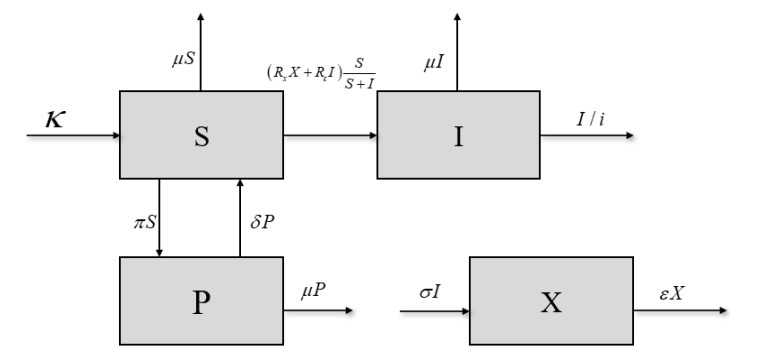
The study of drug side effects is a significant task in drug discovery. Candidate drugs with unaccepted side effects must be eliminated to prevent risks for both patients and pharmaceutical companies. Thus, all side effects for any candidate drug should be determined. However, this task, which is carried out through traditional experiments, is time-consuming and expensive. Building computational methods has been increasingly used for the identification of drug side effects. In the present study, a new path-based method was proposed to determine drug side effects. A heterogeneous network was built to perform such method, which defined drugs and side effects as nodes. For any drug and side effect, the proposed path-based method determined all paths with limited length that connects them and further evaluated the association between them based on these paths. The strong association indicates that the drug has a side effect with a high probability. By using two types of jackknife test, the method yielded good performance and was superior to some other network-based methods. Furthermore, the effects of one parameter in the method and heterogeneous network was analyzed.
Citation: Meng Jiang, Bo Zhou, Lei Chen. Identification of drug side effects with a path-based method[J]. Mathematical Biosciences and Engineering, 2022, 19(6): 5754-5771. doi: 10.3934/mbe.2022269
[1] | Asma A Alhashmi, Manal Abdullah Alohali, Nazir Ahmad Ijaz, Alaa O. Khadidos, Omar Alghushairy, Ahmed Sayed . Bayesian optimization with deep learning based pepper leaf disease detection for decision-making in the agricultural sector. AIMS Mathematics, 2024, 9(7): 16826-16847. doi: 10.3934/math.2024816 |
[2] | Xiaoxiao Liu, Chunrui Zhang . Forest model dynamics analysis and optimal control based on disease and fire interactions. AIMS Mathematics, 2024, 9(2): 3174-3194. doi: 10.3934/math.2024154 |
[3] | Ahmed Alshehri, Saif Ullah . Optimal control analysis of Monkeypox disease with the impact of environmental transmission. AIMS Mathematics, 2023, 8(7): 16926-16960. doi: 10.3934/math.2023865 |
[4] | Kai Zhang, Yunpeng Ji, Qiuwei Pan, Yumei Wei, Yong Ye, Hua Liu . Sensitivity analysis and optimal treatment control for a mathematical model of Human Papillomavirus infection. AIMS Mathematics, 2020, 5(3): 2646-2670. doi: 10.3934/math.2020172 |
[5] | Ahmed Alshehri, Miled El Hajji . Mathematical study for Zika virus transmission with general incidence rate. AIMS Mathematics, 2022, 7(4): 7117-7142. doi: 10.3934/math.2022397 |
[6] | Naveed Iqbal, Ranchao Wu, Yeliz Karaca, Rasool Shah, Wajaree Weera . Pattern dynamics and Turing instability induced by self-super-cross-diffusive predator-prey model via amplitude equations. AIMS Mathematics, 2023, 8(2): 2940-2960. doi: 10.3934/math.2023153 |
[7] | Fahad Al Basir, Konstantin B. Blyuss, Ezio Venturino . Stability and bifurcation analysis of a multi-delay model for mosaic disease transmission. AIMS Mathematics, 2023, 8(10): 24545-24567. doi: 10.3934/math.20231252 |
[8] | Jinji Du, Chuangliang Qin, Yuanxian Hui . Optimal control and analysis of a stochastic SEIR epidemic model with nonlinear incidence and treatment. AIMS Mathematics, 2024, 9(12): 33532-33550. doi: 10.3934/math.20241600 |
[9] | Salma M. Al-Tuwairqi, Asma A. Badrah . Modeling the dynamics of innate and adaptive immune response to Parkinson's disease with immunotherapy. AIMS Mathematics, 2023, 8(1): 1800-1832. doi: 10.3934/math.2023093 |
[10] | Teekam Singh, Ramu Dubey, Vishnu Narayan Mishra . Spatial dynamics of predator-prey system with hunting cooperation in predators and type I functional response. AIMS Mathematics, 2020, 5(1): 673-684. doi: 10.3934/math.2020045 |
The study of drug side effects is a significant task in drug discovery. Candidate drugs with unaccepted side effects must be eliminated to prevent risks for both patients and pharmaceutical companies. Thus, all side effects for any candidate drug should be determined. However, this task, which is carried out through traditional experiments, is time-consuming and expensive. Building computational methods has been increasingly used for the identification of drug side effects. In the present study, a new path-based method was proposed to determine drug side effects. A heterogeneous network was built to perform such method, which defined drugs and side effects as nodes. For any drug and side effect, the proposed path-based method determined all paths with limited length that connects them and further evaluated the association between them based on these paths. The strong association indicates that the drug has a side effect with a high probability. By using two types of jackknife test, the method yielded good performance and was superior to some other network-based methods. Furthermore, the effects of one parameter in the method and heterogeneous network was analyzed.
Rice is a staple food for human consumption, especially Asians. Therefore, the production, consumption, and commerce of rice mostly occur in Asia. However, only 6% of rice is exported, and the remainder is consumed within the country. Unfortunately, sometimes the rice production does not meet the domestic demand since rice plants are infected with diseases. Brown spot disease is quite common that decreases the rice yield. It is caused by the spread of fungus called Bipolaris oryzae (Helminthosporium oryzae Breda de Haan.).
Every stage of the rice plant's life cycle makes it susceptible to numerous plant diseases. Diseases have the potential to lower rice crop production overall and in terms of quality. It is generally recognized that the pathogen can harm plants throughout several phases of storage, seed germination and seedling establishment, growth, and reproductive phase. One of fungal diseases infects rice is brown spot disease. Brown spot disease is a plant disease caused by a fungus that can reduce rice yield from around 4% to 52%. The disease disperses by letting the spore flow along with the wind and falls into the spikelet or seed. Consequently, the rice is damaged. Besides, the disease also leaves spots on rice seeds and causes low yield. In addition, if the infected seed is planted, the paddy field will be infected. The disease can be transmitted through seed and air, which are called seed-borne, and air-borne. Moreover, the fungus can live in the uncared field and poor soil. This means that the disease can invade the paddy field if it is not eradicated. Based on the problem, farmers need to control the disease by applying chemical substance, for example fungicide [1]. However, the chemical affects the environment and humans. Therefore, the strategy of controlling the spreading of disease is necessary.
Fungicide is a chemical that is used to restrain the growth of fungus spores. However, the fungicide not only affects the fungus spores but also affects agriculture products. There are three types of fungicide including: chemical fungicide, botanical fungicide, and biocontrol fungicide [8]. Since the chemical fungicide affects the environment and is poisonous to humans, fungicide containing natural extract such as botanical and biocontrol fungicide should be used. In the sense of fungus elimination, the botanical fungicide has higher performance than biocontrol fungicide. The mathematical model is a tool that can describe the spreading of disease that leads to the disease control strategy [9,10,11,12]. For example, the epidemiology mathematical model which considers the botanical fungicide is developed for controlling plant disease and preventive treatments of plants [7,13].
The SIR model [18,19,20], which has three compartment subclasses: susceptible, infected, and removed, is the general form of the epidemiological model. Mckendrick [21] proposed a fundamental model of SIR transmission in 1927. Song and Takeuchi used natural birth and death rates to study population dynamics. The plant population is separated into five compartments: susceptible, exposed, infected, post-infectious (removed), and protected with curative and preventive therapy, according to a mathematical model created and examined by Savary et al. [22]. The SIP model, or maize plant disease model, was presented by Windarto et al. [4].
In SIX model [2] studies the dynamics of susceptible plant population infected plant population pathogen population which in the actual situation that plants must be protected from fungus. This research selected the SIX model as a base for development using the real situation. Then, SIXP model was developed to match the situation we are interested in by adding protected plant populations denoted by P. After that, we analyzed the stability of the model developed for leaf brown spot disease with a standard incidence rate [15]. Then, we discussed the optimal control of fungicide to prevent the spread. Some numerical examples compare SIX model and SIXP model.
The proposed model is particularly well suited for describing diseases such as two infections in leaf brown spot disease and analysis the proposed model.
The proposed model is developed based on the SIX model [2], which is the mathematical model of the spreading of leaf brown spot disease in rice. We consider the effect of leaf brown spot disease on the rice population dynamics and add the rice population stage that are retreated with the botanical fungicide to protect them from the disease.
In this model, there are two types of population including plant population and pathogen population X. The plant population is classified into three categories: susceptible plant populations S, infected plant populations I, and protected plant populations P. The interaction between all populations is shown in Figure 1.
The compartment model can be written as the following system:
dSdt=κ−πS(t)+δP(t)−(RxX(t)+RcI(t))S(t)S(t)+I(t)−μS(t), | (1) |
dIdt=(RxX(t)+RcI(t))S(t)S(t)+I(t)−I(t)i−μI(t), | (2) |
dXdt=σI(t)−εX(t), | (3) |
dPdt=πS(t)−δP(t)−μP(t). | (4) |
The feasible region of the system in Eqs (1)–(4) is
Ω={(S,I,X,P)∈R4,S,I,X,P≥0,S+I+X+P≤κμ}. |
The parameters in Eqs (1)–(4) are positive. The meaning of the parameters is presented in Table 1.
Notation | Meaning | Unit |
κ | Planted rate of susceptible | NSites day-1 |
Rx | Rate of primary infection | day-1 |
Rc | Rate of secondary infection | day-1 |
μ | Natural death rate | day-1 |
1/i | Death rate caused by infection | day-1 |
σ | Growth rate of pathogen population | day-1 |
ε | Death rate of pathogen | day-1 |
π | The effectiveness of botanical fungicides | day-1 |
δ | Rate of damage to the fungicide given to the plant | day-1 |
The proposed model gives two equilibrium points, namely pathogen-free equilibrium (E0) and pathogen equilibrium (E1). The notation of pathogen-free equilibrium point is expressed by
E0(S0c,I0c,X0c,P0c)=E0(κ(δ+μ)μ(δ+μ+π),0,0,κπμ(δ+μ+π)). | (5) |
Another equilibrium point is E1(S∗c,I∗c,X∗c,P∗c), which is pathogen equilibrium, where
S∗c=κ(δ+μ)(μδ+μπ+μ2)+(μ+1i)(δ+μ)(iRxσ+Rcεiε(μi+1)−1),I∗c=κ(δ+μ)(iRxσ+Rcεiε(μi+1)−1)(μδ+μπ+μ2)+(μ+1i)(δ+μ)(iRxσ+Rcεiε(μi+1)−1),X∗c=σκ(δ+μ)(iRxσ+Rcεiε(μi+1)−1)ε[(μδ+μπ+μ2)+(μ+1i)(δ+μ)(iRxσ+Rcεiε(μi+1)−1)],P∗c=πκ(μδ+μπ+μ2)+(μ+1i)(δ+μ)(iRxσ+Rcεiε(μi+1)−1). | (6) |
The basic reproduction number (R0) is the number of secondarily infected plants that infect from only one initial infected plant. The value of R0 can calculates by the technique of Van Den Driessche and Watmough [16]
FV−1=[00000εRc+σRxε(μ+1i)Rxε000000000]. | (7) |
The dominant eigen value gives us R0,
R0=iRxσ+Rcεiε(μi+1). | (8) |
The systems of equations from model could determine the stability of their equilibrium points.
By substituting all parameters in the systems and found all equilibrium points. Then, linearizing the system at each equilibrium point and considering the stability of the system at the point.
Theorem 2.1. The pathogen-free equilibrium E0(κ(δ+μ)μ(δ+μ+π),0,0,κπμ(δ+μ+π)) of the system (1)–(4) is locally asymptotically stable when the basic reproduction number is less than one, and otherwise is unstable.
Proof. To show that the system of Eqs (1)–(4) is locally asymptotically stable, the Jacobian matrix is used to evaluate the pathogen-free equilibrium E0 as shown by
J(E0)=|−π−μ−λ−Rc−Rxδ0Rc−(1i+μ)−λRx00σ−ε−λ0π00−μ−δ−λ|. |
The eigenvalues of J(E0) are obtained by solving det(J(E0)−λI)=0. We receive the eigenvalues of J(E0) from the following characteristic equation
(λ+π+μ)(λ+μ+δ)[(λ−Rc+1i+μ)(λ+ε)−σRx]−π[−σRxδ+(λ+ε)δ(λ−Rc+1i+μ)]=0, |
(λ+a)(λ+b)[(λ−Rc+c)(λ+ε)−σRx]−π[−σRxδ+(λ+ε)δ(λ−Rc+c)]=0, |
−σRx[(λ+a)(λ+b)−πδ]+(λ−Rc+c)(λ+ε)[(λ+a)(λ+b)−πδ]=0, |
[(λ+a)(λ+b)−πδ][(λ−Rc+c)(λ+ε)−σRx]=0, |
(λ2+λa+λb+ab−πδ)[(λ−Rc+c)(λ+ε)−σRx]=0, |
(λ2+λ(a+b)+ab−πδ)[λ2+λ(c+ε−Rc)+(εc−εRc−σRx)]=0, |
where
a=π+μ,b=δ+μ,c=1i+μ. |
The eigenvalues of the Jacobian matrix for the pathogen-free equilibrium can be computed as
P1(λ1,2)=−(a+b)±√(a+b)2−4(ab−πδ)2;λ1=−(π+μ),λ2=−(δ+μ), |
P2(λ3,4)=−(c+ε−Rc)±√(c+ε−Rc)2−4(εc−εRc−σRx)2;λ3=−(1i+μ), |
λ4=−ε+Rc. |
It is obvious that all these eigenvalues are negative when ε > Rc.
By using the Routh-Hurwitz theorem [17], all eigenvalues are negative or complex eigenvalues with negative real part if and only if R0=(Rxσi+Rcεi)ε(1+μi)<1. Thus, the pathogen-free equilibrium point is locally asymptotically stable when the basic reproduction number is less than one.
Global stability of pathogen-free equilibrium is present in Theorem 2.2.
Theorem 2.2. The pathogen-free equilibrium E0 is globally asymptotically stable if R0>1, when I=0.
Proof. Let V is the Lyapunov function defined by
V(S,I,P)=g(SS0c)+I+g(PP0c), |
where, g(x)=x−1−lnx, which is positive function. Since I = 0, X = 0 then V≥0. We want to clarify that V is a positive function on the domain Ω.
The derivative of V respect to time evaluated at the solution of model in Eqs (1)–(4) is derived by
˙V=(1−S0cS)˙S+˙I+(1−P0cP)˙P=(1−S0cS)(κ−πS+δP−(RxX+RcI)SS+I−μS)+(RxX+RcI)SS+I−(Ii+μI)+(1−P0cP)(πS−δP−μP)=κ(1−S0cS)+πS0−δPS0cS+(RxX+RcI)S0cS+I−μS+μS0c−(Ii+μI)−πSP0cP+δP0c−μP+μP0c=κ(1−S0cS)+πS0−(πS−μP)S0cS+(RxX+RcI)S0cS+I−μS+μS0c−(Ii+μI)−P(δ+μ)P0cP+δP0c−μP+μP0c=κ(1−S0cS)+μPS0cS+(RxX+RcI)S0cS+I−μS+μS0c−(Ii+μI)−μP=κ(1−S0cS)+πSμS0c(δ+μ)S+(RxX+RcI)S0cS+I−μS+μS0c−(Ii+μI)−μP=κ(1−S0cS)+μS0(1+π(δ+μ))+(RxX+RcI)S0cS+I−(Ii+μI)−μ(P+S)=κ(1−S0cS)+μS0c((δ+μ+π)(δ+μ))+(RxX+RcI)S0cS+I−(Ii+μI)−μ(πS(δ+μ)+S)=μS0c(δ+μ+π)(δ+μ)(2−S0cS)+(RxX+RcI)S0cS+I−(Ii+μI)−μS((δ+μ+π)(δ+μ))=μS0c(δ+μ+π)(δ+μ)(2−S0cS−SS0c)+(1i+μ)I(i(RxX+RcI(μi+1)I)S0cS+I−1)=μS0c(δ+μ+π)(δ+μ)(2−S0cS−SS0c)+(1i+μ)I(i(RxσIε+RcI(μi+1)I)S0cS+I−1)=μS0c(δ+μ+π)(δ+μ)(2−S0cS−SS0c)+(1i+μ)I(i(Rxσ+εRcε(μi+1))S0cS+I−1)=μS0c(δ+μ+π)(δ+μ)(2−S0cS−SS0c)+(1i+μ)I(R0S0cS+I−1). |
From the relation between arithmetic and geometric means 2−S0S−SS0<0.
Considering the second term, in the case that R0 = 1, we have ˙V<0 if and only if I = 0.
From Eq (3), I(t)=0 causes X→0 as t→∞. Then, by using X(t) = 0 in Eq (1), we find S(t)→κ(δ+μ)μ(δ+μ+π) as t→∞.
In addition, when R0<1, we obtain ˙V<0 if and only if
S+I=κ(δ+μ)μ(δ+μ+π) |
or I=0, which is the largest compact invariant set of Eqs (1)–(4). Then, by using LaSalle's invariant principle, we can conclude that every solution of the mathematical model in Eqs (1)–(4) with an initial value in Ω tends to the pathogen-free equilibrium.
The stability of the pathogen equilibrium is presented in Theorem 3.
Theorem 2.3. If R0>1, the pathogen equilibrium E1 is locally asymptotic stable
Proof. Let J(E1) be the Jacobian matrix in Eqs (1)–(4) derived from the pathogen equilibrium E1 is expressed by
|(RxX∗c+RcI∗c)S∗c(S∗c+I∗c)2−(RxX∗c+RcI∗)S∗c+I∗c−μ−π−λ(RxX∗c+RcI∗c)S∗c(S∗c+I∗c)2−RcS∗cS∗c+I∗c−RxS∗cS∗c+I∗cδ−(RxX∗c+RcI∗c)S∗c(S∗c+I∗c)2+(RxX∗c+RcI∗)S∗c+I∗cRcS∗cS∗c+I∗c−1i−μ−(RxX∗c+RcI∗c)S∗c(S∗c+I∗c)2−λRxS∗cS∗c+I∗c00σ−ε−λ0π00−δ−μ−λ|=0 |
and
E1=(S∗c,S∗c(R0−1),σS∗c(R0−1)ε,πS∗cb), |
where
S∗c=κbd+bc(R0−1),b=δ+μ,c=1i+μ,d=[μδ+μπ+μ2],R0=(Rxσi+Rcεi)ε(1+μi). |
Eigenvalues of J(E1) satisfy the following characteristic equation
a0λ4+a1λ3+a2λ2+a3λ+a4=0, |
where
a1=a+b+q+ε+RxσεR0,a2=aε+bε+qε+qb+d+qaR0+cqR0[R0−1]+bRxσεR0+aRxσεR0,a3=dε+qεb+qaεR0+cqbR0[R0−1]+cqεR0[R0−1]+qabR0+dRxσεR0,a4=qεdR0+qεbcR0[R0−1]. |
where
a=π+μ,b=δ+μ,c=1i+μ,d=[μδ+μπ+μ2],q=(Rxσ+Rcε)ε[R0−1]R0,R0=(Rxσi+Rcεi)ε(1+μi). |
The eigenvalues have a negative real part, so the equilibrium point is stable with the conditions below:
a1,a3,a4>0,a1a2−a3>0,a1a2a3−a3a3−a1a1a4>0, |
which are related to the condition of Routh Hurwitz. Thus, the pathogen equilibrium point is stable when R0=(Rxσi+Rcεi)ε(1+μi)>1.
At endemic equilibrium point [14] in theorem we use of compound matrix theorem in order to address dynamics stability issues that arose from the solution of nonlinear differential equations.
Theorem 2.4. Assume that R0>1 and ε > Rc, the pathogen equilibrium E1 is globally asymptotic stable.
Proof. We prove the global asymptotic stability of the model (1)–(4) with endemic equilibrium point, we consider the non-linear equations in the model (1)–(4) for which the Jacobian matrix at disease-endemic equilibrium points is:
J(E1)=[(RxX∗c+RcI∗c)S∗c(S∗c+I∗c)2−(RxX∗c+RcI∗c)(S∗c+I∗c)−μ−π(RxX∗c+RcI∗c)S∗c(S∗c+I∗c)2−RcS∗cS∗c+I∗c−RxS∗cS∗c+I∗cδ−(RxX∗c+RcI∗c)S∗c(S∗c+I∗c)2+(RxX∗c+RcI∗)S∗c+I∗cRcS∗cS∗c+I∗c−1i−μ−(RxX∗c+RcI∗c)S∗c(S∗c+I∗c)2RxS∗cS∗c+I∗c00σ−ε0π00−δ−μ]. | (9) |
Furthermore, the general form of third additive compound matrix J|1|, is given by
J|1|=[j11+j22+j33j34−j24j14j43j11+j22+j44j23−j13−j42j32j11+j33+j44j12j41−j31j21j22+j33+j44], | (10) |
where
j11+j22+j33=(−2μ−π−ε−1i−(RxX∗c+RcI∗c)(S∗c+I∗c)+RcS∗cS∗c+I∗c),j11+j22+j44=(−3μ−π−δ−1i−(RxX∗c+RcI∗c)(S∗c+I∗c)+RcS∗cS∗c+I∗c),j11+j33+j44=(−2μ−δ−ε−π−(RxX∗c+RcI∗c)(S∗c+I∗c)+(RxX∗c+RcI∗c)S∗c(S∗c+I∗c)2),j22+j33+j44=(−2μ−1i−δ−ε−(RxX∗c+RcI∗c)S∗c(S∗c+I∗c)2+RcS∗cS∗c+I∗c). |
From matrices (9) and (10) implies that
J|1|=[−j1100δ0−j22RxS∗cS∗c+I∗cRxS∗cS∗c+I∗c0σ−j33(RxX∗c+RcI∗c)S∗c(S∗c+I∗c)2−RcS∗cS∗c+I∗cπ0−(RxX∗c+RcI∗c)S∗c(S∗c+I∗c)2+(RxX∗c+RcI∗)S∗c+I∗c−j44], | (11) |
where
−j11=−(2μ+π+ε+1i+(RxX∗c+RcI∗c)(S∗c+I∗c)−RcS∗cS∗c+I∗c),−j22=−(3μ+π+δ+1i+(RxX∗c+RcI∗c)(S∗c+I∗c)−RcS∗cS∗c+I∗c),−j33=−(2μ+ε+π+δ+(RxX∗c+RcI∗c)(S∗c+I∗c)−(RxX∗c+RcI∗c)S∗c(S∗c+I∗c)2),−j44=−(2μ+1i+δ+ε+(RxX∗c+RcI∗c)S∗c(S∗c+I∗c)2−RcS∗cS∗c+I∗c). |
Consider P(χ)=diag{P(t),X(t),I(t),S(t)}, the inverse of P(χ) is given as
P−1(χ)=diag{1P(t),1X(t),1I(t),1S(t)}, |
the derivative with respect to time is
Pf(χ)=diag{•P(t),•X(t),•I(t),•S(t)}, |
while
PfP−1=diag{•P(t)P(t),•X(t)X(t),•I(t)I(t),•S(t)S(t)}, |
and
PJ|1|P−1=[−j1100δP∗cS∗c0−j22X∗cRxS∗cI∗c(S∗c+I∗c)X∗cRxS∗cS∗c(S∗c+I∗c)0σI∗cX∗c−j33I∗cS∗c((RxX∗c+RcI∗c)S∗c(S∗c+I∗c)2−RcS∗cS∗c+I∗c)πS∗cP∗c0S∗cI∗c[(RxX∗c+RcI∗c)I∗c(S∗c+I∗c)2]−j44]. |
So that
M=PfP−1+PJ|1|P−1, |
M=[˙P(t)P(t)−j1100δP∗cS∗c0˙X(t)X(t)−j22X∗cRxS∗cI∗c(S∗c+I∗c)X∗cRxS∗cS∗c(S∗c+I∗c)0σI∗cX∗c˙I(t)I(t)−j33I∗cS∗c((RxX∗c+RcI∗c)S∗c(S∗c+I∗c)2−RcS∗cS∗c+I∗c)πS∗cP∗c0S∗cI∗c[(RxX∗c+RcI∗c)I∗c(S∗c+I∗c)2]˙S(t)S(t)−j44]. |
From the system of equation, we obtain
δP∗cS∗c=˙S(t)S(t)−κS+π+(RxX∗c+RcI∗c)(S∗c+I∗c)+μ,σI∗cX∗c=˙X(t)X(t)+ε,πS∗cP∗c=˙P(t)P(t)+δ+μ. |
Now, consequently we are to find ˉhi(t),i=1,2,3,4, by assuming that Mij are the entries of matrix M, such that
ˉh1(t)=M11+4∑j≠1∧j=2|M1j|, |
ˉh1(t)=˙P(t)P(t)−(2μ+π+ε+1i+(RxX∗c+RcI∗c)(S∗c+I∗c)−RcS∗cS∗c+I∗c)+|δP∗cS∗c|, |
ˉh1(t)=˙P(t)P(t)−(2μ+π+ε+1i)−(RxX∗c+RcI∗c)(S∗c+I∗c)+RcS∗cS∗c+I∗c+δP∗cS∗c, |
ˉh1(t)=˙P(t)P(t)−(2μ+π+ε+1i)−(RxX∗c+RcI∗c)(S∗c+I∗c)+RcS∗cS∗c+I∗c+˙S(t)S(t)−κS+π+(RxX∗c+RcI∗c)(S∗c+I∗c)+μ, |
ˉh1(t)=˙P(t)P(t)+˙S(t)S(t)−(μ+1i+κS∗c)−ε+RcR0, |
ˉh1(t)≤˙P(t)P(t)+˙S(t)S(t)−(μ+1i+ε). |
ˉh2(t)=M22+∑4j=1∧j≠2|M2j|, |
ˉh2(t)=˙X(t)X(t)−(3μ+π+δ+1i+(RxX∗c+RcI∗c)(S∗c+I∗c)−RcS∗cS∗c+I∗c)+|X∗cRxS∗cI∗c(S∗c+I∗c)|+|X∗cRxS∗cS∗c(S∗c+I∗c)|, |
ˉh2(t)=˙X(t)X(t)−(3μ+π+δ+1i)−(RxX∗c+RcI∗c)S∗c+I∗c+RcS∗cS∗c+I∗c+X∗cRxS∗cI∗c(S∗c+I∗c)+X∗cRxI∗cI∗c(S∗c+I∗c), |
ˉh2(t)=˙X(t)X(t)−(3μ+π+δ+1i)−(RxX∗c+RcI∗c)S∗c+I∗c+RcS∗cS∗c+I∗c+X∗cRxI∗c[S∗c(S∗c+I∗c)+I∗c(S∗c+I∗c)], |
ˉh2(t)=˙X(t)X(t)−(3μ+π+δ+1i)−(RxX∗c+RcI∗c)S∗c+I∗c+RcS∗cS∗c+I∗c+X∗cRxI∗c, |
ˉh2(t)=˙X(t)X(t)−(3μ+π+δ+1i)−(RxX∗c+RcI∗c)S∗c+I∗c+RcS∗cS∗c+I∗c+σS∗c(R0−1)εRxS∗c(R0−1), |
ˉh2(t)=˙X(t)X(t)−(3μ+π+δ+1i)−(RxX∗c+RcI∗c)S∗c+I∗c+RcS∗cS∗c+I∗c+Rxσε(S∗c+I∗c)(S∗c+I∗c), |
ˉh2(t)=˙X(t)X(t)−(3μ+π+δ+1i)−(RxX∗c+RcI∗c)S∗c+I∗c+RcS∗cS∗c+I∗c+RxσS∗cε(S∗c+I∗c)+RxσI∗cε(S∗c+I∗c), |
ˉh2(t)=˙X(t)X(t)−(2μ+π+δ)−(RxX∗c+RcI∗c)S∗c+I∗c+RxσI∗cε(S∗c+I∗c)+Rxσ+εRcεR0−1i−μ, |
ˉh2(t)=˙X(t)X(t)−(2μ+π+δ)−(RxX∗c+RcI∗c)S∗c+I∗c+RxX∗c(S∗c+I∗c), |
ˉh2(t)=˙X(t)X(t)−(2μ+π+δ+RcI∗cS∗c+I∗c), |
ˉh2(t)≤˙X(t)X(t)−(2μ+π+δ). |
ˉh3(t)=M33+4∑j=1∧j≠3|M3j|, |
ˉh3(t)=˙I(t)I(t)−(2μ+ε+π+δ+(RxX∗c+RcI∗c)(S∗c+I∗c)−(RxX∗c+RcI∗c)S∗c(S∗c+I∗c)2)+|σI∗cX∗c|+|I∗cS∗c((RxX∗c+RcI∗c)S∗c(S∗c+I∗c)2−RcS∗cS∗c+I∗c)|, |
ˉh3(t)=˙I(t)I(t)−(2μ+ε+π+δ)−(RxX∗c+RcI∗c)I∗c(S∗c+I∗c)2+σI∗cX∗c+((RxX∗c+RcI∗c)I∗c(S∗c+I∗c)2−RcI∗cS∗c+I∗c), |
ˉh3(t)=˙I(t)I(t)−(2μ+ε+π+δ)+σI∗cX∗c−RcI∗cS∗c+I∗c˙I(t)I(t), |
ˉh3(t)=˙I(t)I(t)−(2μ+ε+π+δ)+˙X(t)X(t)+ε−RcI∗cS∗c+I∗c, |
ˉh3(t)=˙I(t)I(t)+˙X(t)X(t)−(2μ+π+δ+RcI∗cS∗c+I∗c), |
ˉh3(t)≤˙I(t)I(t)+˙X(t)X(t)−(2μ+π+δ). |
ˉh4(t)=M44+4∑j=1∧j≠3|M4j| |
ˉh4(t)=˙S(t)S(t)−(2μ+1i+δ+ε+(RxX∗c+RcI∗c)S∗c(S∗c+I∗c)2−RcS∗cS∗c+I∗c)+|πS∗cP∗c|+|S∗cI∗c[(RxX∗c+RcI∗c)I∗c(S∗c+I∗c)2]|, |
ˉh4(t)=˙S(t)S(t)−(2μ+1i+δ+ε)−(RxX∗c+RcI∗c)S∗c(S∗c+I∗c)2+RcS∗cS∗c+I∗c+πS∗cP∗c+S∗cI∗c[(RxX∗c+RcI∗c)I∗c(S∗c+I∗c)2], |
ˉh4(t)=˙S(t)S(t)−(2μ+1i+δ+ε)+RcS∗cS∗c+I∗c+πS∗cP∗c, |
ˉh4(t)=˙S(t)S(t)−(2μ+1i+δ+ε)+RcS∗cS∗c+I∗c+˙P(t)P(t)+δ+μ, |
ˉh4(t)=˙S(t)S(t)+˙P(t)P(t)−(μ+1i)−ε+RcR0, |
ˉh4(t)≤˙S(t)S(t)+˙P(t)P(t)−(μ+1i+ε). |
Now, in R4 we assume a vector (m1,m2,m3,m4). The Lozinskii measure l(M) is defined as l(M)=ˉhi(t), i=1,2,3,4. The integration of the Lozinskii measure l(M) and taking the limits as t→∞ lead to the following equations.
g1=limt→∞supsup1t∫t0ˉh1(t)dt≤1tlimt→∞supsup1t∫t0˙P(t)P(t)+˙S(t)S(t)−(μ+1i+ε)dt<−(μ+1i+ε), | (12) |
g2=limt→∞supsup1t∫t0ˉh2(t)dt≤1tlimt→∞supsup1t∫t0˙X(t)X(t)−(2μ+π+δ)dt<−(2μ+π+δ), | (13) |
g3=limt→∞supsup1t∫t0ˉh3(t)dt≤1tlimt→∞supsup1t∫t0˙I(t)I(t)+˙X(t)X(t)−(2μ+π+δ)dt<−(2μ+π+δ), | (14) |
g4=limt→∞supsup1t∫t0ˉh4(t)dt≤1tlimt→∞supsup1t∫t0˙S(t)S(t)+˙P(t)P(t)−(μ+1i+ε)dt<−(μ+1i+ε). | (15) |
Now, the combination of inequalities from Eqs (12)–(15) and by the assumption ε > Rc, we can assert that
g=limt→0supsup1t∫t0l(M)dt<0. |
The system containing only four non-linear equations of model Eqs (1)–(4) is globally asymptotically stable around its interior equilibrium (S∗c,I∗c,X∗c,P∗c).
The basic parameters are carried on the sensitivity analysis. They are checked and identified the parameter that can impact the basic reproduction number. The sensitivity analysis is informed that each parameter's significance is disease transmission for control the spread of brown disease on leaf brown disease. The explicit expression of R0 is given by
R0=(Rxσi+Rcεi)ε(1+μi). |
Definition 2.1. The normalized forward sensitivity index of a variable, u, that depends differentiable on a parameter, p, is defined as:
ψup=(∂u∂p)(pu). |
The sensitivity indices of the basic reproductive number calculate by used parameters of endemic equilibrium point that are shown in Table 2 [2].
Notation | Biological meaning | Value |
Rx | Rate of primary infection | 0.1890 |
Rc | Rate of secondary infection | 0.8110 |
μ | Natural death rate | -0.1362 |
i | Death rate of infect | 0.8638 |
σ | Growth rate of the pathogen population | 0.1890 |
ε | Death rate of pathogen | - 0.1890 |
To consider the relationship between the primary infection (Rx) and the rate of and death rate of pathogen (ε), it was found that the basic reproductive number will increase when Rx increased at the tiny ε as shown in Figure 2a.
To consider the relationship between the rate of secondary infection (Rc) and the growth rate of pathogen (σ), it was found that the basic reproductive number will increase when Rc and σ increased as shown in Figure 2b.
To consider the relationship between the rate of secondary infection (Rc) and the primary infection (Rx), it was found that the basic reproductive number will increase when Rc increases. Despite the increase of Rx, the value of R0 marginally increased as shown in Figure 2c.
To consider the relationship between the natural death rate (μ) and rate of secondary infection (Rc), it was found that the basic reproductive number will increase when Rc and increased at the tiny μ as shown in Figure 2d.
The spreading of leaf brown spot disease is controlled by using the botanical fungicide. The goal is to decrease the amount of infected rice. Therefore, Pontryagin's principle, shown below, is used to control the number of rice [5,8,10]:
J(u)=∫tft0[A0I(t)+A1u2(t)]dt. |
Where tf is the final time, A0 is balancing constant coefficients of the infected plant while A1 is weight coefficients for control measure and variable u(t) is the control variable of the preventive treatment. With the objective function J(u). The goal is to find the optimal control u∗(t) such that:
J(u∗)=min{J(u)}. |
The state variable for the model
x(t)=[S(t)I(t)X(t)P(t)], |
and the constraint:
dSdt=κ−uπS+δP−(RxX+RcI)SS+I−μS, |
dIdt=(RxX+RcI)SS+I−μI−Ii, |
dXdt=σI−εX, |
dPdt=uπS−δP−μP. |
The system should satisfy the condition:
0≤u(t)≤1,0≤t≤tf,S(0)=S0>0,I(0)=I0≥0,X(0)=X0≥0,P(0)=P0≥0. |
We define Hamiltonian function as H=f(x,u,t)+λ'g(x,u,t), which equivalent to:
H=A0I(t)+A1u2(t)+λ1(κ−uπS+δP−(RxX+RcI)SS+I−μS) |
+λ2((RxX+RcI)SS+I−μI−Ii)+λ3(σI−εX)+λ4(uπS−δP−μP), |
where λ1(t),λ2(t),λ3(t),λ4(t) are the co-state variable or the Lagrange multiplier of the optimization problem. The necessary conditions that an optimal control is archived, it must satisfy the following Pontryagin's principle:
● State equation:
•S=∂H∂•λ1=κ−uπS+δP−(RxX+RcI)SS+I−μS, |
•I=∂H∂•λ2=(RxX+RcI)SS+I−μI−Ii, |
•X=∂H∂•λ3=σI−εX, |
•P=∂H∂•λ4=uπS−δP−μP. |
● Co-state equation:
•λ1=−∂H∂S=λ1(uπ+(RxX+RcI)(S+I)−(RxX+RcI)S(S+I)2+μ)−λ2((RxX+RcI)(S+I)−(RxX+RcI)S(S+I)2)−λ4uπ, |
•λ2=−∂H∂I=−A0−λ1((RxX+RcI)S(S+I)2−RcS(S+I))+λ2((RxX+RcI)S(S+I)2−RcS(S+I)2+μ+1i)−λ3σ, |
•λ3=−∂H∂X=(λ1−λ2)(RxSS+I)+λ3ε, |
•λ4=−∂H∂P=λ4(δ+μ)−λ1δ. |
● Transversality conditions:
λ1(tf)=λ2(tf)=λ3(tf)=λ4(tf)=0, |
for t0≤t≤tf
● Stationer condition
∂H∂u=0, |
then
u=(λ1−λ4)πS2A1. |
Since 0≤u(t)≤1, then we get:
u=min{max(0,(λ1−λ4)πS2A1),1}, |
as the optimal control of the system.
In this section, we present some numerical simulations to ensure the analytic results by considering the pathogen-free and pathogen condition. For simulation that uses pathogen-free situation, the initial conditions using for calculation are denoted as S(0) = 200, I(0) = 400, X(0) = 400, P(0) = 0 and ε > Rc. Here, the proposed model is simulated from initial time to 140 days. The models were simulated by RK4 method with step size 0.01 which guaranteed the stability of the numerical solutions. The parameters and initial data in each case of simulation were shown in Table 3. There were two results of the simulation. The first results showed the dynamic model for pathogen-free. The second results showed dynamics model for the pathogen, was shown in Figures 3 and 4 respectively.
Notation | Biological meaning | Valu R0 < 1 | Value R0 > 1 | Unit | References |
κ | Planted rate of susceptible | 10 | 10 | NSites day-1 | [2] |
Rx | Rate of primary infection | 0.05 | 0.4 | day-1 | [2] |
Rc | Rate of secondary infection | 0.05 | 0.1 | day-1 | [2] |
μ | Natural death rate | 0.0083 | 0.0083 | day-1 | [2] |
1/i | Death rate of infect | 1/19 | 1/19 | day-1 | [6] |
σ | Growth rate of the pathogen | 0.0072 | 0.0072 | day-1 | [3] |
ε | Death rate of pathogen | 0.1236 | 0.1236 | day-1 | [4] |
π | The effective of botanical fungicides | 0.1 | 0.1 | day-1 | [8] |
δ | The rate of damage to the botanical fungicide given to the plant host | 0.001 | 0.001 | day-1 | [8] |
In case of the disease-free condition, the basic reproductive number is not greater than one that gives the disease-free equilibrium (E0), which is local asymptotically stable. The model is used the basic reproductive number about 0.8684. The simulation was shown the number of infectious, pathogen and susceptible sites decreased but the protected plant increased. The solution converges to the disease-free state, which means the infection and pathogen tend to zero when there is appropriate time as shown in Figure 3.
The model is used the basic reproductive number about 2.0236 in case of endemic condition, which is greater than one. So, it leads to the endemic equilibrium (E1) giving the global asymptotically stable when R0 is greater than 1. The simulation was shown that the infectious, pathogen and susceptible sites converged to the endemic equilibrium steady state when there is a suitable long time while the number of the protected plant increased. The solution curve tends to the endemic equilibrium as shown in Figure 4.
In Figures 5 and 6, the result of susceptible plant with control is lower than that without control because the susceptible site is moved to the protected site as shown in Figure 5. In the same way, the result of infected plant with control is lower than in case of without control because the infected site was protected by botanical fungicide as shown in Figure 6. Therefore, these results can be interpreted that the botanical fungicide can reduce the spread of leaf brown spot disease.
In Figure 7 show the proportion of fungicide used to protect plant from fungi. Here we choose A0=2,A1=5 using optimal control requires to maintain the control at 100% for 119 days before dropping to its lower bound. it appears that in the presence of fungicide control, susceptible plant and protected plant will grow more than without fungicide control.
In this paper, we have presented and analyzed a differential system of the SIXP model. The model has two equilibrium points. The disease-free equilibrium (E0) and endemic equilibrium (E1). It is found that R0<1 the disease-free equilibrium is locally asymptotically stable as guaranteed by Theorem 2.1. The Lyapunov function theory is used for disease-free equilibrium that is globally asymptotically stable if R0<1 the as guaranteed by Theorem 2.2. The epidemic equilibrium of the model is locally asymptotically stable when R0>1 as guaranteed by Theorem 2.3. The Lozinskii measure and additive compound Metrix Theorem is used to address dynamics stability issues that arose from the solution of nonlinear differential equations. If R0>1, the endemic equilibrium is globally asymptotic stable as guaranteed by Theorem 2.4.
As a result, we discovered that the numerical simulation results verified the analytical findings of the propagation of the leaf brown spot disease in rice with standard incidence rate. The occurrence of brown spot illnesses in rice can be represented by the developed model. The endemic equilibrium and the disease-free equilibrium are the two equilibrium points in the SIXP models. Constructing a suitable Lyapunov function is observed that the global asymptotic stability of the disease-free equilibrium depends on the basic reproduction number (R0). If R0<1 the, then the endemic equilibrium is globally asymptotically stable by using the estimate of the Lozinskii measure applied to the systems. The sensitivity indices of the reproductive number and endemic equilibrium are determined and optimal control strategy. Parameters in the model illustrate that to reduce the spreading of leaf brown spot disease, the parameters Rc, Rx, i, σ must be reduced. In practice, botanical fungicide is one factor that can reduce the value of Rc, Rx, i, σ. The control plots we developed indicate that the site of susceptible and infected decreased in the optimality system. The results show that the modified model by considering the botanical fungicide can reduce the spreading of leaf brown spot disease. In addition, the numerical simulations were also to support the theoretical hypothesis and approach epidemic control. The study results suggest that the botanical fungicide can reduce the spreading of leaf brown spot disease.
The authors would like to express their gratitude to King Mongkut's University of Technology Thonburi, Thailand, for funding this research, the Science Achievement Scholarship of Thailand, Thailand Science Research and Innovation (TSRI) Basic Research Fund: Fiscal year 2022, and Ubon Ratchathani Rajabhat University, Thailand for supporting this research.
The authors declare no conflicts of interest.
[1] |
S. Shabani-Mashcool, S. A. Marashi, S. Gharaghani, NDDSA: A network- and domain-based method for predicting drug-side effect associations, Inform. Process. Manag., 57 (2020), 102357. https://doi.org/10.1016/j.ipm.2020.102357 doi: 10.1016/j.ipm.2020.102357
![]() |
[2] |
Y. J. Ding, J. J. Tang, F. Guo, Identification of drug-side effect association via multiple information integration with centered kernel alignment, Neurocomputing, 325 (2019), 211–224. https://doi.org/10.1016/j.neucom.2018.10.028 doi: 10.1016/j.neucom.2018.10.028
![]() |
[3] |
A. Lakizadeh, S. M. H. Mir-Ashrafi, Drug repurposing improvement using a novel data integration framework based on the drug side effect, Inform. Med. Unlocked, 23 (2021), 100523. https://doi.org/10.1016/j.imu.2021.100523 doi: 10.1016/j.imu.2021.100523
![]() |
[4] |
E. Pauwels, V. Stoven, Y. Yamanishi, Predicting drug side-effect profiles: a chemical fragment-based approach, BMC Bioinformatics, 12 (2011), 169. https://doi.org/10.1186/1471-2105-12-169 doi: 10.1186/1471-2105-12-169
![]() |
[5] |
S. Jamal, S. Goyal, A. Shanker, A. Grover, Predicting neurological adverse drug reactions based on biological, chemical and phenotypic properties of drugs using machine learning models, Sci. Rep., 7 (2017), 872. https://doi.org/10.1038/s41598-017-00908-z doi: 10.1038/s41598-017-00908-z
![]() |
[6] |
Y. Zheng, H. Peng, S. Ghosh, C. Lan, J. Li, Inverse similarity and reliable negative samples for drug side-effect prediction, BMC Bioinformatics, 19 (2019), 554. https://doi.org/10.1186/s12859-018-2563-x doi: 10.1186/s12859-018-2563-x
![]() |
[7] |
M. Liu, Y. Wu, Y. Chen, J. Sun, Z. Zhao, X. W. Chen, et al., Large-scale prediction of adverse drug reactions using chemical, biological, and phenotypic properties of drugs, J. Am. Med. Inform. Assoc., 19 (2012), e28–35. https://doi.org/10.1136/amiajnl-2011-000699 doi: 10.1136/amiajnl-2011-000699
![]() |
[8] |
S. Dey, H. Luo, A. Fokoue, J. Hu, P. Zhang, Predicting adverse drug reactions through interpretable deep learning framework, BMC Bioinformatics, 19 (2018), 476. https://doi.org/10.1186/s12859-018-2544-0 doi: 10.1186/s12859-018-2544-0
![]() |
[9] |
L. Chen, T. Huang, J. Zhang, M. Y. Zheng, K. Y. Feng, Y. D. Cai, et al., Predicting drugs side effects based on chemical-chemical interactions and protein-chemical interactions, BioMed Res. Int., 2013 (2013), 485034. https://doi.org/10.1155/2013/485034 doi: 10.1155/2013/485034
![]() |
[10] |
W. Zhang, F. Liu, L. Luo, J. Zhang, Predicting drug side effects by multi-label learning and ensemble learning, BMC Bioinformatics, 16 (2015), 365. https://doi.org/10.1186/s12859-015-0774-y doi: 10.1186/s12859-015-0774-y
![]() |
[11] |
N. Atias, R. Sharan, An algorithmic framework for predicting side effects of drugs, J. Comput. Biol., 18 (2011), 207–218. https://doi.org/10.1089/cmb.2010.0255 doi: 10.1089/cmb.2010.0255
![]() |
[12] |
E. Muñoz, V. Novácek, P. Y. Vandenbussche, Facilitating prediction of adverse drug reactions by using knowledge graphs and multi-label learning models, Brief. Bioinform., 20 (2017), 190–202. https://doi.org/10.1093/bib/bbx099 doi: 10.1093/bib/bbx099
![]() |
[13] | W. Zhang, Y. Chen, S. Tu, F. Liu, Q. Qu, Drug side effect prediction through linear neighborhoods and multiple data source integration, in IEEE International Conference on Bioinformatics and Biomedicine, (2016), 427–434. https://doi.org/10.1109/BIBM.2016.7822555 |
[14] | E. Munoz, V. Novacek, P. Y. Vandenbussche, Using drug similarities for discovery of possible adverse reactions, AMIA Annu. Symp. Proc., 2016 (2016), 924–933. |
[15] |
X. Zhao, L. Chen, J. Lu, A similarity-based method for prediction of drug side effects with heterogeneous information, Math. Biosci., 306 (2018), 136–144. https://doi.org/10.1016/j.mbs.2018.09.010 doi: 10.1016/j.mbs.2018.09.010
![]() |
[16] |
H. Liang, L. Chen, X. Zhao, X. Zhang, Prediction of drug side effects with a refined negative sample selection strategy, Comput. Math. Method. M., 2020 (2020), 1573543. https://doi.org/10.1155/2020/1573543 doi: 10.1155/2020/1573543
![]() |
[17] |
X. Zhao, L. Chen, Z. H. Guo, T. Liu, Predicting drug side effects with compact integration of heterogeneous networks, Curr. Bioinform., 14 (2019), 709–720. https://doi.org/10.2174/1574893614666190220114644 doi: 10.2174/1574893614666190220114644
![]() |
[18] |
X. Guo, W. Zhou, Y. Yu, Y. Ding, J. Tang, F. Guo, A novel triple matrix factorization method for detecting drug-side effect association based on kernel target alignment, BioMed Res. Int., 2020 (2020), 4675395. https://doi.org/10.1155/2020/4675395 doi: 10.1155/2020/4675395
![]() |
[19] |
Y. Ding, J. Tang, F. Guo, Identification of drug-side effect association via semi-supervised model and multiple kernel learning, IEEE J. Biomed. Health, 23 (2019), 2619–2632. https://doi.org/10.1109/JBHI.2018.2883834 doi: 10.1109/JBHI.2018.2883834
![]() |
[20] | H. Tong, C. Faloutsos, J. Pan, Fast random walk with restart and its applications, in Sixth International Conference on Data Mining, (2006), 613–622. https://doi.org/10.1109/ICDM.2006.70 |
[21] |
D. E. Carlin, B. Demchak, D. Pratt, E. Sage, T. Ideker, Network propagation in the cytoscape cyberinfrastructure, PLoS Comput. Biol., 13 (2017), e1005598. https://doi.org/10.1371/journal.pcbi.1005598 doi: 10.1371/journal.pcbi.1005598
![]() |
[22] |
M. Kuhn, M. Campillos, I. Letunic, L. J. Jensen, P. Bork, A side effect resource to capture phenotypic effects of drugs, Mol. Syst. Biol., 6 (2010), 343. https://doi.org/10.1038/msb.2009.98 doi: 10.1038/msb.2009.98
![]() |
[23] |
M. Kuhn, D. Szklarczyk, S. Pletscher-Frankild, T. H. Blicher, C. von Mering, L. J. Jensen, et al., STITCH 4: integration of protein–chemical interactions with user data, Nucleic Acids Res., 42 (2014), D401–D407. https://doi.org/10.1093/nar/gkt1207 doi: 10.1093/nar/gkt1207
![]() |
[24] |
D. Weininger, SMILES, a chemical language and information system. 1. Introduction to methodology and encoding rules, J. Chem. Inf. Comput. Sci., 28 (1988), 31–36. https://doi.org/10.1021/ci00057a005 doi: 10.1021/ci00057a005
![]() |
[25] |
X. Xiao, W. Zhu, B. Liao, J. Xu, C. Gu, B. Ji, et al., BPLLDA: predicting lncRNA-Disease associations based on simple paths with limited lengths in a heterogeneous network, Front. Genet., 9 (2018), 411. https://doi.org/10.3389/fgene.2018.00411 doi: 10.3389/fgene.2018.00411
![]() |
[26] |
W. Ba-Alawi, O. Soufan, M. Essack, P. Kalnis, V. B. Bajic, DASPfind: new efficient method to predict drug-target interactions, J. Cheminformatics, 8 (2016), 15. https://doi.org/10.1186/s13321-016-0128-4 doi: 10.1186/s13321-016-0128-4
![]() |
[27] |
Z. H. You, Z. A. Huang, Z. Zhu, G. Y. Yan, Z. W. Li, Z. Wen, et al., PBMDA: a novel and effective path-based computational model for miRNA-disease association prediction, PLoS Comput. Biol., 13 (2017), e1005455. https://doi.org/10.1371/journal.pcbi.1005455 doi: 10.1371/journal.pcbi.1005455
![]() |
[28] |
J. Gao, B. Hu, L. Chen, A path-based method for identification of protein phenotypic annotations, Curr. Bioinform., 16 (2021), 1214–1222. https://doi.org/10.2174/1574893616666210531100035 doi: 10.2174/1574893616666210531100035
![]() |
[29] |
S. Kohler, S. Bauer, D. Horn, P. N. Robinson, Walking the interactome for prioritization of candidate disease genes, Am. J. Hum. Genet., 82 (2008), 949–958. https://doi.org/10.1016/j.ajhg.2008.02.013 doi: 10.1016/j.ajhg.2008.02.013
![]() |
[30] |
Y.J. Li, J. C. Patra, Genome-wide inferring gene-phenotype relationship by walking on the heterogeneous network, Bioinformatics, 26 (2010), 1219–1224. https://doi.org/10.1093/bioinformatics/btq108 doi: 10.1093/bioinformatics/btq108
![]() |
[31] |
X. Chen, M. X. Liu, G. Y. Yan, Drug-target interaction prediction by random walk on the heterogeneous network, Mol. BioSyst., 8 (2012), 1970–1978. https://doi.org/10.1039/C2MB00002D doi: 10.1039/C2MB00002D
![]() |
[32] |
L. Chen, T. Liu, X. Zhao, Inferring anatomical therapeutic chemical (ATC) class of drugs using shortest path and random walk with restart algorithms, BBA Mol. Basis Dis., 1864 (2017), 2228–2240. https://doi.org/10.1016/j.bbadis.2017.12.019 doi: 10.1016/j.bbadis.2017.12.019
![]() |
[33] |
L. Chen, Y. H. Zhang, Z. Zhang, T. Huang, Y. D. Cai, Inferring novel tumor suppressor genes with a protein-protein interaction network and network diffusion algorithms, Mol. Ther. Methods Clin. Dev., 10 (2018), 57–67. https://doi.org/10.1016/j.omtm.2018.06.007 doi: 10.1016/j.omtm.2018.06.007
![]() |
[34] |
S. Lu, K. Zhao, X. Wang, H. Liu, X. Ainiwaer, Y. Xu, et al., Use of laplacian heat diffusion algorithm to infer novel genes with functions related to uveitis, Front. Genet., 9 (2018), 425. https://doi.org/10.3389/fgene.2018.00425 doi: 10.3389/fgene.2018.00425
![]() |
[35] |
H. Y. Liang, B. Hu, L. Chen, S. Q. Wang, Aorigele, Recognizing novel chemicals/drugs for anatomical therapeutic chemical classes with a heat diffusion algorithm, BBA Mol. Basis. Dis., 1866 (2020), 165910. https://doi.org/10.1016/j.bbadis.2020.165910 doi: 10.1016/j.bbadis.2020.165910
![]() |
[36] |
M. Imanishi, Y. Hori, M. Nagaoka, Y. Sugiura, Design of novel zinc finger proteins: towards artificial control of specific gene expression, Eur. J. Pharm. Sci., 13 (2001), 91–97. https://doi.org/10.1016/S0928-0987(00)00212-8 doi: 10.1016/S0928-0987(00)00212-8
![]() |
[37] |
M. Alirezaei, E. Mordelet, N. Rouach, A. C. Nairn, J. Glowinski, J. Premont, Zinc-induced inhibition of protein synthesis and reduction of connexin-43 expression and intercellular communication in mouse cortical astrocytes, Eur. J. Neurosci., 16 (2002), 1037–1044. https://doi.org/10.1046/j.1460-9568.2002.02180.x doi: 10.1046/j.1460-9568.2002.02180.x
![]() |
[38] |
K. H. Ibs, L. Rink, Zinc-altered immune function, J. Nutr., 133 (2003), 1452s–1456s. https://doi.org/10.1093/jn/133.5.1452S doi: 10.1093/jn/133.5.1452S
![]() |
[39] |
Z. A. Bhutta, R. E. Black, K. H. Brown, J. M. Gardner, S. Gore, A. Hidayat, et al., Prevention of diarrhea and pneumonia by zinc supplementation in children in developing countries: Pooled analysis of randomized controlled trials, J. Pediatr., 135 (1999), 689–697. https://doi.org/10.1016/S0022-3476(99)70086-7 doi: 10.1016/S0022-3476(99)70086-7
![]() |
[40] |
D. E. Roth, S. A. Richard, R. E. Black, Zinc supplementation for the prevention of acute lower respiratory infection in children in developing countries: meta-analysis and meta-regression of randomized trials, Int. J. Epidemiol., 39 (2010), 795–808. https://doi.org/10.1093/ije/dyp391 doi: 10.1093/ije/dyp391
![]() |
[41] |
D. Hulisz, Efficacy of zinc against common cold viruses: an overview, J. Am. Pharm. Assoc., 44 (2004), 594–603. https://doi.org/10.1331/1544-3191.44.5.594.Hulisz doi: 10.1331/1544-3191.44.5.594.Hulisz
![]() |
[42] |
R. O. Suara, J. E. Crowe, Effect of zinc salts on respiratory syncytial virus replication, Antimicrob. Agents Ch., 48 (2004), 783–790. https://doi.org/10.1128/AAC.48.3.783-790.2004 doi: 10.1128/AAC.48.3.783-790.2004
![]() |
[43] | D. Li, L. Z. Wen, H. Yuan, Observation on clinical efficacy of combined therapy of zinc supplement and jinye baidu granule in treating human cytomegalovirus infection, Zhongguo Zhong xi yi jie he za zhi, 25 (2005), 449–451. |
[44] |
F. Femiano, F. Gombos, C. Scully, Recurrent herpes labialis: a pilot study of the efficacy of zinc therapy, J. Oral Pathol. Med., 34 (2005), 423–425. https://doi.org/10.1111/j.1600-0714.2005.00327.x doi: 10.1111/j.1600-0714.2005.00327.x
![]() |
[45] |
M. Singh, R. R. Das, Zinc for the common cold, Cochrane Database Syst. Rev., 6 (2013), CD001364. https://doi.org/10.1002/14651858.CD001364.pub4 doi: 10.1002/14651858.CD001364.pub4
![]() |
[46] |
M. Lazzerini, H. Wanzira, Oral zinc for treating diarrhoea in children, Cochrane Database Syst. Rev., 12 (2016), CD005436. https://doi.org/10.1002/14651858.CD005436.pub5 doi: 10.1002/14651858.CD005436.pub5
![]() |
[47] |
F. Sakai, S. Yoshida, S. Endo, H. Tomita, Double-blind, placebo-controlled trial of zinc picolinate for taste disorders, Acta oto-laryngol., 122 (2002), 129–133. https://doi.org/10.1080/00016480260046517 doi: 10.1080/00016480260046517
![]() |
[48] | A. R. Watson, A. Stuart, F. E. Wells, I. B. Houston, G. M. Addison, Zinc supplementation and its effect on taste acuity in children with chronic renal failure, Hum. Nutr. Clin. Nutr., 37 (1983), 219–225. |
[49] |
J. Cervantes, A. E. Eber, M. Perper, V. M. Nascimento, K. Nouri, J. E. Keri, The role of zinc in the treatment of acne: A review of the literature, Dermatol. Ther., 31 (2018), e12576. https://doi.org/10.1111/dth.12576 doi: 10.1111/dth.12576
![]() |
[50] | A. Y. Bedikian, M. Valdivieso, L. K. Heilbrun, R. H. Withers, G. P. Bodey, E. J. Freireich, Glycerol: an alternative to dexamethasone for patients receiving brain irradiation for metastatic disease, South. Med. J., 73 (1980), 1210–1214. |
[51] |
M. S. Frank, M. C. Nahata, M. D. Hilty, Glycerol: a review of its pharmacology, pharmacokinetics, adverse reactions, and clinical use, Pharmacotherapy, 1 (1981), 147–160. https://doi.org/10.1002/j.1875-9114.1981.tb03562.x doi: 10.1002/j.1875-9114.1981.tb03562.x
![]() |
[52] |
J. Wang, Y. Ren, S. F. Wang, L. D. Kan, L. J. Zhou, H. M. Fang, et al., Comparative efficacy and safety of glycerol versus mannitol in patients with cerebral oedema and elevated intracranial pressure: A systematic review and meta-analysis, J. Clin. Pharm. Ther., 46 (2021), 504–514. https://doi.org/10.1111/jcpt.13314 doi: 10.1111/jcpt.13314
![]() |
[53] |
J. Wang, Y. Ren, L. J. Zhou, L. D. Kan, H. Fan, H. M. Fang, Glycerol Infusion Versus Mannitol for Cerebral Edema: A Systematic Review and Meta-analysis, Clin. Ther., 43 (2021), 637–649. https://doi.org/10.1016/j.clinthera.2021.01.010 doi: 10.1016/j.clinthera.2021.01.010
![]() |
[54] |
E. Righetti, M. G. Celani, T. A. Cantisani, R. Sterzi, G. Boysen, S. Ricci, Glycerol for acute stroke, Cochrane Database Syst. Rev., 2 (2004), CD000096. https://doi.org/10.1002/14651858.CD000096.pub2 doi: 10.1002/14651858.CD000096.pub2
![]() |
[55] |
A. Frei, C. Cottier, P. Wunderlich, E. Lüdin, Glycerol and dextran combined in the therapy of acute stroke. A placebo-controlled, double-blind trial with a planned interim analysis, Stroke, 18 (1987), 373–379. https://doi.org/10.1161/01.STR.18.2.373 doi: 10.1161/01.STR.18.2.373
![]() |
[56] |
E. Lin, Glycerol utilization and its regulation in mammals, Annu. Rev. Biochem., 46 (1977), 765–795. https://doi.org/10.1146/annurev.bi.46.070177.004001 doi: 10.1146/annurev.bi.46.070177.004001
![]() |
[57] |
Y. Yu, C. Kumana, I. Lauder, Y. Cheung, F. Chan, M. Kou, et al., Treatment of acute cortical infarct with intravenous glycerol. A double-blind, placebo-controlled randomized trial, Stroke, 24 (1993), 1119–1124. https://doi.org/10.1161/01.STR.24.8.1119 doi: 10.1161/01.STR.24.8.1119
![]() |
[58] |
B. á Rogvi-Hansen, G. Boysen, Intravenous Glycerol Treatment of Acute Stroke – A Statistical Review, Cerebrovasc. Dis., 2 (1992), 11–13. https://doi.org/10.1159/000108981 doi: 10.1159/000108981
![]() |
[59] |
H. L. Philpott, S. Nandurkar, J. Lubel, P. R. Gibson, Drug-induced gastrointestinal disorders, Frontline Gastroente., 5 (2014), 49–57. http://dx.doi.org/10.1136/flgastro-2013-100316 doi: 10.1136/flgastro-2013-100316
![]() |
[60] | S. Saleem, How to induce arrhythmias with dopamine, in Arrhythmia Induction in the EP Lab, Springer, (2019), 81–89. https://doi.org/10.1007/978-3-319-92729-9_9 |
[61] |
R. Ceravolo, C. Rossi, E. Del Prete, U. Bonuccelli, A review of adverse events linked to dopamine agonists in the treatment of Parkinson's disease, Expert Opin. Drug Saf., 15 (2016), 181–198. https://doi.org/10.1517/14740338.2016.1130128 doi: 10.1517/14740338.2016.1130128
![]() |
![]() |
![]() |
Notation | Meaning | Unit |
κ | Planted rate of susceptible | NSites day-1 |
Rx | Rate of primary infection | day-1 |
Rc | Rate of secondary infection | day-1 |
μ | Natural death rate | day-1 |
1/i | Death rate caused by infection | day-1 |
σ | Growth rate of pathogen population | day-1 |
ε | Death rate of pathogen | day-1 |
π | The effectiveness of botanical fungicides | day-1 |
δ | Rate of damage to the fungicide given to the plant | day-1 |
Notation | Biological meaning | Value |
Rx | Rate of primary infection | 0.1890 |
Rc | Rate of secondary infection | 0.8110 |
μ | Natural death rate | -0.1362 |
i | Death rate of infect | 0.8638 |
σ | Growth rate of the pathogen population | 0.1890 |
ε | Death rate of pathogen | - 0.1890 |
Notation | Biological meaning | Valu R0 < 1 | Value R0 > 1 | Unit | References |
κ | Planted rate of susceptible | 10 | 10 | NSites day-1 | [2] |
Rx | Rate of primary infection | 0.05 | 0.4 | day-1 | [2] |
Rc | Rate of secondary infection | 0.05 | 0.1 | day-1 | [2] |
μ | Natural death rate | 0.0083 | 0.0083 | day-1 | [2] |
1/i | Death rate of infect | 1/19 | 1/19 | day-1 | [6] |
σ | Growth rate of the pathogen | 0.0072 | 0.0072 | day-1 | [3] |
ε | Death rate of pathogen | 0.1236 | 0.1236 | day-1 | [4] |
π | The effective of botanical fungicides | 0.1 | 0.1 | day-1 | [8] |
δ | The rate of damage to the botanical fungicide given to the plant host | 0.001 | 0.001 | day-1 | [8] |
Notation | Meaning | Unit |
κ | Planted rate of susceptible | NSites day-1 |
Rx | Rate of primary infection | day-1 |
Rc | Rate of secondary infection | day-1 |
μ | Natural death rate | day-1 |
1/i | Death rate caused by infection | day-1 |
σ | Growth rate of pathogen population | day-1 |
ε | Death rate of pathogen | day-1 |
π | The effectiveness of botanical fungicides | day-1 |
δ | Rate of damage to the fungicide given to the plant | day-1 |
Notation | Biological meaning | Value |
Rx | Rate of primary infection | 0.1890 |
Rc | Rate of secondary infection | 0.8110 |
μ | Natural death rate | -0.1362 |
i | Death rate of infect | 0.8638 |
σ | Growth rate of the pathogen population | 0.1890 |
ε | Death rate of pathogen | - 0.1890 |
Notation | Biological meaning | Valu R0 < 1 | Value R0 > 1 | Unit | References |
κ | Planted rate of susceptible | 10 | 10 | NSites day-1 | [2] |
Rx | Rate of primary infection | 0.05 | 0.4 | day-1 | [2] |
Rc | Rate of secondary infection | 0.05 | 0.1 | day-1 | [2] |
μ | Natural death rate | 0.0083 | 0.0083 | day-1 | [2] |
1/i | Death rate of infect | 1/19 | 1/19 | day-1 | [6] |
σ | Growth rate of the pathogen | 0.0072 | 0.0072 | day-1 | [3] |
ε | Death rate of pathogen | 0.1236 | 0.1236 | day-1 | [4] |
π | The effective of botanical fungicides | 0.1 | 0.1 | day-1 | [8] |
δ | The rate of damage to the botanical fungicide given to the plant host | 0.001 | 0.001 | day-1 | [8] |