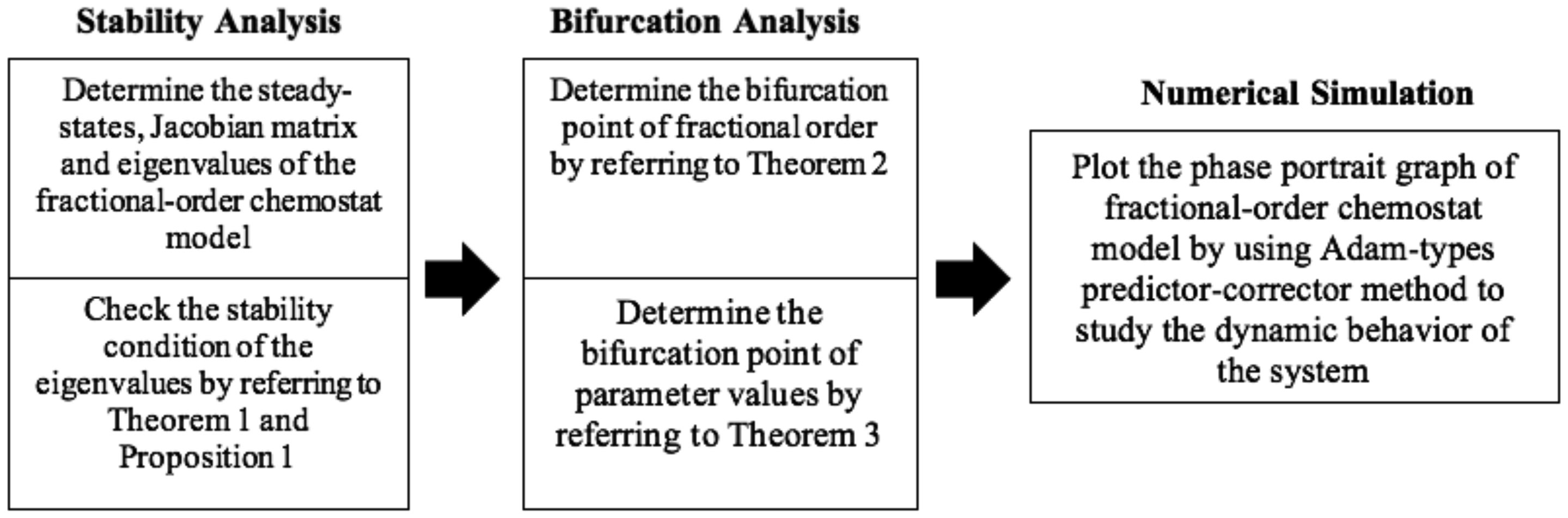
The issue of non-fragile sampled-data control for synchronizing Markov jump Lur'e systems (MJLSs) with time-variant delay was investigated. The time-variant delay allowed for uncertainty that was constrained to an interval with defined upper and lower boundaries. The components of the nonlinear function within the MJLSs were considered to satisfy either Lipschitz continuity or non-decreasing monotonicity. Numerically tractable conditions that ensured stochastic synchronization with a predefined L2−L∞ disturbance attenuation level for the drive-response MJLSs were established, utilizing time-dependent two-sided loop Lyapunov-Krasovskii functionals, together with integral and matrix inequalities. An exact mathematical expression of the desired controller gains can be obtained based on these conditions. Finally, an example with numerical simulation was employed to demonstrate the effectiveness of the proposed control strategies.
Citation: Dandan Zuo, Wansheng Wang, Lulu Zhang, Jing Han, Ling Chen. Non-fragile sampled-data control for synchronizing Markov jump Lur'e systems with time-variant delay[J]. Electronic Research Archive, 2024, 32(7): 4632-4658. doi: 10.3934/era.2024211
[1] | Shaimaa A. M. Abdelmohsen, D. Sh. Mohamed, Haifa A. Alyousef, M. R. Gorji, Amr M. S. Mahdy . Mathematical modeling for solving fractional model cancer bosom malignant growth. AIMS Biophysics, 2023, 10(3): 263-280. doi: 10.3934/biophy.2023018 |
[2] | Mati ur Rahman, Mehmet Yavuz, Muhammad Arfan, Adnan Sami . Theoretical and numerical investigation of a modified ABC fractional operator for the spread of polio under the effect of vaccination. AIMS Biophysics, 2024, 11(1): 97-120. doi: 10.3934/biophy.2024007 |
[3] | Yasir Nadeem Anjam, Mehmet Yavuz, Mati ur Rahman, Amna Batool . Analysis of a fractional pollution model in a system of three interconnecting lakes. AIMS Biophysics, 2023, 10(2): 220-240. doi: 10.3934/biophy.2023014 |
[4] | Marco Menale, Bruno Carbonaro . The mathematical analysis towards the dependence on the initial data for a discrete thermostatted kinetic framework for biological systems composed of interacting entities. AIMS Biophysics, 2020, 7(3): 204-218. doi: 10.3934/biophy.2020016 |
[5] | Mehmet Yavuz, Kübra Akyüz, Naime Büşra Bayraktar, Feyza Nur Özdemir . Hepatitis-B disease modelling of fractional order and parameter calibration using real data from the USA. AIMS Biophysics, 2024, 11(3): 378-402. doi: 10.3934/biophy.2024021 |
[6] | Larisa A. Krasnobaeva, Ludmila V. Yakushevich . On the dimensionless model of the transcription bubble dynamics. AIMS Biophysics, 2023, 10(2): 205-219. doi: 10.3934/biophy.2023013 |
[7] | Carlo Bianca . Differential equations frameworks and models for the physics of biological systems. AIMS Biophysics, 2024, 11(2): 234-238. doi: 10.3934/biophy.2024013 |
[8] | Mohammed Alabedalhadi, Mohammed Shqair, Ibrahim Saleh . Analysis and analytical simulation for a biophysical fractional diffusive cancer model with virotherapy using the Caputo operator. AIMS Biophysics, 2023, 10(4): 503-522. doi: 10.3934/biophy.2023028 |
[9] | Bertrand R. Caré, Pierre-Emmanuel Emeriau, Ruggero Cortini, Jean-Marc Victor . Chromatin epigenomic domain folding: size matters. AIMS Biophysics, 2015, 2(4): 517-530. doi: 10.3934/biophy.2015.4.517 |
[10] | David Gosselin, Maxime Huet, Myriam Cubizolles, David Rabaud, Naceur Belgacem, Didier Chaussy, Jean Berthier . Viscoelastic capillary flow: the case of whole blood. AIMS Biophysics, 2016, 3(3): 340-357. doi: 10.3934/biophy.2016.3.340 |
The issue of non-fragile sampled-data control for synchronizing Markov jump Lur'e systems (MJLSs) with time-variant delay was investigated. The time-variant delay allowed for uncertainty that was constrained to an interval with defined upper and lower boundaries. The components of the nonlinear function within the MJLSs were considered to satisfy either Lipschitz continuity or non-decreasing monotonicity. Numerically tractable conditions that ensured stochastic synchronization with a predefined L2−L∞ disturbance attenuation level for the drive-response MJLSs were established, utilizing time-dependent two-sided loop Lyapunov-Krasovskii functionals, together with integral and matrix inequalities. An exact mathematical expression of the desired controller gains can be obtained based on these conditions. Finally, an example with numerical simulation was employed to demonstrate the effectiveness of the proposed control strategies.
Numerous mathematical models have been established to predict and study the biological system. In the past four decades, there have been far-reaching research on improving cell mass production in chemical reactors [1]. The chemostat model is used to understand the mechanism of cell mass growth in a chemostat. A chemostat is an apparatus for continuous culture that contains bacterial populations. It can be used to investigate the cell mass production under controlled conditions. This reactor provides a dynamic system for population studies and is suitable to be used in a laboratory. A substrate is continuously added into the reactor containing the cell mass, which grows by consuming the substrate that enters through the inflow chamber. Meanwhile, the mixture of cell mass and substrate is continuously harvested from the reactor through the outflow chamber. The dynamics in the chemostat can be investigated by using the chemostat model [2].
Ordinary differential equations (ODEs) are commonly used for modelling biological systems. However, most biological systems behaviour has memory effects, and ODEs usually neglect such effects. The fractional-order differential equations (FDEs) are taken into account when describing the behaviour of the systems' equations. A FDEs is a generalisation of the ODEs to random nonlinear order [3]. This equation is more effective because of its good memory, among other advantages [4]–[8]. The errors occuring from the disregarded parameters when modelling of phenomena in real-life also can be reduced. FDEs are also used to efficiently replicate the real nature of various systems in the field of engineering and sciences [9]. In the past few decades, FDEs have been used in biological systems for various studies [5], [6], [10]–[17].
Since great strides in the study of FDEs have been developed, the dynamics in the chemostat can be investigated using the mathematical model of the chemostat in the form of FDEs. Moreover, there have been few studies on the expansion of the chemostat model with fractional-order theory. Thus, we deepen and complete the analysis on the integer-order chemostat model with fractional-order theory and discuss the stability of the equilibrium points of the fractional-order chemostat model. Next, the bifurcation analysis for the fractional-order chemostat model is conducted to identify the bifurcation point that can change the stability of the system. The analysis identifies the values of the fractional-order and the system parameters to ensure the operation of the chemostat is well-controlled.
Recently, there are many approaches to define fractional operators such as by Caputo, Riemann-Liouville, Hadamard, and Grunwald-Letnikov [3]. However, Caputo is often used due to its convenience in various applications [18]. Caputo is also useful to encounter an obstacle where the initial condition is done in the differential of integer-order [19]. In this paper, we applied Caputo derivative to define the system of fractional-order. The Caputo derivative for the left-hand side is defined as
where Г denotes the function of gamma, n is an integer, where
The Adams-type predictor-corrector method is one of the technique that have been proposed for fractional-order differential equations [19]–[21]. The Adams-type predictor-corrector method is a analysis of numerical algorithm that involves two basics steps: predictor and corrector. The predictor formula can be described as
meanwhile the corrector formula can be determined by
The predictor-corrector method is also called as the PECE (Predict, Evaluate, Correct, Evaluate) method [22]. The procedure of the predictor-corrector method can be explained as follows
(i) Calculate the predictor step,
(ii) Evaluate
(iii) Calculate the corrector step,
(iv) Evaluate
The procedure repeatedly predicts and corrects the value until the corrected value becomes a converged number [21]. This method is able to maintain the stability of the properties and has good accuracy. Moreover, this method also has lower computational cost than other methods [23]. The algorithm of the Adams-type predictor-corrector method proposed by [22] is shown in Appendix.
The conditions of stability for integer-order differential equations and fractional-order differential equations are different. Both systems could have the same steady-state points but different stability conditions [24], [25]. The stability condition for fractional-order differential equations can be stated by Theorem 1 and the Routh-Hurwitz stability condition as described by Proposition 1.
Theorem 1
Proposition 1
or
The stability theorem on the fractional-order systems and fractional Routh-Hurwitz stability conditions are introduced to analyze the stability of the model. The fractional Routh-Hurwitz stability conditions is specifically introduced for the eigenvalues of the Jacobian matrix that obtained in quadratic form. The proof of this proposition is shown in Appendix.
Bifurcation can be defined as any sudden change that occurs while a parameter value is varied in the differential equation system and it has a significant influence on the solution [27]. An unstable steady-state may becomes stable and vice versa. A slight changes in the parameter value may change the system's stability. Despite the steady-state point and the eigenvalues of the system of fractional-order are similar as the system of integer-order, the discriminant method used for the stability of the steady-state point is different. Accordingly, the Hopf bifurcation condition in the fractional-order system is slightly different as compared with the integer-order system.
Fractional order α can be selected as the bifurcation parameter in a fractional-order system, but this is not allowed in an integer-order system. The existence of Hopf bifurcation can be stated as in Theorem 2.
Theorem 2
(i) 1. The characteristic equation of chemostat system has a pair of complex conjugate roots,
(ii) Critical value
(iii)
Proof. Condition (i) is not easy to obtain due to the selected parameter's value. However, this condition can be managed under some confined conditions. In fact, the washout steady-state solution of the chemostat model has two negative real roots. The remaining two roots depend on the characteristic of the polynomial from the no-washout steady-state solution.
Condition (ii) can be satisfied with the existence of critical value α* and when
The integer system required p = 0 for the bifurcation's operating condition. For the fractional-order system, the operating condition of the system will change into
In studying the dynamic process of chemostat, the parameters such as
Theorem 3
(i) The characteristic equation of chemostat system has a pair of complex conjugate roots,
(ii) Critical value
(iii)
Proof. This theorem can be proved in the same way as Theorem 2. Therefore, condition (i) can be guaranteed. Condition (ii) can be satisfied with the existence of critical value
For condition (iii), the condition of
Firstly, determine the steady-states, Jacobian matrix and eigenvalues of the fractional-order chemostat model. The stability properties of the fractional-order chemostat model were estimated by using the stability and bifurcation analyses with FDEs by referring to Theorem 1 and Proposition 1. Then, determine the bifurcation point of fractional-order by referring to Theorem 2 and determine the bifurcation point of parameter values by referring to Theorem 3. Next, plot the phase portrait of fractional-order chemostat model by using Adam-types predictor-corrector method to study the dynamic behaviour of the system. Figure 1 depicts the flowchart of this research. This flowchart can be applied to all problems with suitable parameter values.
An integer-order chemostat model that considered a variable yield coefficient and the Monod growth model from [1] is studied in this section. The chemostat system can be written as
with the initial value of
Let Eq. (3.2) equal to zero in order to find the steady-state solutions
By solving Eq. (3.4), the following solutions are obtained
From
Hence, the solutions of steady-state for the chemostat model are
(i) Washout:
(ii) No Washout:
where
The steady-state solutions are physically meaningful if their components are positive. Therefore,
The solution of steady-state in Equation (3.8) represents the washout situation, where the cell mass is wholly removed from the reactor and where the substrate concentration is at the same stock as in the beginning. This state must always be unstable in order to ensure that the cell mass is able to grow in the chemostat. This is because the cell mass will be continuously removed from the chemostat if the washout steady-state is stable. The Jacobian matrix for the washout steady-state solution can be written as
The eigenvalues of this matrix are
The eigenvalues in
The eigenvalues of the Jacobian matrix in terms of the characteristic polynomial are
where
and
The eigenvalues of the no-washout steady-state solution were evaluated with Routh-Hurwitz condition in Proposition 1. Based on the eigenvalues in Eq. (3.15) and by referring to the study by [5], the eigenvalues' condition can be simplified as the following two cases
(i) If b>0 or equivalent to
(ii) If b<0 or equivalent to
Then, if
The parameter values of the fractional-order chemostat model are provided in Table 1. The initial substrate concentration, S0 and ρ were assumed as non-negative values to ensure that the steady-state solutions were physically meaningful. The stability diagram of the steady-state solutions is plotted in Figure 2.
Parameters | Description | Values | Units |
k | Saturation constant | 1.75 | gl−1 |
Q | Dilution rate | 0.02 | l2gr−1 |
µ | Maximum growth rate | 0.3 | h−1 |
γ | Constant in yield coefficient | 0.01 | – |
β | Constant in yield coefficient | 5.25 | lg−1 |
S0 | Input concentration of substrate | 1 | gl−1 |
The washout steady-state solution is stable if Q > 0 and
The steady-state solutions of the fractional-order chemostat model for the parameter values given in Table 1 are
(i) Washout:
(ii) No Washout:
The eigenvalues obtained from the washout steady-state solution are
and the eigenvalues from the no-washout steady-state solution are
Based on Eq. (3.22) to Eq. (3.25), these satisfied the first condition of Hopf bifurcation in Theorem 2. There exists a pair of complex conjugate roots and the other eigenvalues are negative real roots. The transversality condition as the third condition is also satisfied. The eigenvalues of the washout steady-state solution based on the chemostat system is not imaginary, and so there is no existence of Hopf bifurcation in the washout steady-state solution. According to Theorem 2, the critical value of the fractional-order as stated in the second condition can be obtained as
where
Value of p and q are obtained from
The running state of the fractional-order chemostat system when fractional order α at the Hopf bifurcation point is shown. The fractional-order chemostat system changed its stability once Hopf bifurcation occurred. Therefore, we conjecture that the system of fractional-order chemostat may be lost or gain its stability when the fractional order α is less than the Hopf bifurcation point, or
The initial concentration of the substrate, S0, was chosen as the control parameter, while fractional order α was fixed. The solutions of steady-state of the fractional-order chemostat model with S0 as the control parameter are
(i) Washout:
(ii) No Washout:
The eigenvalues obtained from the washout steady-state solution are
and the eigenvalues from the no-washout steady-state solution are
These satisfied the first condition of Hopf bifurcation in Theorem 3. There exist a pair of complex conjugate roots in terms of S0, and the other eigenvalues were negative real roots in terms of S0. The transversality condition as the third condition is also satisfied. According to Theorem 3, the critical value of the fractional order as stated in the second condition can be obtained as follows
By referring to the study by [18], Eq. (3.37) can also be calculated as
From the calculations, the critical value of the initial concentration of the substrate is
The change in the running state when the value of the initial substrate concentration passes through the Hopf bifurcation point is shown. The stability of the fractional-order chemostat system changed once Hopf bifurcation occurred. In
The Hopf bifurcation points of system of fractional-order chemostat and system of integer-order chemostat are different.
The stability analysis of the fractional-order chemostat model was conducted based on the stability theory of FDEs. The integer-order chemostat model was extended to the FDEs. There are two steady-state solutions obtained, which are washout and no-washout steady-state solutions. The Hopf bifurcation of the order of α occured at the solutions of steady-state when the Hopf bifurcation conditions is fulfilled. The results show that the increasing or decreasing the value of α may stabilise the unstable state of the chemostat system. Therefore, the running state of the fractional-order chemostat system is affected by the value of α. The Hopf bifurcation of the initial concentration of the substrate, S0, also occurred when the Hopf bifurcation condition is fulfilled. As the evidence from the phase portrait plots, increase the value of the initial substrate concentration may destabilise the stable state of the chemostat system. The value of the initial substrate should remain at
[1] |
U. Parlitz, L. O. Chua, L. Kocarev, K. S. Halle, A. Shang, Transmission of digital signals by chaotic synchronization, Int. J. Bifurcation Chaos, 2 (1992), 973–977. https://doi.org/10.1142/S0218127492000562 doi: 10.1142/S0218127492000562
![]() |
[2] |
L. Kocarev, U. Parlitz, General approach for chaotic synchronization with applications to communication, Phys. Rev. Lett., 74 (1995), 5028. https://doi.org/10.1103/PhysRevLett.74.5028 doi: 10.1103/PhysRevLett.74.5028
![]() |
[3] |
Q. Xie, G. Chen, E. M. Bollt, Hybrid chaos synchronization and its application in information processing, Math. Comput. Modell., 35 (2002), 145–163. https://doi.org/10.1016/S0895-7177(01)00157-1 doi: 10.1016/S0895-7177(01)00157-1
![]() |
[4] | X. Liao, P. Yu, Absolute Stability of Nonlinear Control Systems, Springer-Verlag, New York, 2008. https://doi.org/10.1007/978-1-4020-8482-9 |
[5] |
L. M. Pecora, T. L. Carroll, Synchronization in chaotic systems, Phys. Rev. Lett., 64 (1990), 821. https://doi.org/10.1103/PhysRevLett.64.821 doi: 10.1103/PhysRevLett.64.821
![]() |
[6] |
Z. Tang, J. H. Park, Y. Wang, J. Feng, Distributed impulsive quasi-synchronization of Lur'e networks with proportional delay, IEEE Trans. Cybern., 49 (2019), 3105–3115. https://doi.org/10.1109/TCYB.2018.2839178 doi: 10.1109/TCYB.2018.2839178
![]() |
[7] |
D. Xuan, Z. Tang, J. Feng, J. H. Park, Cluster synchronization of nonlinearly coupled Lur'e networks: Delayed impulsive adaptive control protocols, Chaos, Solitons Fractals, 152 (2021), 111337. https://doi.org/10.1016/j.chaos.2021.111337 doi: 10.1016/j.chaos.2021.111337
![]() |
[8] |
S. Shao, J. Cao, Y. Hu, X. Liu. Prespecified-time distributed synchronization of Lur'e networks with smooth controllers, Asian J. Control, 24 (2022), 125–136. https://doi.org/10.1002/asjc.2422 doi: 10.1002/asjc.2422
![]() |
[9] |
J. Yang, J. Huang, X. He, W. Yang, Bipartite synchronization of Lur'e network with signed graphs based on intermittent control, ISA Trans., 135 (2023), 290–298. https://doi.org/10.1016/j.isatra.2022.10.002 doi: 10.1016/j.isatra.2022.10.002
![]() |
[10] |
R. Kavikumar, R. Sakthivel, O. M. Kwon, B. Kaviarasan, Reliable non-fragile memory state feedback controller design for fuzzy Markov jump systems, Nonlinear Anal. Hybrid Syst., 35 (2020), 100828. https://doi.org/10.1016/j.nahs.2019.100828 doi: 10.1016/j.nahs.2019.100828
![]() |
[11] |
H. Ji, Y. Li, X. Ding, J. Lu, Stability analysis of boolean networks with Markov jump disturbances and their application in apoptosis networks, Electron. Res. Arch., 30 (2022), 3422–3434. https://doi.org/10.3934/era.2022174 doi: 10.3934/era.2022174
![]() |
[12] |
R. Sakthivel, H. Divya, A. Parivallal, V. T. Suveetha, Quantized fault detection filter design for networked control system with Markov jump parameters, Circuits Syst. Signal Process., 40 (2021), 4741–4758. https://doi.org/10.1007/s00034-021-01693-x doi: 10.1007/s00034-021-01693-x
![]() |
[13] |
H. Liu, J. Cheng, J. Cao, I. Katib, Preassigned-time synchronization for complex-valued memristive neural networks with reaction–diffusion terms and Markov parameters, Neural Networks, 169 (2024), 520–531. https://doi.org/10.1016/j.neunet.2023.11.011 doi: 10.1016/j.neunet.2023.11.011
![]() |
[14] | X. Zhou, S. Zhong, Delay-range-dependent exponential synchronization of Lur'e systems with Markovian switching, Int. J. Math. Comput. Sci., 4 (2010), 407–412. |
[15] | J. Zhou, B. Zhang, Master-slave synchronization of singular Lur'e time-delay systems with Markovian jumping parameters, in 2020 7th International Conference on Information, Cybernetics, and Computational Social Systems (ICCSS), Guangzhou, China, (2020), 164–169. https://doi.org/10.1109/ICCSS52145.2020.9336877 |
[16] |
X. Huang, Y. Zhou, M. Fang, J. Zhou, S. Arik, Finite-time H∞ synchronization of semi-Markov jump Lur'e systems, Mod. Phys. Lett. B, 35 (2021), 2150168. https://doi.org/10.1142/S0217984921501682 doi: 10.1142/S0217984921501682
![]() |
[17] |
J. Zhou, J. Dong, S. Xu, Asynchronous dissipative control of discrete-time fuzzy Markov jump systems with dynamic state and input quantization, IEEE Trans. Fuzzy Syst., 31 (2023), 3906–3920. https://doi.org/10.1109/TFUZZ.2023.3271348 doi: 10.1109/TFUZZ.2023.3271348
![]() |
[18] |
X. Li, X. Qin, Z. Wan, W. Tai, Chaos synchronization of stochastic time-delay Lur'e systems: An asynchronous and adaptive event-triggered control approach, Electron. Res. Arch., 31 (2023), 5589–5608. https://doi.org/10.3934/era.2023284 doi: 10.3934/era.2023284
![]() |
[19] |
S. Santra, M. Joby, M. Sathishkumar, S. M. Anthoni, LMI approach-based sampled-data control for uncertain systems with actuator saturation: application to multi-machine power system, Nonlinear Dyn., 107 (2022), 967–982. https://doi.org/10.1007/s11071-021-06995-y doi: 10.1007/s11071-021-06995-y
![]() |
[20] |
Y. Zhou, X. Chang, W. Huang, Z. Li, Quantized extended dissipative synchronization for semi-Markov switching Lur'e systems with time delay under deception attacks, Commun. Nonlinear Sci. Numer. Simul., 117 (2023), 106972. https://doi.org/10.1016/j.cnsns.2022.106972 doi: 10.1016/j.cnsns.2022.106972
![]() |
[21] |
Q. Li, X. Liu, Q. Zhu, S. Zhong, J. Cheng, Stochastic synchronization of semi-Markovian jump chaotic Lur'e with packet dropouts subject to multiple sampling periods, J. Franklin Inst., 356 (2019), 6899–6925. https://doi.org/10.1016/j.jfranklin.2019.06.005 doi: 10.1016/j.jfranklin.2019.06.005
![]() |
[22] |
T. Yang, Z. Wang, X. Huang, J. Xia, Sampled-data exponential synchronization of Markovian jump chaotic Lur'e systems with multiple time delays, Chaos, Solitons Fractals, 160 (2022), 112252. https://doi.org/10.1016/j.chaos.2022.112252 doi: 10.1016/j.chaos.2022.112252
![]() |
[23] |
C. Ge, X. Liu, Y. Liu, C. Hua, Synchronization of inertial neural networks with unbounded delays via sampled-data control, IEEE Trans. Neural Networks Learn. Syst., 35 (2024), 5891–5901. https://doi.org/10.1109/TNNLS.2022.3222861 doi: 10.1109/TNNLS.2022.3222861
![]() |
[24] |
Y. Ni, Z. Wang, X. Huang, Q. Ma, H. Shen, Intermittent sampled-data control for local stabilization of neural networks subject to actuator saturation: A work-interval-dependent functional approach, IEEE Trans. Neural Networks Learn. Syst., 35 (2024), 1087–1097. https://doi.org/10.1109/TNNLS.2022.3180076 doi: 10.1109/TNNLS.2022.3180076
![]() |
[25] |
Y. Liu, Y. Zhang, L. Liu, S. Tong, C. L. P. Chen, Adaptive finite-time control for half-vehicle active suspension systems with uncertain dynamics, IEEE/ASME Trans. Mechatron., 26 (2021), 168–178. https://doi.org/10.1109/TMECH.2020.3008216 doi: 10.1109/TMECH.2020.3008216
![]() |
[26] |
Y. Wang, C. Hua, P Shi, Improved admissibility criteria for Takagi-Sugeno fuzzy singular systems with time-varying delay, IEEE Trans. Fuzzy Syst., 31 (2023), 2966–2974. https://doi.org/10.1109/TFUZZ.2023.3240250 doi: 10.1109/TFUZZ.2023.3240250
![]() |
[27] | G. Yang, X. Guo, W. Che, W. Guan, Linear Systems: Non-Fragile Control and Filtering, CRC Press, 2013. https://doi.org/10.1201/b14766 |
[28] |
X. Chang, G. Yang, Nonfragile H∞ filter design for T–S fuzzy systems in standard form, IEEE Trans. Ind. Electron., 61 (2014), 3448–3458. https://doi.org/10.1109/TIE.2013.2278955 doi: 10.1109/TIE.2013.2278955
![]() |
[29] |
K. Liu, A. Seuret, Y. Xia, Stability analysis of systems with time-varying delays via the second-order Bessel–Legendre inequality, Automatica, 76 (2017), 138–142. https://doi.org/10.1016/j.automatica.2016.11.001 doi: 10.1016/j.automatica.2016.11.001
![]() |
[30] |
W. Tai, D. Zuo, J. Han, J. Zhou, Fuzzy resilient control for synchronizing chaotic systems with time-variant delay and external disturbance, Int. J. Mod. Phys. B, 35 (2021), 2150177. https://doi.org/10.1142/S0217979221501770 doi: 10.1142/S0217979221501770
![]() |
[31] |
N. T. T. Huyen, M. V. Thuan, N. T. Thanh, T. N. Binh, Guaranteed cost control of fractional-order switched systems with mixed time-varying delays, Comput. Appl. Math., 42 (2023), 370. https://doi.org/10.1007/s40314-023-02505-5 doi: 10.1007/s40314-023-02505-5
![]() |
[32] |
M. Sathishkumar, R. Sakthivel, C. Wang, B. Kaviarasan, S. M. Anthoni, Non-fragile filtering for singular Markovian jump systems with missing measurements, Signal Process., 142 (2018), 125–136. https://doi.org/10.1016/j.sigpro.2017.07.012 doi: 10.1016/j.sigpro.2017.07.012
![]() |
[33] |
X. Qin, J. Dong, X. Zhang, T. Jiang, J. Zhou, H∞ control of time-delayed Markov jump systems subject to mismatched modes and interval conditional probabilities, Arab. J. Sci. Eng., 49 (2024), 7471–7486. https://doi.org/10.1007/s13369-023-08332-4 doi: 10.1007/s13369-023-08332-4
![]() |
[34] |
D. Tong, B. Ma, Q. Chen, Y. Wei, P. Shi, Finite-time synchronization and energy consumption prediction for multilayer fractional-order networks, IEEE Trans. Circuits Syst. II Express Briefs, 70 (2023), 2176–2180. https://doi.org/10.1109/TCSII.2022.3233420 doi: 10.1109/TCSII.2022.3233420
![]() |
[35] |
L. Yao, Z. Wang, X. Huang, Y. Li, Q. Ma, H. Shen, Stochastic sampled-data exponential synchronization of Markovian jump neural networks with time-varying delays, IEEE Trans. Neural Networks Learn. Syst., 34 (2023), 909–920. https://doi.org/10.1109/TNNLS.2021.3103958 doi: 10.1109/TNNLS.2021.3103958
![]() |
[36] |
G. Yang, D. Tong, Q. Chen, W. Zhou, Fixed-time synchronization and energy consumption for Kuramoto-oscillator networks with multilayer distributed control, IEEE Trans. Circuits Syst. II Express Briefs, 70 (2023), 1555–1559. https://doi.org/10.1109/TCSII.2022.3221477 doi: 10.1109/TCSII.2022.3221477
![]() |
[37] |
L. Shanmugam, Y. H. Joo, Design of interval type-2 fuzzy-based sampled-data controller for nonlinear systems using novel fuzzy Lyapunov functional and its application to PMSM, IEEE Trans. Syst. Man Cybern.: Syst., 51 (2021), 542–551. https://doi.org/10.1109/TSMC.2018.2875098 doi: 10.1109/TSMC.2018.2875098
![]() |
[38] |
M. Sathishkumar, R. Sakthivel, F. Alzahrani, B. Kaviarasan, Y. Ren, Mixed H∞ and passivity-based resilient controller for nonhomogeneous Markov jump systems, Nonlinear Anal. Hybrid Syst., 31 (2019), 86–99. https://doi.org/10.1016/j.nahs.2018.08.003 doi: 10.1016/j.nahs.2018.08.003
![]() |
[39] |
X. Jiang, G. Xia, Z. Feng, T. Li, Non-fragile H∞ consensus tracking of nonlinear multi-agent systems with switching topologies and transmission delay via sampled-data control, Inf. Sci., 509 (2020), 210–226. https://doi.org/10.1016/j.ins.2019.08.078 doi: 10.1016/j.ins.2019.08.078
![]() |
[40] |
Z. Yan, D. Zuo, T. Guo, J. Zhou, Quantized H∞ stabilization for delayed memristive neural networks, Neural Comput. Appl., 35 (2023), 16473–16486. https://doi.org/10.1007/s00521-023-08510-3 doi: 10.1007/s00521-023-08510-3
![]() |
[41] |
T. H. Lee, J. H. Park, Improved criteria for sampled-data synchronization of chaotic Lur'e systems using two new approaches, Nonlinear Anal. Hybrid Syst., 24 (2017), 132–145. https://doi.org/10.1016/j.nahs.2016.11.006 doi: 10.1016/j.nahs.2016.11.006
![]() |
[42] |
W. Tai, D. Zuo, Z. Xuan, J. Zhou, Z. Wang, Non-fragile L2−L∞ filtering for a class of switched neural networks, Math. Comput. Simul., 185 (2021), 629–645. https://doi.org/10.1016/j.matcom.2021.01.014 doi: 10.1016/j.matcom.2021.01.014
![]() |
[43] |
J. Zhou, X. Ma, Z. Yan, S. Arik, Non-fragile output-feedback control for time-delay neural networks with persistent dwell time switching: A system mode and time scheduler dual-dependent design, Neural Networks, 169 (2024), 733–743. https://doi.org/10.1016/j.neunet.2023.11.007 doi: 10.1016/j.neunet.2023.11.007
![]() |
[44] |
G. Chen, J. Xia, J. H. Park, H. Shen, G. Zhuang, Sampled-data synchronization of stochastic Markovian jump neural networks with time-varying delay, IEEE Trans. Neural Networks Learn. Syst., 33 (2022), 3829–3841. https://doi.org/10.1109/TNNLS.2021.3054615 doi: 10.1109/TNNLS.2021.3054615
![]() |
[45] |
D. A. Wilson, Convolution and Hankel operator norms for linear systems, IEEE Trans. Autom. Control, 34 (1989), 94–97. https://doi.org/10.1109/9.8655 doi: 10.1109/9.8655
![]() |
[46] |
A. Seuret, F. Gouaisbaut, Hierarchy of LMI conditions for the stability analysis of time-delay systems, Syst. Control Lett., 81 (2015), 1–7. https://doi.org/10.1016/j.sysconle.2015.03.007 doi: 10.1016/j.sysconle.2015.03.007
![]() |
[47] |
H. Zeng, Y. He, M. Wu, J. She, New results on stability analysis for systems with discrete distributed delay, Automatica, 60 (2015), 189–192. https://doi.org/10.1016/j.automatica.2015.07.017 doi: 10.1016/j.automatica.2015.07.017
![]() |
[48] |
A. Seuret, K. Liu, F. Gouaisbaut, Generalized reciprocally convex combination lemmas and its application to time-delay systems, Automatica, 95 (2018), 488–493. https://doi.org/10.1016/j.automatica.2018.06.017 doi: 10.1016/j.automatica.2018.06.017
![]() |
[49] |
K. Zhou, P. P. Khargonekar, Robust stabilization of linear systems with norm-bounded time-varying uncertainty, Syst. Control Lett., 10 (1988), 17–20. https://doi.org/10.1016/0167-6911(88)90034-5 doi: 10.1016/0167-6911(88)90034-5
![]() |
[50] | S. Boyd, L. El. Ghaoui, E. Feron, V. Balakrishnan, Linear Matrix Inequalities in System and Control Theory, SIAM, Philadelphia, PA, USA, 1994. https://doi.org/10.1137/1.9781611970777 |
[51] | M. Wu, Y. He, J. She, Stability Analysis and Robust Control of Time-Delay Systems, Springer, New York, 2010. https://doi.org/10.1007/978-3-642-03037-6 |
1. | Xiaomeng Ma, Zhanbing Bai, Sujing Sun, Stability and bifurcation control for a fractional-order chemostat model with time delays and incommensurate orders, 2022, 20, 1551-0018, 437, 10.3934/mbe.2023020 |
Parameters | Description | Values | Units |
k | Saturation constant | 1.75 | gl−1 |
Q | Dilution rate | 0.02 | l2gr−1 |
µ | Maximum growth rate | 0.3 | h−1 |
γ | Constant in yield coefficient | 0.01 | – |
β | Constant in yield coefficient | 5.25 | lg−1 |
S0 | Input concentration of substrate | 1 | gl−1 |