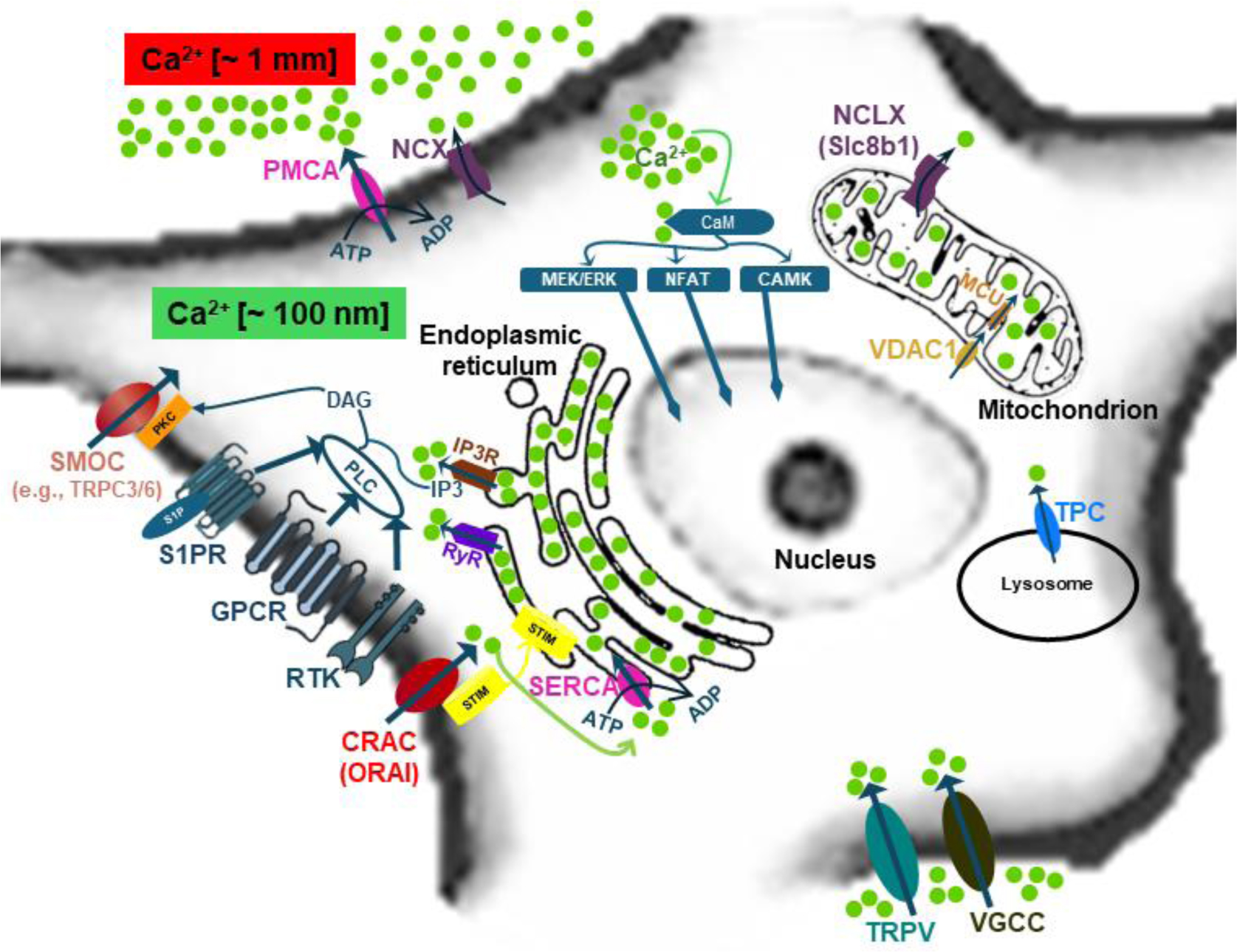
African swine fever (ASF) is an acute, hemorrhagic and severe infectious disease caused by the African swine fever virus (ASFV), and leads to a serious threat to the pig industry in China. Yet the impact of the virus in the environment and contaminated swill on the ASFV transmission is unclear in China. Then we build the ASFV transmission model with the virus in the environment and swill. We compute the basic reproduction number, and prove that the disease-free equilibrium is globally asymptotically stable when R0<1 and the unique endemic equilibrium is globally asymptotically stable when R0>1. Using the public information, parameter values are evaluated. PRCCs and eFAST sensitivity analysis reveal that the release rate of ASFV from asymptomatic and symptomatic infectious pigs and the proportion of pig products from infectious pigs to swill have a significant impact on the ASFV transmission. Our findings suggest that the virus in the environment and contaminated swill contribute to the ASFV transmission. Our results may help animal health to prevent and control the ASFV transmission.
Citation: Haitao Song, Lirong Guo, Zhen Jin, Shengqiang Liu. Modelling and stability analysis of ASFV with swill and the virus in the environment[J]. Mathematical Biosciences and Engineering, 2022, 19(12): 13028-13049. doi: 10.3934/mbe.2022608
[1] | Rongsong Liu, Jiangping Shuai, Jianhong Wu, Huaiping Zhu . Modeling spatial spread of west nile virus and impact of directional dispersal of birds. Mathematical Biosciences and Engineering, 2006, 3(1): 145-160. doi: 10.3934/mbe.2006.3.145 |
[2] | Louis D. Bergsman, James M. Hyman, Carrie A. Manore . A mathematical model for the spread of west nile virus in migratory and resident birds. Mathematical Biosciences and Engineering, 2016, 13(2): 401-424. doi: 10.3934/mbe.2015009 |
[3] | Danfeng Pang, Yanni Xiao . The SIS model with diffusion of virus in the environment. Mathematical Biosciences and Engineering, 2019, 16(4): 2852-2874. doi: 10.3934/mbe.2019141 |
[4] | Azmy S. Ackleh, Keng Deng, Yixiang Wu . Competitive exclusion and coexistence in a two-strain pathogen model with diffusion. Mathematical Biosciences and Engineering, 2016, 13(1): 1-18. doi: 10.3934/mbe.2016.13.1 |
[5] | Zongwei Ma, Hongying Shu . Viral infection dynamics in a spatial heterogeneous environment with cell-free and cell-to-cell transmissions. Mathematical Biosciences and Engineering, 2020, 17(3): 2569-2591. doi: 10.3934/mbe.2020141 |
[6] | Jinyu Wei, Bin Liu . Global dynamics of a Lotka-Volterra competition-diffusion-advection system for small diffusion rates in heterogenous environment. Mathematical Biosciences and Engineering, 2021, 18(1): 564-582. doi: 10.3934/mbe.2021031 |
[7] | Baoxiang Zhang, Yongli Cai, Bingxian Wang, Weiming Wang . Dynamics and asymptotic profiles of steady states of an SIRS epidemic model in spatially heterogenous environment. Mathematical Biosciences and Engineering, 2020, 17(1): 893-909. doi: 10.3934/mbe.2020047 |
[8] | Pengfei Liu, Yantao Luo, Zhidong Teng . Role of media coverage in a SVEIR-I epidemic model with nonlinear incidence and spatial heterogeneous environment. Mathematical Biosciences and Engineering, 2023, 20(9): 15641-15671. doi: 10.3934/mbe.2023698 |
[9] | Xiaowei An, Xianfa Song . A spatial SIS model in heterogeneous environments with vary advective rate. Mathematical Biosciences and Engineering, 2021, 18(5): 5449-5477. doi: 10.3934/mbe.2021276 |
[10] | Junli Liu . Threshold dynamics of a time-delayed hantavirus infection model in periodic environments. Mathematical Biosciences and Engineering, 2019, 16(5): 4758-4776. doi: 10.3934/mbe.2019239 |
African swine fever (ASF) is an acute, hemorrhagic and severe infectious disease caused by the African swine fever virus (ASFV), and leads to a serious threat to the pig industry in China. Yet the impact of the virus in the environment and contaminated swill on the ASFV transmission is unclear in China. Then we build the ASFV transmission model with the virus in the environment and swill. We compute the basic reproduction number, and prove that the disease-free equilibrium is globally asymptotically stable when R0<1 and the unique endemic equilibrium is globally asymptotically stable when R0>1. Using the public information, parameter values are evaluated. PRCCs and eFAST sensitivity analysis reveal that the release rate of ASFV from asymptomatic and symptomatic infectious pigs and the proportion of pig products from infectious pigs to swill have a significant impact on the ASFV transmission. Our findings suggest that the virus in the environment and contaminated swill contribute to the ASFV transmission. Our results may help animal health to prevent and control the ASFV transmission.
The development of the nervous system unfolds through a meticulously orchestrated sequence of events. It begins with the proliferation of neural stem/progenitor cells (NSCs), which then migrate considerable distances from germinal centers to their final destinations. Following migration, these cells undergo differentiation into billions of neurons and glia, ultimately populating the brain [1],[2]. During this intricate process, rhythmic bursts of calcium (Ca2+) signals within developing cells play a pivotal role, guiding specific cellular responses at each stage [3]. These cellular calcium signals are crucial in regulating various aspects of neural development, such as neural induction and proliferation [4],[5].
Stem cells typically reside within specialized niches that influence their behavior, making it crucial to investigate how extracellular calcium is managed in these microenvironments. The modulation of calcium levels in local tissues likely involves distinct mechanisms, yet this aspect remains relatively unexplored due to its intricate technical nature [6]. Therefore, calcium physiology in stem cells is influenced by specific regulation and signaling mediated by intracellular calcium, as well as by the availability of calcium within the stem cell niche [7].
In pathological contexts, NSCs play a critical role in brain repair following injuries. Moreover, oncogenic mutations in NSCs within the ventricular-subventricular zone (V-SVZ) region are consistently linked to the development of glioblastoma (GBM) [8]–[11]. GBM tissues contain a subset of cells known as glioma stem cells (GSCs), which share several characteristics with NSCs and are believed to originate from them [12]. GSCs, known for their rapid proliferation and resistance to current therapies, are believed to be responsible for tumor initiation, growth, and recurrence [13].
The activation of any calcium channel in the cell membrane results in a substantial influx of calcium into the cell, leading to a rapid increase in intracellular calcium levels. This surge in calcium concentration acts as a critical signal that modulates the activity of nearby proteins, initiating a diverse array of biological responses [14].
Calcium ion Ca2+ serves as a highly impactful signaling molecule on cellular function, underscoring its functions as a versatile and widespread second messenger that regulates a diverse range of cellular processes [15]. Within both mature and developing brain tissue, numerous calcium signaling proteins generate diverse calcium signals that orchestrate various cellular processes [16],[17]. Calcium signaling is crucial in multiple aspects of nervous system development [18],[19].
The regulation of cytoplasmic calcium governs a wide range of cellular activities, including neurotransmitter release, intracellular signaling, transcription, excitation–contraction coupling in muscle cells, cell motility and morphology, metabolism, and hormone release [20]. Moreover, the calcium signaling pathway, mediated by specific components of the calcium signaling toolkit, plays a vital role in neurogenesis, influencing neural induction, proliferation, migration, and differentiation of neural cells [18],[21],[22].
As calcium diffuses away from its source, its concentration diminishes sharply with distance due to cytoplasmic buffers and membrane pumps [23]. Consequently, the arrangement of calcium signaling complexes and downstream effectors in close proximity to calcium channels facilitates rapid and precise activation of calcium-dependent responses [24],[25]. Therefore, for many calcium-mediated functions, the physiological outcome depends on the spatial relationship between calcium channels and their calcium-sensitive effectors.
The diversity of cellular functions influenced by cellular calcium signals prompts inquiry into how specificity is conveyed by this versatile messenger. The spatial arrangement of the calcium signal provides targeted instructions to modulate downstream responses [3],[25]. Calcium microdomains, localized calcium signals, swiftly emerge near open calcium channels, establishing spatial calcium gradients characterized by high [Ca2+] levels adjacent to the channel pore, sometimes reaching tens of micromolar [26].
Cytosolic Ca²⁺ levels are altered in response to external stimuli, a process modulated through both extracellular calcium entry and the release of calcium from internal stores [27]. This complex regulation underscores the importance of understanding intracellular calcium oscillation (ICO), a key mechanism in cellular signaling. The dynamics of ICO have been extensively studied from various perspectives, uncovering diverse phenomena such as stochastic resonance, calcium puffs, and coherence resonance, all of which highlight the critical role of calcium-induced calcium release channels [28].
Research on ICO has significantly focused on stochastic effects, spatial structures, and the activation of proteins involved in calcium signaling. Recent studies have investigated the role of non-Gaussian noise within ICO systems, emphasizing its impact on the stability of calcium signaling and underscoring the need to examine the statistical properties of Ca²⁺ concentration velocity [29]. Furthermore, the inclusion of time delays in both active and passive calcium transport processes has been shown to be crucial for accurately modeling ICO dynamics, particularly in systems influenced by additive Gaussian-colored noise. These time delays provide a more comprehensive understanding of the transient dynamics of ICO [28].
Additionally, recent investigations have explored the effects of non-Gaussian noises on ICO, specifically their capacity to induce synchronous or anti-synchronous oscillations. These oscillations can have significant implications for calcium concentration and signaling, further emphasizing the complex interplay between noise and intracellular calcium dynamics [30].
The manuscript will explore diverse calcium signaling pathways, examining their functions and impact on general cellular regulation. It will particularly highlight the dynamics of these pathways in relation to the proliferation of NSCs and the progression of GBM. This analysis will involve discussing the range of regulators and signals influencing the proliferation of these cells, including conditions under which the effects of different calcium modulations have been studied in NSCs or GBM. Furthermore, the manuscript will explore potential avenues for future research and promising targets worthy of investigation for future therapeutic interventions.
The Ca2+ ion plays a pivotal role as a secondary messenger in cellular signaling pathways, orchestrating fundamental processes such as cell growth, migration, and programmed cell death (PCD) [16]. A notable characteristic of cellular dormancy is the exceptionally low cytosolic concentration of calcium, typically less than 100 nmol/L, which contrasts sharply with the much higher levels found extracellularly, spanning a difference of several thousandfold. This marked discrepancy underscores the importance of calcium dynamics in cellular regulation.
The influx of extracellular calcium is mediated by a diverse array of transmembrane proteins, predominantly calcium channels, which act as conduits for calcium entry in response to a range of stimuli. These stimuli encompass membrane depolarization, extracellular signaling molecules, intracellular messengers, mechanical tension, or the depletion of intracellular calcium stores [16].
Notably, different types of calcium channels operate through specialized mechanisms that are finely tuned to respond to specific cellular signals and environmental cues. A comprehensive understanding of these intricate mechanisms is imperative for elucidating the multifaceted role of calcium in cellular physiology and pathology, a topic that will be explored in detail in this article.
In the cytoplasm, calcium levels are typically low and maintained in a stable state, but they can increase substantially within organelles such as the endoplasmic reticulum (ER), lysosomes, and mitochondria, where concentrations may escalate to much higher levels [31]. The expulsion of calcium from the cytoplasm happens against a steep gradient using ATP-dependent mechanisms, which involve plasma membrane Ca2+ ATPases (PMCAs) efflux pumps and, particularly in excitable cells, the Na+/Ca2+ exchanger (NCX) (Figure 1) [32],[33]. Moreover, calcium ions are transported within cells through a series of orchestrated mechanisms. First, ATP-dependent sarcoendoplasmic reticulum calcium ATPase (SERCA) pumps actively move calcium ions into the ER. Concurrently, voltage-dependent anion-selective channel 1 (VDAC1), located in the outer mitochondrial membrane, enables the influx of calcium into the intermembrane space (Figure 1). Subsequently, within the inner mitochondrial membrane, calcium is transferred via the mitochondrial calcium uniporter (MCU) and its associated regulatory proteins, driven by the gradient of the mitochondrial membrane potential [34]. Moreover, calcium can be released from mitochondria through the NCLX (Slc8b1) Na+/Ca2+ exchanger (Kostic & Sekler, 2019). Furthermore, within lysosomes and other acidic organelles, there are two-pore channels (TPC) that release calcium in response to the second messenger nicotinic acid adenine dinucleotide phosphate (NAADP) [35].
In animal cells, store-operated calcium entry (SOCE) is a critical pathway for the entry of calcium ions. This process is mediated by store-operated channels (SOCs), which are activated upon depletion of calcium stores within the ER. SOCs serve a dual function: they refill ER calcium levels and initiate sustained calcium signals that are essential for various cellular functions, including gene expression, secretion, and motility [36].
The activation of store-operated calcium (SOC) channels begins with the stimulation of G protein-coupled receptors (GPCRs) or receptor tyrosine kinases (RTKs), which trigger the phospholipase C (PLC)-IP3 signaling pathway. PLC hydrolyzes phosphatidylinositol-4,5-bisphosphate (PIP2) into diacylglycerol (DAG) and inositol 1,4,5-trisphosphate (IP3) [37]. DAG activates protein kinase C (PKC), leading to the opening of receptor-operated channels (ROCs), such as transient receptor potential canonical 3/6 (TRPC3/6) [37]. These TRPC channels, also known as secondary messenger-operated channels (SMOCs), facilitate the influx of positively charged ions, including calcium (Figure 1) [38],[39].
Moreover, the regulation of intracellular calcium ions profoundly impacts cellular dynamics, primarily managed by the ER as the principal calcium reservoir. Calcium is released into the cytoplasm via inositol 1,4,5-trisphosphate receptors (IP3Rs) and ryanodine receptors (RYRs) triggered by factors like free calcium ions [40].
SOCE is initiated by IP3 signaling, which triggers the release of calcium into the cytoplasm. The resulting decrease in ER calcium levels activates the ER calcium sensors STIM1 and STIM2. These sensors then interact with plasma membrane calcium channels, including Orai1, Orai2, and Orai3, collectively referred to as calcium release-activated channels (CRAC) [36],[41]. The activation of CRAC channels permits the influx of calcium from the extracellular space, thereby amplifying the calcium signal transiently and replenishing ER calcium stores (Figure 1) [42]. Additionally, the IP3 signaling pathway generates transient calcium releases that can lead to oscillatory calcium signals over time [43].
The reduction in ER calcium levels prompts STIM1 to undergo oligomerization and conformational changes, leading to the opening of SOC channels. This allows calcium to enter the cytosol from the extracellular space. Through the action of the SERCA, calcium is then transported back into the ER, replenishing its calcium stores and sustaining calcium influx (Figure 1) [37]. This process activates calcium-dependent signaling molecules that are crucial for various cellular functions.
STIM1 and Orai1 have been identified in neurospheres derived from both embryonic and adult mice, with reduced expression of these proteins being associated with decreased cell proliferation [5]. Furthermore, SOCE is crucial for maintaining ER calcium balance and regulating various cellular functions. CRAC channels are involved in short-term processes such as calcium oscillations and secretion, as well as long-term functions including gene expression pathways [44].
Ryanodine receptors (RyRs) serve as calcium-gated channels in the ER in neurons, skeletal muscle, heart muscle, and smooth muscle cells [45]. In the brain, three distinct isoforms of ryanodine receptors (RYRs)—RYR1, RYR2, and RYR3—encoded by different genes, exhibit unique expression patterns [40],[46]. The regulation of both IP3Rs and RyRs by calcium involves displaying either positive feedback (calcium-induced calcium release) or negative feedback depending on local calcium concentrations [47].
A signaling lipid known as sphingosine 1-phosphate (S1P) modulates cellular functions by activating G protein-coupled receptors (GPCRs) located on the plasma membrane (PM). These GPCRs, designated as S1PR1–5, mediate calcium signaling [48]. Extensive studies have revealed that S1P plays a multifaceted role in regulating cellular calcium signals, which are critical for numerous cellular processes, including metabolism and cell growth.
When S1P binds to its receptors, it initiates a classical downstream signaling pathway involving the activation of PLC. This activation of PLC catalyzes the hydrolysis of PIP2 into two secondary messengers, IP3 and DAG [49],[50]. IP3 then diffuses through the cytoplasm to bind its receptors on the ER, which leads to the release of calcium from IP3-sensitive intracellular stores into the cytosol.
In addition to IP3-mediated calcium release, DAG plays a crucial role in calcium signaling by remaining within the PM where it binds to and activates calcium-permeable ion channels (Figure 1). This binding facilitates ROCE, allowing extracellular calcium to enter the cell [16]. The dual mechanisms of calcium mobilization and entry mediated by S1P signaling ensure a sustained and regulated increase in intracellular calcium levels. This precise regulation is essential for maintaining various physiological processes.
The rhythmic release of calcium ions generates oscillatory calcium signals essential for genetic programs during neural development, including proliferation, migration, and differentiation [22],[51]. The entry and release of calcium through various channels increase cytosolic calcium concentration, activating specific signaling pathways with distinct spatial and temporal characteristics [14]. Generally, the influx of calcium into the cytoplasm from the extracellular space is triggered either by voltage-gated channels, receptor-operated ionotropic channels, or store-operated calcium entry (SOCE) (Figure 1). Calcium sensors detect local signals and relay them to targets beyond their immediate vicinity [52]. During neural growth, calcium signals are mediated by voltage-gated calcium channels (VGCCs), such as L-type calcium channels, and store-operated CRAC channels [53]. These signals involve calcium-binding proteins (CaBPs) such as calmodulin, calcineurin, PKC, S100 proteins, and parvalbumin. CaBPs, including parvalbumin and S100, act to buffer free calcium, while others initiate specific intracellular signaling cascades in response to calcium signals [54],[55]. Approximately 200 calcium-binding proteins have been identified, playing various roles, including buffering calcium to decrease the concentration of free calcium [56].
The initiation of a calcium signal through a calcium channel triggers calcium sensors such as calmodulin, which in turn activate distant signaling pathways (e.g., MAP kinase or calcineurin/NFAT), ultimately leading to genetic activity within the nucleus [57]. Understanding the underlying mechanisms of these diverse localized calcium-driven cellular reactions is crucial for nervous system development and function. For example, calmodulin undergoes changes in its interaction with various effectors upon binding calcium, thereby activating distinct signaling pathways such as MEK/ERK, NFAT, or CAMK (Figure 1) [58].
Moreover, modulation of calcium oscillations influences cellular functions like gene transcription by regulating the activity of calcium-binding proteins (CaBPs). For instance, NFκB, a transcription factor involved in both neuronal survival and apoptosis, exhibits preferential activation in response to low-frequency calcium transients. In contrast, the activation of the nuclear factor of activated T-cells (NFAT) necessitates higher frequencies and sustained calcium signals [59],[60].
A notable example of indirect pathway regulation involves the NFAT family of transcription factors, where calcium ion mobilization plays a central role. Elevated calcium levels stimulate calcineurin, leading to NFAT dephosphorylation and its translocation to the nucleus to regulate gene expression [58]. NFAT-mediated gene transcription is essential for regulating proteins critical to neuronal activity responses [61],[62]. Furthermore, the activity of NFAT is subject to modulation by various sources of calcium entry. For instance, calcium influx through NMDA receptors has been shown to regulate cortical neuronal survival via NFAT signaling pathways [63].
CRAC channels drive NFAT-dependent gene expression during neural stem/progenitor cell development. Calcium signaling via CRAC channels activates NFAT for gene transcription [5]. The physical association between calcineurin and CRAC channels is likely facilitated by A-kinase anchoring protein 79 (AKAP79), directing calcineurin to calcium channels and enhancing NFAT signaling [64],[65]. This relationship is further elaborated in the following section.
The rhythmic fluctuations of calcium ions are proposed to result from a synchronized interplay between calcium influx and release from internal calcium stores [66]. These periodic changes diminish in the absence of calcium entry, highlighting the critical role of calcium influx in sustaining such calcium signals [67]. Consequently, the key elements of the calcium signaling toolkit comprise G-protein-coupled receptors (GPCRs) and receptor tyrosine kinases (RTKs), which detect external signals, along with various calcium-permeable channels that either enable calcium entry across the cell membrane or release calcium from internal stores [19],[68]. Upon the elevation of intracellular calcium levels, downstream processes are initiated involving various calcium-binding effector proteins such as calmodulin, as previously described. Additionally, calcium-sensitive transcription factors, enzymes, and ion channels play critical roles in executing these effector functions [19],[69]. Subsequently, calcium pumps and exchangers restore calcium stores or remove calcium from the cell, thereby regulating the resting intracellular calcium concentration (Figure 1) [70],[71]. The resulting temporal and spatial characteristics of the calcium signal, controlled by these components, encode specific messages that determine the types of cellular programs activated [19].
Voltage-gated calcium channels and neurotransmitter-activated receptors such as GABA and glutamate receptors are among the molecules implicated in facilitating the crucial calcium influx necessary for maintaining these fluctuations. During early brain development, when synaptic connections are not yet established, glutamate and GABA act via NMDA and GABAA receptors in a paracrine, nonsynaptic mode of communication between cells, resulting in increased intracellular calcium levels [72],[73].
Voltage-gated calcium channels (VGCCs) are essential for calcium ion influx following membrane depolarization (Figure 1). These channels activate in response to an increase in membrane potential, leading to the opening of the channel pore [74]. As key transducers of membrane potential changes, VGCCs convert these changes into intracellular calcium transients, initiating numerous physiological events. In mammals, there are ten members of the VGCC family, each fulfilling distinct roles in cellular signal transduction [74].
Notably, VGCCs are also present in non-excitable cells, including various cancer cells [75]. The development of new animal and cellular models, along with the emergence of large data sets and unbiased genome screens, has enhanced our understanding of the unexpected roles VGCCs play in these non-excitable cells [76]. Further details regarding the presence of these channels in NSCs and GBM are provided in the following sections.
Another calcium channel with known voltage-gated properties is the transient receptor potential vanilloid (TRPV) channel, part of the TRP family of channels (Figure 1) [77]. TRPVs are calcium-permeable channels that respond to chemical ligands, heat, and mechanical stretching. Different TRPV channels play distinct roles in physiological processes, such as sensing mechanical and osmotic changes. TRPV1-TRPV4 channels respond to heat and act as chemosensors, while TRPV5 and TRPV6 are highly selective calcium channels. TRPV2 is a cation channel regulated by growth factors that may play a role in oncogenesis [78].
Ligand-gated ion channels (LGICs) are activated, or "gated", upon binding with neurotransmitters. This activation induces a structural alteration that results in the opening of the channel, allowing ion flow. LGICs include both inhibitory, anion-selective receptors such as GABAA and glycine receptors, and excitatory, cation-selective receptors like nicotinic acetylcholine (nAchR), serotonin 5-HT3, and ionotropic glutamate receptors [79].
LGICs significantly impact cellular calcium regulation. For instance, ligand-gated GABA receptors induce depolarizing chloride currents, which can subsequently activate voltage-gated calcium channels. In contrast, ligand-gated glutamate receptors facilitate the influx of sodium and calcium ions [79]. Furthermore, ligand-gated transient receptor potential (TRP) channels [21], metabotropic transmitter receptors [80], and mechanoreceptors [81] contribute to spontaneous calcium fluctuations. These fluctuations play a crucial role in directing neural development and synaptic plasticity.
NSCs possess a sophisticated ability to respond to a wide range of external signals, with each signal exerting specific effects on NSC behavior and regulation. This intricate regulatory network was highlighted by detailed transcriptomic analyses of purified adult NSCs, which revealed the fundamental importance of calcium-dependent signaling pathways in these cells [82]. Moreover, studies employing advanced calcium imaging techniques have unveiled the existence of intercellular calcium waves in NSCs, propagating through gap junctions. These waves enable intricate communication not only among NSCs themselves but also with neighboring niche astrocytes, functioning in both normal physiological conditions and pathological states [83],[84]. Additionally, live cell calcium imaging has provided insights into spontaneous calcium oscillations observed in NSC/progenitors within the V-SVZ [85].
Beyond facilitating cellular communication, calcium signaling plays a pivotal role in orchestrating essential stages of the cell cycle, including phase transitions, immediate early gene transcription, and the regulation of quiescence and cell division [86]. Within the proliferative niche of the brain, three distinct cell types are identifiable: progenitor cells, which share similarities with stem cells, transient amplifying cells (TAPs), and migrating neuroblasts. Progenitor cells undergo slow division, giving rise to rapidly proliferating TAPs through asymmetric division. Subsequently, TAPs differentiate into neuroblasts characterized by reduced proliferation and a migratory phenotype [87]. This dynamic cellular hierarchy underscores the intricate interplay of calcium signaling in coordinating neurogenic processes within the brain, influencing fundamental aspects of cellular behavior and fate determination in neural development and regeneration.
Studies have highlighted the crucial role of calcium signaling pathways in regulating fundamental processes such as self-renewal and differentiation in stem cells [7]. Specifically, calcium ions have emerged as pivotal factors in preserving pluripotency, which is the capability to differentiate into various cell types of embryonic stem cells [88]. This highlights the significance of calcium in orchestrating the delicate balance between maintaining stemness and allowing cells to specialize in different lineages.
In the context of cell cycle dynamics, calcium influx assumes a pivotal role in stirring stem cells from a dormant state into an active phase [89],[90]. This transition is critical for initiating cellular activities necessary for growth and development. The orchestration of these calcium-driven processes underscores the intricate regulatory mechanisms guiding stem cell fate decisions.
Numerous studies have emphasized the vital role of maintaining NSCs and their functionality through precise regulation of intracellular calcium levels, particularly within the ER. For instance, the propagation of calcium waves essential for NSC self-renewal post-injury relies on IP3 signaling [84]. Conversely, inducing calcium release from the ER via the pro-apoptotic protein Bax leads to increased cell death, which can be significantly mitigated by siRNA-mediated inhibition of IP3R expression [91]. These findings underscore the pivotal role of maintaining precise calcium homeostasis in sustaining the NSC population.
In non-excitable cells such as NSCs and GBM, IP3Rs are widely distributed and activated by IP3 generated through plasma membrane receptors, including GPCRs and RTKs, which activate PLC (Figure 2) [43],[92]. Various extracellular signals initiate calcium responses by inducing calcium release from intracellular stores and calcium influx from the extracellular environment, with SOCE being the primary mode of calcium entry in stem cells [37]. SOCE involves the opening of calcium channels in response to the depletion of intracellular calcium stores, a mechanism finely tuned to meet the dynamic calcium demands during various stages of stem cell activity and differentiation.
SOCE mediated by STIM1 and Orai1 consistently occurs in NSCs across various developmental stages, from embryonic to adult NSCs, indicating the conservation of this calcium entry mechanism throughout development and into adulthood. Disruption of this mechanism, such as through the introduction of a non-functional Orai1 mutant (R93W Orai1) or conditional knockouts of Orai1 in the brain, significantly impairs NSC proliferation both in vitro and in vivo (Figure 2) [5]. These effects are likely mediated through calcineurin/NFAT-regulated transcription of cell cycle proteins, as inhibiting calcineurin/NFAT signaling results in a similar impairment of proliferation observed with the loss of CRAC channel function, such as Orai1 (Figure 2) [5]. These discoveries establish CRAC channels as a novel mechanism for regulating calcium signaling, gene expression, and proliferation in NSCs. However, NSCs are derived from a non-excitable epithelial cell lineage, making them more akin to non-neuronal cell types in terms of calcium influx [93], thus suggesting that CRAC channels could function as a primary mechanism for calcium regulation in controlling NSC proliferation.
Calcium-associated proteins (CaAPs) play a crucial role in governing the functionality of neural progenitor cells (NPCs). Notably, NFAT, particularly its NFATc1 and NFATc3 variants, exhibit activity in cultured V-SVZ cells of neonatal rodents, responding specifically to localized calcium signals from SOC activation [94]. The NFAT inhibitor VIVIT, which selectively and potently inhibits the calcineurin/NFAT interaction, retards the cell cycle in NPCs and reduces their differentiation potential (Figure 2) [88]. This highlights NFAT's role in preserving a multipotent stem cell phenotype.
In the V-SVZ, SOCE, triggered by extracellular cues such as EGF or SDF1, plays a critical role in regulating NSC behavior toward GBM [5],[85],[95]. SOCs are primarily composed of Orai1, which mediates the calcium-release-activated calcium current (Icrac) and TRPC proteins. TRPC proteins predominantly serve as calcium-permeable non-selective cation channels, with TRPC1 being notably involved in interactions with Orai1. Furthermore, STIM1 is known to directly activate Orai1 and may potentially interact with TRPC1 [37],[96]. This calcium entry, mediated by Orai1 and transient receptor potential canonical 1 (TRPC1), is essential for NSC self-renewal and division (Figure 2) [5]. These intricate interactions highlight the complexity of calcium signaling networks and their crucial roles in the cellular physiology of non-excitable cells.
Notably, the activation of SOCs with glutamate or muscarine has been shown to promote NSC self-renewal [85],[97]. Conversely, inhibiting SOCE shifts NSC division from symmetric proliferative to asymmetric, highlighting the pivotal role of SOCs in maintaining or expanding the NSC population [85]. However, the exact mechanism through which SOCs influence cell division remains unclear, possibly involving the activation of specific calcium regulators via SOCE or the positioning of STIM and Orai proteins at the cleavage furrow [98].
Studies have illuminated the critical role played by SOCs in NSCs, underscoring the significance of SOCE in transmitting mechanical signals triggered by cerebrospinal fluid flow [99]. Specifically, the epithelial sodium channel (ENaC) located in the primary cilium of NSCs functions as a mechanosensor, necessitating subsequent SOCE to modulate cell proliferation within the V-SVZ in response to cerebrospinal fluid flow. These findings highlight the indispensable role of SOCs in integrating NSC behavior with extracellular cues, particularly in dynamic fluid environments within the brain.
Furthermore, investigations in neurons suggest that following store depletion, STIM1 exhibits inhibitory effects on the activity of voltage-gated calcium CaV 1.2 and CaV 3.1 VGCCs present in quiescent NSCs, potentially contributing to the maintenance of a quiescent state (Figure 2) [100]. This observation suggests a potential regulatory role of STIM1 in controlling NSC activity through modulation of calcium channel function, emphasizing the complexity of calcium signaling pathways in NSCs and their involvement in regulating fundamental aspects of NSC physiology, such as proliferation and quiescence.
A study of neural circuits has revealed a critical link between cortical input and cholinergic interneurons in the SVZ. This interaction directly influences the proliferative behavior of quiescent neural stem cells (qNSCs) within the SVZ [101]–[103]. Specifically, cortical input activates muscarinic 3 (M3) receptors on qNSCs, triggering the release of intracellular calcium from the ER [104]. This calcium release acts as a pivotal signaling mechanism that governs the activation and proliferation of NSCs within the SVZ, highlighting the complex regulatory pathways orchestrating neural stem cell dynamics in the adult brain.
Cells in the V-SVZ exhibit high responsiveness to extracellular nucleotides, which trigger calcium mobilization. This response is particularly pronounced following brain injury due to the release of nucleotides from damaged cells. The heightened sensitivity of V-SVZ cells to extracellular nucleotides indicates a critical role for these molecules beyond their involvement in pathology; they are essential in regulating the neurogenic niche [105].
Nucleoside triphosphate diphosphohydrolase-2 (NTDPase2) further underscores the fundamental role of nucleotides in cellular dynamics. Knockout studies of NTDPase2 reveal an increase in intermediate progenitors within the V-SVZ, emphasizing the importance of elevated nucleotide levels in promoting cellular proliferation within this microenvironment [106].
Extracellular nucleotides exert their influence in the V-SVZ through metabotropic G-protein-coupled purinergic P2Y receptors and ionotropic purinergic P2X receptors, which initiate calcium transients [107],[108]. Notably, the P2X7R subtype is present in ependymal (E) cells and TAPs, functioning as a scavenger receptor involved in phagocytosis in the absence of ATP and inhibiting neuroblast proliferation when activated by ATP [107],[109],[110]. This diversity of receptors underscores the intricate and nuanced cellular responses to extracellular nucleotides, reflecting complex regulatory mechanisms within the V-SVZ.
In neural development, the involvement of TRP channels in calcium signaling and neural progenitor cell behavior reveals intriguing complexities. These TRP channels serve a dual function—enabling calcium influx and governing the proliferation of neural progenitors [21]. Notably, while VGGCs initially show limited impact during early neural stem cell (NSC) stages, their significance becomes pronounced in later developmental phases [111],[112].
During these advanced stages, a pivotal shift occurs as progenitors become responsive to GABAergic signals, which at this juncture act as depolarizing stimuli—a departure from their typical inhibitory role in mature neurons. This shift correlates with an upsurge in the expression of voltage-gated calcium channels (Figure 2). The importance of this transition is underscored by studies showing that the absence of either excitatory GABAergic transmission or voltage-gated calcium channels results in diminished proliferation, emphasizing the critical role of this signaling cascade in regulating progenitor cell activity [111],[113].
Turning to the V-SVZ, TRPV1 channels are present in approximately 20% of NSCs, TAPs, and neuroblasts during the early postnatal period [114]. However, as neurogenesis diminishes in one-month-old mice, TRPV1 expression also declines [114],[115]. The intriguing possibility emerges that TRPV1 expression may be reinstated in adulthood under physiological conditions that foster neurogenesis [114].
Furthermore, the impact of TRPV1 becomes evident upon its deletion in mice, leading to a notable increase in proliferating cells but a decrease in the differentiation of these cells into neurons or glia within the neurogenic niches of the postnatal brain. This finding suggests a pivotal role for TRPV1 in modulating the delicate balance between proliferation and differentiation of neural precursors, providing valuable insights into the molecular mechanisms governing neural development and regeneration (Figure 2) [114].
Neurotransmitters play a critical role in modulating NSC behavior by activating calcium-coupled LGICs, which are key regulators of NSC function. Glutamate-responsive ionotropic receptors, including NMDA, AMPA, and kainate subtypes, are expressed by NSCs and neuroblasts (Figure 2) [116],[117]. Although the specific functions of glutamate receptors in NSCs are still under investigation, kainate and AMPA receptors are known to induce calcium influx in neuroblasts. This activation regulates migration speed along the lateral ventricles (LV) and promotes proliferation, particularly facilitating brain repair after a stroke [117],[118].
Signaling mediated by metabotropic glutamate receptors (mGluR) [119] and NMDA receptors (NMDARs) is implicated in the proliferation of late-stage progenitors expressing doublecortin (DCX) [120]. Notably, blocking NMDARs or inhibiting L-type calcium channels impedes the proliferation of hippocampal progenitors, underscoring the critical role of activity-dependent pathways in regulating NSC proliferation [120].
Astrocyte-released glutamate significantly impacts neuroblast migration, influencing the integration of adult-born neurons into neural circuits [121]. Additionally, NMDARs contribute to oligodendrocyte differentiation within the V-SVZ [122]. AMPA receptors are present in oligodendrocyte precursor cells (OPCs) during the repair of corpus callosum demyelination [123]. OPCs are crucial progenitors responsible for generating new oligodendrocytes and are equipped with various neurotransmitter receptors and calcium-permeable ion channels, enabling them to establish direct synaptic connections with neurons and act as postsynaptic targets [124]–[127].
Within these synaptic interactions, OPCs undergo transient activation of ionotropic glutamate or GABAA receptors in their processes. This activation impacts OPC proliferation, differentiation, and injury response, thereby linking neural activity to the regulation of OPC behavior [128]–[132].
Studies in larval zebrafish have revealed periodic increases in intracellular calcium levels among OPCs in the developing spinal cord, with variations depending on cell body location and heightened activity in less myelinated areas [133]. Additionally, activation of olfactory neurons by odorants triggers calcium surges within OPCs located in activated glomeruli in the olfactory bulb, independent of myelination [134]. These observations suggest distinct physiological states among OPCs and underscore regional differences in their responsiveness to neuronal activity. Activation of locus coeruleus (LC) neurons during state transitions induces similar increases in calcium activity in OPCs transitioning from quiescence to activity [135]. Behavioral interventions, such as intensive motor learning, can stimulate OPCs to differentiate and produce additional myelin—a phenomenon termed “adaptive myelination” [136]. This underscores the remarkable adaptability of OPCs in response to environmental stimuli and behavioral changes, contributing significantly to nervous system plasticity. The regulation of OPC behavior as progenitors for generating oligodendrocytes, particularly through intracellular calcium dynamics, exemplifies a sophisticated mechanism whereby OPCs integrate signals from the surrounding neural environment to modulate myelination and support neural function and adaptation under both normal physiological conditions and in disease states.
Studies have demonstrated the presence of VGCCs L-type CaV1.2 and T-type CaV3.1 channels in V-SVZ cell cultures, which consist of NSCs and their progeny (Figure 2) [137]. Furthermore, a thorough analysis of purified NSCs using flow cytometry has revealed that quiescent NSCs exhibit elevated expression levels of both L- and T-type VGCCs [116]. Electrophysiological investigations, coupled with pharmacological studies, have further characterized the functional activity of L- and T-type currents in NSCs, demonstrating their activation by GABA under physiological conditions [138].
The continuous synthesis of GABA by neuroblasts within the germinal niche activates GABAA receptors expressed on NSCs, maintaining these cells in a dormant state (Figure 2) [116],[139],[140]. The depolarization induced by GABA triggers the opening of CaV channels, leading to calcium influx, a process that can be effectively blocked by specific antagonists such as nifedipine for L-type channels or mibefradil for T-type channels [138].
The presence of acetylcholine (ACh) in the brain, even before synaptogenesis and neurotransmission, suggests its role in unconventional neurotransmitter signaling, critical for neuronal cell proliferation via cholinergic receptor pathways [141]. Neuronal progenitor cells express both muscarinic (mAChRs) and nicotinic (nAChRs) acetylcholine receptors [142],[143], and stimulating these receptors has been shown to promote proliferation and neurogenesis [144].
NSCs possess muscarinic receptors responsive to acetylcholine, leading to brief calcium signals through store-operated channels [5],[85], along with ionotropic nAChRs, including α3 and α4 subtypes [145]. Furthermore, α7 nAChRs are present in the V-SVZ, where neuroblasts and TAPs express these receptors (Figure 2) [146],[147]. Acetylcholine exposure in the V-SVZ originates from a subset of cholinergic neurons within the rodent V-SVZ [101],[102],[145]. Activation of local cholinergic neurons or acute nicotine administration significantly enhances in vivo neurogenesis [145],[148]. Specific pharmacological and genetic studies have demonstrated that α7 nAChRs promote neuronal differentiation but inhibit V-SVZ cell proliferation under normal conditions and in response to ischemia, while the β2-nAChR subunit regulates the survival of newborn neurons [146],[149].
Activation of AChRs induces calcium influx, which triggers mitogen-activated protein kinase (MAPK) and ERK signaling pathways that regulate proliferation and DNA synthesis (Figure 2) [150],[151]. Intracellular calcium levels can modulate the MAPK cascade through two mechanisms: calcium-dependent tyrosine kinase (PYK2) or calmodulin, both converging on the Ras pathway to activate MAPK [152].
Therefore, the presence and action of acetylcholine in the brain, particularly through its interaction with cholinergic receptors and intracellular calcium influx, serve as critical modulators of neuronal proliferation and differentiation. This highlights its significance in early brain development and neurogenesis, specifically through the dynamic regulation of calcium signaling pathways.
These findings highlight the intricate control of NSC activity through neurotransmitter signaling and calcium voltage-gated ion channels. They suggest potential approaches to manipulate NSC behavior for neural repair and regeneration, presenting novel opportunities for therapeutic interventions that promote neurogenesis and tissue recovery.
Exploring a system with potential insights into the initiation and advancement of brain tumors, particularly GBM, the most lethal form of adult brain cancer, presents an intriguing path for investigation [153],[154]. GBM tumors, akin to neurospheres, comprise a heterogeneous mix of cells, indicating significant diversity [154]. Evidence suggests that cells initiating glioblastoma share resemblances with NSCs [153]. Additionally, a critical role of calcium is governing various aspects of GBM tumor development, including cellular quiescence, proliferation, and migration [155]. Investigating the molecular, cellular, and calcium signaling characteristics of distinct cell types within neurospheres cultured in vitro is imperative for gaining insights into the etiology and progression of GBM and related neurodegenerative disorders. The establishment and comprehensive characterization of primary brain cancer cell cultures derived from patients are fundamental steps in identifying the impact of various calcium regulation and modulation on GBM progression, thereby aiming to identify novel therapeutic targets for these pathological conditions [156].
The manipulation of GSC characteristics, particularly the development of a dormant state crucial for evading current anti-cancer therapies, seems to involve intracellular calcium reservoirs within organelles such as the ER and mitochondria. The role of IP3R in this mechanism was elucidated through the discovery of bisacodyl, a selective cytotoxic molecule that specifically targets GSCs cultured under conditions inducing quiescence [157]. Further investigations unveiled that bisacodyl acts on IP3R to hinder calcium release from the ER [158], suggesting a pivotal role for ER-mediated calcium regulation in sustaining GSC dormancy and resistance to chemotherapy.
In addition to the ER, the regulation of calcium by mitochondria may also play a role in governing the stem cell properties of GSCs. Transcriptomic analysis of the calcium machinery highlighted an upsurge in the expression of the mitochondrial calcium transporter MCU and the solute carrier family 8 (Ca2+/Na+ exchanger), member 3 (SLC8A3) in GSCs (Figure 3) [159]. The actively dividing cells display more prolonged calcium signals compared to dormant GSCs, and changes in calcium responses are associated with alterations in mitochondrial structure [160].
The regulation of GSCs by SOC channels is still poorly understood. One possible explanation is that SOC channels may impact the division mode of GSCs [161]. GSCs have the capability to undergo two distinct types of division: symmetric proliferative expansion, which yields two GSCs and amplifies the GSC pool within the tumor, or asymmetric division, producing one GSC and one non-GSC progeny, thereby sustaining the GSC population [162]. SOC inhibitors diminish symmetric cell division in subventricular NSCs, which are potential progenitors for GBM [85]. This diminishes self-renewal and leads to a reduction in the stem cell population, mirroring the effects observed in GSCs treated with BMP4 [163].
Examination of the transcriptome of GSCs has unveiled a heightened presence of calcium channels and associated signaling pathways. These pathways play pivotal roles in coordinating crucial cellular responses to external cues, distinguishing them from their more differentiated non-stem counterparts in GBM, which demonstrate elevated levels of calcium buffers [164]. The disruption of calcium homeostasis and signaling dynamics may potentially enhance tumorigenic processes. Accordingly, observations have shown that epigenetic medications, which augment the stemness of GSCs, can influence the calcium signaling pathway [165].
The analysis of genes involved in calcium signaling revealed significant changes in genes associated with SOCE. Particularly, Orai1 showed increased expression levels in both GBM tissues and GSCs [159]. The increased expression of Orai1 and/or SOC channels in GSCs may play a role in sustaining or expanding the GSC population, thereby promoting the invasive characteristics of glioblastoma cells [166]. Blocking SOC channels with SKF-96365 reduces the growth of GSCs and triggers a gene expression pattern linked to cellular quiescence in these cells [160]. Moreover, the analysis comparing transcriptomic profiles between stem-like and non-stem-like GBM cells demonstrated a notable increase in genes associated with calcium signaling pathways within the stem-like cell population [164].
The involvement of SOCE in GSCs underscores the significance of calcium in maintaining stemness, suggesting that SOCE functions as a critical calcium regulatory mechanism implicated in preserving stem cell characteristics in both normal and cancerous contexts [85],[167]–[169]. SOC-mediated calcium influx activates Ca2+/calmodulin-dependent protein kinase II (CaMKII) and NFAT signaling pathways (Figure 3) [58],[170]–[172]. Notably, inhibition of either CaMKII or NFAT diminishes stemness in GBM cell cultures, suggesting that calcium signaling plays a crucial role in maintaining or expanding the subset of tumor-initiating cells responsible for GBM recurrence [173]–[175].
While calcium channels are recognized as essential constituents in various cancer stem cells, the specific functions of SOC channels remain to be fully elucidated [176],[177]. This central involvement of calcium signaling is not confined to stem cells but also extends to overall tumor progression, leading to propositions that calcium dyshomeostasis is a hallmark of cancer [178],[179]. Therefore, it would be beneficial to plan future studies focused on investigating how calcium dyshomeostasis impacts GSC activities and to thoroughly examine the calcium toolkit involved in GBM initiation, progression, and recurrence [166],[180].
Calcium-dependent signaling pathways significantly influence the stemness and function of GSCs, as evidenced by differential NFAT1 expression between GSCs and differentiated cells. Silencing NFAT1 reduced GSC viability, self-renewal, and migration in vitro, while also impairing tumorigenesis in vivo. Conversely, NFAT1 overexpression promoted glioma growth through interaction with neurodevelopment protein 1-like 1 (NDEL1), collectively sustaining a primitive stem cell state in GSCs [173]. Furthermore, NFAT2 was identified as a critical regulator of GSCs, especially within the mesenchymal subtype of glioblastoma, where it enhances invasion, clonogenicity, and tumor growth by modulating HDAC1 (Figure 3) [175].
The analysis of brain tumor tissue from GBM patients revealed an overexpression of SOC, specifically TRPC1 and Orai1, along with their ER activator, STIM1 [180],[181]. Studies on conventional GBM cell lines have demonstrated that SOC overexpression promotes proliferation and invasion (Figure 3) [166],[182],[183], although there has been some controversy regarding this finding [184]. However, the precise role of SOC in GSC, the subset of cells responsible for tumor initiation, growth, and relapse, remains unclear [185]. Notably, SOCs are implicated in regulating the self-renewal of adult neural stem cells in the SVZ, which are considered potential cells of origin for GSC [8],[85].
A transcriptomic analysis underscores the significant involvement of calcium signaling in GSCs. Specifically, the entry of calcium through SOC channels plays a critical role in sustaining GSC stemness, thereby contributing to the aggressive characteristics of GBM [161],[164]. SOC channels facilitate prolonged calcium influx triggered by various extracellular signals like HGF or S1P, both recognized drivers of glioma progression and stemness promotion in GBM cells (Figure 3) [186]–[189]. Importantly, GSCs derived from patient tumor samples exhibit expression of Orai1, TRPC1, and STIM1, mirroring the expression in adult neural stem cells, which are considered as a source of origin for GSCs, at least in part [8],[85],[185],[190],[191].
A study has shown that specific SOC inhibitors can effectively reduce S1P-induced calcium entry. This finding supports the idea that GSCs express functional SOC channels capable of responding to signals from the tumor microenvironment [187]. The ability of SOC inhibitors to reduce calcium entry highlights the significance of these channels in cellular signaling processes within GSCs. Therefore, SOC-mediated calcium influx may play a pivotal role in enabling GSCs to transduce and integrate information from their surrounding tumor microenvironment, potentially influencing tumor growth and progression. Understanding this mechanism could open new avenues for modulating SOC channels to disrupt critical signaling pathways in glioblastoma.
Studies have demonstrated that T-type CaV3.2 channels are markedly elevated in GSCs compared to non-GSC tumor cells and normal tissue (Figure 3) [164],[192]. This elevation suggests that CaV3.2 channels play a critical role in the unique physiological properties and aggressive behavior of GSCs. Hypoxia, a well-established driver of resistance to anticancer therapies, further enhances the presence of CaV3.2 in GSCs. Conversely, treatment with mibefradil, an FDA-approved CaV3.2 inhibitor commonly used for hypertension, significantly reduces the GSC population by inducing their differentiation and diminishing their viability [192],[193].
Research indicates that GSCs exhibit higher levels of AMPA receptors compared to fully differentiated non-stem tumor cells (Figure 3) [164],[194]. Research has shown that AMPA receptors expressed by human glioma cells, cultured under conditions resembling stem cells, facilitate interactions between tumor cells and nearby neurons via neuron-glioma glutamatergic synapses. These investigations have clarified that neuronal activity-induced release of glutamate promotes glioma progression by stimulating glioma proliferation and invasion through calcium-dependent pathways involving AMPA receptors [195],[196].
Researchers discovered that atracurium besylate, functioning as a nAChR antagonist, is a potent compound capable of significantly inhibiting the clonogenic potential and inducing astroglial differentiation in GSCs isolated from glioblastoma patients. Conversely, the administration of a nAChR agonist counteracted the inhibitory effect of atracurium besylate on GSC self-renewal. Additionally, this study demonstrated that mice transplanted with GSCs pre-treated with atracurium besylate exhibited significantly improved survival rates, suggesting that blockade of nAChRs could diminish GSC stemness and/or cell proliferation (Figure 3) [197]. Future research should investigate how calcium regulation associated with nAChR activity in GSCs influences stemness and cell proliferation. This could uncover molecular mechanisms that may serve as potential therapeutic targets to control GSC activity.
The presence of extracellular nucleotides in the microenvironment has a profound impact on the behavior of GSCs. Research suggests that GSCs exhibit a significantly higher release of extracellular adenosine, estimated to be approximately ten times more compared to their differentiated tumor cell counterparts [198]. This surplus of adenosine can act as either an autocrine or paracrine signaling molecule, activating G-protein-coupled purinergic P2Y1R receptors to stimulate GSC proliferation or ionotropic purinergic P2X7 receptors to inhibit it [199]. The elevated adenosine release by GSCs may contribute to altered signaling pathways and regulatory mechanisms within the tumor microenvironment, potentially influencing tumor progression, immune evasion, and therapeutic responses.
Studies have demonstrated elevated expression levels of TRPV1 and TRPV2 in glioblastoma compared to normal tissue (Figure 3) [180]. Functional investigations have revealed distinct roles for these receptors in the progression of glioblastoma. Activation of TRPV1 induces tumor cell death, a process enhanced by the release of endovanilloids (natural compounds acting as internal ligands and triggers for TRPV1 channels) from migrating NSCs into the tumor mass. This phenomenon significantly reinforces the tumor-suppressive effect exerted by NSCs [114]. Conversely, TRPV2 works to diminish the stemness of GSCs. Increased expression of TRPV2 reduces GSC proliferation and promotes their differentiation into glial cells, as demonstrated by studies in mice implanted with TRPV2-overexpressing GSCs [200].
CaBPs also play a significant role in influencing GSC stemness. Notably, increased expression of S100A4 has been associated with a poorer prognosis in glioma patients, particularly those with glioblastoma of the mesenchymal molecular subgroup [201]. Furthermore, cells expressing S100A4 are enriched with GSCs and play a crucial role in GSC self-renewal and survival. Targeted elimination of S100A4-expressing cells in genetically modified mice prone to spontaneous gliomas effectively inhibited tumor expansion [201].
Understanding calcium dynamics and its multifaceted roles in cancer cell concentration is crucial. This involves exploring each step and their collective impact on calcium levels within cancer cells. Future research should prioritize investigating calcium's specific roles in GBM using sophisticated methodologies. These studies should encompass diverse approaches, including various human cell cultures and mouse models—both implanted and natural. The goal is to identify optimal techniques for studying calcium's influence on GBM at cellular and molecular levels.
Moreover, studying calcium signaling and its role in regulating NSCs within the SVZ and analyzing the behavior of different cell populations could greatly enhance our understanding of GBM. Through comparisons and identification of key differences, we can elucidate the factors and signals that contribute to uncontrolled cell proliferation in GBM.
Recent research emphasizes the crucial role of calcium in the adult brain, particularly within NSCs and GSCs [161],[202]. Understanding how the calcium toolkit regulates essential functions in adult brain stem cells, both in health and disease, is vital. This understanding holds significant promise for identifying clinical targets [177],[202] and advancing therapeutic strategies by manipulating calcium signaling pathways to enhance stem cell function, particularly in addressing brain cancer.
The progressive decline in stem cell capacity for self-renewal and differentiation with age is a key contributor to age-related human diseases, underscoring the complexity of aging, which affects cells, tissues, organs, and organisms through interconnected changes [203],[204]. Stem cell functionality is intricately regulated by epigenetic modifications, metabolic pathways, and levels of reactive oxygen species (ROS), all of which contribute to the decline in stem cell function observed with aging [205],[206]. Central to these age-related changes are alterations in gene expression patterns, influenced by precise calcium regulation, resulting in reduced stem cell populations and slower proliferation rates [207]. Calcium signaling, in particular, plays a critical role in modulating multiple processes, coordinating the overall impact of diverse mechanisms on aging [208],[209].
However, as individuals age, the capacity of stem cells for self-renewal and differentiation becomes increasingly limited, accompanied by a decrease in the overall number of stem cells [204],[210]. Gaining a deeper understanding of the cellular processes that contribute to aging will provide insights into the role of calcium in these processes and its significant impact on cell function over time. This area of research remains relatively underexplored and warrants further investigation to elucidate why humans age at certain rates and why these rates differ compared to other organisms.
The capacity of second messengers to manage extensive information and coordinate diverse cellular processes is a phenomenon that is partly understood through the inherent characteristics of cells, allowing for tailored responses to calcium signals [211]. Importantly, only cells equipped with a contractile apparatus exhibit shortening in response to calcium transients, a reaction exclusive to cells expressing essential calcium channels [22].
In this intricate process, spatiotemporal decoding is essential for connecting a calcium signal to specific cellular responses. Different manifestations of cytoplasmic calcium elevations—sustained, transient, or oscillatory—affect organelles accordingly [212]. The biological basis of calcium oscillations involves complex feedback loops among cytoplasmic calcium levels, intracellular calcium reservoirs, and the regulatory mechanisms governing calcium transport across the plasma membrane [213].
Understanding the periodic fluctuations of calcium involves examining their frequency, duration, and amplitude, which directly influence different calcium mechanisms [214]. Several studies indicate that various factors such as spike width, amplitude, sustainability, and baseline calcium levels work together to produce distinct functional outcomes [213].
Understanding all these parameters of calcium regulation in cells is essential for comprehending calcium's roles and functions, as well as how it can be better utilized for therapeutic interventions related to brain repair, aging, or GBS.
Furthermore, regulation of cellular processes heavily depends on the kinetics of calcium binding to specific effectors and their subsequent enzymatic impact on cellular targets. Key effectors in this context include calmodulin (CaM) and Ca2+/calmodulin-dependent protein kinases (CaMKs) [27]. Furthermore, cytoplasmic calcium levels are not uniform; they can create localized calcium microdomains near CRAC channels, ryanodine receptors, and IP3Rs, located at the endoplasmic reticulum–plasma membrane junction [215].
The significance of these microdomains is illustrated by the MCU, which acts as a universal regulator of intracellular calcium signaling across mammalian cell types [216]. The MCU's affinity for calcium is insufficient for activation at typical cytosolic calcium levels. However, it becomes effective near mitochondria-endoplasmic reticulum contacts (MAMs), where calcium concentrations increase during ER calcium release [215],[217]. Understanding this mechanism of intracellular calcium regulation may provide valuable insights into the progression of GSCs and their role in local GBM growth. This knowledge has the potential to pave the way for future research and the identification of novel therapeutic targets.
Moreover, nuclear calcium dynamics, which directly regulate cellular functions within the nucleus, are influenced by calcium influx from stores within the nuclear envelope and the nucleoplasmic reticulum, both of which are continuous with the ER. The distinct roles and independent regulation of nuclear versus cytoplasmic calcium are mediated by NFAT isoforms [218]. Specifically, NFAT1 is preferentially activated in microdomains near CRAC channels, indicating a localized and specific calcium signaling response. Conversely, NFAT4 activation necessitates elevated levels of both cytoplasmic and nuclear calcium, illustrating the complex interplay between these cellular compartments [219]. The intricate regulation of nuclear calcium signaling underscores the sophisticated mechanisms that cells employ to precisely modulate their functions, ensuring appropriate responses to varying physiological conditions. Gaining a deeper understanding of all facets of calcium regulation will enhance our knowledge of calcium's role in cellular processes and aid in the discovery of new therapeutic options.
The microenvironments where NSCs, GSCs, and other cells reside play a crucial role in shaping their responses to physiological or pathological conditions [185],[220]. In these specialized niches, various signals trigger transient increases in calcium concentrations, occurring either locally within cellular microdomains or spanning the entire cell [16]. The spatio-temporal dynamics of these calcium signals are pivotal for determining cellular reactions to external stimuli [212], emphasizing the critical need for precise regulation of calcium levels to maintain optimal cellular functions.
To achieve calcium regulation, a complex network of molecules is involved. Calcium permeable channels facilitate the influx of calcium from the extracellular space into the cell across the plasma membrane, while intracellular calcium is released from reservoirs such as the ER and mitochondria. Moreover, calcium pumps and exchangers work to restore calcium balance within cellular stores or remove excess calcium from the cytosol. Additionally, CaBPs function as buffers to regulate cytosolic calcium concentrations or act as effectors in downstream signaling pathways [221],[222]. While this knowledge has been known for some time, future experiments should focus on understanding how calcium regulation dynamics occur within microdomains and in the cellular niche. It is crucial to investigate how changes in calcium concentration within different compartments are affected by various factors and how they can be controlled, leading to specific outcomes. This knowledge is pivotal for comprehending calcium signaling and its dynamic regulatory processes, such as those involved in cellular proliferation and the progression of glioblastoma multiforme (GBM) growth over time. Understanding these mechanisms can also shed light on aging processes and diseases related to calcium regulation, ultimately facilitating the discovery of future therapeutic targets for treating associated disorders.
The authors declare they have not used Artificial Intelligence (AI) tools in the creation of this article.
[1] |
C. Alonso, M. Borca, L. Dixon, Y Revilla, F. ROdriguez, J. M. Escribano, ICTV virus taxonomy profile: Asfarviridae, J. Gen. Virol., 99 (2018), 613–614. https://doi.org/10.1099/jgv.0.001049 doi: 10.1099/jgv.0.001049
![]() |
[2] | C. M. Fauquet, M. A. Mayo, J. Maniloff, U. Desselberger, L. A. Ball, Virus taxonomy: VIIIth report of the International Committee on Taxonomy of Viruses, Academic Press, 2005. |
[3] |
S. Costard, B. Wieland, W. De Glanville, F. Jori, R. Rowlands, W. Vosloo, et al., African swine fever: how can global spread be prevented?, Phil. Trans. R. Soc. B, 364 (2009), 2683–2696. https://doi.org/10.1098/rstb.2009.0098 doi: 10.1098/rstb.2009.0098
![]() |
[4] |
C. Guinat, A. Gogin, S. Blome, G. Keil, R. Pollin, D. U. Pfeiffer, et al., Transmission routes of African swine fever virus to domestic pigs: current knowledge and future research directions, Vet. Rec., 178 (2016), 262–267. https://doi.org/10.1136/vr.103593 doi: 10.1136/vr.103593
![]() |
[5] |
H. Nishiura, Early efforts in modeling the incubation period of infectious diseases with an acute course of illness, Emerg. Themes Epidemiology, 4 (2007), 1–12. https://doi.org/10.1186/1742-7622-4-2 doi: 10.1186/1742-7622-4-2
![]() |
[6] |
I. Galindo, C. Alonso, African swine fever virus: a review, Viruses, 9 (2017), 103. https://doi.org/10.3390/v9050103 doi: 10.3390/v9050103
![]() |
[7] |
C. J. Quembo, F. Jori, W. Vosloo, L. Health, Genetic characterization of African swine fever virus isolates from soft ticks at the wildlife/domestic interface in Mozambique and identification of a novel genotype, Transbound. Emerg. Dis., 65 (2018), 420–431. https://doi.org/10.1111/tbed.12700 doi: 10.1111/tbed.12700
![]() |
[8] | E. R. Tulman, G. A. Delhon, B. K. Ku, D. L. Rock, African Swine Fever Virus. In: Van Etten, J.L. (eds) Lesser Known Large dsDNA Viruses. Current Topics in Microbiology and Immunology, Springer, Berlin, Heidelberg, 2009. https://doi.org/10.1007/978-3-540-68618-7_2 |
[9] |
X. Shen, Z. Pu, Y. Li, S. Yu, F. Guo, T. Luo, et al., Phylogeographic patterns of the African swine fever virus, J. Infect., 79 (2019), 174–187. https://doi.org/10.1016/j.jinf.2019.05.004 doi: 10.1016/j.jinf.2019.05.004
![]() |
[10] |
T. Wang, Y. Sun, H. J. Qiu, African swine fever: an unprecedented disaster and challenge to China, Infec. Dis. Poverty, 7 (2018), 66–70. https://doi.org/10.1186/s40249-018-0495-3 doi: 10.1186/s40249-018-0495-3
![]() |
[11] |
J. Bao, Q. Wang, P. Lin, C. Liu, L. Li, X. Wu, et al., Genome comparison of African swine fever virus China/2018/AnhuiXCGQ strain and related European p72 Genotype II strains, Transbound. Emerg. Dis., 66 (2019), 1167–1176. https://doi.org/10.1111/tbed.13124 doi: 10.1111/tbed.13124
![]() |
[12] |
X. Zhou, N. Li, Y. Luo, Y. E. Liu, F. Miao, T. Chen, et al., Emergence of African swine fever in China, 2018, Transbound. Emerg. Dis., 65 (2018), 1482–1484. https://doi.org/10.1111/tbed.12989 doi: 10.1111/tbed.12989
![]() |
[13] |
S. Ge, J. Li, X. Fan, F. Liu, L. Li, Q. Wang, et al., Molecular characterization of African swine fever virus, China, 2018, Emerg. Infect. Dis., 24 (2018), 2131–2133. https://doi.org/10.3201/eid2411.181274 doi: 10.3201/eid2411.181274
![]() |
[14] |
Q. Wang, W. Ren, J. Bao, S. Ge, J. Li, L. Li, et al., The first outbreak of African swine fever was confirmed in China, China Animal Health Inspection, 35 (2018), 1–4. https://doi.org/10.3969/j.issn.1005-944X.2018.09.001 doi: 10.3969/j.issn.1005-944X.2018.09.001
![]() |
[15] |
Y. Wang, L. Gao, Y. Li, Q. Xu, H. Yang, C. Shen, et al., African swine fever in China: Emergence and control, J. Biosaf. Biosecur., 1 (2019), 7–8. https://doi.org/10.1016/j.jobb.2019.01.006 doi: 10.1016/j.jobb.2019.01.006
![]() |
[16] |
H. Song, W. Jiang, S. Liu, Global dynamics of two heterogeneous SIR models with nonlinear incidence and delays, Int. J. Biomath., 9 (2016), 1650046. https://doi.org/10.1142/S1793524516500467 doi: 10.1142/S1793524516500467
![]() |
[17] |
H. Song, S. Liu, W. Jiang, Global dynamics of a multistage SIR model with distributed delays and nonlinear incidence rate, Math. Methods Appl. Sci., 40 (2017), 2153–2164. https://doi.org/10.1002/mma.4130 doi: 10.1002/mma.4130
![]() |
[18] |
H. Song, F. Li, Z. Jia, Z. Jin, S. Liu, Using traveller-derived cases in Henan Province to quantify the spread of COVID-19 in Wuhan, China, Nonlinear Dyn., 101 (2020), 1821–1831. https://doi.org/10.1007/s11071-020-05859-1 doi: 10.1007/s11071-020-05859-1
![]() |
[19] |
H. Song, Z. Jia, Z. Jin, S. Liu, Estimation of COVID-19 outbreak size in Harbin, China, Nonlinear Dyn., 106 (2021), 1229–1237. https://doi.org/10.1007/s11071-021-06406-2 doi: 10.1007/s11071-021-06406-2
![]() |
[20] |
H. Song, G. Fan, S. Zhao, H. Li, Q. Huang, D. He, Forecast of the COVID-19 trend in India: a simple modelling approach, Math. Biosci. Eng., 18 (2021), 9775–9786. https://doi.org/10.3934/mbe.2021479 doi: 10.3934/mbe.2021479
![]() |
[21] |
H. Song, G. Fan, Y. Liu, X. Wang, D. He, The Second Wave of COVID-19 in South and Southeast Asia and the Effects of Vaccination, Front. Med., 8 (2021), 773110. https://doi.org/10.3389/fmed.2021.773110 doi: 10.3389/fmed.2021.773110
![]() |
[22] |
H. Song, F. Liu, F. Li, C. Cao, H. Wang, Z. Jia, et al., Modeling the second outbreak of COVID-19 with isolation and contact tracing, Discrete Cont. Dyn.-B, 27 (2022), 5757–5777. https://doi.org/10.3934/dcdsb.2021294 doi: 10.3934/dcdsb.2021294
![]() |
[23] | H. Song, Z. Jin, C. Shan, L. Chang, The spatial and temporal effects of Fog-Haze pollution on the influenza transmission, Int. J. Biomath., (2022), 2250096. https://doi.org/10.1142/S1793524522500966 |
[24] |
F. I. Korennoy, V. M. Gulenkin, A. E. Gogin, T. Vergne, A. K. Karaulov, Estimating the basic reproductive number for African swine fever using the Ukrainian historical epidemic of 1977, Transbound. Emerg. Dis., 64 (2017), 1858–1866. https://doi.org/10.1111/tbed.12583 doi: 10.1111/tbed.12583
![]() |
[25] |
C. Guinat, A. L. Reis, C. L. Netherton, L. Goatley, D. U. Pfeiffer, L.Dixon, Dynamics of African swine fever virus shedding and excretion in domestic pigs infected by intramuscular inoculation and contact transmission, Vet. Res., 45 (2014), 1–9. https://doi.org/10.1186/s13567-014-0093-8 doi: 10.1186/s13567-014-0093-8
![]() |
[26] |
M. B. Barongo, R. P. Bishop, E. M. Fevre, D. L. Knobel, A. Ssematimba, A mathematical model that simulates control options for African swine fever virus (ASFV), PLoS One, 11 (2016), e0158658. https://doi.org/10.1371/journal.pone.0158658 doi: 10.1371/journal.pone.0158658
![]() |
[27] |
X. O'Neill, A. White, F. Ruiz-Fons, C. Gortazar, Modelling the transmission and persistence of African swine fever in wild boar in contrasting European scenarios, Sci. Rep., 10 (2020), 1–10. https://doi.org/10.1038/s41598-020-62736-y doi: 10.1038/s41598-020-62736-y
![]() |
[28] |
J. Li, Z. Jin, Y. Wang, X. Sun, Q. Xu, J. Kang, et al., Data-driven dynamical modelling of the transmission of African swine fever in a few places in China, Transbound. Emerg. Dis., 69 (2021), e646–e658. https://doi.org/10.1111/tbed.14345. doi: 10.1111/tbed.14345
![]() |
[29] |
J. M. Sanchez-Vizcaino, L. Mur, J. C. Gomez-Villamandos, L. Carrasco, An update on the epidemiology and pathology of African swine fever, J. Comp. Pathol., 152 (2015), 9–21. https://doi.org/10.1016/j.jcpa.2014.09.003 doi: 10.1016/j.jcpa.2014.09.003
![]() |
[30] | M. Arias, J. M. Sanchez-Vizcaino, A. Morilla, K. J. Yoon, J. J. Zimmerman, African swine fever, Trends in emerging viral infections of swine, (2002), 119–124. |
[31] | H. K. Khalil, Nonlinear Systems, New York: Macmillan Co., 1992. |
[32] |
P. van den Driessche, J. Watmough, Reproduction numbers and sub-threshold endemic equilibria for compartmental models of disease transmission, Math. Biosci., 180 (2002), 29–48. https://doi.org/10.1016/S0025-5564(02)00108-6 doi: 10.1016/S0025-5564(02)00108-6
![]() |
[33] | Lasalle J. The Stability of Dynamical Systems, SIAM, Philadelphia, 1976. |
[34] | The China Animal Health Endemic Center, https://www.cahec.cn/. |
[35] |
X. Zhang, X. Rong, J. Li, M. Fan, Y. Wang, X. Sun, et al., Modeling the outbreak and control of African swine fever virus in large-scale pig farms, J. Theor. Biol., 526 (2021), 110798. https://doi.org/10.1016/j.jtbi.2021.110798 doi: 10.1016/j.jtbi.2021.110798
![]() |
[36] |
M. B. Barongo, K. Stahl, B. Bett, R. P. Bishop. E. M. Fevre, T. Aliro, et al., Estimating the basic reproductive number (R0) for African swine fever virus (ASFV) transmission between pig herds in Uganda, PloS One, 10 (2015), e0125842. https://doi.org/10.1371/journal.pone.0125842 doi: 10.1371/journal.pone.0125842
![]() |
[37] | M. B. Bitamale, Modelling the transmission dynamics and the effect of different control strategies for African swine fever virus in East Africa, Ph.D thesis, University of Pretoria, 2018. http://hdl.handle.net/2263/67858 |
[38] |
S. Marino, I. B. Hogue, C. J. Ray, D. E. Kirschner, A methodology for performing global uncertainty and sensitivity analysis in systems biology, J. Theor. Biol., 254 (2008), 178–196. https://doi.org/10.1016/j.jtbi.2008.04.011 doi: 10.1016/j.jtbi.2008.04.011
![]() |
1. | Abdelrazig K. Tarboush, Zhengdi Zhang, The Diffusive Model for West Nile Virus on a Periodically Evolving Domain, 2020, 2020, 1076-2787, 1, 10.1155/2020/6280313 | |
2. | Yasir Ezzeldien Salih Amin, Eltayib Hassan Ahmed-Abakur, West Nile virus IgG antibodies among blood donors in Sudan: a cross-sectional study, 2022, 49-50, 20522975, 101062, 10.1016/j.nmni.2022.101062 | |
3. | Kangkang Chang, Zhenyu Zhang, Guizhen Liang, Threshold dynamics of a nonlocal diffusion West Nile virus model with spatial heterogeneity, 2023, 8, 2473-6988, 14253, 10.3934/math.2023729 |