death in the absence of time delays in identical coupled oscillators, Phys. Rev. E: Stat. Nonlinear Soft Matter Phys., 76 (2007), 035201. https://doi.org/10.1103/PhysRevE.76.035201 doi: 10.1103/PhysRevE.76.035201
![]() |
[41] |
A. Sharma, P. R. Sharma, M. D. Shrimali, Amplitude death in nonlinear oscillators with indirect coupling, Phys. Lett. A, 376 (2012), 1562–1566. https://doi.org/10.1016/j.physleta.2012.03.033 doi: 10.1016/j.physleta.2012.03.033
![]() |
[42] |
C. R. Hens, O. I. Olusola, P. Pal, S. K. Dana, Oscillation death in diffusively coupled oscillators by local repulsive link, Phys. Rev. E: Stat. Nonlinear Soft Matter Phys., 88 (2013), 034902. https://doi.org/10.1103/PhysRevE.88.034902 doi: 10.1103/PhysRevE.88.034902
![]() |
[43] |
B. K. Bera, C. Hens, D. Ghosh, Emergence of amplitude death scenario in a network of oscillators under repulsive delay interaction, Phys. Lett. A, 380 (2016), 2366–2373. https://doi.org/10.1016/j.physleta.2016.05.028 doi: 10.1016/j.physleta.2016.05.028
![]() |
[44] |
N. K. Kamal, P. R. Sharma, M. D. Shrimali, Oscillation suppression in indirectly coupled limit cycle oscillators, Phys. Rev. E: Stat. Nonlinear Soft Matter Phys., 92 (2015), 022928. https://doi.org/10.1103/PhysRevE.92.022928 doi: 10.1103/PhysRevE.92.022928
![]() |
[45] |
P. R. Sharma, N. K. Kamal, U. K. Verma, K. Suresh, K. Thamilmaran, M. D. Shrimali, Suppression and revival of oscillation in indirectly coupled limit cycle oscillators, Phys. Lett. A, 380 (2016), 3178–3184. https://doi.org/10.1016/j.physleta.2016.07.041 doi: 10.1016/j.physleta.2016.07.041
![]() |
[46] |
A. Sharma, U. K. Verma, M. D. Shrimali, Phase-flip and oscillation-quenching-state transitions through environmental diffusive coupling, Phys. Rev. E: Stat. Nonlinear Soft Matter Phys., 94 (2016), 062218. https://doi.org/10.1103/PhysRevE.94.062218 doi: 10.1103/PhysRevE.94.062218
![]() |
[47] |
J. Choi, P. Kim, Reservoir computing based on quenched chaos, Chaos, Solitons Fractals, 140 (2020), 110131. https://doi.org/10.1016/j.chaos.2020.110131 doi: 10.1016/j.chaos.2020.110131
![]() |
[48] |
E. Ullner, A. Zaikin, E. I. Volkov, J. García-Ojalvo, Multistability and clustering in a population of synthetic genetic oscillators via phase-repulsive cell-to-cell communication, Phys. Rev. Lett., 99 (2007), 148103. https://doi.org/10.1103/PhysRevLett.99.148103 doi: 10.1103/PhysRevLett.99.148103
![]() |
[49] |
A. Takamatsu, Spontaneous switching among multiple spatio-temporal patterns in three-oscillator systems constructed with oscillatory cells of true slime mold, Physica D, 223 (2006), 180–188. https://doi.org/10.1016/j.physd.2006.09.001 doi: 10.1016/j.physd.2006.09.001
![]() |
1. | Giulia Carigi, Eliseo Luongo, Dissipation Properties of Transport Noise in the Two-Layer Quasi-geostrophic Model, 2023, 25, 1422-6928, 10.1007/s00021-023-00773-z | |
2. | Franco Flandoli, Eliseo Luongo, 2023, Chapter 6, 978-3-031-18987-6, 69, 10.1007/978-3-031-18988-3_6 | |
3. | Roberta Bianchini, Chiara Saffirio, Fluid instabilities, waves and non-equilibrium dynamics of interacting particles: a short overview, 2022, 5, 2640-3501, 1, 10.3934/mine.2023033 | |
4. | Lucio Galeati, Dejun Luo, LDP and CLT for SPDEs with transport noise, 2023, 2194-0401, 10.1007/s40072-023-00292-y | |
5. | Franco Flandoli, Dejun Luo, Eliseo Luongo, 2D Smagorinsky-Type Large Eddy Models as Limits of Stochastic PDEs, 2024, 34, 0938-8974, 10.1007/s00332-024-10028-4 | |
6. | Antonio Agresti, Eliseo Luongo, Global well-posedness and interior regularity of 2D Navier–Stokes equations with stochastic boundary conditions, 2024, 390, 0025-5831, 2727, 10.1007/s00208-024-02812-0 | |
7. | Francesco Grotto, Eliseo Luongo, Mario Maurelli, Uniform approximation of 2D Navier-Stokes equations with vorticity creation by stochastic interacting particle systems, 2023, 36, 0951-7715, 7149, 10.1088/1361-6544/ad0aab | |
8. | Dejun Luo, Enhanced Dissipation for Stochastic Navier–Stokes Equations with Transport Noise, 2023, 1040-7294, 10.1007/s10884-023-10307-w | |
9. | Franco Flandoli, Eliseo Luongo, 2023, Chapter 3, 978-981-99-0387-0, 75, 10.1007/978-981-99-0385-6_3 | |
10. | Dejun Luo, Danli Wang, Well Posedness and Limit Theorems for a Class of Stochastic Dyadic Models, 2023, 55, 0036-1410, 1464, 10.1137/22M1511497 | |
11. | Dejun Luo, Bin Tang, Stochastic inviscid Leray-α model with transport noise: Convergence rates and CLT, 2023, 234, 0362546X, 113301, 10.1016/j.na.2023.113301 | |
12. | Chang Liu, Dejun Luo, Finite time mixing and enhanced dissipation for 2D Navier-Stokes equations by Ornstein–Uhlenbeck flow, 2025, 38, 0951-7715, 025009, 10.1088/1361-6544/ada50f |