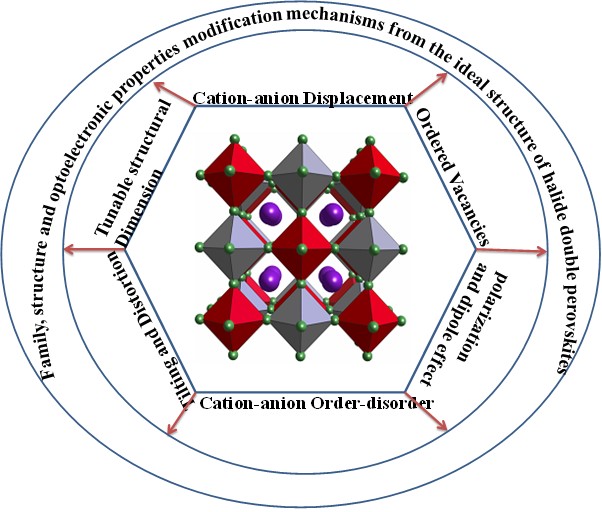
The stability issues in the widely known CH3NH3PbI3, lead to the development of alternative halide double perovskite materials, which has received great attention in recent times. Although the stability issue of double halide perovskite seems promising, their device performance remains far inferior to CH3NH3PbI3 and with challenges for further improvements. Furthermore, the power conversion efficiency of single junction organic-inorganic halide perovskite is now 24.2% and 29.15% for the textured monolithic perovskite/silicon tandem solar cell; however, for the all-inorganic halide perovskite solar cell, it is 7.11%, and halide double perovskite solar cells are based on A2BⅠBⅢX6 (A = monocation, B = cation or vacancy, X = halide) such as Cs2AgBiBr6, Cs2TiBr6, Cs2AgTlBr6 and Cs2Ag(Bi1−xInx)Br6, being 2.8% and 3.3%, respectively. This creates big questions and concerns about the performance improvement of A2BⅠBⅢX6-based perovskite solar cells. Not only is this a concern, but there are many other big challenges faced by halide double perovskite solar cells. Such big challenges include: (a) geometric constraints and limited integration with interfacial materials; (b) dynamic disorder, a wide band gap, and a localized conduction band caused by a cubic unit cell that restrains the interactions of orbitals; (c) high processing temperature which may limit the diverse applications; and (d) low electronic dimensionality that makes them less appropriate for single junction solar cell purpose, etc. Moreover, the origin of electronic and optical properties such as the polarizability, the presence of molecular dipoles, and their influence on the dynamics of the photo-excitations remain bottleneck concerns that need to be elucidated. We roadmap performance sustainable improvement, which is suggested with a particular focus on engineering material surface and bulk, band gap, interfacial, composition, doping, device architectural, polar, and domain order. The reason that this review was developed was to forward great contributions to the readers and commercial ventures.
Citation: Etsana Kiros Ashebir, Berhe Tadese Abay, Taame Abraha Berhe. Sustainable A2BⅠBⅢX6 based lead free perovskite solar cells: The challenges and research roadmap for power conversion efficiency improvement[J]. AIMS Materials Science, 2024, 11(4): 712-759. doi: 10.3934/matersci.2024036
[1] | M. F. Achoi, S. Kato, N. Kishi, T. Soga . Improved photovoltaic properties of ((CH3NH3)1-xCsx)3Bi2I9: (x = 0-1.0) hybrid perovskite solar cells via a hot immersion method. AIMS Materials Science, 2024, 11(4): 605-619. doi: 10.3934/matersci.2024031 |
[2] | Lucangelo Dimesso, Michael Wussler, Thomas Mayer, Eric Mankel, Wolfram Jaegermann . Inorganic alkali lead iodide semiconducting APbI3 (A = Li, Na, K, Cs) and NH4PbI3 films prepared from solution: Structure, morphology, and electronic structure. AIMS Materials Science, 2016, 3(3): 737-755. doi: 10.3934/matersci.2016.3.737 |
[3] | Nhung Thi-Tuyet Hoang, Anh Thi-Kim Tran, Nguyen Van Suc, The-Vinh Nguyen . Antibacterial activities of gel-derived Ag-TiO2-SiO2 nanomaterials under different light irradiation. AIMS Materials Science, 2016, 3(2): 339-348. doi: 10.3934/matersci.2016.2.339 |
[4] | Radi A. Jishi . Modified Becke-Johnson exchange potential: improved modeling of lead halides for solar cell applications. AIMS Materials Science, 2016, 3(1): 149-159. doi: 10.3934/matersci.2016.1.149 |
[5] | R.A. Silva, C.O. Soares, R. Afonso, M.D. Carvalho, A.C. Tavares, M.E. Melo Jorge, A. Gomes, M.I. da Silva Pereira, C.M. Rangel . Synthesis and electrocatalytic properties of La0.8Sr0.2FeO3−δ perovskite oxide for oxygen reactions. AIMS Materials Science, 2017, 4(4): 991-1009. doi: 10.3934/matersci.2017.4.991 |
[6] | Leydi J. Cardenas F., Josep Ma. Chimenos, Luis C. Moreno A., Elaine C. Paris, Miryam R. Joya . Enhancing Co3O4 nanoparticles: Investigating the impact of nickel doping and high-temperature annealing on NiCo2O4/CoO heterostructures. AIMS Materials Science, 2023, 10(6): 1090-1104. doi: 10.3934/matersci.2023058 |
[7] | Sree Sourav Das, Zach Fox, Md Dalim Mia, Brian C Samuels, Rony Saha, Ravi Droopad . Demonstration of ferroelectricity in PLD grown HfO2-ZrO2 nanolaminates. AIMS Materials Science, 2023, 10(2): 342-355. doi: 10.3934/matersci.2023018 |
[8] | Jamal Alnofiay, Ahmed Al-Shahrie, Elsayed Shalaan . Green synthesis of high-performance gallium oxide supercapacitor: A path to outstanding energy density. AIMS Materials Science, 2024, 11(6): 1065-1082. doi: 10.3934/matersci.2024051 |
[9] | Marek Konieczny . Transformation superplasticity of laminated CuAl10Fe3Mn2 bronze-intermetallics composites. AIMS Materials Science, 2020, 7(3): 312-322. doi: 10.3934/matersci.2020.3.312 |
[10] | Dao Viet Thang, Le Thi Mai Oanh, Nguyen Cao Khang, Nguyen Manh Hung, Do Danh Bich, Du Thi Xuan Thao, Nguyen Van Minh . Structural, magnetic and electric properties of Nd and Ni co-doped BiFeO3 materials. AIMS Materials Science, 2017, 4(4): 982-990. doi: 10.3934/matersci.2017.4.982 |
The stability issues in the widely known CH3NH3PbI3, lead to the development of alternative halide double perovskite materials, which has received great attention in recent times. Although the stability issue of double halide perovskite seems promising, their device performance remains far inferior to CH3NH3PbI3 and with challenges for further improvements. Furthermore, the power conversion efficiency of single junction organic-inorganic halide perovskite is now 24.2% and 29.15% for the textured monolithic perovskite/silicon tandem solar cell; however, for the all-inorganic halide perovskite solar cell, it is 7.11%, and halide double perovskite solar cells are based on A2BⅠBⅢX6 (A = monocation, B = cation or vacancy, X = halide) such as Cs2AgBiBr6, Cs2TiBr6, Cs2AgTlBr6 and Cs2Ag(Bi1−xInx)Br6, being 2.8% and 3.3%, respectively. This creates big questions and concerns about the performance improvement of A2BⅠBⅢX6-based perovskite solar cells. Not only is this a concern, but there are many other big challenges faced by halide double perovskite solar cells. Such big challenges include: (a) geometric constraints and limited integration with interfacial materials; (b) dynamic disorder, a wide band gap, and a localized conduction band caused by a cubic unit cell that restrains the interactions of orbitals; (c) high processing temperature which may limit the diverse applications; and (d) low electronic dimensionality that makes them less appropriate for single junction solar cell purpose, etc. Moreover, the origin of electronic and optical properties such as the polarizability, the presence of molecular dipoles, and their influence on the dynamics of the photo-excitations remain bottleneck concerns that need to be elucidated. We roadmap performance sustainable improvement, which is suggested with a particular focus on engineering material surface and bulk, band gap, interfacial, composition, doping, device architectural, polar, and domain order. The reason that this review was developed was to forward great contributions to the readers and commercial ventures.
A definitive objective of materials utilized in optoelectronic applications ought to be founded on the "triple-E" terms: financially economical, vitality effective, and naturally efficient. Despite their very great execution in lead halide perovskite based solar cells with efficiencies reaching 24.2% and the rising application for lasers [1], light-radiating diodes (LEDs) [2], field-impact light-producing transistors (FETs) [3], organic−inorganic lead halide perovskites experience the ill effects of substances of lethal, contaminating, and bioaccumulative Pb, which may, in the end, hamper their commercialization. Progressively, it turns out to be of essential significance to consider and create alternative classes of lead-free halide double perovskites for various optoelectronic applications [4].
The need to create practical advances is dependent on ecologically neighborly, earth-inexhaustible, and financially savvy materials that normally drive the consideration toward the change metals with especially appealing metals (e.g., Fe2+, Cu2+, Zn2+) and post-transition elements [5,6]. For example, tin [7,8], rubidium [9,10], indium-silver [11,12], titanium [13], bismuth and silver-bismuth [11,14,15,16,17], and copper [18] based perovskites have been found as potential choices to toxic perovskites. Thanks to their rich science, their utilization may tremendously broaden engineered courses of new halide double perovskites for photovoltaic and light-radiating applications, enhancing the tunability of the material. Moreover, the discovery of lead-free double perovskites provides a feasible way of searching for air-stable and environmentally benign solar cell absorbers [19,20,21,22,23]. Since the 1970s, a large number of double perovskites (which are well known as elpasolites) with nominal chemical compositions of A2BⅠBⅢX6 (A and BⅠ = Li+, Na+, K+, Rb+, Cs+, Ag+, Tl+, etc., BⅢ = Bi3+, Al3+, Ln3+, Ga3+, Fe3+, etc., and X = F, Cl, Br, or I) have been initially reported as ferroelectric materials [24,25]. However, the lead and tin free all-inorganic halide double perovskite solar cell power conversion efficiency is far smaller than the power conversion efficiency (PCE) of lead based perovskite solar cells. The lead and tin free all-inorganic halide double perovskites PCE improvement is too slow compared to lead halide perovskites PCE, which has become a great concern and active research direction for the halide double perovskite scientific community. In conjunction with the advancement of halide double perovskite solar cells, interesting concepts covering cationic exchange, doping, and alloying for engineering the electronic structure of double perovskites [26], its crystal structure include preparation approaches, physicochemical properties, and material applications [6,27,28] with various strategies such as hetero-substitution of Pb to form quaternary halide double perovskites [5] and a strategy for achieving small band gaps in this family of materials [29]. Moreover, the PCE of single junction lead based perovskite is now 24.2% [30], 25.2% [31], and 29.15% [32] for the textured monolithic perovskite/silicon tandem solar cell, but the record for lead-free all inorganic cesium tin-germanium triiodide (CsSn0.5Ge0.5I3) solid-solution perovskite is 7.11% [33]. According to the simulation results reported, CsSn0.5Ge0.5I3 perovskite material can have a power conversion efficiency of 18.79% [34] and 24.63% [35]. Furthermore, the CsSn0.5Ge0.5I3/FASnI3 device architecture is reported with a simulated power conversion efficiency of 31.58% [36]. This indicates that the CsSn0.5Ge0.5I3/FASnI3 device is a promising device for high-performance lead-free solar cells.
This efficiency can be achieved if encapsulation mechanisms, fabrication procedures, and material parameters such as defect density, layer thickness, operating temperature, electron affinity, and potential energy are well optimized during an experiment. Moreover, tin-based perovskite solar cells have promising efficiency next to lead-based perovskite solar cells as devices made of Tin based perovskite materials exhibit insufficient stability [37] and contain intrinsically deep defects that are detrimental to the perovskite solar cell performance [38]. Even the most stable CsSn0.5Ge0.5I3 based perovskite solar cell showed 10% decay efficiency after 500 h of continuous operation in an N2 atmosphere under one-sun illumination [33]. Hence, to overcome the toxicity of lead and insufficient stability of Tin, efforts to find alternative perovskite materials, which are earth-abundant, non-toxic, stable, and biocompatible, are in progress.
Based on these needs, lead and tin free A2BⅠBⅢX6 based all-inorganic solar cells such as Cs2AgBiBr6 and Cs2TiBr6 have been reported recently [39]. The records for Cs2AgBiBr6 and Cs2TiBr6 lead and a tin-free, all-inorganic halide double perovskite solar cell are 2.5% [40] (current report is 2.81% [41] with a photoelectronic conversion efficiency of hydrogenated Cs2AgBiBr6 perovskite solar cell of 6.37% [42]) and 3.3% [43], respectively. These are far less than 24.2% for single junction lead-based halide perovskite solar cells and 29.15% for a tandem solar cell. Consequently, the progress is too slow to reach the hybrid lead halide perovskite solar cells. From a theoretical perspective, simulation results reported that Cs2TiBr6 has achieved 11.49% power conversion efficiency [44]. This is promising for manufacturing practical lead-free, double halide perovskite solar cells. More recently, an Au/CuSbS2/Cs2TiBr6/CdS/TCO configuration has achieved a power conversion efficiency of 23.77%, as reported by simulated results [45]. This is again a more promising result in this field. Unless the real and bottleneck challenges and solutions are clearly identified, the Cs2AgBiBr6 and Cs2TiBr6 based halide double perovskite solar cell will not be the first alternative solar cell to replace the hybrid lead halide perovskite solar cells. Thus, this review article is designed to identify the challenges and possible solutions for power conversion efficiency improvement of halide, double perovskite solar cells that will be considered a research roadmap for performance improvements in this field. Hence, this is a comprehensive review that concisely reviews the modification strategies from CH3NH3PbI3 into many halide double perovskite materials, the origin of electronic and optical properties variations, and key challenges and areas of research for possible solutions and performance improvement strategies of both Lead and Tin free all-inorganic halide double perovskite solar cells. Moreover, this will provide a new perspective and research roadmap for performance improvement to the scientific community and research industries.
The issue of sustainability in lead halide perovskite solar cells is the biggest challenge. This sustainability concept is not only understood in terms of economic viability, toxicity, and stability but also affordability, availability of materials, energy independence, quality of being able to continue over a period of time, free of hazardous materials, etc. Now, we question whether A2BⅠBⅢX6 such as Cs2AgBiBr6 and Cs2TiBr6 based perovskite solar cells can answer all concepts of sustainability so that it can revolutionize the photovoltaic field of research or not? We seek to answer this question.
The organic-inorganic halide perovskites become capable of photovoltaic and energy applications beyond photovoltaic. However, the large scale commercialization of the perovskite harvester faces considerable limitations due to the toxicity of lead metal and the perovskite absorber degradation [6,46,47,48]. For this reason, there is an interest to discover nontoxic and stable perovskites for the betterment of this field. The degradation concern can be treated by carbon encapsulation, multi-cation substitution, and incorporation of hydrophobic moieties [47,49]. On the other hand, substitution of Pb using non-toxic transition metal atoms is the single strategy to overcome the toxicity problems [46,50,51]. Consequently, the optoelectronic properties [52] such as direct band gaps [19,53], electron mobility [54], defect tolerance, high absorption coefficient [55], small carrier effective masses, intrinsic thermodynamic stability [56], long charge carrier diffusion lengths [57], low exciton binding energies, higher dielectric constant, and compatibility with low-cost and solution-supported fabrication procedures [58] are considered promising candidate properties to replace lead-based solar cell light harvesting materials in perovskite solar cells. Solving the predictable restrictions of solution-processed semiconductors is another important candidate topic that needs to be considered in the lead replacement process [59,60,61,62,63]. Moreover, there are promising candidate materials that can overcome the issue of toxicity, instability, lifetime, and device deterioration of lead based halide perovskite. Such materials are the topic of this review, with a general formula assigned as A2BⅠBⅢX6 based lead free perovskite solar cells.
Solar cell devices made of organic-inorganic lead halide perovskite material (CH3NH3PbI3) has been reported in 2009 with a cell efficiency of 3.81% [64], indicating that CH3NH3PbI3 with an efficiency of 24.2% [30] was achieved in 2019 and has reached 29.15% [32]. This is promising if the device made further optimized continuously. Gradually, modification of CH3NH3PbI3 has been done at three sites, as shown in Scheme 1 [65]: strategy (1) halogen atoms like chlorine, bromine, and a combination of all these halogens [66,67,68,69]; strategy (2) organic part [70,71,72,73]; and strategy (3) at an inorganic Pb metal site such as Ge [74], resulting in many derivatives of CH3NH3PbI3 materials classified as organic-inorganic perovskites, fully inorganic perovskites, and halide double perovskites etc. All these modifications are the subject of this section and are classified as cation displacement at A and B site and anion displacement at X-site. Furthermore, all these cation and anion modifications, as well as the tunable structural dimensions, cation-anion order-disorder, distortions, and tilting of the octahedral unit, BX6, from the ideal structure, are collectively considered as the origin of diversity in materials structure, dimension, and optoelectronic properties. Based on these modifications, iodide, bromide, mixed metals, and mixed I/Br ratio-based double perovskites such as Cs2AgBiBr6, Cs2AgBiCl6, Cs2AgInCl6, Cs2NaBiCl6, Cs2Ag(SbxBi1−x)Br6, and Cs2AgBi(Br/I)6 or Cs2AgBiBr6−xIx, have become widely known double perovskite light absorbing materials [75]. The challenge in these materials is the large band gap. To solve this challenge, compositional engineering plays a great role in discovering new optical and electrical properties in these materials. Mixed compositions in the metal and halogen site have been investigated to explore these materials [76,77]. This approach helps to discover stable and nontoxic halide double perovskite materials, which are the main focus of this review.
In addition to the structural variations, optoelectronic properties such as absorption, electron-hole diffusion length, carrier life time, carrier mobility, charge carrier dynamics, electronic structure such as band structure and density of state, effective mass of electron, thermal properties, and other electrical properties such as ferroelectric, pyroelectric, and piezoelectric properties are the major material properties that cause differences in device efficiency; moreover, everyone wants to deal with this for further energy application of materials [78]. Recently, the field of organic-inorganic hybrid halide perovskite has revolutionized the field of photovoltaics. Moreover, modifying the halide perovskites gives new halide double perovskites for which the stated properties are not well studied. Thus, understanding the origin of these optoelectronic property variations would be of great interest for the development of halide double perovskite material performance. In this section, molecular dipoles, tilting of octahedron, transition metal cation size and its oxidation states, band structure, photoluminescence, and other optoelectric properties are considered as potential origins of improvement of the performance for Cs2AgBiBr6 and Cs2TiBr6 halide double perovskite solar cells.
Regardless of fast improvements in both photovoltaic and light producing device execution, the comprehension of the optoelectronic properties of halide double perovskites is as yet fragmented. Specifically, the polarization of the material, the nearness of sub-atomic dipoles, and their impact on the dynamics of the photo-excitations remains an open issue to be cleared up. From now on, the imperative subject of discussion has been the seemingly perpetual polarization of the perovskite under connected electric fields [79,80]. This impact shows itself as a huge hysteresis in the deliberate current–voltage curve of perovskite sun-oriented cells and LEDs in planar hetero-junction designs, contingent upon the contact materials used [79,81,82]. The vast majority of the ongoing work underpins the speculation that particle migration [79,82,83] ought to rule any ferroelectric conduct at room temperature [84]. In addition to these polarization effects, there is another effect that causes problems in efficiency estimation [85]. The hysteresis effect transforms the dominant behavior from normal (Figure 1a−c) to be inverted (Figure 1d−f) owing to the decreasing scan rate with a stabilized current−voltage curve at an equilibrium point [86]. The estimated efficiency in the inverted behavior is different from the dominant normal behavior. Those devices showing high efficiency have lower surface recombination that can be a source of the hysteresis effect. Moreover, the presence of the hysteresis effect originates from ion migration, charge carrier trapping/detrapping, and ferroelectric polarization [87]. This hysteresis effect is not only affecting device efficiency but also device stability [87]. Owing to its negative impact on efficiency and stability, mechanisms to avoid its presence via strategies such as either working on device architecture, increasing grain sizes, interface engineering, and additive doping have been suggested [87,88].
Researchers such as Leijtens et al. [89] explored the impact of a connected outer electric field on the photograph-energized types of CH3NH3PbI3 thin movies, both at room temperature and at low temperature, by checking the photoluminescence (PL) yield and PL rots. At room temperature, these creators discovered proof for an electric field–incited decrease of radiative bimolecular bearer recombination together with the movement of charged imperfections that influence the non-radiative rot rate of the photoexcited species. This has been allotted to field-incited arrangement of the atomic dipoles, which diminishes the vibrational opportunity of the cross section and the related nearby screening, which results in a more grounded electron gap interaction [89].
Diverse degrees of tilting of the octahedral structure offer ascent to various precious crystal fields, which results in various electronic and optical properties. The degrees of tilting may influence the band structure, electron transport properties, photoluminescence, and dielectric conduct. Tilting at the organic cage causes distortion of peroveskite lattice either by tilting at PbX6 octahedra or brings isotropic lattice contractions. In the first case, it increases the band gap by reducing orbital overlap; in the second case, it reduces the band gap [90,91,92]. Understanding characteristics of inorganic octahedral tilt is an important issue to understand band structure and electron hole transport in halide perovskite materials. In this case, corner connected octahedral structure where distortion, including octahedral tilting, plays its own role. This lattice distortion brings structural flexibility where various phases are created; not only octahedral tilting but also off-centering at the A and B site of the octahedral inorganic structure, which causes phase transformation to result in high temperature cubic, tetragonal, and a low temperature orthorhombic–phases (Figure 2a). This happens owing to the occurrence of two types of octahedral rotation: in-phase and out-phase rotations (Figure 2b) [93]. The cubic aristotype structure happens at a higher temperature, but tetragonal, orthorhombic, and monoclinic phases occur at a lower temperature. This leads to lattice dynamics, resulting in phase diversity [94].
To understand the surface structure relaxation process in organic inorganic hybrid 2D perovskite, Yan Shao et al. studied how to unlock the surface octahedral tilt in Ruddlesden-Popper perovskites [95]. The result indicated that surface-enhanced octahedral tilt is correlated with excitonic redshift, which enhances inversion asymmetry normal to the direction of a quantum well and promotes Rashba spin splitting for n > 1. The surface structure relaxation is related to the exfoliated monolayer surface and bulk interface (Figure 2c) while the surface-enhanced octahedral tilt is related to a rotation of the anion cage classified as out-of-plane and in-plane tilt (Figure 2d). Furthermore, the characteristic inorganic octahedral tilt affects the optoelectronic properties such as charge carrier lifetime and band gap [96]. This long charge carrier lifetime in halide perovskite is owing to the presence of octahedral tile, related to dynamic disorder, which impacts the optoelectronic performance [97].
The key point that researchers deal with regarding materials property is to obtain promising material property for certain promising applications [20]. Thus, many researchers are working on the halide double perovskites for some applications [98] because these materials show promising solar cell absorbers [20,99], tandem solar cells [32,99,100], photocatalytics [20,27,101,102,103], and light emission devices [20]. Moreover, the applications of halide double perovskites for energy storage systems such as batteries have shown promising research progress [104,105,106,107]. In this regard, vacancies are observed to facilitate ion conductivity, surface area, and ion migration, which are vital to energy storage systems such as batteries [108]. Furthermore, MA2SnCl6 as a cathode demonstrates the highest specific capacity of 589.98 mAh·g−1 while Cs2NaBiCl6 as an anode demonstrates over 99% Coulombic efficiency with a specific capacity of about 300 mAh·g–1 [105]. Thus, Cs2NaErCl6: Li+ as the negative electrode achieves a coulomb efficiency of nearly 100% with specific capacity of 120 mAh·g–1 at 300 mA·g–1 after 500 cycles [107]. Although it is believed that halide double perovskites are suitable for energy storage systems such as batteries, research will continue to confirm whether this progress is confidential enough to use them in batteries in the practical work. Until this practical application of halide double perovskites is confirmed, this application will be debatable in the research world. Another application of halide double perovskites such as the Cs3Bi2Cl9 electrode for thin film based supercapacitor achieved a higher specific surface area (26.4 m2·g−1), areal capacitance of 64 mF·cm−2, and 6.6 μWh·cm−2 energy density much higher than the lead-based devices [109]. With this perspective, halide double perovskites have achieved many possible applications but limited research in fuel cell applications. Hence, new possible breakthroughs are expected to become great research news for the proposed fuel cell applications. Furthermore, X-ray detectors require high sensitivity and acceptable doses applied in security screening, nondestructive industrial inspection, and imaging [110]. Moreover, imaging and self-powered X-ray detection use halide double perovskites such as Cs2AgBiCl6 with a limit of detection of 241 nGy·s–1 and sensitivity of 325.78 μCGy·air–1·cm–2 [111]. Valuable characteristics such as lower trap density (1.18 × 109 cm–3), high carrier mobility-lifetime product (5.36 × 10–4 cm2·V–1), and the high dark resistivity (3.1 × 1010 Ω·cm) presented in halide double perovskites make them possible candidates for X-ray detector applications [111]. Finally, opportunities and challenges remain that are addressed elsewhere for possible application of halide double perovskite materials in X-ray detectors [112].
As a result, revolutionary advances have been claimed in lead halide perovskite photovoltaic, i.e., PCE has reached greater than 24.2% [30] for a single junction organic inorganic halide perovskite in only a few years of development [61,113,114] and 29.15% for a textured monolithic perovskite/silicon tandem solar cell [31], rendering lead halide perovskites a unique type of material for solar energy capture; however, the record for lead free all inorganic halide perovskite is 7.11%, and for both lead and tin free all inorganic halide double perovskite solar cells, the record is 2.8% [33] and 3.3% [43,115], which is far less than 24.2% for a single junction and 29.15% for a tandem solar cell. Unlike their encouraging efficiency, lead and tin based perovskite solar cells face both the toxicity of Pb metal and device instability [47,116,117], which limits the practical applications. Consequently, a great deal has been dedicated to find alternative perovskite materials such as lead and tin free halide double perovskites and lead and tin free all inorganic perovskites [118,119,120,121]. The great opportunity to use double halide perovskites is because of the following important points [122,123]: (1) more stable to moisture compared to (MA)PbI3. A 30-day exposure to 55% humidity [99] causes no decomposition in the material, whereas (MA)PbI3 is mostly converted to PbI2 [20,124]. (2) Halide double perovskites are more stable to heat compared to (MA)PbX3. Volatile organics in (MA)PbX3 perovskites leave the material at low temperatures. Although CsPbI3 is more thermally stable, it does not form a 3D perovskite under ambient conditions. In contrast, the Bi-containing perovskites can be heated to 350 ℃ without decomposition [28,125,126]. Furthermore, (3) long photoluminescence lifetimes (660 ns), which are very promising for solar-cell applications and band gap, are ideal for pairing with Si absorbers in a dual-absorber tandem solar cell [125]. On the other hand, halide double perovskites have challenges relating to their physical chemistry and chemical physics, to date, which need further investigations for more advancement. The big challenge in halide double perovskites is a larger band gap causing poor light absorption and low efficiency. This creates a big question and concern that can cause A2BⅠBⅢX6 based lead-free double perovskites solar cells to be an acceptable alternative to replace organic inorganic halide perovskite solar cells in the market in order to realize the practical applications [127,128,129]. The key challenging mechanisms during performance improvements are suggested as follows, which will be considered core ideas for advancement. These forward-looking points of discussion are expected to improve the photovoltaic performance in addition to the questions raised elsewhere [128].
Regardless of the quick development of halide double perovskite solar cells, the cell power conversion efficiency remains about 2.5%, which was achieved using Cs2AgBiBr6 [33] and 3.3% using Cs2TiBr6 [43]. Furthermore, the strategy to improve both material and device efficiency made of these materials becomes a great concern. Moreover, looking at halide double perovskite light absorbers of high-quality with high absorption coefficient, longer diffusion length, and free of defects, trap states with less radiative centers are concerning. In accordance with the Shockley-Queisser limit, a theoretical maximum PCE of 16.4% can be obtained with an E.g. of 2.2 eV, thus holding promise for working double perovskite based photovoltaics [129]. Even though the theoretical maximum PCE of Cs2AgBiBr6 does not reach the highest reported PCEs of single-junction MAPbI3-based photovoltaic cells, Cs2AgBiBr6 is a promising candidate for applications in tandem solar cells, as was already shown for MAPbBr3, which features a slightly larger E.g. of 2.3 eV [130]. Not only are the energy alignment and band gap with the charge extraction layers important, but the charge collection efficiency is vital to manufacture high-efficiency solar cells [40].
This is another key challenge in halide double perovskite solar cells that remains an unexplored area of research. Furthermore, poor interfacial integration might lead to broadening of the depletion width, poor band alignment, inefficient charge collection, enhanced recombination, insufficient charge transport, and poor overall device performance. For instance, such mismatch in energy levels has occurred in materials such as (MA)2AgBiI6 and Cs2PdBr6. For example, (MA)2AgBiI6 was observed as not compatible with TiO2 but was well-matched with SnO2 and C60 [131].
Whether or not they are as favorable as lead-based perovskites is not well known. The optoelectronic properties of Cs2AgBiBr6 and Cs2AgBi1−xSbxBr6 have displayed a comparable rate-transport mechanism, which can act as an alternative absorber to lead-based perovskites [15]. The charge carrier density of both Cs2AgBiBr6 and Cs2AgBi1−xSbxBr6 is limited to 1016 cm−3 and a mobility of 0.8 cm2/Vs as well as tunable band gap of 2.0 to 1.6 eV [132,133]. Thus, this charge transport property requires greater attention for further improvement of the field. The carrier mobility of Cs2AgBiBr6 is 0.3–11 cm2/Vs with electron and hole mobility of 17 and 14 cm2/Vs, respectively [134]. Other charge transport properties of Cs2AgBi1−xSbxBr6 have not been reported. Thus, further study is required to clarify and fill the limitation not yet reported.
The quantum confinement effect in the last shells due to restricted orbital interactions of the adjacent cations, particularly B′ and B″ cations in the cubic unit cell of the AB′B″X6 double halide perovskite materials, might result in a narrow and localized conduction band edge as well as a broad energy gap, which deteriorate the device performance [27,127]. Consequently, finding a way to move into another unite cell structure is expected to overcome such challenges.
Halide perovskites have both low and higher dimensionality. Such dimensions have their own electronic and optical properties that impact the efficiency and stability of halide perovskites. This structural dimension at the molecular level ranges from 0D to 3D (Figure 3, Table 1), where D stands for the word 'dimension'. High dimensionality, i.e., 3D lead halide perovskites, achieved high performance. The majority of these materials demonstrated 3D dimensionality [28]. On the other hand, halide double perovskites showed broad band gaps, which means structural dimensionality is a factor affecting PCE in addition to the electronic band gap that is affected by the electronic dimensionality. This might make halide double perovskites inappropriate for a single junction solar cell function though these materials crystallize into 3D [119]. Furthermore, the most explored halide perovskite are 2D and 3D, while those least explored are 0D and 1D. The reason why they are unexplored lower dimensional halide perovskites is because of site isolation and strong confinement [135].
Properties of low and high dimensional structures | |||
0D [136] | 1D [137,138] | 2D [139,140,141] | 3D [142,143,144] |
● Individual metal halide octahedral anions or metal halide clusters are completely surrounded and isolated by the organic cations. ● Molecular perovskite units are embedded in the crystal lattice. ● General chemical formula is A4BX6, where a represents monovalent organic cations and BX6 represents metal halide octahedra. ● Weaken the light absorption range and hinder charge transport. |
● The metal halide octahedral are corner-sharing, edge-sharing, or face-sharing to form a 1D nanowire. ● Either linear or zigzag configurations. ● Chemical formulas are variable depending on the connecting methods and the organic cations chosen. ● Weaken the light absorption range and hinder charge transport. ● High stability of low dimensional perovskites. |
● High stability of low dimensional perovskites. ● Suppress moisture invasion. ● Prevent moisture intrusion. ● Improve thermal stability. ● Suppress ion migration. ● The links between octahedra [BX6]4−are separated by large organic cations along crystallographic planes. ● The chemical formula is generally described as (A')m(A)n−1BnX3n+1. ● The 2D perovskite is more stable than its 3D phases. ● 2D perovskite-based PSCs do not exhibit ideal performance due to their larger bandgap, low carrier mobility and weaker carrier transport resulting from insulating spacer cations. |
● Superior efficiency of 3D perovskites. ● Consists of a network of corner-sharing BX6, where the B atom is a divalent metal cation (typically Ge2+, Sn2+ or Pb2+) and X is a monovalent anion (typically Cl−, Br−, I−). ● Sensitive to moisture invasion. ● Does not prevent moisture intrusion. ● The chemical formula is generally described as ABX3. ● The 3D perovskite is more unstable than its 2D phases. ● 3D perovskite-based PSCs exhibit ideal performance due to their narrow band gap, high carrier mobility and stronger carrier transport resulting from insulating spacer cations. |
The higher performance of organic, inorganic halide perovskites was achieved due to their direct and lower band gap, but halide double perovskites possess indirect and wide band gaps which limit the absorption of light for solar cells and is not ideal for thin film PV applications cases, and this field needs band gap engineering. For instance, in recent times, bismuth and silver based perovskites were anticipated as a choice to replace MAPbI3 [51,125]. The band gaps are indirect, which is not ideal for single junction solar cell applications, though bismuth/silver based double perovskites absorb 1.9 to 2.2 eV [145]. The reason that indirect semiconductors are not preferable compared to direct semiconductors is due to the weak oscillator strengths for radiative recombination and for optical absorption, which might be a challenge if there is low carrier mobility. Similarly, Cs2AgBiBr6 has a 1.95−2.19 eV indirect band gap [146,147], showing a comparable lifetime with optimized lead halide perovskite films [148,149]. Solving the issue of non-radiative recombinations from its indirect band gap makes them promising for photovoltaic application. The charge mobility in the polycrystalline Cs2AgBiBr6 is 1 cm2·V−1s−1 [135], while in the single crystal it is 11.81 cm2·V−1s−1, indicating that charge transport property is superior in single crystals.
The energy state of a given semiconductor is described via energy disorder since it impacts charge transport, carrier mobility, and recombination rate [117,150]. Such disorder may be dynamic (caused by carrier phonon interactions) and/or static (caused by impurities or defects) disorder, which influence inter-band structure as shown in Figure 4 [151]. In organic solar cells, energetic disorder is caused by the synergy of intra- and intermolecular interactions that originated from intermolecular disorder, the unfavorable molecular packing behavior [152]. In mixed halide perovskites, the origin of the disorder would be compositional disorder causing inhomogeneity of halide ions [153,154]. The great debate here is: which type of energy disorder matters for carrier recombination dynamics, charge transport, band-tail structure, carrier mobility, carrier trapping, and ultimately device performance? Thus, understanding energetic disorder in halide double perovskites becomes necessary of the time scales connected with atomic and molecular motions as well as the variation of the disorder from a single crystal to the thin film [155]. Knowing the variations might also be vital during the processing of these light-absorbing materials and hence strongly correlated with the device performance.
There are variations reflecting the coordination and local chemistry. As these uncertainties transfer to the octahedral and tolerance factors, the stability ranges proposed so far are descriptive rather than predictive. There are some geometric factors restricting the formation of stable 3D halide double perovskites. Many promising routes towards Pb-free halide double perovskites have been explored to replace Pb by elements such as germanium [74], bismuth, or antimony and noble metals [126,131]. However, the geometrical constraints imposed by the necessity of forming an ideal perovskite lattice, in addition to the common oxidation states of these optional cations (e.g., +3 of Bi and Sb), still cause this to be a difficult discovery of lead-free materials that could rival the optoelectronic properties of prototypes such as MAPbI3.
While organic inorganic halide perovskites need annealing temperatures usually less than 100 ℃, the halide double perovskites are fabricated only at a higher annealing temperature up to 285 ℃ [40,156]. Such prerequisites for fabrication might damage the integration of the device architecture and further restrict wide range function of these materials. Additionally, such high energy consumption for materials fabrication is not economically cost-effective, environmentally none green, and is not sustainable. As a result, looking for alternative methods that could minimize such higher processing temperature becomes an impressive research area in this field.
In spite of considerable contributions, there is no well-refined guideline and theoretical understanding for what kind of mixed B-site cation halide double perovskites should be used and how to design Pb and Sn free halide double perovskites for enhancing collective optical, electrical, thermal, and mechanical properties and long term stabilities of these materials for wide range applications. In order to minimize such bottleneck challenges, detailed knowledge of the electronic structures of these materials with respect to B'/B'' cation arrangement and design become attractive research directions in the field of halide double perovskites.
Stoichiometric design of the perovskite [156] has been known as a strategy to develop solar cells with enhanced performance. Moreover, unique stoichiometric design and compatibility of various interfacial device layers are essential aspects that require more attention [19,26,157].
Elucidating the arrangement and position of the ions is another concern in halide double perovskites. Because the B cations generally determine the physical properties of double perovskites, there are three B-cation sublattice types known for double perovskites: random, rock salt, and layered. The latter two are ordered arrangements. In some situations, it is observed that the order is not complete, especially in the A'A''B'B''X6 structure. Interestingly, order-disorder effects are mainly encountered when the charge variation between B' and B" is two or less. Although the degree of order is an important subject, the dynamic nature of order-disorder behavior is a quite useful parameter [11,46,158,159].
Unlike organic inorganic halide perovskites, achieving high quality film in halide double perovskites becomes a challenge. High quality film might be defined as a material with excellent crystallinity, uniform morphology, less defects, high absorption coefficient, superior ambipolar carrier transport ability [160], and high coverage or pinhole-free film [161,162]. All these parameters are well known to deter both materials and device performance in halide double perovskite solar cells if the quality of these materials is not well optimized during processing steps. For that reason, the light harvesting and active layer that is the halide double perovskite monolayer in the perovskite solar cells is one of the very important research topics that researchers deal with. Moreover, controlling and designing the colloidal properties of the precursor and solvent engineering [60,163,164] should get considerable attention to enhance the required film with high quality [165,166,167].
In order to overcome the key challenging mechanisms faced by halide double perovskite solar cells, suggesting key areas of research that will be considered as research roadmap for improvement of both material and device performance is quite important. These include engineering microstructure, surface and bulk properties, grain boundary and domain wall engineering, polar order engineering, band gap and band structure engineering, colloidal engineering, composition engineering, optoelectronic engineering, device architectural engineering, interface and defect engineering, equilibrium and non-equilibrium quantum transport, and ferroelectric engineering, as shown in Figure 5.
Engineering microstructure, surface, and bulk properties of halide double perovskites are important mechanisms and research areas for improvement for both materials and devices [20,50,124,168,169]. Microstructure influences virtually all aspects of the behavior of materials. Moreover, it is the spatial distribution of material in the useful object that is the goal of the endeavor and is the most richly variable; thus, this is the most susceptible to control of the elements in the materials structural hierarchy. Engineering microstructure of materials [168,170] comprises the concepts of morphology or topological properties and geometry, thermodynamic properties, surface and bulk properties, and grain growth as well as bulk and nano-scale domain structures.
The halide double perovskite microstructure might offer optional lanes for conductivity and electron−hole disjointing. Because grain boundaries have an impact on the long-term stability along with the ferroelastic domain boundaries that might be different from regular grain boundaries, the finding of ferroelasticity offers a novel parameter to pursue in favor of enhancing their stability as well as facilitating their extensive implementation [171]. Additionally, grain engineering and morphology control through the introduction of additives to the perovskite precursor is another way for performance improvement [31]. Sub-micron-sized crystal grains in perovskite thin films, on the other hand, have been obtaining considerable attention due to their fundamental morphological properties in the course of twin domains [172,173], which are highly essential for understanding the basic mechanisms, for instance, of low recombination rate and long diffusion length of the device's high performance [174]. Moreover, the presence of charged domain walls will significantly reduce the band gap by 20%−40% and can serve as segregated channels for the motions of charge carriers, while the presence of uncharged domain walls has no substantial impact on the band gap [175].
Effect of polarization, molecular dipoles, tilting of octahedron and transition metal cation size, and its oxidation states on photo-excitation, band structure, and photoluminescence are not well investigated. Notwithstanding the microscopic model, polar order is accepted to recline at the center of the ferroelectric photovoltaic impact [176]. Besides, it is outstanding that interactions between lattice, orbital, and polarization order parameters in ferroelectric materials initiate upgrades of their physical properties close to phase boundaries [177]. On the road to advanced performance, conventional approaches focus on reducing the band gap to superior fit the solar spectrum, while reducing the basic relation between polar order and photovoltaic effect is ignored [177]. Hence, spotlighting both narrowing the band gap and basic relation between polar order and photovoltaic effect has become an attractive research topic.
The fundamental bottleneck restraining the function of halide double perovskites is their bigger energy gap. The most favorable band gap for the single junction solar cells ought to be close to 1.3 eV [178]. As a result, band gap and band structure engineering are vital if halide double perovskites are to be suggested optional contender materials as shown in Figure 6. On the other hand, Cs2Ag0.95Ga0.05BiBr6 based double halide peroveskite solar cells show a small performance improvement of 4.52% via band gap reduction [179]. If the suggested engineering strategies are implemented, it is promising to maximize the power conversion efficiency of these solar materials.
As shown in Figure 6, the band gap and band structure of various Cs2AgBiBr6 derivatives are shown as indirect band gap semiconductor materials, substituting Bi using Sb and In elements. Bi 6p orbitals are the conduction band minimum for Cs2AgBiBr6 (Figure 6a), while the Bi 6s orbitals construct their valence band maximum with a calculated indirect band gap of 2.0 eV. Furthermore, a valence band maximum is constructed by the Sb 5s orbital observed above the Bi 6s orbitals for Cs2AgBi0.75Sb0.25Br6 (Figure 6b) whiles its conduction band minimum is constructed from the Sb 5p orbital. The 1.58 eV indirect band gap is obtained from this calculation. The conduction band minimum for Cs2AgSbBr6 is derived from Sb 5p orbitals, but its valence band maximum is derived from Sb 5s orbitals (Figure 6c) with a calculated band gap of 1.67 eV. Further substitution with In metal results in a new band structure, introducing 4s orbital in the lower conduction band, but Bi 6s orbitals drives the valence band maximum with an indirect band gap structure. Thus, a larger band gap of 2.28 eV has been calculated for Cs2AgBi0.25In0.75Br6 (Figure 6d, e), which is larger than that of Cs2AgBiBr6. Increasing In concentration leads to strong coupling in the [InBr6] octahedral, resulting in a reduced gap caused by the In 5s-derived conduction band. These band structures obtained by Sb and In atomic substitution suggest that every atom introduced has its own band structure and unique optoelectronic properties with unique optical and electrical performance. Thus, understanding band structure matters for optoelectronic properties and the application of a given semiconductor.
The nucleation and growth, coordination chemistry, and coordination engineering of halide double perovskites need to be studied: In addition to the photophysics properties, the nucleation and growth, coordination chemistry, and coordination engineering concepts are equally important and quite essential for developing new halide double perovskite materials [181]. Therefore, colloidal engineering, colloidal chemistry, and ion exchange reactions during synthesis of halide double perovskites are important ideas that need to be controlled for synthesizing high quality thin film for high performance.
The computational details on the structure of Cs2AgBiBr6 double perovskites were reported recently, as shown in Figure 7a, b [182]. This structure is taken as the starting material for its derivatives such as Cs2AgSb0.5Bi0.5Br6, Cs2Ag(Bi1−xTlx)Br6, etc. From these reports, Cs2AgBiBr6 crystalizes as a face-centered cubic structure with a space group of Fm3m, as shown in Figure 7a. The atomic positions of the Ag atom is at (4a) (0, 0, 0), Cs atom is at (8c) (0.25, 0.25, 0.25), Br is at (24e) (ux, 0, 0), and Bi atom is at (4b) (0.5, 0.5, 0.5). Its tetragonal structure (Figure 6b) crystalizes at low temperatures with the atomic position of Br2 atom at (4e) (0, 0, uz), Br1 atom at (8 h) (ux, uy, 0), Ag atom at (2b) (0, 0, 0.5), Bi atom at (2a) (0, 0, 0), and Cs atom at (4d) (0.5, 0, 0.25) [182]. Furthermore, the band structure of Cs2AgBiBr6 with an indirect band gap character is shown in Figure 7c, d. The band edges are mostly formulated from Br, Bi, and Ag states. The Bi–p states contribute to the conduction band while Ag–d and Br–p hybridized states contribute to the formation of a valence band. The band edges are made of Br, Bi, and Ag states. Bi–p states contribute to the formation of conduction bands while Br–p and Ag–d hybridized states contribute to the formation of valence bands. The possible electron transition occurs in Ag–s and Bi–p antibonding states from Br–3p and Ag–4d bonding. Thus, the optoelectronic properties of Cs2AgBiBr6 are influenced by Ag, Bi, and Br atoms. Furthermore, all possible compositional displacements and addition of atoms at a given ratio can take place at these atoms for possible modifications of the electrical and optical properties as well as other applications.
Cation and anion displacement, cation-anion order-disorder effects, cation size mismatch, distortion, tilting, ordered vacancies, hydrogen bonding, charge disproportionation, and stereochemistry or lone pair effects need to get more attention in order to improve stability, inferior material, device performance, and engineering a new halide double perovskite material [13,119,152,153,154]. In addition, the creation of new chemical compositions using new elements in the mixed halide double perovskite structure is an acceptable strategy to tune the band gap and other optoelectronic properties [183]. Currently, Cs2AgBiBr6 and Cs2TiBr6-based lead and tin-free all-inorganic halide double perovskites are reported as alternative types of perovskite solar cells. The latter has an energy gap of 1.8 eV in addition to its > 100 nm carrier diffusion length [43]. The composition engineering due to modifications at the halide anion from Br to I results in the reduction in band gap ranging from 1.8 to 1.0 eV [37]. This band gap is suitable for single junction halide double perovskite solar cells [37]. Furthermore, modifications at the cation site, A-site, and B site are not confirmed. Similarly, Cs2AgBiBr6 has a high band gap, tunging from 1.83 to 2.21 eV [125,40,184]. Composition engineering at the A, B, and X sites may reduce the band gap to make it more suitable for single-junction halide double perovskite solar cells [185]. Recently, precursor engineering has been suggested as a route to enhance the power conversion efficiency of Cs2AgBiBr6 materials, showing 40% enhancement [186]. This result indicates that precursor engineering is one type of composition engineering that is responsible for obtaining smooth, large grains, and high-coverage Cs2AgBiBr6 films.
The indirect band gap characteristics of the inorganic double perovskite band structure have been reported as shown in Figure 8 [77]. In this report, pure Sb(Cs2AgSbBr6, Cs2AgSb0.5Bi0.5Br6) and pure Bi(Cs2AgBiBr6) double perovskites have been studied (Figure 8). Electronic band structures were computed employing the TB09 method, considering both the inclusion of spin–orbit coupling (depicted by the black lines) and its exclusion (represented by the red lines). Materials under investigation include pure Sb alloy Cs2AgSbBr6 (Figure 8a), mixed alloy Cs2AgSb0.5Bi0.5Br6 (Figure 8b), and pure Bi alloy Cs2AgBiBr6 (Figure 8c). The valence band maximum (VBM) of these three compounds is situated at the X point, whereas the conduction band minimum is positioned at the L point in the reciprocal space. This observation signifies the indirect band gap nature of these compounds. Band gaps obtained from calculation inclusion with SOC are lower than that of exclusion with SOC. This results from SOC leads to band degeneracy resulting in a reduction of the band gap. Band gaps calculated with the inclusion of SOC are lower than those obtained without SOC. This discrepancy arises because SOC introduces band degeneracy, consequently decreasing the band gap in these systems. Indirect band gaps calculated by TB09-PBE (excluded SOC) of pure Cs2AgSbBr6, mixed Cs2AgSb0.5Bi0.5Br6, and pure Cs2AgBiBr6 are 1.69, 1.96, and 2.17 eV, respectively.
The indirect band gaps of 1.69, 1.96, and 2.17 eV are reported for pure Cs2AgSbBr6, mixed Cs2AgSb0.5Bi0.5Br6, and pure Cs2AgBiBr6, respectively [77]. The conduction band of these materials is dominated by the Ag–d orbitals while the valence band is dominated by Sb–p orbitals in pure Cs2AgSbBr6 and Cs2AgSb0.5Bi0.5Br6 but Br–p orbitals in pure Cs2AgBiBr6 [77]. Hence, it is possible to investigate photoactive materials for various applications as a function of compositional engineering in the field of lead-free halide perovskite materials.
Ferroelectrics, dielectrics, piezoelectric, pyroelectrics, electro- and photocatalytic properties, thermoelectric properties, electrical transport properties, and other optoelectric properties have not been studied [11,20,171,187,188]. Optical transition in identical inversion symmetry is symmetry forbidden, leading to poor optical absorption, as shown in Figure 9a [189]. For instance, in Cs2AgTlCl6 the band gap transition at contains the A1g orbital in the CB and Eg orbital in the VB (Figure 9b) owing to grade symmetry, which results in forbidden band gaps. Furthermore, the optical transition from
to L progressively becomes allowed (Figure 9c). Such isoelectronic properties are observed in Cs2AgInCl6, where Figure 9d shows the formation of a new conduction band owing to increasing Tl3+ concentration in Cs2Ag(Bi1−xTlx)Br6. Introducing Tl3+ reduces the band gap of Cs2Ag(Bi1−xTlx)Br6 since the Cs2AgBiBr6 band includes Tl3+ 6s orbitals (Figure 9d) [189]. From this perspective, the band structure depends on the composition of metal atoms incorporated in the structure of the semiconductor.
Moreover, the microscopic physics related properties that mater the photovoltaic effect are vital properties in the field of photovoltaics. This property becomes inadequately known and tarnished when we discover the double perovskite solar cells. Furthermore, diverse methods have been suggested to explain research outputs in a variety of device architectures, such as asymmetric momentum distribution of the non-equilibrium carriers [190,191], shift in current through coherent evolution [192,193,194], polarization-dependent interfacial band bending [195,196], and bulk depolarization field [195].
From a material properties and applications perspective, understanding and extracting information on the presence or absence of ferroelectrics in halide perovskite materials will greatly contribute to multifunctionalities in the field of electronics beyond photovoltaic; additionally, symmetry and structure of the crystalline unit cell determines the ferroelectricity of materials [197]. For ferroelectricity to be detected, the primary requirement is the presence of a non-centrosymmetric unit cell. According to the definition of Anderson and Blount [198], a ferroelectric material has to encompass several criteria: (1) a continuous structural transition; (2) a non-centrosymmetric low temperature structure; and (3) a structure with a unique polar axis (a rotational axis of symmetry without a mirror plane perpendicular to it) at low-temperatures [199,200]. All these properties clarify the origin of ferroelectricity [201]. Whether or not a material has such a polar axis is decided exclusively by its crystal structure and creates the delicate variation between pyro- and piezoelectric. LiOsO3, whose existence was first predicted half a century ago [202] has been identified [203] as an example of a ferroelectric metal, and has been established. It is likely that materials in this class could show some attractive physical properties. For instance, at enhanced temperatures, the existence of ferroelectric-like soft phonons can stabilize non-centrosymmetric superconductivity [204].
In addition to this, there are essential situations for bulk ferroelectricity to be present [205]: (1) noncentrosymmetric properties without inversion symmetry; (2) characteristic polarity; (3) an assembly of polar unit cells facing a similar direction that can make a "polar domain"; and (4) polarization switching. In addition to the electrical responses, ferroelectricity can be structurally explained using periodically ordered polar domains. Like the other ferroelectric materials, the relationship between the crystal thickness, D, and domain periodicity, ω, obey the Landau–Lifshitz–Kittle model scaling relationship as ω ~D0.6 for MAPbI3 perovskites. Due to differences in doping, the crystal synthesis could affect the ω–D relation. Derived from the periodic structure and etching, the domains appear to develop a head-to-head orientation.
Based on these facts, ferroelectricity in halide perovskites remains elusive. Inorganic materials with ferroelectric properties like BiFeO3 have revealed a huge possibility in many other functionalities beyond photovoltaic [206]. At this time, application in optoelectronic devices and the related semiconducting properties of stannate or hybrid organo-plumbate become burning issues. With respect to their structural properties, these materials are appropriate for investigation regarding ferroelectricity [207]. As a result, the architecture of molecular ferroelectric semiconductors based on these materials demonstrates an opportunity to get novel or high-performance semiconductor ferroelectrics. In this section, we will address ferroelectric properties in both halide perovskite and fully inorganic perovskites. Until now, since this is a greatly arguable matter, additional proof for ferroelectricity of the material has to be present and is, therefore, shown. With the application of steady-state conditions, very important points become energetic as follows [205,208]:
(a) Buildup of unlike charged species at the outer surface of the polar bulk (e.g., adsorption of free charges or charged molecules from the adjacent electrodes or environment, respectively). This event, known as "pyroelectricity", is straight confirmation for accessible polarity. Previously, a clear experimental proof demonstrated that at room temperature (RT), cubic MAPbBr3 is not pyroelectric [209]. In addition, it has been evidenced practically that tetragonal MAPbI3, is pyroelectric [205].
(b) Buildup of mobile holes and electrons from the polar bulk material can happen if the bulk materials is adequately conductive, beyond ~10−6 S·cm−1, a sensible value for a low bandgap semiconductor, like that of MAPbI3. Thus, leakage currents ought to govern the dielectric response and are influenced by the bulk overall polarization [205].
(c) Stacking of domains of contrary polarity to reduce Coulomb repulsion, except at the price of creating a "domain-wall". For several famous ferroelectric materials, the periodicity between the domains, ω, scales with the crystal thickness the length of its polar axis, D, as ω ~ Dγ, where γ is the power reliance that is hypothetically equivalent to 0.5 [210,211]. The tetragonal MAPbI3 follows this rule [205].
(d) Accordingly, the quarrel on ferroelectricity in MAPbI3 has not been concluded with clear termination, which inspires us to review ferroelectric behavior of various perovskite materials such as MAPbI3, FAPbX3, CsSnI3, RbSnI3, and layered and double perovskites in the next subsections.
Domains created by the sharing of spontaneous polarization in space are important to characterize ferroelectric materials. These domains, in a given ferroelectric material, are illustrated in Figure 10. Arrows point out the trend of net polarization in domains and the constituent of the polarization vector. The constituent of the polarization at a 90° angle to the domain boundary leads to a bound charge at the boundary, as shown in Eq 1 [212,213]:
ρpol=∇P | (1) |
where, ρpol is the bound charge density. This bound charge gives rise to a depolarizing electric field in a reverse direction to the spontaneous polarization. The existence of strongly-ordered polarized domains in the perovskite gives rise to the formation of channels for minimizing charge recombinations and efficient charge transports in the device, which result in a high short-circuit current (JSC) and fill factor (FF). This important domain ordering is, nevertheless, doubtful to take place in actual devices. In the case of arbitrarily interrelated polarization in domains, a realistic scenario, it has been illustrated that holes and electrons emerge to pursue pathways or channels in the perovskite, leading to lower charge recombinations and efficient charge transports in the photovoltaic cell as proved by high FFs [213].
The Pi (C·m−2) polarization is produced in dielectric and insulating materials with the application of the electric field vector Ei (V·m−1) (Eq 2):
Pi=χijEj | (2) |
where χij (F·m−1) is the 2nd-rank tensor identified as the material's dielectric susceptibility. Eq 2 is valid only in a linear limit for nonlinear materials or for linear materials. Generally, the polarization, Pi, depends on higher-order terms of the field. Similarly, the total surface charge density induced in the material by applying the applied field is obtained using the dielectric displacement vector, Di (C·m−2), shown in Eq 3:
Di=ε0EiCPi | (3) |
Scalar ε0 = 8.854 × 10−12 F·m−1 and is called the dielectric permittivity of a vacuum. Thus, Eq 4 is obtained from Eqs 2 and 3, that:
Di=ε0Ei+χijEj=ε0δijEj+χijEj=(ε0δij+χij)Ej=εijEj | (4) |
where δij is Kronecker's symbol and εij = ε0δij + χij is the material's dielectric permittivity. δij = 0, for i ≠ j; δij = 1 for i = j. In most ferroelectric materials, εij ≠ χij and ε0δij ≪ χij. In fact, the comparative dielectric permittivity, kij = εij/ε0, identified as a material dielectric constant is usually taken compared to the dielectric permittivity. Taking free energy opinion, it can simply be illustrated that χij (also εij and kij) have to be a symmetrical tensor (χij = χji) with only six autonomous mechanisms [214,215,216].
Like ferroelectrics, it would of great interest to deal with and understand piezoelectric properties and applications of halide perovskite materials. The word piezoelectrics is used to explain attractive properties of technological important classes of materials with a diversity of functionalities, ranging from ultrasound transducers, fuel injectors, and waveguide devices to gyroscopes and accelerometers [217,218,219]. It is of high technological interest to explore the piezoelectric properties of the hybrid perovskites for applications such as piezoelectric generators or energy harvesting devices [220,221]. In this section, origin of piezoelectrics, the sunrise of piezoelectrics, methods to enhance hybrid perovskite piezoelectrics, possibility of constructing dampers, and low loss piezoelectrics are issues that should not be missed while studying piezoelectrics. Other important points are also discussed.
The piezoelectric effect consists of strain development under the application of an electric field. Moreover, piezoelectricity is a basic procedure that includes multiple piezoelectric equations and diverse electromechanical effects. The direct piezoelectric effect relates to the produced polarization that builds up in i direction (ΔPi) with an applied stress in direction j (σj in Voigt notation) as in Eq 5 [222]:
ΔPi=dijσj | (5) |
where, dij is 3rd-rank tensor, and every dij is typically called a piezoelectric coefficient or direct piezoelectric strain coefficient in units of pC/N. An additional piezoelectric equation between the polarization with the strain η is specified by Eq 6 [222]:
ΔPi=eijηj | (6) |
where the eij is the piezoelectric stress coefficient in the unit of C/m2. It is indicated that the dij and eij parameters are associated with each other using stiffness and/or elastic compliances, though dij is simple to determine experimentally. The total generated polarization articulated in the c direction is given by Eq 7 [222]:
ΔP3=e33η3+e31(η1+η2) | (7) |
where, Eqs 8 and 9 are obtained:
η1=(x−x0)x0 | (8) |
η2=(y−y0)y0 |
η3=(z−z0)z0 | (9) |
are strains along the x, y, and z axis, with x0, y0, and z0 as lattice constants for a structure not strained.
In order to disclose the source of piezoelectricity [223], it is important to split the total polarization originated from contributions of the (1) A-site MA cations and (2) B-site Pb atoms. The Pb atom induced polarization is measured using D(Pb)/Vu × PPb = Z33 [222], where Z33 is the Born effective charge of Pb, D(Pb) is the average displacement of Pb alongside the c axis with respect to the center of its I6 cage, and Vu is the volume of the primitive unit cell. Thus, +4.24 is the measured value from the contribution of Z33, which is considerably greater than the supposed charge of Pb (+2.0) in a pure ionic image [222] that implies the presence of a dynamic charge transfer coupled with the change of Pb–I bond length and the strong covalence of the Pb–I bonds. It is obvious that both molecular dipoles and Pb displacements are in charge of the total polarization in which Pb atoms contributes nearly all the piezoelectric response with negligible contribution from the MA+ molecules.
In perovskites with unusual atomic substitutions in the ABX3 architecture, the competition between B–X metal-halide bond and A–X hydrogen bond monitors the piezoelectric characteristics, drawing attention to the perspective of a halide perovskite design for manipulative and useful photopiezoelectrics and photoferroelectrics [222], such as applications in sensors, actuators, and energy harvesting [219,224,225,226]. Because of the pairing of semiconducting properties with its piezoelectrics, micro- and nanowires of piezoelectric semiconductors have been utilized as fundamental building blocks for developing ground-breaking devices [227], including piezo-phototronic devices [228,229], piezoelectric diodes [230], piezoelectric field-effect transistors [231], piezoelectric chemical sensors [232], and nanogenerators [233,234,235].
Thus, halide perovskites possess a cubic crystal structure that gives rise to ferroelectric polarizations similar to other classes of perovskites with identical structural characters [180,236,237,238,239]. Coll et al. have demonstrated the polarization switching and light-enhanced piezoelectricity of CH3NH3PbI3 materials [240]. Furthermore, an output piezoelectric of 2.7 V and 140 nA·cm−2 have been reported [241], hindering the practical functionalities due to its modest output performance. Moreover, the highest piezoelectric current density and output voltage of 3.8 μA·cm−2 and 8.5 V have been demonstrated from the piezoelectric nanogenerator under periodically vertical compression. Multilayered halide perovskites even have large polarization or strong piezoelectricity ≈1540 p·CN−1 [242]. This output is quite promising compared to other literature reports [243]. This could help charge LED and capacitors through a bridge rectifier.
In the field of halide double perovskites, the interfacial properties, especially those between the active perovskite layer and hole transporting layers, are not well touched. Recently, using Cu2O as hole transporting material, a power conversion efficiency of 11.32% Cs2AgBiBr6 based solar cell was achieved, as indicated by the numerical simulations [244]. This indicates that the hole transport layer with a suitable alignment to the active layer makes an efficient charge transport process that can improve the challenges faced by Cs2TiBr6 and Cs2AgBiBr6. Furthermore, the grain boundary interfaces present in the active perovskite layer in addition to the other grain boundary interfaces present between various layers is a vital research lesson for improving the PEC and the performance. This is because considerable detrimental trap states in the electronic state of the valence and conduction band states of the halide double perovskite semiconductors might exist, which increases the carrier recombination rate and reduces the open circuit voltage significantly. Therefore, the engineering of the grain boundaries to eliminate the detrimental trap states in polycrystalline thin films becomes a long-standing and important issue for high performance optoelectronic devices [245,246,247,248,249]. In addition to the detrimental trap states, understanding the defect properties of the surface and bulk perovskite films, grain boundary, and surface passivation mechanisms, defect properties of the interface during engineering the device architecture is quite a crucial aspect that needs serious interface and defect engineering [245,246].
Moreover, Cs2TiBr6 is reported as a sensitive material to defect density [44,250,251] though it demonstrates a power conversion efficiency of 14.8% [252]. If all possible solar parameters are optimized, this solar material is a promising candidate for the future of peroveskite solar cells. Moreover, the numerical approach shows that a power conversion efficiency of 24.49% can be achieved by Cs2PtI6-based Cs2TiBr6 perovskite solar cells while Cs2TiBr6 is just 9.81% if it is well optimized [253]. Furthermore, the poor device performance of Cs2TiBr6 based peroveskite solar cells is because of the bulk defect present within the Cs2TiBr6 active light absorbing layer. Hence, defect passivation approaches are expected to solve defect related challenges in these materials. Thus, based on this defect optimization method, 9.9% efficiency has been achieved for Cs2TiBr6-based peroveskite solar cells [254]. Defects are also important to reconstruct band edges as shown in Figure 11. Such defects are intentionally incorporated defects useful to create new semiconducting materials [245]. The dominant metal orbital character of the bands is shown in color. Arrows show the direct (Figure 11a, b, (MA)2TlBiBr6) and indirect (Figure 11c, Cs2AgBiBr6) band gap transitions. In the primitive unit cell of 1 the VBM appears at X, however, in the conventional setting shown in Figure 11 the VBM is at Γ owing to band folding.
Engineering the light harvesting active halide double peroveskite layer and interface between the active layer and different charge transporting layers as well as between the transporting layers and the charge collecting electrodes are indispensable prerequisites for appreciable performance. However, engineering the materials and the interface among various layers is not adequate. This is because the closing stages are eventually paying attention to the device performance for a specific application. As a result, engineering appropriate device architecture [255,256,257] is mandatory.
Halide double perovskites become less suitable solutions to replace the unstable organic-inorganic halide perovskite solar cells. This is due to their indirect or direct as well as larger band gaps [18,40,147]. Therefore, doping engineering [18,200] is another strategy to achieve a band gap, which is suitable for light harvesting applications [23,185,258,259,260] This along with a chemical substitution approach might be done in all lattice sites of the double perovskite (A2(B+, B3+)X6) available for substitution [23,185,258]. In this case, the degrees of freedom, with over 34 choices of trivalent metals available for selection, are mostly provided from the B3+ lattice site [259,260,261].
These transport properties and the effect of the spin-orbit on these properties is unclear: Studies on these properties could shed light to provide insightful understanding on why inferior device performances of halide double perovskites solar cells are happening.
A2BⅠBⅢX6 based lead-free halide double perovskite solar cells have been reported as promising nontoxic and stable candidates to replace organic-inorganic halide perovskite solar cells. Thus, such lead-free halide double perovskites have a (1) diversity of various families, (2) diversity of structural, optical and electrical properties, and (3) wide range of energy applications. Furthermore, the key origins of these diversities and variations are: cation displacement, cation anion ordering, ordered vacancies, tuneability of structural dimension, octahedral distortions, and tilting from the ideal structure. In addition, polarization, molecular dipoles, and tilting on the band structure are key origins of optoelectronic properties in halide double perovskites. Although lead-free halide double perovskite solar cells have promising opportunities such as a relatively longer photoluminescence lifetime and higher stability towards moisture, temperature, and light compared to lead halide perovskites, these solar cell devices have bottleneck challenges at material, interface, and device levels. Such challenges include: (a) inferior material and device power conversion efficiency, which is 2.5% using Cs2AgBiBr6 and 3.3% using Cs2TiBr6; (b) limited integration with interfacial materials; (c) quantum confinement effect of interacting orbitals of adjacent cations, which might result in broader band gaps and localized conduction bands; (d) high processing temperature, which may limit the diverse applications; and (e) low electronic dimensionality, making them less appropriate for single junction solar cell functions. Thus, it is suggested that material surfaces and bulk engineering, device architectural engineering, interfacial engineering, composition engineering, band gap engineering, doping engineering, polar and domain order engineering, etc., may be possible mechanisms to overcome the challenges. In addition, promising candidate characteristics, for instance, long charge carrier diffusion lengths, defect tolerance, low exciton binding energies, small carrier effective masses, intrinsic thermodynamic stability, direct band gaps, high absorption coefficient, and compatibility with low-cost and solution-supported fabrication procedures are highlighted for further considerations. This review will serve as a research roadmap for performance improvement for the lead and tin free halide double perovskite solar cell scientific community, research institutions, and commercial enterprises. Moreover, the challenge in most solar cells is the issue of sustainability.
With this in mind, the sustainability issue of A2BⅠBⅢX6 such as Cs2AgBiBr6 and Cs2TiBr6 perovskite solar cells shall be analyzed not only in terms of the balance among environment, equity, economy, affordability, energy independence, availability of desirable materials, and conditions over the long term but also the quality of being able to continue over a period of time. Thus, the researchers have to work in a way that they can confirm the sustainability issue of A2BⅠBⅢX6 based perovskite solar cells.
The authors declare they have not used Artificial Intelligence (AI) tools in the creation of this article.
The first author, Etsana Kiros Ashebir, is responsible for manuscript writing, literature review, and the conceptualization, while Berhe Tadesse Abay is participated as a co-member of the project, bringing in expertise in materials properties and assisting with data analysis. Taame Abraha Berhe is main advisor of the research project, providing guidance and expertise in the field of halide double perovskite solar cell as well as serving the corresponding author of the idea and content designer. All authors have read and approved the final manuscript.
The authors declare no conflict of interest.
[1] |
Xing GH, Mathews N, Lim SS, et al. (2014) Low-temperature solution-processed wavelength-tunable perovskites for lasing. Nature Mater 13: 476–480. https://doi.org/10.1038/nmat3911 doi: 10.1038/nmat3911
![]() |
[2] |
Tan ZK, Moghaddam RS, Lai ML, et al. (2014) Bright light-emitting diodes based on organometal halide perovskite. Nature Nanotech 9: 687–692. https://doi.org/10.1038/nnano.2014.149 doi: 10.1038/nnano.2014.149
![]() |
[3] |
Chin XY, Cortecchia D, Yin J, et al. (2015) Lead iodide perovskite light-emitting field-effect transistor. Nat Commun 6: 7383. https://doi.org/10.1038/ncomms8383 doi: 10.1038/ncomms8383
![]() |
[4] |
Boix PP, Nonomura K, Mathews N, et al. (2014) Current progress and future perspectives for organic/inorganic perovskite solar cells. Mater Today 17: 16–23. https://doi.org/10.1016/j.mattod.2013.12.002 doi: 10.1016/j.mattod.2013.12.002
![]() |
[5] |
Zhao XG, Yang DW, Ren JC, et al. (2018) Rational design of halide double perovskites for optoelectronic applications. Joule 2: 1662–1673. https://doi.org/10.1016/j.joule.2018.06.017 doi: 10.1016/j.joule.2018.06.017
![]() |
[6] | Li ZZ, Yin WJ (2018) Recent progress in Pb-free stable inorganic double halide perovskites. J Semicond 39: 071003. https://dx.doi.org/10.1088/1674-4926/39/7/071003 |
[7] |
Kumar MH, Dharani S, Leong WL, et al. (2014) Lead-free halide perovskite solar cells with high photocurrents realized through vacancy modulation. Adv Mater 26: 7122–7127. https://doi.org/10.1002/adma.201401991 doi: 10.1002/adma.201401991
![]() |
[8] |
Hao F, Stoumpos CC, Cao DH, et al. (2014) Lead-free solid-state organic–inorganic halide perovskite solar cells. Nat Photon 8: 489–494. https://doi.org/10.1038/nphoton.2014.82 doi: 10.1038/nphoton.2014.82
![]() |
[9] |
Harikesh PC, Mulmudi HK, Ghosh B, et al. (2016) Rb as an alternative cation for templating inorganic lead-free perovskites for solution processed photovoltaics. Chem Mater 28: 7496–7504. https://doi.org/10.1021/acs.chemmater.6b03310 doi: 10.1021/acs.chemmater.6b03310
![]() |
[10] |
Linaburg ETM, Matthew R, Majher Jackson D, et al. (2017) Cs1-xRbxPbCl3 and Cs1-xRbxPbBr3 solid solutions: Understanding octahedral tilting in lead halide perovskites. Chem Mater 29: 3507-3514. https://doi.org/10.1021/acs.chemmater.6b05372 doi: 10.1021/acs.chemmater.6b05372
![]() |
[11] |
Volonakis G, Haghighirad AA, Milot RL (2017) Cs2InAgCl6: A new lead-free halide double perovskite with direct band gap. J Phys Chem Lett 8: 772–778. https://doi.org/10.1021/acs.jpclett.6b02682 doi: 10.1021/acs.jpclett.6b02682
![]() |
[12] |
Zhao XG, Yang DW, Sun YH, et al. (2017) Cu-In halide perovskite solar absorbers. J Am Chem Soc 139: 6718–6725. https://doi.org/10.1021/jacs.7b02120 doi: 10.1021/jacs.7b02120
![]() |
[13] |
Ju MG, Chen M, Zhou YY, et al. (2018) Earth-abundant nontoxic titanium (Ⅳ)-based vacancy-ordered double perovskite halides with tunable 1.0 to 1.8 eV bandgaps for photovoltaic applications. ACS Energy Lett 3: 297-304. https://doi.org/10.1021/acsenergylett.7b01167 doi: 10.1021/acsenergylett.7b01167
![]() |
[14] |
Pazoki M, Johansson MB, Zhu HM, et al. (2016) Bismuth iodide perovskite materials for solar cell applications: Electronic structure, optical transitions, and directional charge transport. J Phys Chem C 120: 29039-29046. https://doi.org/10.1021/acs.jpcc.6b11745 doi: 10.1021/acs.jpcc.6b11745
![]() |
[15] |
Hutter EM, Gelvez-Rueda MC, Bartesaghi D, et al. (2018) Band-like charge transport in Cs2AgBiBr6 and mixed antimony-bismuth Cs2AgBi1-xSbxBr6 halide double perovskites. ACS Omega 3: 11655-11662. https://doi.org/10.1021/acsomega.8b01705 doi: 10.1021/acsomega.8b01705
![]() |
[16] |
Gray MB, McClure ET, Woodward PM (2019) Cs2AgBiBr6-xClx solid solutions–band gap engineering with halide double perovskites. J Mater Chem C 7: 9686-9689. https://doi.org/10.1039/C9TC02674F doi: 10.1039/C9TC02674F
![]() |
[17] |
Hong KH, Kim J, Debbichi L, et al. (2017) Band gap engineering of Cs3Bi2I9 perovskites with trivalent atoms using a dual metal cation. J Phys Chem C 121: 969-974. https://doi.org/10.1021/acs.jpcc.6b12426 doi: 10.1021/acs.jpcc.6b12426
![]() |
[18] | Karmakar A, Dodd MS, Agnihotri S, et al. (2018) Cu(Ⅱ)-doped Cs2SbAgCl6 double perovskite: A lead-free, low-bandgap material. Chem Mater 30: 8280-8290. https://doi.org/10.1021/acs.chemmater.8b03755 |
[19] |
Zhou J, Xia Z, Molokeev MS, et al. (2017) Composition design, optical gap and stability investigations of lead-free halide double perovskite Cs2AgInCl6. J Mater Chem A 5: 15031-15037. https://doi.org/10.1039/C7TA04690A doi: 10.1039/C7TA04690A
![]() |
[20] |
Chu L, Ahmad W, Liu W, et al (2019) Lead-free halide double perovskite materials: A new superstar toward green and stable optoelectronic applications. Nanomicro Lett 11: 16. https://doi.org/10.1007/s40820-019-0244-6 doi: 10.1007/s40820-019-0244-6
![]() |
[21] |
Tang Y, Liang M, Chang B, et al. (2019) Lead-free double halide perovskite Cs3BiBr6 with well-defined crystal structure and high thermal stability for optoelectronics. J Mater Chem C 7: 3369-3374. https://doi.org/10.1039/C8TC05480K doi: 10.1039/C8TC05480K
![]() |
[22] |
Luo J, Wang X, Li S, et al. (2018) Efficient and stable emission of warm-white light from lead-free halide double perovskites. Nature 563: 541-545. https://doi.org/10.1038/s41586-018-0691-0 doi: 10.1038/s41586-018-0691-0
![]() |
[23] |
Filip MR, Liu X, Miglio A, et al. (2018) Phase diagrams and stability of lead-free halide double perovskites Cs2BB'X6: B = Sb and Bi, B' = Cu, Ag, and Au, and X = Cl, Br, and I. J Phys Chem C 122: 158-170. https://doi.org/10.1021/acs.jpcc.7b10370 doi: 10.1021/acs.jpcc.7b10370
![]() |
[24] |
Flerova IN, Goreva MV, Aleksandrova KS, et al. (1998) Phase transitions in elpasolites (ordered perovskites). Mater Sci Eng R 24: 81-151. https://doi.org/10.1016/S0927-796X(98)00015-1 doi: 10.1016/S0927-796X(98)00015-1
![]() |
[25] |
Morss LR, Siegal M, Stenger L, et al. (1970) Preparation of cubic chloro complex compounds of trivalent metals: Cs2NaMCl6. Inorg Chem 9: 1771-1775. https://doi.org/10.1021/ic50089a034 doi: 10.1021/ic50089a034
![]() |
[26] |
Khalfin S, Bekenstein Y (2019) Advances in lead-free double perovskite nanocrystals, engineering band-gaps and enhancing stability through composition tunabilty. Nanoscale 11: 8665-8679. https://doi.org/10.1039/C9NR01031A doi: 10.1039/C9NR01031A
![]() |
[27] |
Igbari F, Wang ZK, Liao LS (2019) Progress of lead-free halide double perovskites. Adv Energy Mater 9: 1803150. https://doi.org/10.1002/aenm.201803150 doi: 10.1002/aenm.201803150
![]() |
[28] |
Meyer E, Mutukwa D, Zingwe N, et al. (2018) Lead-free halide double perovskites: A review of the structural, optical, and stability properties as well as their viability to replace lead halide perovskites. Metals 8: 667. https://doi.org/10.3390/met8090667 doi: 10.3390/met8090667
![]() |
[29] |
Slavney AH, Leppert L, Valdes AS, et al. (2018) Small-bandgap halide double perovskites. Angew Chem Int Ed 57: 12765 https://doi.org/10.1002/anie.201807421 doi: 10.1002/anie.201807421
![]() |
[30] | NREL Efficiency Chart. This Plot Is Courtesy of the National Renewable Energy Laboratory, Golden, CO. Available from: https://www.nrel.gov/pv/assets/pdfs/best-reserch-cell-efficiencies..20190411.pdf (accessed on 14 April 2019). |
[31] |
Chen B, Yu Z, Liu K, et al. (2019) Grain engineering for perovskite/silicon monolithic tandem solar cells with efficiency of 25.4%. Joule 3: 177-190. https://doi.org/10.1016/j.joule.2018.10.003 doi: 10.1016/j.joule.2018.10.003
![]() |
[32] |
Al-Ashouri A, Köhnen E, Li B, et al. (2020) Monolithic perovskite/silicon tandem solar cell with 29% efficiency by enhanced hole extraction. Science 370: 1300-1309. https://doi.org/10.1126/science.abd401 doi: 10.1126/science.abd401
![]() |
[33] |
Chen M, Ju MG, Garces HF, et al. (2019) Highly stable and efficient all-inorganic lead-free perovskite solar cells with native-oxide passivation. Nat Commun 10: 16. https://doi.org/10.1038/s41467-018-07951-y doi: 10.1038/s41467-018-07951-y
![]() |
[34] |
Nalianya MA, Awino C, Barasa H, et al. (2021) Numerical study of lead free CsSn0.5Ge0.5I3 perovskite solar cell by SCAPS-1D. Optik 248: 168060. https://doi.org/10.1016/j.ijleo.2021.168060 doi: 10.1016/j.ijleo.2021.168060
![]() |
[35] |
Liu X, Li T, Hao L, et al. (2023) Lead free CsSn0.5Ge0.5I3 perovskite solar cell with different layer properties via SCAPS-1D simulation. CJCE 101: 6792-6806. https://doi.org/10.1002/cjce.25009 doi: 10.1002/cjce.25009
![]() |
[36] |
Singh NK, Agarwal A (2023) Performance assessment of sustainable highly efficient CsSn0.5Ge0.5I3/FASnI3 based perovskite solar cell: A numerical modelling approach. Opt Mater 139: 113822. https://doi.org/10.1016/j.optmat.2023.113822 doi: 10.1016/j.optmat.2023.113822
![]() |
[37] |
Aktas E, Rajamanickam N, Pascual J, et al. (2022) Challenges and strategies toward long-term stability of lead-free tin-based perovskite solar cells. Commun Mater 3: 104. https://doi.org/10.1038/s43246-022-00327-2 doi: 10.1038/s43246-022-00327-2
![]() |
[38] |
Saparov B, Sun JP, Meng W, et al. (2016) Thin film deposition and characterization of a Sn-deficient perovskite derivative Cs2SnI6. Chem Mater 28: 2315–2322. https://doi.org/10.1021/acs.chemmater.6b00433 doi: 10.1021/acs.chemmater.6b00433
![]() |
[39] |
López-Fernández I, Valli D, Wang CY, et al. (2024) Lead-free halide perovskite materials and optoelectronic devices: Progress and prospective. Adv Funct Mater 34: 2307896. https://doi.org/10.1002/adfm.202307896 doi: 10.1002/adfm.202307896
![]() |
[40] |
Greul E, Petrus ML, Binek A, et al. (2017) Highly stable, phase pure Cs2AgBiBr6 double perovskite thin films for optoelectronic applications. J Mater Chem A 5: 19972–19981. https://doi.org/10.1039/C7TA06816F doi: 10.1039/C7TA06816F
![]() |
[41] |
Yang X, Chen Y, Liu P, et al. (2020) Simultaneous power conversion efficiency and stability enhancement of Cs2AgBiBr6 lead-free inorganic perovskite solar cell through adopting a multifunctional dye interlayer. Adv Funct Mater 30: 2001557. https://doi.org/10.1002/adfm.202001557 doi: 10.1002/adfm.202001557
![]() |
[42] |
Zhang Z, Sun Q, Lu Y, et al. (2022) Hydrogenated Cs2AgBiBr6 for significantly improved efficiency of lead-free inorganic double perovskite solar cell. Nat Commun 13: 3397. https://doi.org/10.1038/s41467-022-31016-w doi: 10.1038/s41467-022-31016-w
![]() |
[43] |
Chen M, Ju MG, Carl AD, et al. (2018) Cesium titanium(IV) bromide thin films based stable lead-free perovskite solar cells. Joule 2: 1–13. https://doi.org/10.1016/j.joule.2018.01.009 doi: 10.1016/j.joule.2018.01.009
![]() |
[44] |
Ahmed S, Jannat F, Khan MAK, et al. (2021) Numerical development of eco-friendly Cs2TiBr6 based perovskite solar cell with all-inorganic charge transport materials via SCAPS-1D. Optik 225: 165765. https://doi.org/10.1016/j.ijleo.2020.165765 doi: 10.1016/j.ijleo.2020.165765
![]() |
[45] |
Mercy PAM, Wilson KSJ (2023) Development of environmental friendly high performance Cs2TiBr6 based perovskite solar cell using numerical simulation. Appl Surf Sci Adv 15: 100394. https://doi.org/10.1016/j.apsadv.2023.100394 doi: 10.1016/j.apsadv.2023.100394
![]() |
[46] |
Zhang P, Yang J, Wei SH (2018) Manipulation of cation combinations and configurations of halide double perovskites for solar cell absorbers. J Mater Chem A 6: 1809–1815. https://doi.org/10.1039/C7TA09713A doi: 10.1039/C7TA09713A
![]() |
[47] |
Berhe TA, Su WN, Chen CH, et al. (2016) Organometal halide perovskite solar cells: Degradation and stability. Energy Environ Sci 9: 323–356. https://doi.org/10.1039/C5EE02733K doi: 10.1039/C5EE02733K
![]() |
[48] |
Dai X, Deng Y, Van BCH, et al. (2019) Meniscus fabrication of halide perovskite thin films at high throughput for large area and low-cost solar panels. IJEM 1: 022004. https://doi.org/10.1088/2631-7990/ab263e doi: 10.1088/2631-7990/ab263e
![]() |
[49] |
Tiing TV (2018) Octadecylamine-functionalized single-walled carbon nanotubes for facilitating the formation of a monolithic perovskite layer and stable solar cells. Adv Funct Mater 28: 1705545. https://doi.org/10.1002/adfm.201705545 doi: 10.1002/adfm.201705545
![]() |
[50] |
Wu S, Liu Y (2023) Recent advancements and manipulation strategies of colloidal Cs2BIBⅢX6 lead-free halide double perovskite nanocrystals. Nano Res 16: 5572–5591. https://doi.org/10.1007/s12274-022-5232-3 doi: 10.1007/s12274-022-5232-3
![]() |
[51] |
Volonakis GF, Haghighirad MR, Sakai AA, et al. (2016) Lead-free halide double perovskites via heterovalent substitution of noble metals. J Phys Chem Lett 7: 1254-1259. https://doi.org/10.1021/acs.jpclett.6b00376 doi: 10.1021/acs.jpclett.6b00376
![]() |
[52] |
Roknuzzaman M, Zhang C, Ostrikov K, et al. (2019) Electronic and optical properties of lead-free hybrid double perovskites for photovoltaic and optoelectronic applications. Sci Rep 9: 718. https://doi.org/10.1038/s41598-018-37132-2 doi: 10.1038/s41598-018-37132-2
![]() |
[53] |
Stoumpos CC, Malliakas CD, Kanatzidis MG, et al. (2013) Semiconducting tin and lead iodide perovskites with organic cations: Phase transitions, high mobilities and near-infrared photoluminescent properties. Inorg Chem 52: 9019–9038. https://doi.org/10.1021/ic401215x doi: 10.1021/ic401215x
![]() |
[54] |
Ponseca CS, Savenije TJ, Abdellah M, et al. (2014) Organometal halide perovskite solar cell materials rationalized: Ultrafast charge generation, high and microsecond-long balanced mobilities, and slow recombination. J Am Chem Soc 136: 5189–5192. https://doi.org/10.1021/ja412583t doi: 10.1021/ja412583t
![]() |
[55] |
Pham HQ, Holmes RJ, Aydil ES, et al. (2019) Lead-free double perovskites Cs2InCuCl6 and (CH3NH3)2InCuCl6: Electronic, optical and electrical properties. Nanoscale 11: 11173–11182. https://doi.org/10.1039/C9NR01645G doi: 10.1039/C9NR01645G
![]() |
[56] |
Wei F, Deng Z, Sun S (2017) Synthesis and properties of a lead-free hybrid double perovskite: (CH3NH3)2AgBiBr6. Chem Mater 29: 1089–1094. https://doi.org/10.1021/acs.chemmater.6b03944 doi: 10.1021/acs.chemmater.6b03944
![]() |
[57] |
Xing G, Mathews N, Sun S, et al. (2013) Long-range balanced electron- and hole-transport lengths in organic-inorganic CH3NH3PbI3. Science 342: 344–347. https://doi.org/10.1126/science.124316 doi: 10.1126/science.124316
![]() |
[58] |
Etgar L, Gao P, Xue Z, et al. (2012) Mesoscopic CH3NH3PbI3/TiO2 heterojunction solar cells. J Am Chem Soc 134: 17396–17399. https://doi.org/10.1021/ja307789s doi: 10.1021/ja307789s
![]() |
[59] |
Ball JM, Lee MM, Hey A, et al. (2013) Low-temperature processed meso-superstructured to thin-film perovskite solar cells. Energy Environ Sci 6: 1739–1743. https://doi.org/10.1039/C3EE40810H doi: 10.1039/C3EE40810H
![]() |
[60] |
Jeon NJ, Noh JH, Kim YC, et al. (2014) Solvent engineering for high-performance inorganic–organic hybrid perovskite solar cells. Nat Mater 13: 897–903. https://doi.org/10.1038/nmat4014 doi: 10.1038/nmat4014
![]() |
[61] |
Burschka J, Pellet N, Moon SJ, et al. (2013) Sequential deposition as a route to high-performance perovskite-sensitized solar cells. Nature 499: 316–319. https://doi.org/10.1038/nature12340 doi: 10.1038/nature12340
![]() |
[62] |
Liu D, Kelly TL (2014) Perovskite solar cells with a planar heterojunction structure prepared using room-temperature solution processing techniques. Nat Photon 8: 133–138. https://doi.org/10.1038/nphoton.2013.342 doi: 10.1038/nphoton.2013.342
![]() |
[63] |
Eperon GE, Stranks SD, Menelaou C (2014) Formamidinium lead trihalide: A broadly tunable perovskite for efficient planar heterojunction solar cells. Energy Environ Sci 7: 982–988. https://doi.org/10.1039/C3EE43822H doi: 10.1039/C3EE43822H
![]() |
[64] |
Kojima A, Teshima K, Shirai Y, et al. (2009) Organometal halide perovskites as visible-light sensitizers for photovoltaic cells. J Am Chem Soc 131: 6050–6051. https://doi.org/10.1021/ja809598r doi: 10.1021/ja809598r
![]() |
[65] |
Ghanshyam PVB, Kim C, Lookman T (2016) Finding new perovskite halides via machine learning. Front Mater 3: 19. https://doi.org/10.3389/fmats.2016.00019 doi: 10.3389/fmats.2016.00019
![]() |
[66] |
Gonzalez-Pedro V, Juarez-Perez EJ, Arsyad WS (2014) General working principles of CH3NH3PbX3 perovskite solar cells. Nano Lett 14: 888–893. https://doi.org/10.1021/nl404252e doi: 10.1021/nl404252e
![]() |
[67] |
Uddin MA, Calabro RL, Kim DY, et al. (2018) Halide exchange and surface modification of metal halide perovskite nanocrystals with alkyltrichlorosilanes. Nanoscale 10: 16919–16927. https://doi.org/10.1039/C8NR04763D doi: 10.1039/C8NR04763D
![]() |
[68] |
Protesescu L, Yakunin S, Bodnarchuk MI (2015) Nanocrystals of cesium lead halide perovskites (CsPbX3, X = Cl, Br, and I): Novel optoelectronic materials showing bright emission with wide color gamut. Nano Lett 15: 3692–3696. https://doi.org/10.1021/nl5048779 doi: 10.1021/nl5048779
![]() |
[69] |
Nedelcu G, Protesescu L, Yakunin S (2015) Fast anion-exchange in highly luminescent nanocrystals of cesium lead halide perovskites (CsPbX3, X = Cl, Br, I). Nano Lett 15: 5635–5640. https://doi.org/10.1021/acs.nanolett.5b02404 doi: 10.1021/acs.nanolett.5b02404
![]() |
[70] |
Jeon JHN, Yang WS, Kim YC, et al. (2015) Compositional engineering of perovskite materials for high-performance solar cells. Nature 517: 476–480. https://doi.org/10.1038/nature14133 doi: 10.1038/nature14133
![]() |
[71] |
Lee JW, Kim DH, Kim KS, et al. (2015) Formamidinium and cesium hybridization for photo- and moisture-stable perovskite solar cell. Adv Energy Mater 5: 1501310. https://doi.org/10.1002/aenm.201501310 doi: 10.1002/aenm.201501310
![]() |
[72] |
Park YH, Jeong I, Bae S, et al. (2017) Inorganic rubidium cation as an enhancer for photovoltaic performance and moisture stability of HC(NH2)2PbI3 perovskite solar cells. Adv Funct Mater 27: 1605988. https://doi.org/10.1002/adfm.201605988 doi: 10.1002/adfm.201605988
![]() |
[73] |
Locardi F, Cirignano M, Dmitry BZD, et al. (2018) Colloidal synthesis of double perovskite Cs2AgInCl6 and Mn-doped Cs2AgInCl6 nanocrystals. J Am Chem Soc 140: 12989–12995 https://doi.org/10.1021/jacs.8b07983 doi: 10.1021/jacs.8b07983
![]() |
[74] |
Stoumpos CCF, Clark L, Kim DJ, et al. (2015) Hybrid germanium iodide perovskite semiconductors: Active lone pairs, structural distortions, direct and indirect energy gaps, and strong nonlinear optical properties. J Am Chem Soc 137: 6804-6819. https://doi.org/10.1021/jacs.5b01025 doi: 10.1021/jacs.5b01025
![]() |
[75] |
Berhe TA, Su WN, Hwang BJ (2024) Halide perovskites' multifunctional properties: Coordination engineering, coordination chemistry, electronic interactions and energy applications beyond photovoltaics. Inorganics 12: 182. https://doi.org/10.3390/inorganics12070182 doi: 10.3390/inorganics12070182
![]() |
[76] |
Hua WAE, Johansson MB, Wang J, (2021) Mixed-halide double perovskite Cs2AgBiX6 (X = Br, I) with tunable optical properties via anion exchange. ChemSusChem 14: 4507–4515. https://doi.org/10.1002/cssc.202101146 doi: 10.1002/cssc.202101146
![]() |
[77] |
Abdulmutta T, Rassamessan A (2024) First-principles study on structural, electronic and optical properties of mixed alloys of Cs2Ag(SbxBi). Comput Mater Sci 239. https://doi.org/10.1039/D3RA02566G doi: 10.1039/D3RA02566G
![]() |
[78] |
Noel NK, Stranks SD, Abate A, et al. (2014). Lead-free organic–inorganic tin halide perovskites for photovoltaic applications. Energy Environ Sci 7: 3061–3068. https://doi.org/10.1039/C4EE01076K doi: 10.1039/C4EE01076K
![]() |
[79] |
Xiao ZY, Shao Y, Wang Y, et al. (2015) Giant switchable photovoltaic effect in organometal trihalide perovskite devices. Nat Mater 14: 193-198. https://doi.org/10.1038/nmat4150 doi: 10.1038/nmat4150
![]() |
[80] |
Bertoluzzi LS, Liu RS, Lee L, et.al. (2015) Cooperative kinetics of depolarization in CH3NH3PbI3 perovskite solar cells. Energy Environ Sci 8: 910–915. https://doi.org/10.1039/C4EE03171G doi: 10.1039/C4EE03171G
![]() |
[81] |
Snaith HJA, Ball A, Eperon JM, et al. (2014) Anomalous hysteresis in perovskite solar cells. J Phys Chem Lett 5: 1511–1515. https://doi.org/10.1021/jz500113x doi: 10.1021/jz500113x
![]() |
[82] |
Tress WM, Moehl N, Zakeeruddin T, et al. (2015) Understanding the rate-dependent J-V hysteresis, slow time component, and aging in CH3NH3PBI3 perovskite solar cells: The role of a compensated electric field. Energy Environ Sci 8: 995–1004. https://doi.org/10.1039/C4EE03664F doi: 10.1039/C4EE03664F
![]() |
[83] |
Hoke ET, Slotcavage DJ, Dohner ER, et al. (2015) Reversible photo-induced trap formation in mixed-halide hybrid perovskites for photovoltaics. Chem Sci 6: 613–617. https://doi.org/10.1039/C4SC03141E doi: 10.1039/C4SC03141E
![]() |
[84] |
Fan ZX, Sun J, Chen K, et al. (2015) Ferroelectricity of CH3NH3PbI3 perovskite. J Phys Chem Lett 6: 1155–1161. https://doi.org/10.1021/acs.jpclett.5b00389 doi: 10.1021/acs.jpclett.5b00389
![]() |
[85] |
Rong Y, Hu Y, Ravishankar S, et al. (2017) Tunable hysteresis effect for perovskite solar cells, Energy Environ Sci 10: 2383–2391. https://doi.org/10.1039/C7EE02048A doi: 10.1039/C7EE02048A
![]() |
[86] |
Balaguera EH, Bisquert J (2024) Accelerating the assessment of hysteresis in perovskite solar cells. ACS Energy Lett 9: 478–486. https://doi.org/10.1021/acsenergylett.3c02779 doi: 10.1021/acsenergylett.3c02779
![]() |
[87] | Singh R, Parashar M (2020) Origin of hysteresis in perovskite solar cells, In: Ren JZ, Kan ZP, Soft-Matter Thin Film Solar Cells: Physical Processes and Device Simulation, New York: AIP Publishing LLC. https://doi.org/10.1063/9780735422414_001 |
[88] |
Kim HS, Park NG (2014) Parameters affecting Ⅰ–Ⅴ hysteresis of CH3NH3PbI3 perovskite solar cells: Effects of perovskite crystal size and mesoporous TiO2 layer. J Phys Chem Lett 5: 2927–2934. https://doi.org/10.1021/jz501392m doi: 10.1021/jz501392m
![]() |
[89] |
Leijtens T, Ram SKA, Eperon GE, et al. (2015) Modulating the electron-hole interaction in a hybrid lead halide perovskite with an electric field. J Am Chem Soc 137: 15451–15459. https://doi.org/10.1021/jacs.5b09085 doi: 10.1021/jacs.5b09085
![]() |
[90] |
Chaves A, Azadani JG, Alsalman H, et al. (2020) Low bandgap engineering of two-dimensional semiconductor materials. npj 2D Mater Appl 4: 29. https://doi.org/10.1038/s41699-020-00162-4 doi: 10.1038/s41699-020-00162-4
![]() |
[91] |
Prasanna R, Gold-Parker A, Leijtens T, et al. (2017) Band gap tuning via lattice contraction and octahedral tilting in perovskite materials for photovoltaics. J Am Chem Soc 139: 11117–11124. https://doi.org/10.1021/jacs.7b04981 doi: 10.1021/jacs.7b04981
![]() |
[92] |
Lee JH, Bristowe NC, Lee JH, et al. (2016) Resolving the physical origin of octahedral tilting in halide perovskites. Chem Mater 28: 4259–4266. https://doi.org/10.1021/acs.chemmater.6b00968 doi: 10.1021/acs.chemmater.6b00968
![]() |
[93] |
Bechtel JS, Van der Ven A (2018) Octahedral tilting instabilities in inorganic halide perovskites. Phys Rev Materials 2: 025401. https://doi.org/10.1103/PhysRevMaterials.2.025401 doi: 10.1103/PhysRevMaterials.2.025401
![]() |
[94] |
Yang RX, Skelton JM, Silva EL, et al. (2017) Spontaneous octahedral tilting in the cubic inorganic cesium halide perovskites CsSnX3 and CsPbX3 (X = F, Cl, Br, I). J Phys Chem Lett 8: 4720-4726. https://doi.org/10.1021/acs.jpclett.7b02423 doi: 10.1021/acs.jpclett.7b02423
![]() |
[95] |
Shao Y, Gao W, Yan H, (2022) Unlocking surface octahedral tilt in twodimensional Ruddlesden-Popper perovskites. Nat Commun 13: 138. https://doi.org/10.1038/s41467-021-27747-x doi: 10.1038/s41467-021-27747-x
![]() |
[96] |
Klarbring J (2018) Low energy paths for octahedral tilting in inorganic halide perovskites. Phys Rev B 99: 104105. https://doi.org/10.1103/PhysRevB.99.104105 doi: 10.1103/PhysRevB.99.104105
![]() |
[97] |
Munson KT, Kennehan ER, Doucette GS, et al. (2018) Dynamic disorder dominates delocalization, transport, and recombination in halide perovskites. Chem 4: 2826-2843. https://doi.org/10.1016/j.chempr.2018.09.00199 doi: 10.1016/j.chempr.2018.09.00199
![]() |
[98] |
Lei H, Hardy D, Gao F (2021) Lead‐free double perovskite Cs2AgBiBr6: Fundamentals, applications and perspectives. Adv Funct Mater 31: 2105898. https://doi.org/10.1002/adfm.202105898 doi: 10.1002/adfm.202105898
![]() |
[99] | Karunadasa HI, Slavney AH (2022) Alloyed halide double perovskites as solar-cell absorbers. Available from: https://www.osti.gov/servlets/purl/1892693. |
[100] |
Palmstrom AF, Eperon GE, Leijtens T, et al. (2019) Enabling flexible all-perovskite tandem solar cells. Joule 3: 1–12. https://doi.org/10.1016/j.joule.2019.05.009 doi: 10.1016/j.joule.2019.05.009
![]() |
[101] |
Zhou L, Xu YF, Chen BX, et al. (2018) Synthesis and photocatalytic application of stable lead free Cs2AgBiBr6 perovskite nanocrystals. Small 14: 1703762. https://doi.org/10.1002/smll.201703762 doi: 10.1002/smll.201703762
![]() |
[102] |
Zhang ZZ, Liang Y, Huang H, et al. (2019) Stable and highly efficient photocatalysis with lead-free double-perovskite of Cs2AgBiBr6. Angew Chem Int Ed 58: 7263–7267. https://doi.org/10.1002/anie.201900658 doi: 10.1002/anie.201900658
![]() |
[103] |
Cho J, DuBose JT, Kamat PV (2020) Charge injection from excited Cs2AgBiBr6 quantum dots into semiconductor oxides. Chem Mater 32: 510–517. https://doi.org/10.1021/acs.chemmater.9b04243 doi: 10.1021/acs.chemmater.9b04243
![]() |
[104] |
Ippili S, Kim JH, Jella V, et al. (2023) Halide double perovskite-based efficient mechanical energy harvester and storage devices for self-charging power unit. Nano Energy 107: 108148. https://doi.org/10.1016/j.nanoen.2022.108148 doi: 10.1016/j.nanoen.2022.108148
![]() |
[105] |
Wu H, Pi J, Liu Q, et al. (2021) All-inorganic lead free double perovskite li-battery anode material hosting high Li+ ion concentrations. J Phys Chem Lett 12: 4125–4129. https://doi.org/10.1021/acs.jpclett.1c00041 doi: 10.1021/acs.jpclett.1c00041
![]() |
[106] |
Zhang L, Miao J, Li J, et al. (2020) Halide perovskite materials for energy storage applications. Adv Funct Mater 30: 2003653. https://doi.org/10.1002/adfm.202003653 doi: 10.1002/adfm.202003653
![]() |
[107] |
Yang S, Liang Q, Wu H, et al. (2022) Lead-free double perovskite Cs2NaErCl6: Li+ as high-stability anodes for Li-ion batteries. J Phys Chem Lett 13: 4981–4987. https://doi.org/10.1021/acs.jpclett.2c01052 doi: 10.1021/acs.jpclett.2c01052
![]() |
[108] |
Choon SL, Lim HN (2024) Impact of vacancies in halide perovskites for batteries and supercapacitors. Mater Today Energy 43: 101577. https://doi.org/10.1016/j.mtener.2024.101577 doi: 10.1016/j.mtener.2024.101577
![]() |
[109] |
Yadav A, Saini A, Kumar P, et al. (2024) Lead-free halide perovskites for high-performance thin-film flexible supercapacitor applications. J Mater Chem C 12: 197–206. https://doi.org/10.1039/D3TC02784H doi: 10.1039/D3TC02784H
![]() |
[110] |
Tan Y, Mu G, Chen M, et al. (2023) X-ray detectors based on halide perovskite materials. Coatings 13: 211. https://doi.org/10.3390/coatings13010211 doi: 10.3390/coatings13010211
![]() |
[111] |
Tailor NK, Ghosh J, Afroz MA, et al. (2022) Self-powered X-ray detection and imaging using Cs2AgBiCl6 lead-free double perovskite single crystal. ACS Appl Electron Mater 4: 4530–4539. https://doi.org/10.1021/acsaelm.2c00752 doi: 10.1021/acsaelm.2c00752
![]() |
[112] |
Li Z, Zhou F, Yao H, et al. (2021) Halide perovskites for high-performance X-ray detector. Materials Today 48: 155–175. https://doi.org/10.1016/j.mattod.2021.01.028 doi: 10.1016/j.mattod.2021.01.028
![]() |
[113] |
Saparov B, Mitzi DB (2016) Organic–inorganic perovskites: structural versatility for functional materials design. Chem Rev 116: 4558–4596. https://doi.org/10.1021/acs.chemrev.5b00715 doi: 10.1021/acs.chemrev.5b00715
![]() |
[114] | Best Research-Cell Efficiency Chart. (2019) Available from: https://www.nrel.gov/pv/cell-efficiency.html. |
[115] |
Bhojak V, Bhatia D, Jain PK (2022) Investigation of photocurrent efficiency of Cs2TiBr6 double perovskite solar cell. Mater Today Proc 66: 3692–3697. https://doi.org/10.1016/j.matpr.2022.07.443 doi: 10.1016/j.matpr.2022.07.443
![]() |
[116] |
Qin X, Zhao Z, Wang Y, et al. (2017) Recent progress in stability of perovskite solar cells. J Semicond 38: 011002. https://doi.org/10.1088/1674-4926/38/1/011002 doi: 10.1088/1674-4926/38/1/011002
![]() |
[117] |
Berhe TA, Cheng JH, Su WN, et al. (2017) Identification of the physical origin behind disorder, heterogeneity, and reconstruction and their correlation with the photoluminescence lifetime in hybrid perovskite thin films. J Mater Chem A 5: 21002–21015. https://doi.org/10.1039/C7TA04615D doi: 10.1039/C7TA04615D
![]() |
[118] |
Xiao Z, Meng W, Wang J, et al. (2017) Searching for promising new perovskite-based photovoltaic absorbers: The importance of electronic dimensionality. Mater Horiz 4: 206–216. https://doi.org/10.1039/C6MH00519E doi: 10.1039/C6MH00519E
![]() |
[119] |
Zhang Q, Ting H, Wei S, et al. (2018) Recent progress in lead-free perovskite (-like) solar cells. Mater Today Energy 8: 157–165. https://doi.org/10.1016/j.mtener.2018.03.001 doi: 10.1016/j.mtener.2018.03.001
![]() |
[120] |
Kanno S, Imamura Y, Hada M (2019) Alternative materials for perovskite solar cells from materials informatics. Phys Rev Mater 3: 075403. https://doi.org/10.1103/PhysRevMaterials.3.075403 doi: 10.1103/PhysRevMaterials.3.075403
![]() |
[121] |
Liang L, Gao P (2018) Lead-free hybrid perovskite absorbers for viable application: Can we eat the cake and have it too? Adv Sci (Weinh) 5: 1700331. https://doi.org/10.1002/advs.201700331 doi: 10.1002/advs.201700331
![]() |
[122] |
Abate A (2017) Perovskite solar cells go lead free. Joule 1: 659–664. https://doi.org/10.1016/j.joule.2017.09.007 doi: 10.1016/j.joule.2017.09.007
![]() |
[123] |
Xu Q, Yang D, Lv J, et al. (2018) Perovskite solar absorbers: Materials by design. Small Methods 2: 1700316. https://doi.org/10.1002/smtd.201700316 doi: 10.1002/smtd.201700316
![]() |
[124] |
Volonakis G, Giustino F (2018) Surface properties of lead-free halide double perovskites: Possible visible-light photo-catalysts for water splitting featured. Appl Phys Lett 112: 243901. https://doi.org/10.1063/1.5035274 doi: 10.1063/1.5035274
![]() |
[125] |
Slavney AH, Hu T, Lindenberg AM, et al. (2016) A bismuth-halide double perovskite with long carrier recombination lifetime for photovoltaic applications. J Am Chem Soc 138: 2138-2141. https://doi.org/10.1021/jacs.5b13294 doi: 10.1021/jacs.5b13294
![]() |
[126] | Karunadasa HI, Slavney AH (2017) Halide double perovskite Cs2AgBiBr6 solar-cell absorber having long carrier lifetimes. |
[127] |
Savory CN, Walsh A, Scanlon DO (2016) Can Pb-free halide double perovskites support high-efficiency solar cells? ACS Energy Lett 1: 949-955. https://doi.org/10.1021/acsenergylett.6b00471 doi: 10.1021/acsenergylett.6b00471
![]() |
[128] |
Pecunia V, Occhipinti LG, Chakraborty A (2020) Lead-free halide perovskite photovoltaics: Challenges, open questions, and opportunities. APL Materials 8: 100901. https://doi.org/10.1063/5.0022271 doi: 10.1063/5.0022271
![]() |
[129] |
Rühle S (2017) The detailed balance limit of perovskite/silicon and perovskite/CdTe tandem solar cells. Phys Status Solidi A 214: 1600955. https://doi.org/10.1002/pssa.201600955 doi: 10.1002/pssa.201600955
![]() |
[130] |
Sheng R, Ho-Baillie AWY, Huang SJ, et al. (2015) Four-terminal tandem solar cells using CH3NH3PbBr3 by spectrum splitting. J Phys Chem Lett 6: 3931-3934. https://doi.org/10.1021/acs.jpclett.5b01608 doi: 10.1021/acs.jpclett.5b01608
![]() |
[131] |
Sani F, Shafie S, Lim HN (2018) Advancement on lead-free organic-inorganic halide perovskite solar cells: A review. Materials 11: 1008. https://doi.org/10.3390/ma11061008 doi: 10.3390/ma11061008
![]() |
[132] |
Righetto M, Caicedo-Dávila S, Sirtl MT, et al. (2023) Alloying effects on charge-carrier transport in silver–bismuth double perovskites. J Phys Chem Lett 14: 10340–10347 https://doi.org/10.1021/acs.jpclett.3c02750 doi: 10.1021/acs.jpclett.3c02750
![]() |
[133] |
Bartesaghi D, Slavney AH, Gélvez-Rueda MC, et al. (2018) Charge carrier dynamics in Cs2AgBiBr6 double perovskite. J Phys Chem C 122: 4809–4816. https://doi.org/10.1021/acs.jpcc.8b00572 doi: 10.1021/acs.jpcc.8b00572
![]() |
[134] |
Joshua-Leveillee GV, Giustino F (2021) Phonon-limited mobility and electronphonon coupling in lead-free halide double perovskites. J Phys Chem Lett 12: 4474–4482. https://doi.org/10.1021/acs.jpclett.1c00841 doi: 10.1021/acs.jpclett.1c00841
![]() |
[135] |
Lin H, Zhou C, Tian Y (2018) Low-dimensional organometal halide perovskites. ACS Energy Lett 3: 54-62. https://doi.org/10.1021/acsenergylett.7b00926 doi: 10.1021/acsenergylett.7b00926
![]() |
[136] |
Han D, Shi H, Ming W, et al. (2018) Unraveling luminescence mechanisms in zero-dimensional halide perovskites. J Mater Chem C 6: 6398-6405. https://doi.org/10.1039/C8TC01291A doi: 10.1039/C8TC01291A
![]() |
[137] |
Duan D, Ge C, Rahaman MZ, et al. (2023) Recent progress with one-dimensional metal halide perovskites: from rational synthesis to optoelectronic applications. NPG Asia Mater 15: 8. https://doi.org/10.1038/s41427-023-00465-0 doi: 10.1038/s41427-023-00465-0
![]() |
[138] |
Qiu T, Hu Y, Xu F (2018) Recent advances in one-dimensional halide perovskites for optoelectronic applications. Nanoscale 10: 20963-20989. https://doi.org/10.1039/C8NR05862H doi: 10.1039/C8NR05862H
![]() |
[139] |
Chen X, Zhou H, Wang H (2021) 2D/3D halide perovskites for optoelectronic devices. Front Chem 9. https://doi.org/10.3389/fchem.2021.715157 doi: 10.3389/fchem.2021.715157
![]() |
[140] |
Fiorentino F, Albaqami MD, Poli I, et al. (2022) Thermal- and light-induced evolution of the 2D/3D interface in lead-halide perovskite films. ACS Appl Mater Interfaces 14: 34180-34188. https://doi.org/10.1021/acsami.1c09695 doi: 10.1021/acsami.1c09695
![]() |
[141] |
Kim EB, Akhtar MS, Shin HS, et al. (2021) A review on two-dimensional (2D) and 2D-3D multidimensional perovskite solar cells: Perovskites structures, stability, and photovoltaic performances. J Photochem Photobiol C 48: 100405. https://doi.org/10.1016/j.jphotochemrev.2021.100405 doi: 10.1016/j.jphotochemrev.2021.100405
![]() |
[142] |
Etgar L (2018) The merit of perovskite's dimensionality; can this replace the 3D halide perovskite? Energy Environ Sci 11: 234-242. https://doi.org/10.1039/C7EE03397D doi: 10.1039/C7EE03397D
![]() |
[143] |
Marongiu D, Saba M, Quochi F (2019) The role of excitons in 3D and 2D lead halide perovskites. J Mater Chem C 7: 12006-12018. https://doi.org/10.1039/C9TC04292J doi: 10.1039/C9TC04292J
![]() |
[144] |
Moral RF, Perini CAR, Kodalle T, et al. (2024) Anion and cation migration at 2D/3D halide perovskite interfaces. ACS Energy Lett 9: 2703-2716. https://doi.org/10.1021/acsenergylett.4c00728 doi: 10.1021/acsenergylett.4c00728
![]() |
[145] |
Filip MR, Hillman S, Haghighirad AA, et al. (2016) Band gaps of the lead-free halide double perovskites: Cs2BiAgCl6 and Cs2BiAgBr6 from theory and experiment. J Phys Chem Lett 7: 2579-2585. https://doi.org/10.1021/acs.jpclett.6b01041 doi: 10.1021/acs.jpclett.6b01041
![]() |
[146] |
McClure ETB, Windl MR, Woodward PMW (2016) Cs2AgBiX6 (X = Br, Cl): New visible light absorbing, lead-free halide perovskite semiconductors. Chem Mater 28: 1348-1354. https://doi.org/10.1021/acs.chemmater.5b04231 doi: 10.1021/acs.chemmater.5b04231
![]() |
[147] |
Luo SLJ, Wu H, Zhou Y, et al. (2018) Cs2AgInCl6 double perovskite single crystals: Parity forbidden transitions and their application for sensitive and fast UV photodetectors. ACS Photonics 5: 398. https://doi.org/10.1021/acsphotonics.7b00837 doi: 10.1021/acsphotonics.7b00837
![]() |
[148] |
Zhou HC, Li Q, Luo G, et al. (2014) Interface engineering of highly efficient perovskite solar cells. Science 345: 542-546. https://doi.org/10.1126/science.1254050 doi: 10.1126/science.1254050
![]() |
[149] |
De Quilettes DWV, Stranks SM, Nagaoka SD, et al. (2015) Impact of microstructure on local carrier lifetime in perovskite solar cells. Science 348: 683-686. https://doi.org/10.1126/science.aaa5333 doi: 10.1126/science.aaa5333
![]() |
[150] |
Rui SHH, Chen T, Gui R, et al. (2023) Extracting energetic disorder in organic solar cells using percolation models. ChemPhysMater 2: 52–57. https://doi.org/10.1016/j.chphma.2022.03.002 doi: 10.1016/j.chphma.2022.03.002
![]() |
[151] |
Wang DD, Li YS, Yang YG, et al. (2024) Energetic disorder dominates optical properties and recombination dynamics in tin-lead perovskite nanocrystals. eScience 100279. https://doi.org/10.1016/j.esci.2024.100279 doi: 10.1016/j.esci.2024.100279
![]() |
[152] |
Yang C, Ma L, Xu Y (2022) Reduced energetic disorder enables over 14% efficiency in organic solar cells based on completely non-fused-ring donors and acceptors. Sci China Chem 65: 2604–2612. https://doi.org/10.1007/s11426-022-1449-4 doi: 10.1007/s11426-022-1449-4
![]() |
[153] |
Diez-Cabanes V, Samuele G, Beljonne D, et al. (2023) On the origin of energetic disorder in mixed halides lead perovskites. Adv Optical Mater 12: 2301105. https://doi.org/10.1002/adom.202301105 doi: 10.1002/adom.202301105
![]() |
[154] |
Liu Y, Banon JP, Frohna K, et al. (2023) The electronic disorder landscape of mixed halide perovskites. ACS Energy Lett 8: 250-258. https://doi.org/10.1021/acsenergylett.2c02352 doi: 10.1021/acsenergylett.2c02352
![]() |
[155] |
Yin A, Zhang D, Cheung SH, et al. (2018) On the understanding of energetic disorder, charge recombination and voltage losses in all-polymer solar cells. J Mater Chem C 6: 7855–7863. https://doi.org/10.1039/C8TC02689K doi: 10.1039/C8TC02689K
![]() |
[156] |
Igbari F, Wang R, Wang ZK, et al. (2019) Composition stoichiometry of Cs2AgBiBr6 films for highly efficient lead-free perovskite solar cells. Nano Lett 19: 2066–2073. https://doi.org/10.1021/acs.nanolett.9b00238 doi: 10.1021/acs.nanolett.9b00238
![]() |
[157] |
Bush KA, Frohna K, Prasanna R, et al. (2018) Compositional engineering for efficient wide band gap perovskites with improved stability to photoinduced phase segregation. ACS Energy Lett 3: 428–435. https://doi.org/10.1021/acsenergylett.7b01255 doi: 10.1021/acsenergylett.7b01255
![]() |
[158] |
Yang J, Zhang P, Wei SH (2018) Band structure engineering of Cs2AgBiBr6 perovskite through order–disordered transition: A first-principle study. J Phys Chem Lett 9: 31–35. https://doi.org/10.1021/acs.jpclett.7b02992 doi: 10.1021/acs.jpclett.7b02992
![]() |
[159] |
Kim J, Kim H, Mahesh C, et al. (2018) Impacts of cation ordering on bandgap dispersion of double perovskites. APL Materials 6: 084903. https://doi.org/10.1063/1.5027230 doi: 10.1063/1.5027230
![]() |
[160] |
Liu Z, Li H, Qin C, et al. (2019) Solution-processed inorganic perovskite flexible photodetectors with high performance. Nanoscale Res Lett 14: 284. https://doi.org/10.1186/s11671-019-3120-x doi: 10.1186/s11671-019-3120-x
![]() |
[161] | Chilvery A, Das S, Guggilla P, et al. (2016) A perspective on the recent progress in solution-processed methods for highly efficient perovskite solar cells. STAM 17: 650–658. http://dx.doi.org/10.1080/14686996.2016.1226120 |
[162] |
Seo JW, Noh JH, Seok SI (2016) Rational strategies for efficient perovskite solar cells. Acc Chem Res 49: 562–572. https://doi.org/10.1021/acs.accounts.5b00444 doi: 10.1021/acs.accounts.5b00444
![]() |
[163] |
Arain Z, Liu C, Yang Y, et al. (2019) Elucidating the dynamics of solvent engineering for perovskite solar cells. Sci China Mater 62: 161–172. https://doi.org/10.1007/s40843-018-9336-1 doi: 10.1007/s40843-018-9336-1
![]() |
[164] |
Li J, Yang R, Que L, et al. (2019) Optimization of anti-solvent engineering toward high performance perovskite solar cells. J Mater Res 34: 2416–2424. https://doi.org/10.1557/jmr.2019.122 doi: 10.1557/jmr.2019.122
![]() |
[165] |
Duan J, Xu H, Sha W, et al. (2019) Inorganic perovskite solar cells: An emerging member of the photovoltaic community. J Mater Chem A 7: 21036–21068. https://doi.org/10.1039/C9TA06674H. doi: 10.1039/C9TA06674H
![]() |
[166] |
Li B, Fu L, Li S, et al. (2019) Pathways toward high-performance inorganic perovskite solar cells: Challenges and strategies. J Mater Chem A 7: 20494–20518. https://doi.org/10.1039/C9TA04114A doi: 10.1039/C9TA04114A
![]() |
[167] |
Wang P, Zhang X, Zhou Y, et al. (2018) Solvent-controlled growth of inorganic perovskite films in dry environment for efficient and stable solar cells. Nat Commun 9: 2225. https://doi.org/10.1038/s41467-018-04636-4 doi: 10.1038/s41467-018-04636-4
![]() |
[168] | DeHoff RT (1999) Engineering of microstructures. Mater Res 2: 111–126. http://dx.doi.org/10.1590/S1516-14391999000300002 |
[169] | Clemens H, Mayer S, Scheu C (2017) Microstructure and properties of engineering materials: From fundamentals to applications, In: Staron P, Schreyer A, Clemens H, Mayer S, In Neutrons and Synchrotron Radiation in Engineering Materials Science, New York: John Wiley & Sons. https://doi.org/10.1002/9783527684489.ch1 |
[170] |
Szuromi P (1997) Microstructural engineering of materials. Science 277: 1183. https://doi.org/10.1126/science.277.5330.1183 doi: 10.1126/science.277.5330.1183
![]() |
[171] |
Chen L, Paillard C, Zhao HJ, et al. (2018) Tailoring properties of hybrid perovskites by domain-width engineering with charged walls. npj Computat Mater 4: 75. https://doi.org/10.1038/s41524-018-0134-3 doi: 10.1038/s41524-018-0134-3
![]() |
[172] |
Schade L, Wright AD, Johnson RD, et al. (2019) Structural and optical properties of Cs2AgBiBr6 double perovskite. ACS Energy Lett 4: 299–305. https://doi.org/10.1021/acsenergylett.8b02090 doi: 10.1021/acsenergylett.8b02090
![]() |
[173] |
Lozhkina OA, Murashkina AA, Elizarov MS, et al. (2018) Microstructural analysis and optical properties of the halide double perovskite Cs2BiAgBr6 single crystals. Chem Phys Lett 694: 18–22. https://doi.org/10.1016/j.cplett.2018.01.031 doi: 10.1016/j.cplett.2018.01.031
![]() |
[174] |
Liu W, Liu Y, Wang J, et al. (2018) Twin domains in organometallic halide perovskite thin-films. Crystals 8: 216. https://doi.org/10.3390/cryst8050216 doi: 10.3390/cryst8050216
![]() |
[175] |
Liu S, Zheng F, Koocher NZ, et al. (2015) Ferroelectric domain wall induced band gap reduction and charge separation in organometal halide perovskites. J Phys Chem Lett 6: 693-699. https://doi.org/10.1021/jz502666j doi: 10.1021/jz502666j
![]() |
[176] |
You L, Zheng F, Fang L, et al. (2018) Enhancing ferroelectric photovoltaic effect by polar order engineering. Sci Adv 4: eaat3438. https://doi.org/10.1126/sciadv.aat3438 doi: 10.1126/sciadv.aat3438
![]() |
[177] |
Li Y, Fu J, Mao X, et al. (2021) Enhanced bulk photovoltaic effect in two-dimensional ferroelectric CuInP2S6. Nat Commun 12: 5896. https://doi.org/10.1038/s41467-021-26200-3 doi: 10.1038/s41467-021-26200-3
![]() |
[178] |
Shockley W, Queisser HJ (1961) Detailed balance limit of efficiency of P-N junction solar cells. J Appl Phys 32: 510–511. https://doi.org/10.1063/1.1736034 doi: 10.1063/1.1736034
![]() |
[179] |
Ihtisham ULH, Khan MI, Ullah A, et al. (2024) Bandgap reduction and efficiency enhancement in Cs2AgBiBr6 double perovskite solar cells through gallium substitution. RSC Adv 14: 5440–5448. https://doi.org/10.1039/D3RA08965G doi: 10.1039/D3RA08965G
![]() |
[180] |
Du KZ, Meng W, Wang X, et al. (2017) Bandgap engineering of lead-free double perovskite Cs2AgBiBr6 through trivalent metal alloying. Angew Chem Int Ed 8158–8162. https://doi.org/10.1002/anie.201703970 doi: 10.1002/anie.201703970
![]() |
[181] |
Yan K, Long M, Zhang T, et al. (2015) Hybrid halide perovskite solar cell precursors: Colloidal chemistry and coordination engineering behind device processing for high efficiency. J Am Chem Soc 137: 4460–4468. https://doi.org/10.1021/jacs.5b00321 doi: 10.1021/jacs.5b00321
![]() |
[182] |
Bellakhdar T, Nabi Z, Bouabdallah B, et al. (2022) Ab initio study of structural, electronic, mechanical and optical properties of the tetragonal Cs2AgBiBr6 halide double perovskite. Appl Phys A 128: 155. https://doi.org/10.1007/s00339-022-05276-8 doi: 10.1007/s00339-022-05276-8
![]() |
[183] |
Merabet B, Alamri H, Djermouni M (2012) Optimal bandgap of double perovskite la-substituted Bi2FeCrO6 for solar cells: An ab initio GGA+U study. Chin Phys Lett 34: 016101. https://doi.org/10.1088/0256-307X/34/1/016101 doi: 10.1088/0256-307X/34/1/016101
![]() |
[184] |
Alotaibi NH, Mustafa GM, Kattan NA (2022) DFT study of double perovskites Cs2AgBiX6 (X = Cl, Br): An alternative of hybrid perovskites. J Solid State Chem 313: 123353. https://doi.org/10.1016/j.jssc.2022.123353 doi: 10.1016/j.jssc.2022.123353
![]() |
[185] |
Creutz SE, Crites EN, De-Siena MC, et al. (2018) Colloidal nanocrystals of lead-free double-perovskite (elpasolite) semiconductors: Synthesis and anion exchange to access new materials. Nano Lett 18: 1118–1123. https://doi.org/10.1021/acs.nanolett.7b04659 doi: 10.1021/acs.nanolett.7b04659
![]() |
[186] |
Hou P, Yang W, Wan N, et al. (2021) Precursor engineering for high-quality Cs2AgBiBr6 films toward efficient lead-free double perovskite solar cells. J Mater Chem C 9: 9659–9669. https://doi.org/10.1039/D1TC01786A doi: 10.1039/D1TC01786A
![]() |
[187] |
Kangsabanik J, Sugathan V, Yadav A, et al. (2018) Double perovskites overtaking the single perovskites: A set of new solar harvesting materials with much higher stability and efficiency. Phys Rev Mater 2: 055401. https://doi.org/10.1103/PhysRevMaterials.2.055401 doi: 10.1103/PhysRevMaterials.2.055401
![]() |
[188] |
Laporte O (1924) Die struktur des eisenspektrums. Z Physik 23: 135–175. https://doi.org/10.1007/BF01327582 doi: 10.1007/BF01327582
![]() |
[189] |
Nathan R, Wolf BAC, Slavney AH, et al. (2021) Doubling the stakes: The promise of halide double perovskites. Angew Chem Int Ed 60: 2–17. https://doi.org/10.1002/anie.202016185 doi: 10.1002/anie.202016185
![]() |
[190] |
Fridkin VM (2001) Bulk photovoltaic effect in noncentrosymmetric crystals. Crystallogr Rep 46: 654–658. https://doi.org/10.1134/1.1387133 doi: 10.1134/1.1387133
![]() |
[191] |
Gao P, Liu HJ, Huang YL (2016) Atomic mechanism of polarization-controlled surface reconstruction in ferroelectric thin films. Nat Commun 7: 11318. https://doi.org/10.1038/ncomms11318 doi: 10.1038/ncomms11318
![]() |
[192] |
Von Baltz WKR (1981) Theory of the bulk photovoltaic effect in pure crystals. Phys Rev B 23: 5590–5596. https://doi.org/10.1103/PhysRevB.23.5590 doi: 10.1103/PhysRevB.23.5590
![]() |
[193] |
Young SM, Rappe AM (2012) First principles calculation of the shift current photovoltaic effect in ferroelectrics. Phys Rev Lett 109: 116601. https://doi.org/10.1103/PhysRevLett.109.116601 doi: 10.1103/PhysRevLett.109.116601
![]() |
[194] |
Young SM, Zheng F, Rappe AM (2012) First-principles calculation of the bulk photovoltaic effect in bismuth ferrite. Phys Rev Lett 109: 236601. https://doi.org/10.1103/PhysRevLett.109.236601 doi: 10.1103/PhysRevLett.109.236601
![]() |
[195] |
Harikesh PC, Wu B, Ghosh B, et al. (2018) Doping and switchable photovoltaic effect in lead-free perovskites enabled by metal cation transmutation. Adv Mater 30: 1802080. https://doi.org/10.1002/adma.201802080. doi: 10.1002/adma.201802080
![]() |
[196] |
Lee D, Baek SH, Kim TH, et al. (2011) Polarity control of carrier injection at ferroelectric/metal interfaces for electrically switchable diode and photovoltaic effects. Phys Rev B 84: 125305. https://doi.org/10.1103/PhysRevB.84.125305 doi: 10.1103/PhysRevB.84.125305
![]() |
[197] |
Li H, Li F, Shena Z, et al. (2021) Photoferroelectric perovskite solar cells: Principles, advances and insights. Nano Today 37: 101062. https://doi.org/10.1016/j.nantod.2020.101062 doi: 10.1016/j.nantod.2020.101062
![]() |
[198] |
Tsien RY (2009) Constructing and exploiting the fluorescent protein paintbox (nobel lecture). Chem Int Ed 48: 5612–5626. https://doi.org/10.1002/anie.200901916 doi: 10.1002/anie.200901916
![]() |
[199] | Liu HY, Zhang HY, Chen XG, et al. (2020) Molecular design principles for ferroelectrics: Ferroelectrochemistry. J Am Chem Soc 142: 15205-15218. https://dx.doi.org/10.1021/jacs.0c07055 |
[200] |
Shi PP, Tang YY, Li PF, et al. (2016) Symmetry breaking in molecular ferroelectrics. Chem Soc Rev 45: 3811-3827. https://doi.org/10.1039/C5CS00308C doi: 10.1039/C5CS00308C
![]() |
[201] |
Li K, Li ZG, Lu J, et al. (2022) Origin of ferroelectricity in two prototypical hybrid organic-inorganic perovskites. J Am Chem Soc 144: 816–823. https://doi.org/10.1021/jacs.1c10188 doi: 10.1021/jacs.1c10188
![]() |
[202] |
Anderson PW, Blount EI (1965) Symmetry considerations on martensitic transformations: 'Ferroelectric' metals? Phys Rev Lett 14: 217–219. https://doi.org/10.1103/PhysRevLett.14.217 doi: 10.1103/PhysRevLett.14.217
![]() |
[203] |
Shi Y, Guo Y, Wang X, et al. (2013) A ferroelectric-like structural transition in a metal. Nat Mater 12: 1024–1027. https://doi.org/10.1038/NMAT3754 doi: 10.1038/NMAT3754
![]() |
[204] |
Yildirim T (2013) Ferroelectric soft phonons, charge density wave instability, and strong electron-phonon coupling in BiS2 layered superconductors: A first-principles study. Phys Rev B 87: 020506(R). https://doi.org/10.48550/arXiv.1210.2418 doi: 10.48550/arXiv.1210.2418
![]() |
[205] |
Rakita Y, Bar-Elli O, Meirzadeha E, et al. (2017) Tetragonal CH3NH3PbI3 is ferroelectric. PNAS 114: E5504–E5512. https://doi.org/10.1073/pnas.1702429114 doi: 10.1073/pnas.1702429114
![]() |
[206] |
Alexe M, Hasse D (2011) Tip-enhanced photovoltaic effects in bismuth ferrite. Nat Commun 2: 256. https://doi.org/10.1038/ncomms1261 doi: 10.1038/ncomms1261
![]() |
[207] |
Liao WQ, Zhang Y, Hu CL (2015) A lead-halide perovskite molecular ferroelectric semiconductor. Nat Commun 6: 7338. https://doi.org/10.1038/ncomms8338 doi: 10.1038/ncomms8338
![]() |
[208] | Guyonnet J (2014) Domain walls in ferroelectric materials, In: Guyonnet J, Ferroelectric Domain Walls, Cham: Springer, 7–24. https://doi.org/10.1007/978-3-319-05750-7_2 |
[209] | Rakita Y (2016) CH3NH3PbBr3 is not pyroelectric, excluding ferroelectricenhanced photovoltaic performance. APL Mater 4: 051101. http://dx.doi.org/10.1063/1.4949760 |
[210] |
Landau L, Lifshitz E (1935) On the theory of the dispersion of magnetic permeability in ferromagnetic bodies. Phys Z Sowjetunion 8: 101–114. https://doi.org/10.1016/B978-0-08-036364-6.50008-9 doi: 10.1016/B978-0-08-036364-6.50008-9
![]() |
[211] |
Kittel C (1946) Theory of the structure of ferromagnetic domains in films and small particles. Phys Rev 70: 965–971. https://doi.org/10.1103/PhysRev.70.965 doi: 10.1103/PhysRev.70.965
![]() |
[212] | Guyonnet J (2014) Ferroelectric Domain Walls, Cham: Springer. https://link.springer.com/book/10.1007/978-3-319-05750-7 |
[213] |
Sherkar TS, Anton KLJ (2016) Can ferroelectric polarization explain the high performance of hybrid halide perovskite solar cells? Phys Chem Chem Phys 18: 331–338. https://doi.org/10.1039/C5CP07117H doi: 10.1039/C5CP07117H
![]() |
[214] | Nye JF (1985) Physical Properties of Crystals, Oxford: Oxford University Press. |
[215] | Lines ME, Glass AM (1979) Principles and Applications of Ferroelectrics and Related Materials, Oxford: Oxford University Press. https://doi.org/10.1093/acprof:oso/9780198507789.001.0001 |
[216] | Mitsui T, Tatsuzaki I, Nakamura E (1976) An Introduction to the Physics of Ferroelectrics, London: Gordon and Breach. |
[217] | Jaffe BC, Jaffe HW (1971) Piezoelectric Ceramics, New York: Academic Press, 313–338. https://doi.org/10.1016/B978-0-323-89952-9.00005-1 |
[218] |
Ballato A (1995) Piezoelectricity: Old effect, new thrusts. IEEE T Ultrason Ferr 42: 916–926. https://doi.org/10.1109/58.464826 doi: 10.1109/58.464826
![]() |
[219] |
Park H, Ha C, Lee JH (2020) Advances in piezoelectric halide perovskites for energy harvesting applications. J Mater Chem A 8: 24353–24367. https://doi.org/10.1039/d0ta08780g doi: 10.1039/d0ta08780g
![]() |
[220] |
Rashkeev S, El-Mellouhi F, Kais S, et al. (2015) Domain walls conductivity in hybrid organometallic perovskites and their essential role in CH3NH3PbI3 solar cell high performance. Sci Rep 5: 11467. https://doi.org/10.1038/srep11467 doi: 10.1038/srep11467
![]() |
[221] |
Ma J, Wang LW (2015) Nanoscale charge localization induced by random ori-entations of organic molecules in hybrid perovskite CH3NH3PbI3. Nano Lett 15: 248–253. https://doi.org/10.1021/nl503494y doi: 10.1021/nl503494y
![]() |
[222] |
Liu S, Zheng F, Grinberg I, et al. (2016) Photoferroelectric and photopiezoelectric properties of organometal halide perovskites. J Phys Chem Lett 7: 1460–1465. https://doi.org/10.1021/acs.jpclett.6b00527 doi: 10.1021/acs.jpclett.6b00527
![]() |
[223] |
Damjanovic D (1998) Ferroelectric, dielectric and piezoelectric properties of ferroelectric thin films and ceramics. Rep Prog Phys 61: 1267. https://doi.org/10.1088/0034-4885/61/9/002 doi: 10.1088/0034-4885/61/9/002
![]() |
[224] |
Sahoo A, Paul T, Makani NH, et al. (2022) High piezoresponse in low-dimensional inorganic halide perovskite for mechanical energy harvesting. Sustain Energ Fuels 6: 4484–4497. https://doi.org/10.1039/d2se00786j doi: 10.1039/d2se00786j
![]() |
[225] |
Butler KT, Frost JM, Walsh A (2015) Ferroelectric materials for solar energy conversion: Photoferroics revisited. Energy Environ Sci 8: 838–848. https://doi.org/10.1039/C4EE03523B doi: 10.1039/C4EE03523B
![]() |
[226] |
Chelil N, Sahnoun M, Benhalima Z, et al. (2023) Insights into the relationship between ferroelectric and photovoltaic properties in CsGeI3 for solar energy conversion. RSC Adv 13: 1955–1963. https://doi.org/10.1039/D2RA06860E doi: 10.1039/D2RA06860E
![]() |
[227] |
Zhang Y, Liu Y, Wang ZL (2011) Fundamental theory of piezotronics. Adv Mater 23: 3004–3013. https://doi.org/10.1002/adma.201100906 doi: 10.1002/adma.201100906
![]() |
[228] |
Hu Y, Chang Y, Fei P, et al. (2010) Designing the electric transport characteristics of zno micro/nanowire devices by coupling piezoelectric and photoexcitation effects. ACS Nano 4: 1234–1240. https://doi.org/10.1021/nn901805g doi: 10.1021/nn901805g
![]() |
[229] |
Hu Y, Zhang Y, Chang Y, et al. (2010) Optimizing the power output of a ZnO photocell by piezopotential. ACS Nano 4: 4220–4224. https://doi.org/10.1021/nn1010045 doi: 10.1021/nn1010045
![]() |
[230] |
He JH, Liu J, Chen LJ, et al (2007) Piezoelectric gated diode of a single ZnO nanowire. Adv Mater 19: 781. https://doi.org/10.1002/adma.200602918 doi: 10.1002/adma.200602918
![]() |
[231] |
Wang X, Zhou J, Song J, et al. (2006) Piezoelectric field effect transistor and nanoforce sensor based on a single ZnO nanowire. Nano Lett 6: 2768. https://doi.org/10.1021/nl061802g doi: 10.1021/nl061802g
![]() |
[232] |
Lao CS, Kaung Q, Wang ZL, et al (2007) Polymer functionalized piezoelectric-FET as humidity/chemical nanosensors. Appl Phys Lett 90: 262107. https://doi.org/10.1063/1.2748097 doi: 10.1063/1.2748097
![]() |
[233] |
Wang ZL, Song J (2006) Piezoelectric nanogenerators based on zinc oxide nanowire arrays. Science 312: 242. https://doi.org/10.1126/science.112400 doi: 10.1126/science.112400
![]() |
[234] |
Wang X, Song J, Liu J, et al. (2007) Direct-current nanogenerator driven by ultrasonic waves. Science 316: 102. https://doi.org/10.1126/science.1139366 doi: 10.1126/science.1139366
![]() |
[235] |
Qin Y, Wang X, Wang ZL (2008). Microfibre-nanowire hybrid structure for energy scavenging. Nature 451: 809. https://doi.org/10.1038/ncomms13146 doi: 10.1038/ncomms13146
![]() |
[236] |
Weber ZD (1978) CH3NH3PbX3 ein Pb(Ⅱ)-system mit kubischer perowskitstruktur/CH3NH3PbX3, a Pb(Ⅱ)-system with cubic perovskite structure. Naturforsch 33: 1443. https://doi.org/10.1515/znb-1978-1214 doi: 10.1515/znb-1978-1214
![]() |
[237] |
Poglitsch DWA (1987) Dynamic disorder in methylammoniumtrihalogenoplumbates(Ⅱ) observed by millimeter‐wave spectroscopy. J Chem Phys 87: 6373. https://doi.org/10.1063/1.453467 doi: 10.1063/1.453467
![]() |
[238] |
Frost JM, Butler KT, Brivio F, et al. (2014) Atomistic origins of high-performance in hybrid halide perovskite solar cells. Nano Lett 14: 2584. https://doi.org/10.1021/nl500390f doi: 10.1021/nl500390f
![]() |
[239] |
Frost JM, Butler KT, Walsh A (2014) Molecular ferroelectric contributions to anomalous hysteresis in hybrid perovskite solar cells. APL Mater 2: 081506. https://doi.org/10.1063/1.4890246 doi: 10.1063/1.4890246
![]() |
[240] |
Coll AGM, Marza EM, Almora O, et al. (2015) Polarization switching and light-enhanced piezoelectricity in lead halide perovskites. J Phys Chem Lett 6: 140 https://doi.org/10.1021/acs.jpclett.5b00502 doi: 10.1021/acs.jpclett.5b00502
![]() |
[241] |
Kim YJ, Dang TV, Choi HJ, et al. (2016) Piezoelectric properties of CH3NH3PbI3 perovskite thin films and their applications in piezoelectric generators. J Mater Chem A 4: 756. https://doi.org/10.1039/C5TA09662F doi: 10.1039/C5TA09662F
![]() |
[242] |
Shahrokhi S, Gao W, Wang Y, et al. (2020) Emergence of ferroelectricity in halide perovskites. Small Methods 4: 2000149. https://doi.org/10.1002/smtd.202000149 doi: 10.1002/smtd.202000149
![]() |
[243] |
Ding R, Liu H, Zhang X, et al. (2016) Flexible piezoelectric nanocomposite generators based on formamidinium lead halide perovskite nanoparticles. Adv Funct Mater 26: 7708. https://doi.org/10.1002/adfm.201602634 doi: 10.1002/adfm.201602634
![]() |
[244] |
Mohandes A, Moradi M, Nadgaran H (2021) Numerical simulation of inorganic Cs2AgBiBr6 as a lead-free perovskite using device simulation SCAPS-1D. Opt Quantum Electron 53: 319. https://doi.org/10.1007/s11082-021-02959-z doi: 10.1007/s11082-021-02959-z
![]() |
[245] |
Slavney AH, Leppert L, Bartesaghi D, et al. (2017) Defect-induced band-edge reconstruction of a bismuth-halide double perovskite for visible-light absorption. J Am Chem Soc 139: 5015–5018. https://doi.org/10.1021/jacs.7b01629 doi: 10.1021/jacs.7b01629
![]() |
[246] |
Wang F, Bai S, Tress W, et al. (2018) Defects engineering for high-performance perovskite solar cells. npj Flex Electron 2: 22. https://doi.org/10.1038/s41528-018-0035-z doi: 10.1038/s41528-018-0035-z
![]() |
[247] |
Han TH, Tan S, Xue J, et al. (2019) Interface and defect engineering for metal halide perovskite optoelectronic devices. Adv Mater 31: e1803515. https://doi.org/10.1002/adma.201803515 doi: 10.1002/adma.201803515
![]() |
[248] |
Xu J, Liu JB, Liu BX, et al. (2019) Defect engineering of grain boundaries in lead-free halide double perovskites for better optoelectronic performance. Adv Funct Mater 29: 1805870. https://doi.org/10.1002/adfm.201805870 doi: 10.1002/adfm.201805870
![]() |
[249] |
Ramesha R (2018) Defect engineering using crystal symmetry. PNAS 115: 9344–9346 https://doi.org/10.1073/pnas.1812554115 doi: 10.1073/pnas.1812554115
![]() |
[250] |
Samanta M, Ahmed SI, Chattopadhyay KK, et al. (2020) Role of various transport layer and electrode materials in enhancing performance of stable environment-friendly Cs2TiBr6 solar cell. Optik 217: 164805. https://doi.org/10.1016/j.ijleo.2020.164805 doi: 10.1016/j.ijleo.2020.164805
![]() |
[251] |
He Y, Xu L, Zheng H, et al. (2021) Numerical exploration of lead-free inorganic perovskite Cs2TiBr6 solar cell. J Phys Conf Ser 2021: 012069. https://doi.org/10.1088/1742-6596/2021/1/012069 doi: 10.1088/1742-6596/2021/1/012069
![]() |
[252] |
Jani MR, Islam MT, Al Amin SM, et al. (2020) Exploring solar cell performance of inorganic Cs2TiBr6 halide double perovskite: A numerical study. Superlattices Microstruct 146: 106652. https://doi.org/10.1016/j.spmi.2020.106652 doi: 10.1016/j.spmi.2020.106652
![]() |
[253] |
Sultana F, Jannat F, Ahmed S, et al. (2023) A comparative numerical approach between lead-free inorganic Cs2TiBr6 and Cs2PtI6-based perovskite solar cells. Results in Opt 13: 100567. https://doi.org/10.1016/j.rio.2023.100567 doi: 10.1016/j.rio.2023.100567
![]() |
[254] |
He Y, Zheng H, Huang S, et.al. (2021) Defect investigation of Ti-based vacancy-ordered double perovskite solar cell using SCAPS-1D. J Phys Conf Ser 2044: 012100. https://doi.org/10.1088/1742-6596/2044/1/012100 doi: 10.1088/1742-6596/2044/1/012100
![]() |
[255] |
Hussain I, Tran HP, Jaksik J, et al. (2018) Functional materials, device architecture, and flexibility of perovskite solar cell. Emergent Mater 1: 133–154. https://doi.org/10.1007/s42247-018-0013-1 doi: 10.1007/s42247-018-0013-1
![]() |
[256] |
Kopacic I, Friesenbichler B, Hoefler S, et al. (2018) Enhanced performance of germanium halide perovskite solar cells through compositional engineering. ACS Appl Energy Mater 1: 343–347. https://doi.org/10.1021/acsaem.8b00007 doi: 10.1021/acsaem.8b00007
![]() |
[257] |
Song Z, Watthage SC, Phillips AB, et al. (2016) Pathways toward high-performance perovskite solar cells: Review of recent advances in organo-metal halide perovskites for photovoltaic applications. JPE 6: 022001. https://doi.org/10.1117/1.JPE.6.022001 doi: 10.1117/1.JPE.6.022001
![]() |
[258] |
Tran TT, Pennella JR, Chamorro JR, et al. (2017) Designing indirect–direct bandgap transitions in double perovskites. Mater Horiz 4: 688. https://doi.org/10.1039/C7MH00239D doi: 10.1039/C7MH00239D
![]() |
[259] |
Giustino F, Snaith HJ (2016) Toward lead-free perovskite solar cells. ACS Energy Lett 1: 1233. https://doi.org/10.1021/acsenergylett.6b00499 doi: 10.1021/acsenergylett.6b00499
![]() |
[260] |
Sun Q, Wang J, Yin JW, et al. (2018) Bandgap engineering of stable lead-free oxide double perovskites for photovoltaics. Adv Mater 30: 1705901. https://doi.org/10.1002/adma.201705901 doi: 10.1002/adma.201705901
![]() |
[261] |
Du KZ, Wang X, Han Q, et al. (2017) Heterovalent B-site Co-alloying approach for halide perovskite bandgap engineering. ACS Energy Lett 2: 2486. https://doi.org/10.1021/acsenergylett.7b00824 doi: 10.1021/acsenergylett.7b00824
![]() |
1. | Mohit Singh, J. P. Tiwari, Solar Cells Based on Pb-Free and Perovskite-Inspired Materials: Futuristic of Perovskite Solar Cells, 2024, 2574-0962, 10.1021/acsaem.4c02047 | |
2. | Hayat Arbouz, Optimization Study of Single Junction Structures Utilizing 1.12 eV Cs2AuBiCl6 Double Perovskite: A Lead-Free Inorganic Absorber for Single and Tandem Solar Cell Applications, 2024, 1003, 1662-9795, 43, 10.4028/p-iIIR9q | |
3. | Taame Abraha Berhe, Etsana Kiros Ashebir, Wei-Nien Su, Bing Joe Hwang, Nano-engineering halide perovskites: towards energy harvesting, nano-plasmonic sensing and photoflexoelectric applications, 2025, 2753-1457, 10.1039/D4YA00442F |
Properties of low and high dimensional structures | |||
0D [136] | 1D [137,138] | 2D [139,140,141] | 3D [142,143,144] |
● Individual metal halide octahedral anions or metal halide clusters are completely surrounded and isolated by the organic cations. ● Molecular perovskite units are embedded in the crystal lattice. ● General chemical formula is A4BX6, where a represents monovalent organic cations and BX6 represents metal halide octahedra. ● Weaken the light absorption range and hinder charge transport. |
● The metal halide octahedral are corner-sharing, edge-sharing, or face-sharing to form a 1D nanowire. ● Either linear or zigzag configurations. ● Chemical formulas are variable depending on the connecting methods and the organic cations chosen. ● Weaken the light absorption range and hinder charge transport. ● High stability of low dimensional perovskites. |
● High stability of low dimensional perovskites. ● Suppress moisture invasion. ● Prevent moisture intrusion. ● Improve thermal stability. ● Suppress ion migration. ● The links between octahedra [BX6]4−are separated by large organic cations along crystallographic planes. ● The chemical formula is generally described as (A')m(A)n−1BnX3n+1. ● The 2D perovskite is more stable than its 3D phases. ● 2D perovskite-based PSCs do not exhibit ideal performance due to their larger bandgap, low carrier mobility and weaker carrier transport resulting from insulating spacer cations. |
● Superior efficiency of 3D perovskites. ● Consists of a network of corner-sharing BX6, where the B atom is a divalent metal cation (typically Ge2+, Sn2+ or Pb2+) and X is a monovalent anion (typically Cl−, Br−, I−). ● Sensitive to moisture invasion. ● Does not prevent moisture intrusion. ● The chemical formula is generally described as ABX3. ● The 3D perovskite is more unstable than its 2D phases. ● 3D perovskite-based PSCs exhibit ideal performance due to their narrow band gap, high carrier mobility and stronger carrier transport resulting from insulating spacer cations. |
Properties of low and high dimensional structures | |||
0D [136] | 1D [137,138] | 2D [139,140,141] | 3D [142,143,144] |
● Individual metal halide octahedral anions or metal halide clusters are completely surrounded and isolated by the organic cations. ● Molecular perovskite units are embedded in the crystal lattice. ● General chemical formula is A4BX6, where a represents monovalent organic cations and BX6 represents metal halide octahedra. ● Weaken the light absorption range and hinder charge transport. |
● The metal halide octahedral are corner-sharing, edge-sharing, or face-sharing to form a 1D nanowire. ● Either linear or zigzag configurations. ● Chemical formulas are variable depending on the connecting methods and the organic cations chosen. ● Weaken the light absorption range and hinder charge transport. ● High stability of low dimensional perovskites. |
● High stability of low dimensional perovskites. ● Suppress moisture invasion. ● Prevent moisture intrusion. ● Improve thermal stability. ● Suppress ion migration. ● The links between octahedra [BX6]4−are separated by large organic cations along crystallographic planes. ● The chemical formula is generally described as (A')m(A)n−1BnX3n+1. ● The 2D perovskite is more stable than its 3D phases. ● 2D perovskite-based PSCs do not exhibit ideal performance due to their larger bandgap, low carrier mobility and weaker carrier transport resulting from insulating spacer cations. |
● Superior efficiency of 3D perovskites. ● Consists of a network of corner-sharing BX6, where the B atom is a divalent metal cation (typically Ge2+, Sn2+ or Pb2+) and X is a monovalent anion (typically Cl−, Br−, I−). ● Sensitive to moisture invasion. ● Does not prevent moisture intrusion. ● The chemical formula is generally described as ABX3. ● The 3D perovskite is more unstable than its 2D phases. ● 3D perovskite-based PSCs exhibit ideal performance due to their narrow band gap, high carrier mobility and stronger carrier transport resulting from insulating spacer cations. |