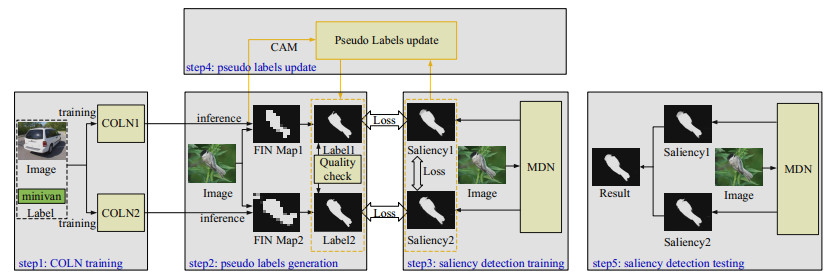
Parkinson's disease (PD) is characterized by the pathological accumulation of α-synuclein, which has driven extensive research into the role of exosomes in disease mechanisms. Exosomes are nanoscale vesicles enriched with proteins, RNA, and lipids that facilitate critical intercellular communication processes. Recent studies have elucidated the role of exosomes in transmitting misfolded proteins among neurons, which significantly impacts the progression of PD. The presence of disease-associated exosomes in cerebrospinal fluid and blood highlights their substantial diagnostic potential for PD. Specifically, exosomes derived from the central nervous system (CNS) have emerged as promising biomarkers because of their ability to accurately reflect pathological states. Furthermore, the isolation of exosomes from distinct brain cell types allows the identification of precise biomarkers, increasing diagnostic specificity and accuracy. In addition to being useful for diagnostics, exosomes hold therapeutic promise given their ability to cross the blood–brain barrier (BBB) and selectively modulate their cargo. These findings suggest that these materials could be used as delivery systems for therapeutic drugs for the treatment of neurodegenerative diseases. This review comprehensively examines the multifaceted roles of exosomes in PD pathogenesis, diagnosis, and treatment. It also addresses the associated clinical challenges and underscores the urgent need for further research and development to fully leverage exosome-based strategies in PD management.
Graphical abstract
Citation: Naeimeh Akbari-Gharalari, Maryam Ghahremani-Nasab, Roya Naderi, Leila Chodari, Farshad Nezhadshahmohammad. The potential of exosomal biomarkers: Revolutionizing Parkinson's disease: How do they influence pathogenesis, diagnosis, and therapeutic strategies?[J]. AIMS Neuroscience, 2024, 11(3): 374-397. doi: 10.3934/Neuroscience.2024023
[1] | Jing Zhou, Ze Chen, Xinhan Huang . Weakly perceived object detection based on an improved CenterNet. Mathematical Biosciences and Engineering, 2022, 19(12): 12833-12851. doi: 10.3934/mbe.2022599 |
[2] | Yu Li, Meilong Zhu, Guangmin Sun, Jiayang Chen, Xiaorong Zhu, Jinkui Yang . Weakly supervised training for eye fundus lesion segmentation in patients with diabetic retinopathy. Mathematical Biosciences and Engineering, 2022, 19(5): 5293-5311. doi: 10.3934/mbe.2022248 |
[3] | Wenli Cheng, Jiajia Jiao . An adversarially consensus model of augmented unlabeled data for cardiac image segmentation (CAU+). Mathematical Biosciences and Engineering, 2023, 20(8): 13521-13541. doi: 10.3934/mbe.2023603 |
[4] | Xin Shu, Xin Cheng, Shubin Xu, Yunfang Chen, Tinghuai Ma, Wei Zhang . How to construct low-altitude aerial image datasets for deep learning. Mathematical Biosciences and Engineering, 2021, 18(2): 986-999. doi: 10.3934/mbe.2021053 |
[5] | Peng Ying, Zhongnian Li, Renke Sun, Xinzheng Xu . Complementary label learning based on knowledge distillation. Mathematical Biosciences and Engineering, 2023, 20(10): 17905-17918. doi: 10.3934/mbe.2023796 |
[6] | Jing Zhang, Baoqun Yin, Yu Zhong, Qiang Wei, Jia Zhao, Hazrat Bilal . A two-stage grasp detection method for sequential robotic grasping in stacking scenarios. Mathematical Biosciences and Engineering, 2024, 21(2): 3448-3472. doi: 10.3934/mbe.2024152 |
[7] | Keying Du, Liuyang Fang, Jie Chen, Dongdong Chen, Hua Lai . CTFusion: CNN-transformer-based self-supervised learning for infrared and visible image fusion. Mathematical Biosciences and Engineering, 2024, 21(7): 6710-6730. doi: 10.3934/mbe.2024294 |
[8] | Junjing Cai, Qiwei Wang, Ce Wang, Yu Deng . Research on cell detection method for microfluidic single cell dispensing. Mathematical Biosciences and Engineering, 2023, 20(2): 3970-3982. doi: 10.3934/mbe.2023185 |
[9] | Vishal Pandey, Khushboo Anand, Anmol Kalra, Anmol Gupta, Partha Pratim Roy, Byung-Gyu Kim . Enhancing object detection in aerial images. Mathematical Biosciences and Engineering, 2022, 19(8): 7920-7932. doi: 10.3934/mbe.2022370 |
[10] | Jingyao Liu, Qinghe Feng, Yu Miao, Wei He, Weili Shi, Zhengang Jiang . COVID-19 disease identification network based on weakly supervised feature selection. Mathematical Biosciences and Engineering, 2023, 20(5): 9327-9348. doi: 10.3934/mbe.2023409 |
Parkinson's disease (PD) is characterized by the pathological accumulation of α-synuclein, which has driven extensive research into the role of exosomes in disease mechanisms. Exosomes are nanoscale vesicles enriched with proteins, RNA, and lipids that facilitate critical intercellular communication processes. Recent studies have elucidated the role of exosomes in transmitting misfolded proteins among neurons, which significantly impacts the progression of PD. The presence of disease-associated exosomes in cerebrospinal fluid and blood highlights their substantial diagnostic potential for PD. Specifically, exosomes derived from the central nervous system (CNS) have emerged as promising biomarkers because of their ability to accurately reflect pathological states. Furthermore, the isolation of exosomes from distinct brain cell types allows the identification of precise biomarkers, increasing diagnostic specificity and accuracy. In addition to being useful for diagnostics, exosomes hold therapeutic promise given their ability to cross the blood–brain barrier (BBB) and selectively modulate their cargo. These findings suggest that these materials could be used as delivery systems for therapeutic drugs for the treatment of neurodegenerative diseases. This review comprehensively examines the multifaceted roles of exosomes in PD pathogenesis, diagnosis, and treatment. It also addresses the associated clinical challenges and underscores the urgent need for further research and development to fully leverage exosome-based strategies in PD management.
Graphical abstract
Alzheimer's disease;
ALG2-interacting protein X;
autophagy–lysosome pathway;
amyotrophic lateral sclerosis;
atypical parkinsonian syndrome;
blood–brain barrier;
corticobasal degeneration;
central nervous system;
cerebrospinal fluid;
dementia with Lewy bodies;
exosomal transfer into cells;
frontotemporal dementia;
glucocerebrosidase (GBA);
β-glucocerebrosidase;
heat shock protein 70;
heat shock protein 90;
Huntington's disease;
intercellular adhesion molecule 1;
intraluminal vesicles;
idiopathic PD;
lymphocyte function-associated antigen 1;
long noncoding RNAs;
leucine-rich repeat kinase 2;
L1 cell adhesion molecule;
microtubule-associated protein;
microtubule-associated protein tau gene;
microRNAs;
multiple sclerosis;
multiple system atrophy;
mesenchymal stem cell;
multivesicular bodies;
olivopontocerebellar atrophy;
oxidized DJ-1;
Parkinson's disease;
PTEN-induced kinase 1;
progressive supranuclear palsy;
rapid eye movement sleep behavior disorder;
RNA interference;
Ser-1292-phosphorylated LRRK2;
shRNA minicircles;
small interfering RNAs;
alpha-synuclein gene;
striatonigral degeneration;
tumor susceptibility gene 101;
α-Synuclein
Salient object detection aims to simulate the human visual system for detecting regions that are most attractive, which is widely used in many computer vision tasks such as image segmentation [1,2], defect detection [3,4], object tracking [5], etc. Traditional methods detect salient object through hand-crafted features [6,7], which usually achieve low performance.
In recent years, deep neural networks (DNN) have achieved great progress in many related fields[8,9,10]. DNN has also been widely used in salient object detection[11,12,13,14,15]. Fully supervised methods [16,17,18] have shown satisfactory results while requiring expensive pixel-level annotation. An experienced annotator often takes several minutes to label one image, and the expensive labeling cost makes it difficult to train a model using large-scale labeled datasets.
Due to fully supervised methods relying on expensive pixel-wise annotation, researchers have considered weakly supervised salient object detection. The weak supervision mainly includes bounding box labeling [19], image category labeling [20,21,22], scribble labeling [23], etc. In these weakly supervised methods, a category annotation based model can be trained with existing large-scale classification datasets (e.g., ImageNet). It can largely reduce the annotation cost while satisfying the requirement for massive data and attract the attention of many researchers.
Category label based weakly supervised methods usually first obtain the coarse location of the foreground via classification network. This step can be implemented with class activation mapping (CAM) [24], while CAM often only provides the most discriminative object part. Wang et al.[25] proposed one method to jointly learn image feature and foreground mask, then performed mask on image feature, which is followed by classification, the classification task guide, the mask and segment foreground. To transform a spatial-dependent masked image feature to a position-independent classification feature, they integrated global max pooling (GMP) and global average pooling (GAP), which needs high computational cost. Since GMP only focuses on the most discriminative object part and often fails to discover the full objects, we propose one coarse object location network (COLN) that only uses GAP. The COLN uses the image classification dataset with category labels to jointly train a foreground inference network (FIN) and an image classification network and generate a FIN map, which means coarse foreground location. Additionally, to conquer the shortcoming of GAP, which may lead to overestimated object areas, we propose one method based on classification accuracy analysis to generate the optimal FIN map that covers the object region and excludes the background region.
In category label based weakly supervised methods, coarse object locations need some refinement to generate pixel-level pseudo-labels which are used to train saliency detection networks. The noise in pseudo-label is a key factor for model performance. We propose one quality check strategy to select high quality pseudo-labels. To this end, we use a slightly different model parameter to learn COLN twice and to obtain one pair of pseudo-labels, when calculating the consistency of pseudo-label pairs to select high quality labels. The high quality pseudo-labels are more robust to the choice of model parameters. A slight adjustment on a model parameter leads to less influence on high quality pseudo-labels and more influence on low quality pseudo-labels. The consistency calculation on two pseudo-labels with a slightly different model parameter is helpful to select high quality pseudo-labels.
Learning one robust saliency detection model from pseudo-labels is also one important step of the weakly supervised method. For this purpose, we propose one multi-decoder neural network (MDN) for saliency detection supervised by a pseudo-label pairs. The MDN is designed to integrate different saliency information from pseudo-label pairs generated by COLN. Each decoder branch is responsible for one pseudo-label. The loss of each decoder and consistency between decoders are both considered. Additionally, we also propose one pseudo-labels update strategy to further optimize the pseudo-labels and improve performance of saliency detection.
Performance evaluation on four public datasets shows that the proposed method outperforms other image category supervision based work.
The main contributions of this work include:
● We propose one COLN to roughly locate the object of an image.
● We propose one novel quality check strategy to generate high quality pseudo labels for saliency detection training.
● We propose one MDN for salient object detection, which is supervised by high quality pseudo-label pairs simultaneously.
● We propose one pseudo-labels update strategy, which iteratively optimizes the pseudo-labels and saliency detection model.
Over the past few decades, researchers have developed many methods for salient object detection. In the early days, researchers [26,27,28,29,30] focused more on traditional features (e.g., color, texture, contrast) to detect the salient region. Deep learning has achieved amazing results in computer vision in recent years, and subsequently, various saliency detection methods based on deep learning have been proposed. These methods mainly include fully supervised and weakly supervised methods.
The fully supervised method uses manual pixel-level annotation as a supervision signal to learn one saliency detection model.
Some methods focused on fusion multi-scale features and enhancing image boundaries to improve detection results. Tang et al.[31] decomposed the task into a low-resolution saliency classification network which aims to identify explicit image regions, and a high-resolution refinement network to precisely refine the saliency values of pixels in uncertain regions. Ma et al.[32] aimed to shrink pairwise aggregated neighboring feature nodes layer by layer so that the aggregated features fuse valid details and semantics together, meanwhile discarding distracting information. Song et al.[33] introduced an implicit function to simulate the equilibrium state of the feature pyramid at infinite depth, and they also proposed a differentiable edge extractor that directly extracts edges from the saliency masks. Zhuge et al.[34] aimed to learn more complete salient objects, aggregate multi-scale features and enhance salient objects. Wu et al.[35] proposed a dynamic convolutional kernel size to capture objects with different sizes. Wu et al.[36] employed an extreme downsampling technique to effectively learn a global view of the whole image and constructed an elegant decoder for recovering object details. Ma et al.[37] designed an enhanced wider field of sensation framework, which allows the network to achieve very significant improvements when dealing with objects with scale variations.
Recent attention mechanisms [38,39,40,41], which focus on important regions of an image, are also widely used in salient object detection tasks. Liu et al.[42] developed a new unified model based on pure transformers, which also improves the performance for high-resolution images. Wang et al.[43] proposed curiosity-driven network and fragment attention to generate enhanced detail-rich saliency maps based on curiosity. Xie et al.[44] designed a framework extracting features from images at different sizes and resolutions, as well as used transformers and convolutional neural network (CNN) backbone independently to achieve promised results on high-resolution images. Fan et al.[45] proposed a high-quality dataset and a data enhancement strategy to train the model to adapt to each different complex scene. Cheng et al.[46] proposed an extremely lightweight model that is about 0.2% parameters size of the current popular larger models and gains comparable performance. Tian et al.[47] designed a selective object saliency module and an object-context-object relation module to unify spatial attention and object-based attention for saliency ranking.
Although these fully supervised methods achieve satisfactory results, they require expensive and time-consuming pixel-level annotations.
To reduce the cost of image annotation, and enable larger datasets to be applied to salient object detection tasks, weakly supervised methods have attracted the interest of researchers.
Image category is one widely considered weak supervision for salient object detection. Wang et al.[25] designed one method that can only use image category annotation. They first divided the image classification task into two subtasks: Image foreground inference and foreground classification. In the image foreground inference task, they used a foreground inference network to obtain one roughly salient foreground, which was refined into pseudo-labels for the network training. This work alleviates the cost of annotation and allows the use of existing large-scale training sets with image-level labels. Li et al.[20] proposed to use the combination of a coarse salient object activation map from the classification network and saliency maps generated from unsupervised methods as pixel-level annotation, and they developed a simple yet very effective algorithm to train fully convolutional networks for salient object detection supervised by these noisy annotations. The algorithm is based on alternately exploiting a graphical model and training a fully convolutional network for model updating. Piao et al.[21] proposed a multi-filter directive network including a saliency network, as well as multiple directive filters. The directive filter is designed to extract and filter more accurate saliency cues from the noisy pseudo labels. The multiple accurate cues from multiple directive filters are then simultaneously propagated to the saliency network with a multi-guidance loss. Piao et al.[22] observed pseudo-labels converted from image-level classification labels always containing noise information and designed a noise-robust adversarial learning framework and a noise-sensitive training strategy to mitigate this problem. Tian et al.[48,49] proposed a novel weakly supervised network with category and subitizing labels for salient instance detection problems.
However, compared to full supervision, image category labeling loses most of the detailed information. Therefore, other researchers have also considered other forms of weak annotation. Zhang et al.[23] trained one network using scribble labeling and designed an edge detection module and an edge-structure-aware module to complement the scribble annotation. Liu et al.[19] proposed a novel weakly supervised method by bounding boxes annotations. They first take the unsupervised methods to generate initial saliency maps and address the over/under prediction problems to obtain the initial pseudo-labels, then iteratively refine the initial labels by learning a multitask map refinement network with saliency bounding boxes. Liang et al.[50] also used bounding boxes annotations on salient object detection in light fields and proposed a fused attention module to utilize light field data from multi-view. Zheng et al.[51] introduced saliency subitizing as the weak supervision. They proposed a saliency subitizing module to generate the initial saliency masks using the subitizing information and a saliency updating module to iteratively refine the generated saliency masks. Liu et al.[52] introduced a label decoupling siamese network to more adequately use the scribble labels and the complementary relationship between salient objects and backgrounds. Zeng et al.[53,54] introduced a unified framework and learned image saliency from category labels, captions, web images, and untagged images, respectively. They designed a classification network and a title production network to predict object categories and generate titles, respectively, while highlighting potential foreground regions. Both networks are also encouraged to detect generally salient regions rather than task-specific regions. They then used predicted foreground regions to generate pseudo-labels to train a saliency detection network.
Although these weakly supervised methods achieved some progress, they still suffer from inaccurate detection of salient objects.
The framework of proposed method is shown in Figure 1, which contains five steps:
1) Training COLN. We use the image classification dataset with category labels to train a FIN jointly with an image classification network and propose one method to generate the optimal FIN map, which covers the object region and excludes the background.
2) Generation high quality pixel-level pseudo-labels on training dataset of saliency detection. We perform COLN inference on training data to obtain coarse object location followed by GrabCut, then adopt one novel quality check strategy to generate high quality pseudo-labels pair.
3) Training MDN for salient object detection, which is supervised by a high quality pseudo-labels pair simultaneously. The MDN network consists of an encoder and two decoders, and each decoder and encoder forms a U-shaped structure.
4) Pseudo-labels update, which further declines impact of pseudo-labels noise and learns a more robust model.
5) Testing salient object detection. In the testing mode, the average of two saliency results predicted by MDN is regarded as the final saliency.
The salient region is often represented by the foreground of an image in classification task. To segment the foreground of an image by a classification task, inspired by [25], one COLN is first proposed, as shown in Figure 2. Given one image with category label, the image is encoded with shared convolutional layers then fed forward through a full convolutional network (FCN) and an FIN to obtain the image feature map and the approximate foreground mask, respectively.
Specifically, we adopt the shared convolutional layers on top of the 16-layer VGG[58] network, including 13 convolutional layers with rectified linear unit (ReLU) nonlinear interleaved layers and four max-pooling layers. FCN and FIN are two sibling sub-networks built on top of the shared layers. The FCN includes a convolutional layer, a batch normalization (BN) layer and a ReLU layer to generate feature map F with 512 channels. The FIN consists of a convolutional layer, a BN and a sigmoid layer to yield a saliency mask M with single channel, which is used to obtain a masked feature map as follows:
ˆFk=Fk⊙M, | (3.1) |
where Fk denotes the k-th channel of the feature map obtained by FCN and ˆFk is the k-th channel of the masked feature map ˆF. The ⊙ means the element point multiplication.
One GAP layer is used to aggregate the masked feature map ˆF into a 512-dimensional image-level feature, which is then passed through a fully connected layer and softmax operation to generate the category probabilities.
To prevent FIN from simply having high responses at all locations, a sparse regularization term is added to the loss function to penalize the high response of FIN on the background. Given a training set {Xi,li}Ni=1 including N sample pairs (images Xi and label li), the loss function of this network consists of two parts as follows:
L=−1NN∑i=1[∑k∈lilog(pk(Xi))+∑k∉lilog(1−pk(Xi))−λ‖M(Xi)‖1], | (3.2) |
where the first and second terms are cross-entropy loss to measure classification accuracy, the third term is the L1 regularization on the saliency mask M predicted by FIN and pk(Xi) is the outputed probability of k-th category.
In loss function (3.2), λ is a predefined weight parameter to control the FIN map size. A larger λ will result in more constraint on the response area of FIN, which will tend to detect incomplete objects. In contrast, smaller λ reduces the constraint on the FIN response area, which allows FIN to overestimate the object and brings the risk of high response on background. One example of FIN map with different λ is shown in Figure 3. The optimal parameter λ is designed to get the optimal FIN map, which covers the object region and excludes the background.
In this work, we propose one method to find the optimal parameter λ over the entire dataset. We carefully analyze the relationship between parameter λ and the classification accuracy. The top-1 classification accuracy against parameter λ is shown in Figure 4. When λ value is very small, the constraint on the FIN response area is limited and the generated FIN contains both object and part of the background, which leads to low classification accuracy. As the parameter λ increases gradually, more penalty is carried on the FIN response area and the generated FIN mainly contains object and excludes the background, which leads to higher classification accuracy. However, if the parameter λ value is too large, increasing penalty on the FIN response area results in the generated FIN only containing incomplete objects and providing lower classification accuracy. Intuition, the optimal parameter λ, which is designed to get the optimal FIN map that covers the object region and excludes the background, obtains the highest classification accuracy. From Figure 4, the optimal parameter λ is set to 2.0×10−5.
We train COLN classification network on one image classification dataset with category labels, then use the COLN network to perform inference on one image without any annotation and obtain its FIN map as the coarse object location.
Our proposed COLN is related to the network proposed by [25], while it exists two main differences:
1) Different global feature aggregation. In weak supervision with category-level labels, some form of global pooling is required to transform a spatial related image feature to a position-independent category feature. Both GMP and GAP have been intensively investigated in the literature. GMP only focuses on the most discriminative object part and often fails to discover the full objects. Zhou et al.[24] holds that GMP is not a suitable solution for the segmentation problem. In contrast, GAP encourages the network to have the same response at all positions and leads to overestimated object areas. The previous work WSS[25] uses a global smooth pooling (GSP) method, which integrates GAP and GMP, while the integration of two feature aggregation methods increases computational cost. Aiming at the deficiency of the method, we only use GAP in the proposed method. To avoid the shortcoming of GAP, which tends to overestimate object areas, we propose one method to calculate the optimal parameter λ for the FIN map which covers the object region and excludes the background.
2) Double COLN training strategy. Locating the foreground of an image by a classification task inevitably exists noise and leads to a significant performance drop. To conquer this problem, we train COLN twice with a slightly different parameter λ and follow with a quality check to select high quality results (presented in Section 3.2), while the network for FIN generation in WSS[25] is only trained once and is difficult to eliminate noise.
We trained the COLN classification network on one image classification dataset with category labels in Section 3.1, then used COLN to perform inference on the saliency training dataset DUTS-TR [25] and obtained the FIN map of each image as coarse object location. The generated coarse object location via the FIN map only has a 16 × 16 resolution, which not only lacks detail structure but also exists noise. We further adopt the unsupervised image segmentation algorithm GrabCut [55,56] to generate a pixel-level pseudo-label, and propose one quality check strategy to select high quality pseudo-labels.
GrabCut [55] is one efficient interactive object segmentation method. In this work, the coarse FIN map can be regarded as one interaction condition for GrabCut. Given one image with the FIN map, we regard the mean map value as one threshold. For each pixel, the map value larger (smaller) than the threshold will be initialized with probable foreground (probable background). Based on this initial label, GrabCut algorithm estimates the color distribution of the foreground and background via a Gaussian mixture model, constructs a graph over the entire image and applies a graph cut [56] optimization to achieve the segmentation result as pseudo-labels. Two segmentation examples are shown in step two of Figure 1.
The generated pseudo-labels with the FIN map and GrabCut still have noise and reduce the saliency detection performance. One solution is to select high quality pseudo-labels and remove low quality parts. To this end, we learn COLN is twice followed by GrabCut refinement, the parameter λ is selected as the optimal value 2.0×10−5 and one approximately optimal value 2.5×10−5 to obtain one pair pseudo-labels, respectively. The slightly different selection on λ leads to these pair results as not entirely equal. For each image I, it generates one pair pseudo-label Y1 and Y2. We find that Y1 and Y2 are often consistent if they both are high quality results and Y1 and Y2 are often largely different if they contain low quality results. We calculate intersection over union (IOU) of the pseudo-label pairs as their consistency measure:
IOU=|Y1∩Y2||Y1∪Y2|, | (3.3) |
where || means the area of the pseudo-label. Some examples are shown in Figure 5. In the top two rows, the pseudo-labels pair contains low quality results and leads to a low IOU. In the bottom two rows, the high quality pseudo-labels pair leads to high IOU.
To further analyze the relationship between IOU and quality pseudo-labels, for the pseudo-label pairs of each image in saliency training dataset DUTS-TR [25], we calculate their IOU and average root mean absolute error (MAE) to indicate absolute difference between pseudo-label pairs and ground-truths. The pseudo-labels are divided into five groups according to IOU (0.0–0.2, 0.2–0.4, etc.) and the image number and average MAE of each group is shown in Table 1. From this statistical result, in general we can find the pseudo-labels with larger IOU ddemonstrating lower MAE, which means higher quality; the conclusion is consistent with Figure 5. Additionally, we can find the pseudo-labels with IOU value in 0.6–0.8, and 0.8–1.0 show comparable MAE and occupy about 87% volume of the dataset. On the other hand, the pseudo-labels with IOU value less than 0.6 show significant deterioration in MAE.
IOU | Image Num | MAE |
0.8–1.0 | 5871 | 0.098 |
0.6–0.8 | 3338 | 0.110 |
0.4–0.6 | 125 | 0.173 |
0.2–0.4 | 403 | 0.171 |
0.0–0.2 | 816 | 0.201 |
Based on the above discussion, the pseudo-label pairs with high IOU probably are high quality pseudo-labels, otherwise they contain low quality pseudo-labels. Inspired by this intuition, we compute the IOU of each pseudo-labels pair. The pseudo-labels with IOU larger than one threshold θ (e.g., 0.6) will be regarded as high quality pseudo-labels to train salient object detection model.
To implement robust saliency detection, we propose MDN, which is supervised by a high quality pseudo-labels pair simultaneously.
The proposed network consists of an encoder and two decoders, which is shown in Figure 6. The input image is first encoded by one shared encoder (e.g., VGGNet-16) and then passed through pyramid pooling module (PPM) [57] to capture important global information of the input image. Taking the VGGNet version of the shared encoder as an example, encoded feature maps corresponding to C = {C2, C3, C4, C5} in the pyramid have downsampling rates of two, four, eight and 16 compared to the size of the input image, respectively. For each decoder branch, the top feature C5 is first sent through the feature aggregation module (FAM) [57] to fuse the coarse and fine level semantic features, then upsampled by factor two, concatenated with upsampled PPM and feature C4 and finally passed through a 3*3 convolution layer and get a fused feature. The operation on this fused feature is similar to C5. This process will be repeated four times and predict one saliency map. The loss of MDN is defined as:
L=2∑k=1Lbce(Pk,Yk)+δ×Lss(P1,P2), | (3.4) |
where Lbce is the binary cross entropy loss (BCE-loss) on one decoder branch, Lss is the similarity loss between two decoders and δ is weight parameter.
Lbce(Pk,Yk)=−1nn∑i[yki∗logpki+(1−yki)∗log(1−pki)], | (3.5) |
Lss(P1,P2)=1nn∑i(p1i−p2i)2, | (3.6) |
where pki and yki represent the elements of the decoder predictions Pk and its pseudo-labels Yk.
Following the earlier work [57], we also jointly train salient object detection and edge detection to further improve performance. We add an extra edge prediction branch built upon each decoder branch to estimate the boundaries of the salient objects, which is shown on the top and bottom side of Figure 6. Based on the FAM [57], we add three residual blocks followed by a 16-channel 3 × 3 convolutional layer for feature compression and a one-channel 1 × 1 convolutional layer for edge prediction. We also concatenate these three 16-channel 3 × 3 convolutional layers and feed them to three consecutive 3 × 3 convolutional layers with 48 channels to transmit the captured edge information to the salient object detection decoder branch for detail enhancement.
The proposed dual decoder architecture adopts two high quality pseudo-labels for model learning. However, in this straightforward approach, the noise in pseudo-labels still may exist and lead to a decline of performance. To conquer the impact of noise, we propose one pseudo-labels update strategy to learn a more robust model.
As described in the previous section, we adopt the COLN classification network to perform inference on saliency training dataset DUTS-TR [25], followed by GrabCut and a high quality pseudo-labels selection strategy to get high quality pseudo-labels, then train an MDN saliency detection network. Given one training image, its average of pseudo-labels pair (Y1 and Y2) is denoted as YA and its average of predictions by MDN (P1 and P2) is denoted as PA. The previous work WSS[25] found that in classification network, the average of the score map across all the channels followed by refinement can also be regarded as one saliency map, which is denoted as RCAM. MAE () is the mean absolute difference between the two saliency maps. Rfn is the pixel-level refinement algorithm GrabCut.
We first compare the average pseudo-labels YA and the average predicted saliency PA. Their MAE is lower than one small threshold α, meaning they both are confident. We regard their average as one updated pseudo-label and regard the further result with Rfn refinement as another updated pseudo-label. If the MAE is higher than one large threshold β, it indicates that the pseudo-labels are probably wrong and should be discarded from the training dataset. In other cases, we regard RCAM as reference, select one more reliable result from YA and PA as one updated pseudo-label and regard the further result with Rfn refinement as another updated pseudo-label. The update strategy is shown in Algorithm 1 and α and β are experimentally set to 15 and 40, respectively.
The training of the MDN saliency detection network and pseudo-label update strategy is alternately carried to improve the performance. We regard pseudo-labels as supervision for the training saliency detection model, then use this trained model and Algorithm 1 to refine pseudo-labels, iteratively carrying the above steps until the result converges.
Algorithm 1 Pseudo label update strategy |
Input: initial pseudo-labels pair Y1 and Y2, MDN predicted saliency pair P1 and P2, classification activated map RCAM
Output: updated pseudo-labels pair Y′1 and Y′2 1: YA=(Y1+Y2)/2 2: PA=(P1+P2)/2 3: if MAE(YA,PA)<α then 4: Y′1=(PA+YA)/2 5: Y′2=Rfn((PA+YA)/2) 6: else if MAE(YA,PA)>β then 7: Remove image and pseudo-labels from training data 8: else if MAE(YA,RCAM)>MAE(PA,RCAM) then 9: Y′1=PA 10: Y′2=Rfn(PA) 11: else 12: Y′1=YA 13: Y′2=Rfn(YA) 14: end if |
The testing or inference of the saliency detection model is carried on the MDN network. Given one input image, MDN predicts two saliency results using two decoder branches and we simply adopt an average of two saliency outputs as a final saliency.
In this section, we first present the implementation details of the proposed method, then compare the performance with the state of the arts methods on four datasets and finally carry an ablation study to prove the effectiveness of our method.
COLN: The weight parameters of the shared layer are initialized using the weight parameters of the pre-trained VGG model[58], while the weights of the other layers are initialized randomly. All input images are downsampled to a resolution of 256 × 256. To increase the training samples, we use random rotation and flipping data augmentation methods. We use small batch stochastic gradient descent (SGD) to minimize the loss function with a batch size of 64 and a momentum of 0.9. The learning rate is initialized to 0.01 and every 20 cycles decrease by a factor of 0.1. The parameter λ in Eq (3.2) is set to 2.0×10−5 and 2.5×10−5 to generate one pair of pseudo-labels. The IOU threshold parameter θ for high quality pseudo-labels selection is set to 0.6.
The training of this classification network includes two stages. In the first stage, we remove the FIN branch and only learn the parameters of the shared encoder, FCN and classification. In the second stage, we freeze the shared encoder, FCN and classification, then add the FIN branch and learn the parameters of the FIN branch. The training is carried on the NVIDIA RTX3090i hardware platform. The first stage trains 40 epochs consuming 30 hours and the second stage trains 10 epochs consuming 10 hours.
MDN: The training uses an Adam optimizer with weights decaying to 5×10−4 and an initial learning rate of 5×10−5, divided by 10 after 15 epochs. The network was trained for a total of 24 epochs consuming four hours on the NVIDIA RTX3090i hardware platform. The backbone parameters of our network VGG-16 were initialized with the corresponding models pre-trained on the ImageNet dataset, and the rest of the models were initialized randomly. The parameter δ in Eq (3.4) is selected with two.
Train data: Compared with image classification datasets, which have only one annotation class per object, the object detection datasets usually contain multiple objects of different classes and are more suitable for the saliency detection task. Following previous work WSS [25], the training of the COLN classification network is carried on the ImageNet object detection datasets, including 456 k objects on more than 200 object categories. Note that we only use the category labels and discard bounding box annotations.
For salient object detection model MDN, following the existing weakly supervised methods, we take DUTS-TR [25] as training set, which contains 10,553 images. Although the training set already has pixel-level annotation, we do not use pixel-level annotation.
Test data: The performance comparison was carried on a test set of four public datasets: DUTS-TE [25], ECSSD [6], PASCAL-S [59] and HKU-IS [60]. The DUTS-TE dataset contains 5019 test images, which contain important scenes for saliency detection. The ECSSD dataset contains more salient objects under complex scenes. The PASCAL-S dataset contains 850 natural images with multiple complex objects and cluttered backgrounds. The HKU-IS dataset contains 4447 images with high-quality pixel-wise annotations.
To compare the effectiveness of these different algorithms, this work adopts two widely used evaluation metrics:
Maximum F-measure (Fβ): The performance metric is calculated by the weighted harmonic of the precision and recall as below:
Fβ=(1+β2)×P×Rβ2×P+R, | (4.1) |
where β is set as 0.3 to raise more importance on precision.
MAE: The difference between the saliency map S and the ground-truth G is shown below:
MAE=1W×HW∑x=1H∑y=1|S(x,y)−G(x,y)|, | (4.2) |
where W and H are width and height of input image, respectively.
We compare our approach with the state of the arts weakly supervised methods, which are shown in Table 2. To make fair comparison, we use the implementation or inference results provided by the authors. Our method is based on image category supervision, compared with other image category supervision based work like WSS[25], ASMO[20], MSW [53], MFNet [21] and NSAL[22], and our method demonstrates significantly better performance on all datasets except a slightly worse MAE performance in the PASCAL-S dataset. Compared with the bounding boxes supervision based work WSB[19], we also show slightly improved performance when compared with the scribble supervision based works SAE[23] and LDS[52]. Compared with the subitizing supervised method SOS[51], we show better MAE and a slightly worse FMeasure. Compared with fully supervised methods[62,63,64], our method shows worse performance, while fully supervised methods need a large number of pixel-level annotations.
Supervision | Method | DUTS-TE | PASCAL-S | ECSSD | HKU-IS | |||||||
Max-F↑ | MAE↓ | Max-F↑ | MAE↓ | Max-F↑ | MAE↓ | Max-F↑ | MAE↓ | |||||
Fully supervised | SAMNet[61] | 0.836 | 0.058 | 0.856 | 0.113 | 0.927 | 0.051 | 0.914 | 0.044 | |||
PAGRN[62] | 0.778 | 0.055 | 0.765 | 0.151 | 0.871 | 0.064 | 0.863 | 0.045 | ||||
PFAN[63] | 0.764 | 0.060 | 0.754 | 0.137 | 0.875 | 0.046 | 0.871 | 0.042 | ||||
UCF[64] | 0.663 | 0.112 | 0.787 | 0.140 | 0.844 | 0.070 | 0.818 | 0.062 | ||||
Bounding-box | WSB[19] | 0.736 | 0.079 | - | - | 0.860 | 0.072 | 0.853 | 0.058 | |||
Subitizing | SOS[51] | - | - | 0.803 | 0.131 | 0.858 | 0.108 | 0.882 | 0.080 | |||
Scribble | SAE[23] | 0.746 | 0.062 | 0.788 | 0.139 | 0.865 | 0.061 | 0.857 | 0.047 | |||
LDS[52] | 0.755 | 0.066 | 0.793 | 0.094 | 0.873 | 0.056 | 0.860 | 0.048 | ||||
Category | WSS[25] | 0.630 | 0.099 | 0.697 | 0.184 | 0.823 | 0.109 | 0.821 | 0.084 | |||
ASMO[20] | 0.614 | 0.116 | 0.752 | 0.145 | 0.837 | 0.112 | 0.846 | 0.088 | ||||
MSW[53] | 0.684 | 0.091 | 0.713 | 0.133 | 0.840 | 0.096 | 0.814 | 0.084 | ||||
MFNet[21] | 0.710 | 0.076 | 0.751 | 0.115 | 0.854 | 0.084 | 0.851 | 0.059 | ||||
NSAL[22] | 0.729 | 0.074 | 0.753 | 0.111 | 0.853 | 0.081 | 0.859 | 0.053 | ||||
Ours | 0.749 | 0.067 | 0.785 | 0.125 | 0.871 | 0.063 | 0.861 | 0.049 |
A few saliency quality comparison examples are shown in Figure 7. Our method achieves promised results in both simple and complex scenes. Compared to WSS[25] and ASMO[20], which both adopt a network that relies on a single pseudo-label training, and MF-Net[21], which only relies on different refinement algorithms to obtain multiple pseudo-labels, our method can greatly reduce the noise of pseudo-labels and, thus, achieves better results.
To further validate the effectiveness of our method, we carry an evaluation on the DUTS-TE [25] dataset with different training strategies, which are shown in Table 3.
Metrics | MDN-SP | MDN-MP | MDN-Edge | MDN-Update |
Max-F↑ | 0.675 | 0.718 | 0.722 | 0.749 |
MAE↓ | 0.118 | 0.096 | 0.077 | 0.067 |
The result of the training saliency detection network using a single pseudo-label and two pseudo-labels is denoted as MDN-SP and MDN-MP, respectively. The MDN-MP shows significantly better performance and FMeasure increased from 0.675 to 0.718, while MAE decreased from 0.118 to 0.096. Based on MDN-MP, we further jointly learn saliency and edge detection, adopt pseudo-labels updating strategy and the results are denoted as MDN-Edge and MDN-Update, respectively. Using edge joint training and updating the pseudo-label both further achieve performance improvement.
To select high quality pseudo-labels for the training saliency detection model, we learn COLN twice and generate two pseudo-labels for each image, the parameter λ is selected as the optimal value λ = 2.0×10−5 and one approximately optimal value λ2 = 2.5×10−5, respectively. Then, the consistency between two pseudo-labels is calculated to measure the quality of pseudo-labels. The optimal parameter λ = 2.0×10−5 is selected from the classification accuracy curve in Figure 4. We carry different selection strategy on the approximately optimal value λ2 to select high quality pseudo-labels, then train saliency detection model MDN-MP and compare their performance against baseline MDN-SP, which is shown in Table 4.
Network | Parameter | DUTS-TE | |
Max-F↑ | MAE↓ | ||
MDN-SP | 0.675 | 0.118 | |
MDN-MP | λ=2.0×10−5, λ2=1.5×10−5 | 0.710 | 0.099 |
λ=2.0×10−5, λ2=2.0×10−5 | 0.702 | 0.108 | |
λ=2.0×10−5, λ2=2.5×10−5 | 0.718 | 0.096 |
The approximately optimal value λ2 is selected as slightly smaller, equal and slightly larger than the optimal value λ. Note that even λ2 is equal to λ, which means even when training COLN twice with the same parameter λ, the learned two COLN models still show little difference due to the randomness of neural network training and generates two different pseudo-labels for each image. This is useful in selecting high quality pseudo-labels, leading to limited performance improvement against MDN-SP. If the approximately optimal value λ2 is selected as slightly smaller or larger than the optimal value λ, they both achieve more performance improvement. This phenomenon can also be explained from another view. The high quality pseudo-labels are more robust to the choice of parameters, and the slight adjustment on weight parameter λ leads to less influence on a high quality pseudo-label and more influence on a low quality pseudo-label, The consistency calculation on two pseudo-labels with a slightly different weight parameter λ is helpful to select high quality pseudo-labels and leads to performance improvement.
In Section 3.2, we compute the IOU of each pseudo-labels pair. The pseudo-labels with IOU larger than one threshold θ will be regarded as high quality pseudo-labels to train the salient object detection model. In order to analyze the influence of threshold θ, we use different threshold parameter settings to select high quality pseudo-labels and train the MDN-MP model, then evaluate the model performance on the DUTS-TE dataset. The threshold θ setting, image number of training dataset and saliency detection performance are shown in Table 5. The larger threshold parameter θ means a more strict pseudo-label selection condition, which can generate pseudo-labels with a higher reliability and achieve performance improvement. On the other hand, the excessively strict condition also decreases the training image number and diversity of training data, which leads to performance drop. The parameter θ with 0.6 shows the best performance, which is selected as the best parameter.
θ | Training image num | DUTS-TE | |
Max-F↑ | MAE↓ | ||
0.8 | 6464 | 0.637 | 0.124 |
0.6 | 9209 | 0.718 | 0.096 |
0.4 | 9335 | 0.679 | 0.099 |
0.2 | 9447 | 0.661 | 0.103 |
In Section 3.4, to further conquer the impact of noise, we propose one pseudo-labels update strategy using parameter α and β.
In order to analyze the influence of parameter α and β, we use different parameter settings to update pseudo-labels and train final model MDN-Update, then evaluate the model performance on the DUTS-TE dataset, which is shown in Table 6. The parameter α with 15 and β with 40 shows the best performance, which is selected as the best parameter.
α | β | DUTS-TE | |
Max-F↑ | MAE↓ | ||
10 | 25 | 0.739 | 0.071 |
10 | 40 | 0.743 | 0.071 |
10 | 55 | 0.740 | 0.072 |
15 | 25 | 0.746 | 0.068 |
15 | 40 | 0.749 | 0.067 |
15 | 55 | 0.733 | 0.077 |
20 | 25 | 0.746 | 0.070 |
20 | 40 | 0.737 | 0.075 |
20 | 55 | 0.735 | 0.076 |
In this work, we proposed one weakly supervised salient object detection method with category annotation. To this end, we proposed COLN to roughly locate the object of an image, then generated pixel-level pseudo-labels and adopted one quality check strategy to select high quality pseudo labels, which supervised the training of MDN saliency detection networks. One pseudo-labels update strategy also was presented to iteratively optimize the pseudo-labels and saliency detection model. The proposed method outperforms other image category supervision based work. Additionally, the proposed method can be applied for other computer vision tasks such as object segmentation, object tracking and video scene understanding. However, the proposed method contains many steps and it is difficult to achieve global optimization. In the future, we will consider one end-to-end method to solve this problem. Although the weakly supervised method can decrease the cost of manual annotation, it still shows a performance gap with a fully supervised method. The trade-offs between annotation cost and accuracy should also be considered in future work.
The authors declare they have not used Artificial Intelligence (AI) tools in the creation of this article.
This work was supported by R & D Program of Beijing Municipal Education Commission (KM202011232014).
The authors declare there is no conflict of interest.
[1] | Global, regional, and national burden of Parkinson's disease, 1990–2016: a systematic analysis for the Global Burden of Disease Study 2016. Lancet Neurol (2018) 17: 939-953. |
[2] | Movement Disorders: Volume 35, Number S1, September 2020. Mov Disord (2020) 35:. https://doi.org/10.1002/mds.28267 |
[3] |
Ascherio A, Schwarzschild MA (2016) The epidemiology of Parkinson's disease: risk factors and prevention. Lancet Neurol 15: 1257-1272. https://doi.org/10.1016/S1474-4422(16)30230-7 ![]() |
[4] |
Kim CY, Alcalay RN (2017) Genetic Forms of Parkinson's Disease. Semin Neurol 37: 135-146. https://doi.org/10.1055/s-0037-1601567 ![]() |
[5] |
Dauer W, Przedborski S (2003) Parkinson's disease: mechanisms and models. Neuron 39: 889-909. https://doi.org/10.1016/S0896-6273(03)00568-3 ![]() |
[6] |
Braak H, Del Tredici K, Bratzke H, et al. (2002) Staging of the intracerebral inclusion body pathology associated with idiopathic Parkinson's disease (preclinical and clinical stages). J Neurol 249 Suppl 3: Iii/1-5. https://doi.org/10.1007/s00415-002-1301-4 ![]() |
[7] |
Jankovic J (2008) Parkinson's disease: clinical features and diagnosis. J Neurol Neurosurg Psychiatry 79: 368-376. https://doi.org/10.1136/jnnp.2007.131045 ![]() |
[8] |
Langston JW (2006) The parkinson's complex: Parkinsonism is just the tip of the iceberg. Ann Neurol 59: 591-596. https://doi.org/10.1002/ana.20834 ![]() |
[9] |
Postuma RB, Berg D, Stern M, et al. (2015) MDS clinical diagnostic criteria for Parkinson's disease. Mov Disord 30: 1591-1601. https://doi.org/10.1002/mds.26424 ![]() |
[10] |
Langston JW (1990) Predicting Parkinson's disease. Neurology 40: suppl 70-74; discussion 75–76. ![]() |
[11] |
Braak H, Del Tredici K, Rüb U, et al. (2003) Staging of brain pathology related to sporadic Parkinson's disease. Neurobiol Aging 24: 197-211. https://doi.org/10.1016/S0197-4580(02)00065-9 ![]() |
[12] |
Bellingham S, Guo B, Coleman B, et al. (2012) Exosomes: Vehicles for the Transfer of Toxic Proteins Associated with Neurodegenerative Diseases?. Front Physiol 3. https://doi.org/10.3389/fphys.2012.00124 ![]() |
[13] |
Guo M, Wang J, Zhao Y, et al. (2020) Microglial exosomes facilitate α-synuclein transmission in Parkinson's disease. Brain 143: 1476-1497. https://doi.org/10.1093/brain/awaa090 ![]() |
[14] |
Yang T, Martin P, Fogarty B, et al. (2015) Exosome delivered anticancer drugs across the blood-brain barrier for brain cancer therapy in Danio rerio. Pharm Res 32: 2003-2014. https://doi.org/10.1007/s11095-014-1593-y ![]() |
[15] |
Goetzl EJ, Boxer A, Schwartz JB, et al. (2015) Altered lysosomal proteins in neural-derived plasma exosomes in preclinical Alzheimer disease. Neurology 85: 40-47. https://doi.org/10.1212/WNL.0000000000001702 ![]() |
[16] |
Pardridge WM (2012) Drug transport across the blood–brain barrier. J Cerebr Blood F Met 32: 1959-1972. https://doi.org/10.1038/jcbfm.2012.126 ![]() |
[17] |
Qu M, Lin Q, Huang L, et al. (2018) Dopamine-loaded blood exosomes targeted to brain for better treatment of Parkinson's disease. J Control Release 287: 156-166. https://doi.org/10.1016/j.jconrel.2018.08.035 ![]() |
[18] |
Haney MJ, Klyachko NL, Zhao Y, et al. (2015) Exosomes as drug delivery vehicles for Parkinson's disease therapy. J Control Release 207: 18-30. https://doi.org/10.1016/j.jconrel.2015.03.033 ![]() |
[19] |
Cooper JM, Wiklander PO, Nordin JZ, et al. (2014) Systemic exosomal siRNA delivery reduced alpha-synuclein aggregates in brains of transgenic mice. Mov Disord 29: 1476-1485. https://doi.org/10.1002/mds.25978 ![]() |
[20] |
Guy R, Offen D (2020) Promising opportunities for treating neurodegenerative diseases with mesenchymal stem cell-derived exosomes. Biomolecules 10: 1320. https://doi.org/10.3390/biom10091320 ![]() |
[21] |
Théry C, Zitvogel L, Amigorena S (2002) Exosomes: composition, biogenesis and function. Nat Rev Immunol 2: 569-579. https://doi.org/10.1038/nri855 ![]() |
[22] |
Akbari-Gharalari N, Khodakarimi S, Nezhadshahmohammad F, et al. (2024) Exosomes in neuron-glia communication: A review on neurodegeneration. Bioimpacts 14: 30153-30153. https://doi.org/10.34172/bi.2023.30153 ![]() |
[23] |
Kalluri R, LeBleu VS (2020) The biology, function, and biomedical applications of exosomes. Science 367. https://doi.org/10.1126/science.aau6977 ![]() |
[24] | Omrani M, Beyrampour-Basmenj H, Jahanban-Esfahlan R, et al. (2023) Global trend in exosome isolation and application: an update concept in management of diseases. Mol Cell Biochem : 1-13. https://doi.org/10.1007/s11010-023-04756-6 |
[25] |
Lachenal G, Pernet-Gallay K, Chivet M, et al. (2011) Release of exosomes from differentiated neurons and its regulation by synaptic glutamatergic activity. Mol Cell Neurosci 46: 409-418. https://doi.org/10.1016/j.mcn.2010.11.004 ![]() |
[26] |
Wubbolts R, Leckie RS, Veenhuizen PT, et al. (2003) Proteomic and biochemical analyses of human B cell-derived exosomes. Potential implications for their function and multivesicular body formation. J Biol Chem 278: 10963-10972. https://doi.org/10.1074/jbc.M207550200 ![]() |
[27] |
van Meer G, Voelker DR, Feigenson GW (2008) Membrane lipids: where they are and how they behave. Nat Rev Mol Cell Biol 9: 112-124. https://doi.org/10.1038/nrm2330 ![]() |
[28] |
Zhang Y, Bi J, Huang J, et al. (2020) Exosome: A Review of Its Classification, Isolation Techniques, Storage, Diagnostic and Targeted Therapy Applications. Int J Nanomedicine 15: 6917-6934. https://doi.org/10.2147/IJN.S264498 ![]() |
[29] |
Skokos D, Le Panse S, Villa I, et al. (2001) Mast cell-dependent B and T lymphocyte activation is mediated by the secretion of immunologically active exosomes. J Immunol 166: 868-876. https://doi.org/10.4049/jimmunol.166.2.868 ![]() |
[30] |
Chen G, Huang AC, Zhang W, et al. (2018) Exosomal PD-L1 contributes to immunosuppression and is associated with anti-PD-1 response. Nature 560: 382-386. https://doi.org/10.1038/s41586-018-0392-8 ![]() |
[31] |
Valadi H, Ekström K, Bossios A, et al. (2007) Exosome-mediated transfer of mRNAs and microRNAs is a novel mechanism of genetic exchange between cells. Nat Cell Biol 9: 654-659. https://doi.org/10.1038/ncb1596 ![]() |
[32] |
Sharma A, Johnson A (2020) Exosome DNA: Critical regulator of tumor immunity and a diagnostic biomarker. J Cell Physiol 235: 1921-1932. https://doi.org/10.1002/jcp.29153 ![]() |
[33] |
Harding CV, Heuser JE, Stahl PD (2013) Exosomes: looking back three decades and into the future. Cell Biol 200: 367-371. https://doi.org/10.1083/jcb.201212113 ![]() |
[34] |
Pravin DP, Aashutosh US (2016) Molecular Biomarkers for Diagnosis & Therapies of Alzheimer's Disease. AIMS Neurosci 3: 433-453. https://doi.org/10.3934/Neuroscience.2016.4.433 ![]() |
[35] |
Yakubovich E, Polischouk A, Evtushenko V (2022) Principles and problems of exosome isolation from biological fluids. Biochem Mosc Suppl S 16: 115-126. https://doi.org/10.1134/S1990747822030096 ![]() |
[36] |
Kučuk N, Primožič M, Knez Ž, et al. (2021) Exosomes engineering and their roles as therapy delivery tools, therapeutic targets, and biomarkers. Int J Mol Sci 22: 9543. https://doi.org/10.3390/ijms22179543 ![]() |
[37] |
Budnik V, Ruiz-Cañada C, Wendler F (2016) Extracellular vesicles round off communication in the nervous system. Nat Rev Neurosci 17: 160-172. https://doi.org/10.1038/nrn.2015.29 ![]() |
[38] |
Desplats P, Lee HJ, Bae EJ, et al. (2009) Inclusion formation and neuronal cell death through neuron-to-neuron transmission of alpha-synuclein. Proc Natl Acad Sci U S A 106: 13010-13015. https://doi.org/10.1073/pnas.0903691106 ![]() |
[39] |
Crews L, Spencer B, Desplats P, et al. (2010) Selective Molecular Alterations in the Autophagy Pathway in Patients with Lewy Body Disease and in Models of α-Synucleinopathy. PLOS ONE 5: e9313. https://doi.org/10.1371/journal.pone.0009313 ![]() |
[40] |
Minakaki G, Menges S, Kittel A, et al. (2018) Autophagy inhibition promotes SNCA/alpha-synuclein release and transfer via extracellular vesicles with a hybrid autophagosome-exosome-like phenotype. Autophagy 14: 98-119. https://doi.org/10.1080/15548627.2017.1395992 ![]() |
[41] |
Delenclos M, Trendafilova T, Mahesh D, et al. (2017) Investigation of Endocytic Pathways for the Internalization of Exosome-Associated Oligomeric Alpha-Synuclein. Front Neurosci 11. https://doi.org/10.3389/fnins.2017.00172 ![]() |
[42] |
Huang Y, Liu Z, Li N, et al. (2022) Parkinson's Disease Derived Exosomes Aggravate Neuropathology in SNCA*A53T Mice. Ann Neurol 92: 230-245. https://doi.org/10.1002/ana.26421 ![]() |
[43] |
Han C, Xiong N, Guo X, et al. (2019) Exosomes from patients with Parkinson's disease are pathological in mice. J Mol Med (Berl) 97: 1329-1344. https://doi.org/10.1007/s00109-019-01810-z ![]() |
[44] | Sano K, Atarashi R, Satoh K, et al. (2018) Prion-Like Seeding of Misfolded α-Synuclein in the Brains of Dementia with Lewy Body Patients in RT-QUIC. Mol Neurobiol 55: 3916-3930. https://doi.org/10.1007/s12035-017-0624-1 |
[45] |
Danzer KM, Kranich LR, Ruf WP, et al. (2012) Exosomal cell-to-cell transmission of alpha synuclein oligomers. Mol Neurodegener 7: 42. https://doi.org/10.1186/1750-1326-7-42 ![]() |
[46] |
Stuendl A, Kunadt M, Kruse N, et al. (2016) Induction of α-synuclein aggregate formation by CSF exosomes from patients with Parkinson's disease and dementia with Lewy bodies. Brain 139: 481-494. https://doi.org/10.1093/brain/awv346 ![]() |
[47] |
Herman S, Djaldetti R, Mollenhauer B, et al. (2022) CSF-derived extracellular vesicles from patients with Parkinson's disease induce symptoms and pathology. Brain 146: 209-224. https://doi.org/10.1093/brain/awac261 ![]() |
[48] | Yakabi K, Berson E, Montine KS, et al. (2023) Human cerebrospinal fluid single exosomes in Parkinson's and Alzheimer's diseases. bioRxiv : 2023.2012.2022.573124. https://doi.org/10.1101/2023.12.22.573124 |
[49] |
Shi M, Liu C, Cook TJ, et al. (2014) Plasma exosomal α-synuclein is likely CNS-derived and increased in Parkinson's disease. Acta Neuropathol 128: 639-650. https://doi.org/10.1101/2023.12.22.573124 ![]() |
[50] |
Kenwrick S, Watkins A, Angelis ED (2000) Neural cell recognition molecule L1: relating biological complexity to human disease mutations. Hum Mol Genet 9: 879-886. https://doi.org/10.1093/hmg/9.6.879 ![]() |
[51] |
Niu M, Li Y, Li G, et al. (2020) A longitudinal study on α-synuclein in plasma neuronal exosomes as a biomarker for Parkinson's disease development and progression. Eur J Neurol 27: 967-974. https://doi.org/10.1111/ene.14208 ![]() |
[52] |
Yan YQ, Pu JL, Zheng R, et al. (2022) Different patterns of exosomal α-synuclein between Parkinson's disease and probable rapid eye movement sleep behavior disorder. Eur J Neurol 29: 3590-3599. https://doi.org/10.1111/ene.15537 ![]() |
[53] |
Postuma RB, Gagnon JF, Bertrand JA, et al. (2015) Parkinson risk in idiopathic REM sleep behavior disorder: preparing for neuroprotective trials. Neurology 84: 1104-1113. https://doi.org/10.1212/WNL.0000000000001364 ![]() |
[54] | Shim KH, Go HG, Bae H, et al. (2021) Decreased Exosomal Acetylcholinesterase Activity in the Plasma of Patients With Parkinson's Disease. Front Aging Neurosci 13. https://doi.org/10.3389/fnagi.2021.665400 |
[55] |
Si X, Tian J, Chen Y, et al. (2019) Central Nervous System-Derived Exosomal Alpha-Synuclein in Serum May Be a Biomarker in Parkinson's Disease. Neuroscience 413: 308-316. https://doi.org/10.1016/j.neuroscience.2019.05.015 ![]() |
[56] | Kluge A, Bunk J, Schaeffer E, et al. (2022) Correction to: Detection of neuron-derived pathological α-synuclein in blood. Brain 146: e6-e6. https://doi.org/10.1093/brain/awac370 |
[57] |
Zheng H, Xie Z, Zhang X, et al. (2021) Investigation of α-Synuclein Species in Plasma Exosomes and the Oligomeric and Phosphorylated α-Synuclein as Potential Peripheral Biomarker of Parkinson's Disease. Neuroscience 469: 79-90. https://doi.org/10.1016/j.neuroscience.2021.06.033 ![]() |
[58] |
Zhentang C, Yufeng W, Genliang L, et al. (2019) α-Synuclein in salivary extracellular vesicles as a potential biomarker of Parkinson's disease. Neurosci Lett 696: 114-120. https://doi.org/10.1016/j.neulet.2018.12.030 ![]() |
[59] |
Rani K, Mukherjee R, Singh E, et al. (2019) Neuronal exosomes in saliva of Parkinson's disease patients: A pilot study. Parkinsonism Relat D 67: 21-23. https://doi.org/10.1016/j.parkreldis.2019.09.008 ![]() |
[60] |
Kang W, Chen W, Yang Q, et al. (2016) Salivary total α-synuclein, oligomeric α-synuclein and SNCA variants in Parkinson's disease patients. Sci Rep 6: 28143. https://doi.org/10.1038/srep28143 ![]() |
[61] |
Shu H, Zhang P, Gu L (2024) Alpha-synuclein in peripheral body fluid as a biomarker for Parkinson's disease. Acta Neurol Belg 124: 831-842. https://doi.org/10.1007/s13760-023-02452-2 ![]() |
[62] |
Clements CM, McNally RS, Conti BJ, et al. (2006) DJ-1, a cancer- and Parkinson's disease-associated protein, stabilizes the antioxidant transcriptional master regulator Nrf2. Proc Natl Acad Sci U S A 103: 15091-15096. https://doi.org/10.1073/pnas.0607260103 ![]() |
[63] | Jang J, Jeong S, Lee SI, et al. (2018) Oxidized DJ-1 levels in urine samples as a putative biomarker for Parkinson's disease. Parkinsons Dis 2018. https://doi.org/10.1155/2018/1241757 |
[64] |
An C, Pu X, Xiao W, et al. (2018) Expression of the DJ-1 protein in the serum of Chinese patients with Parkinson's disease. Neurosci Lett 665: 236-239. https://doi.org/10.1016/j.neulet.2017.12.023 ![]() |
[65] |
Waragai M, Nakai M, Wei J, et al. (2007) Plasma levels of DJ-1 as a possible marker for progression of sporadic Parkinson's disease. Neurosci Lett 425: 18-22. https://doi.org/10.1016/j.neulet.2007.08.010 ![]() |
[66] |
T. dos Santos MC, Scheller D, Schulte C, et al. (2018) Evaluation of cerebrospinal fluid proteins as potential biomarkers for early stage Parkinson's disease diagnosis. PLOS ONE 13: e0206536. https://doi.org/10.1371/journal.pone.0206536 ![]() |
[67] |
Zhao Z-H, Chen Z-T, Zhou R-L, et al. (2019) Increased DJ-1 and α-synuclein in plasma neural-derived exosomes as potential markers for Parkinson's disease. Front Aging Neurosci 10: 438. https://doi.org/10.3389/fnagi.2018.00438 ![]() |
[68] |
Gonzales PA, Pisitkun T, Hoffert JD, et al. (2009) Large-Scale Proteomics and Phosphoproteomics of Urinary Exosomes. J Am Soc Nephrol 20: 363-379. https://doi.org/10.1681/ASN.2008040406 ![]() |
[69] |
Pisitkun T, Shen R-F, Knepper MA (2004) Identification and proteomic profiling of exosomes in human urine. P Natl Acad Sci 101: 13368-13373. https://doi.org/10.1073/pnas.0403453101 ![]() |
[70] | Ho DH, Yi S, Seo H, et al. (2014) Increased DJ-1 in urine exosome of Korean males with Parkinson's disease. Biomed Res Int 2014: 704678. https://doi.org/10.1155/2014/704678 |
[71] |
Goedert M, Spillantini MG (2006) A century of Alzheimer's disease. Science 314: 777-781. https://doi.org/10.1126/science.1132814 ![]() |
[72] |
Spillantini MG, Goedert M (2013) Tau pathology and neurodegeneration. Lancet Neurol 12: 609-622. https://doi.org/10.1016/S1474-4422(13)70090-5 ![]() |
[73] |
Wills J, Jones J, Haggerty T, et al. (2010) Elevated tauopathy and alpha-synuclein pathology in postmortem Parkinson's disease brains with and without dementia. Exp Neurol 225: 210-218. https://doi.org/10.1016/j.expneurol.2010.06.017 ![]() |
[74] |
Edwards TL, Scott WK, Almonte C, et al. (2010) Genome-wide association study confirms SNPs in SNCA and the MAPT region as common risk factors for Parkinson disease. Ann Hum Genet 74: 97-109. https://doi.org/10.1111/j.1469-1809.2009.00560.x ![]() |
[75] |
Simón-Sánchez J, Schulte C, Bras JM, et al. (2009) Genome-wide association study reveals genetic risk underlying Parkinson's disease. Nat Genet 41: 1308-1312. https://doi.org/10.1038/ng.487 ![]() |
[76] |
Vermilyea SC, Christensen A, Meints J, et al. (2022) Loss of tau expression attenuates neurodegeneration associated with α-synucleinopathy. Transl Neurodegener 11: 34. https://doi.org/10.1186/s40035-022-00309-x ![]() |
[77] |
Shi M, Kovac A, Korff A, et al. (2016) CNS tau efflux via exosomes is likely increased in Parkinson's disease but not in Alzheimer's disease. Alzheimers Dement 12: 1125-1131. https://doi.org/10.1016/j.jalz.2016.04.003 ![]() |
[78] |
Berg D, Schweitzer KJ, Leitner P, et al. (2005) Type and frequency of mutations in the LRRK2 gene in familial and sporadic Parkinson's disease*. Brain 128: 3000-3011. https://doi.org/10.1093/brain/awh666 ![]() |
[79] |
Paisán-Ruíz C, Jain S, Evans EW, et al. (2004) Cloning of the gene containing mutations that cause PARK8-linked Parkinson's disease. Neuron 44: 595-600. https://doi.org/10.1016/j.neuron.2004.10.023 ![]() |
[80] | Rosenbusch KE, Kortholt A (2016) Activation Mechanism of LRRK2 and Its Cellular Functions in Parkinson's Disease. Parkinsons Dis 2016: 7351985. https://doi.org/10.1155/2016/7351985 |
[81] |
Fraser KB, Moehle MS, Daher JP, et al. (2013) LRRK2 secretion in exosomes is regulated by 14-3-3. Hum Mol Genet 22: 4988-5000. https://doi.org/10.1093/hmg/ddt346 ![]() |
[82] |
Fraser KB, Moehle MS, Alcalay RN, et al. (2016) Urinary LRRK2 phosphorylation predicts parkinsonian phenotypes in G2019S LRRK2 carriers. Neurology 86: 994-999. https://doi.org/10.1212/WNL.0000000000002436 ![]() |
[83] |
Di Maio R, Hoffman EK, Rocha EM, et al. (2018) LRRK2 activation in idiopathic Parkinson's disease. Sci Transl Med 10. https://doi.org/10.1126/scitranslmed.aar5429 ![]() |
[84] |
Fraser KB, Rawlins AB, Clark RG, et al. (2016) Ser(P)-1292 LRRK2 in urinary exosomes is elevated in idiopathic Parkinson's disease. Mov Disord 31: 1543-1550. https://doi.org/10.1002/mds.26686 ![]() |
[85] |
Wang H (2021) MicroRNAs, Parkinson's Disease, and Diabetes Mellitus. Int J Mol Sci 22: 2953. https://doi.org/10.3390/ijms22062953 ![]() |
[86] |
Gui Y, Liu H, Zhang L, et al. (2015) Altered microRNA profiles in cerebrospinal fluid exosome in Parkinson disease and Alzheimer disease. Oncotarget 6: 37043-37053. https://doi.org/10.18632/oncotarget.6158 ![]() |
[87] |
Nie C, Sun Y, Zhen H, et al. (2020) Differential Expression of Plasma Exo-miRNA in Neurodegenerative Diseases by Next-Generation Sequencing. Front Neurosci 14: 438. https://doi.org/10.3389/fnins.2020.00438 ![]() |
[88] | Yao YF, Qu MW, Li GC, et al. (2018) Circulating exosomal miRNAs as diagnostic biomarkers in Parkinson's disease. Eur Rev Med Pharmacol Sci 22: 5278-5283. |
[89] |
Margis R, Margis R, Rieder CR (2011) Identification of blood microRNAs associated to Parkinsonĭs disease. J Biotechnol 152: 96-101. https://doi.org/10.1016/j.jbiotec.2011.01.023 ![]() |
[90] |
Botta-Orfila T, Morató X, Compta Y, et al. (2014) Identification of blood serum micro-RNAs associated with idiopathic and LRRK2 Parkinson's disease. J Neurosci Res 92: 1071-1077. https://doi.org/10.1002/jnr.23377 ![]() |
[91] |
Vallelunga A, Ragusa M, Di Mauro S, et al. (2014) Identification of circulating microRNAs for the differential diagnosis of Parkinson's disease and Multiple System Atrophy. Front Cell Neurosci 8. https://doi.org/10.3389/fncel.2014.00156 ![]() |
[92] |
Cao XY, Lu JM, Zhao ZQ, et al. (2017) MicroRNA biomarkers of Parkinson's disease in serum exosome-like microvesicles. Neurosci Lett 644: 94-99. https://doi.org/10.1016/j.neulet.2017.02.045 ![]() |
[93] |
Qian X, Zhao J, Yeung PY, et al. (2019) Revealing lncRNA Structures and Interactions by Sequencing-Based Approaches. Trends Biochem Sci 44: 33-52. https://doi.org/10.1016/j.tibs.2018.09.012 ![]() |
[94] |
Wu P, Zuo X, Deng H, et al. (2013) Roles of long noncoding RNAs in brain development, functional diversification and neurodegenerative diseases. Brain Res Bull 97: 69-80. https://doi.org/10.1016/j.brainresbull.2013.06.001 ![]() |
[95] |
Zou J, Guo Y, Wei L, et al. (2020) Long Noncoding RNA POU3F3 and α-Synuclein in Plasma L1CAM Exosomes Combined with β-Glucocerebrosidase Activity: Potential Predictors of Parkinson's Disease. Neurotherapeutics 17: 1104-1119. https://doi.org/10.1007/s13311-020-00842-5 ![]() |
[96] | Boer DEC, van Smeden J, Bouwstra JA, et al. (2020) Glucocerebrosidase: Functions in and Beyond the Lysosome. J Clin Med 9. https://doi.org/10.3390/jcm9030736 |
[97] |
Fanciulli A, Wenning GK (2015) Multiple-system atrophy. N Engl J Med 372: 249-263. https://doi.org/10.1056/NEJMra1311488 ![]() |
[98] |
Gilman S, Wenning GK, Low PA, et al. (2008) Second consensus statement on the diagnosis of multiple system atrophy. Neurology 71: 670-676. https://doi.org/10.1212/01.wnl.0000324625.00404.15 ![]() |
[99] |
Fanciulli A, Stankovic I, Krismer F, et al. (2019) Multiple system atrophy. Int Rev Neurobiol 149: 137-192. https://doi.org/10.1016/bs.irn.2019.10.004 ![]() |
[100] |
Galvin JE, Lee VM, Trojanowski JQ (2001) Synucleinopathies: clinical and pathological implications. Arch Neurol 58: 186-190. https://doi.org/10.1001/archneur.58.2.186 ![]() |
[101] |
Wenning GK, Ben-Shlomo Y, Hughes A, et al. (2000) What clinical features are most useful to distinguish definite multiple system atrophy from Parkinson's disease?. J Neurol Neurosurg Psychiatry 68: 434-440. https://doi.org/10.1136/jnnp.68.4.434 ![]() |
[102] |
Jiang C, Hopfner F, Katsikoudi A, et al. (2020) Serum neuronal exosomes predict and differentiate Parkinson's disease from atypical parkinsonism. J Neurol Neurosurg Psychiatry 91: 720-729. https://doi.org/10.1136/jnnp-2019-322588 ![]() |
[103] |
Dutta S, Hornung S, Kruayatidee A, et al. (2021) α-Synuclein in blood exosomes immunoprecipitated using neuronal and oligodendroglial markers distinguishes Parkinson's disease from multiple system atrophy. Acta Neuropathol 142: 495-511. https://doi.org/10.1007/s00401-021-02324-0 ![]() |
[104] |
Höglinger GU, Respondek G, Stamelou M, et al. (2017) Clinical diagnosis of progressive supranuclear palsy: The movement disorder society criteria. Mov Disord 32: 853-864. https://doi.org/10.1002/mds.26987 ![]() |
[105] |
Manna I, Quattrone A, De Benedittis S, et al. (2021) Exosomal miRNA as peripheral biomarkers in Parkinson's disease and progressive supranuclear palsy: A pilot study. Parkinsonism Relat Disord 93: 77-84. https://doi.org/10.1016/j.parkreldis.2021.11.020 ![]() |
[106] |
McKeith IG, Dickson DW, Lowe J, et al. (2005) Diagnosis and management of dementia with Lewy bodies: third report of the DLB Consortium. Neurology 65: 1863-1872. https://doi.org/10.1212/01.wnl.0000187889.17253.b1 ![]() |
[107] | Lippa CF, Duda JE, Grossman M, et al. (2007) DLB and PDD boundary issues. Diagnosis Treatment Molecular Pathology Biomarkers 68: 812-819. https://doi.org/10.1212/01.wnl.0000256715.13907.d3 |
[108] |
Bonanni L, Thomas A, Onofrj M (2006) Diagnosis and management of dementia with Lewy bodies: third report of the DLB Consortium. Neurology 66: 1455; author reply 1455. https://doi.org/10.1212/01.wnl.0000224698.67660.45 ![]() |
[109] |
Emre M, Aarsland D, Brown R, et al. (2007) Clinical diagnostic criteria for dementia associated with Parkinson's disease. Mov Disord 22: 1689-1707; quiz 1837. https://doi.org/10.1002/mds.21507 ![]() |
[110] |
Walker Z, Possin KL, Boeve BF, et al. (2015) Lewy body dementias. Lancet 386: 1683-1697. https://doi.org/10.1016/S0140-6736(15)00462-6 ![]() |
[111] |
Swallow DMA, Counsell CE (2023) The evolution of diagnosis from symptom onset to death in progressive supranuclear palsy (PSP) and corticobasal degeneration (CBD) compared to Parkinson's disease (PD). J Neurol 270: 3464-3474. https://doi.org/10.1007/s00415-023-11629-x ![]() |
[112] |
Meloni M, Agliardi C, Guerini FR, et al. (2023) Oligomeric α-synuclein and tau aggregates in NDEVs differentiate Parkinson's disease from atypical parkinsonisms. Neurobiol Dis 176: 105947. https://doi.org/10.1016/j.nbd.2022.105947 ![]() |
[113] |
Jiang C, Hopfner F, Berg D, et al. (2021) Validation of α-Synuclein in L1CAM-Immunocaptured Exosomes as a Biomarker for the Stratification of Parkinsonian Syndromes. Mov Disord 36: 2663-2669. https://doi.org/10.1002/mds.28591 ![]() |
[114] |
Müller T (2012) Drug therapy in patients with Parkinson's disease. Translational Neurodegener 1: 1-13. https://doi.org/10.1186/2047-9158-1-10 ![]() |
[115] |
Zhuang X, Xiang X, Grizzle W, et al. (2011) Treatment of brain inflammatory diseases by delivering exosome encapsulated anti-inflammatory drugs from the nasal region to the brain. Mol Ther 19: 1769-1779. https://doi.org/10.1038/mt.2011.164 ![]() |
[116] | Lai CP-K, Breakefield XO (2012) Role of exosomes/microvesicles in the nervous system and use in emerging therapies. Front Physiol 3: 228. https://doi.org/10.3389/fphys.2012.00228 |
[117] |
Akbari-Gharalari N, Ghahremani-Nasab M, Naderi R, et al. (2023) Improvement of spinal cord injury symptoms by targeting the Bax/Bcl2 pathway and modulating TNF-α/IL-10 using Platelet-Rich Plasma exosomes loaded with dexamethasone. AIMS Neurosci 10: 332-353. https://doi.org/10.3934/Neuroscience.2023026 ![]() |
[118] |
Kojima R, Bojar D, Rizzi G, et al. (2018) Designer exosomes produced by implanted cells intracerebrally deliver therapeutic cargo for Parkinson's disease treatment. Nat Commun 9: 1305. https://doi.org/10.1038/s41467-018-03733-8 ![]() |
[119] |
Izco M, Blesa J, Schleef M, et al. (2019) Systemic exosomal delivery of shRNA minicircles prevents parkinsonian pathology. Mol Ther 27: 2111-2122. https://doi.org/10.1016/j.ymthe.2019.08.010 ![]() |
[120] |
Mianehsaz E, Mirzaei HR, Mahjoubin-Tehran M, et al. (2019) Mesenchymal stem cell-derived exosomes: a new therapeutic approach to osteoarthritis?. Stem Cell Res Ther 10: 1-13. https://doi.org/10.1186/s13287-019-1445-0 ![]() |
[121] |
Li Z, Liu F, He X, et al. (2019) Exosomes derived from mesenchymal stem cells attenuate inflammation and demyelination of the central nervous system in EAE rats by regulating the polarization of microglia. Int Immunopharmacol 67: 268-280. https://doi.org/10.1016/j.intimp.2018.12.001 ![]() |
[122] |
Vilaça-Faria H, Salgado AJ, Teixeira FG (2019) Mesenchymal stem cells-derived exosomes: a new possible therapeutic strategy for Parkinson's disease?. Cells 8: 118. https://doi.org/10.3390/cells8020118 ![]() |
[123] |
Heris RM, Shirvaliloo M, Abbaspour-Aghdam S, et al. (2022) The potential use of mesenchymal stem cells and their exosomes in Parkinson's disease treatment. Stem Cell Res Ther 13: 371. https://doi.org/10.1186/s13287-022-03050-4 ![]() |
[124] |
Abrishamdar M, Jalali MS, Yazdanfar N (2023) The role of exosomes in pathogenesis and the therapeutic efficacy of mesenchymal stem cell-derived exosomes against Parkinson's disease. Neurol Sci 44: 2277-2289. https://doi.org/10.1007/s10072-023-06706-y ![]() |
[125] |
Kattaia A, Abd EL-Baset S, Abdul-Maksoud R, et al. (2022) The therapeutic potential of exosomes derived from mesenchymal stem cells in experimentally induced hypertensive encephalopathy. J Med Histol 6: 16-33. https://doi.org/10.21608/jmh.2022.145535.1100 ![]() |
[126] |
Tomlinson PR, Zheng Y, Fischer R, et al. (2015) Identification of distinct circulating exosomes in Parkinson's disease. Ann Clin Transl Neur 2: 353-361. https://doi.org/10.1002/acn3.175 ![]() |
[127] |
Sarko DK, McKinney CE (2017) Exosomes: origins and therapeutic potential for neurodegenerative disease. Front Neurosci 11: 82. https://doi.org/10.3389/fnins.2017.00082 ![]() |
1. | Lina Du, Chunjie Ma, Junhao Chen, Huimin Zheng, Xiushan Nie, Zan Gao, Survey on deep learning-based weakly supervised salient object detection, 2025, 09574174, 127497, 10.1016/j.eswa.2025.127497 |
IOU | Image Num | MAE |
0.8–1.0 | 5871 | 0.098 |
0.6–0.8 | 3338 | 0.110 |
0.4–0.6 | 125 | 0.173 |
0.2–0.4 | 403 | 0.171 |
0.0–0.2 | 816 | 0.201 |
Supervision | Method | DUTS-TE | PASCAL-S | ECSSD | HKU-IS | |||||||
Max-F↑ | MAE↓ | Max-F↑ | MAE↓ | Max-F↑ | MAE↓ | Max-F↑ | MAE↓ | |||||
Fully supervised | SAMNet[61] | 0.836 | 0.058 | 0.856 | 0.113 | 0.927 | 0.051 | 0.914 | 0.044 | |||
PAGRN[62] | 0.778 | 0.055 | 0.765 | 0.151 | 0.871 | 0.064 | 0.863 | 0.045 | ||||
PFAN[63] | 0.764 | 0.060 | 0.754 | 0.137 | 0.875 | 0.046 | 0.871 | 0.042 | ||||
UCF[64] | 0.663 | 0.112 | 0.787 | 0.140 | 0.844 | 0.070 | 0.818 | 0.062 | ||||
Bounding-box | WSB[19] | 0.736 | 0.079 | - | - | 0.860 | 0.072 | 0.853 | 0.058 | |||
Subitizing | SOS[51] | - | - | 0.803 | 0.131 | 0.858 | 0.108 | 0.882 | 0.080 | |||
Scribble | SAE[23] | 0.746 | 0.062 | 0.788 | 0.139 | 0.865 | 0.061 | 0.857 | 0.047 | |||
LDS[52] | 0.755 | 0.066 | 0.793 | 0.094 | 0.873 | 0.056 | 0.860 | 0.048 | ||||
Category | WSS[25] | 0.630 | 0.099 | 0.697 | 0.184 | 0.823 | 0.109 | 0.821 | 0.084 | |||
ASMO[20] | 0.614 | 0.116 | 0.752 | 0.145 | 0.837 | 0.112 | 0.846 | 0.088 | ||||
MSW[53] | 0.684 | 0.091 | 0.713 | 0.133 | 0.840 | 0.096 | 0.814 | 0.084 | ||||
MFNet[21] | 0.710 | 0.076 | 0.751 | 0.115 | 0.854 | 0.084 | 0.851 | 0.059 | ||||
NSAL[22] | 0.729 | 0.074 | 0.753 | 0.111 | 0.853 | 0.081 | 0.859 | 0.053 | ||||
Ours | 0.749 | 0.067 | 0.785 | 0.125 | 0.871 | 0.063 | 0.861 | 0.049 |
Metrics | MDN-SP | MDN-MP | MDN-Edge | MDN-Update |
Max-F↑ | 0.675 | 0.718 | 0.722 | 0.749 |
MAE↓ | 0.118 | 0.096 | 0.077 | 0.067 |
Network | Parameter | DUTS-TE | |
Max-F↑ | MAE↓ | ||
MDN-SP | 0.675 | 0.118 | |
MDN-MP | λ=2.0×10−5, λ2=1.5×10−5 | 0.710 | 0.099 |
λ=2.0×10−5, λ2=2.0×10−5 | 0.702 | 0.108 | |
λ=2.0×10−5, λ2=2.5×10−5 | 0.718 | 0.096 |
θ | Training image num | DUTS-TE | |
Max-F↑ | MAE↓ | ||
0.8 | 6464 | 0.637 | 0.124 |
0.6 | 9209 | 0.718 | 0.096 |
0.4 | 9335 | 0.679 | 0.099 |
0.2 | 9447 | 0.661 | 0.103 |
α | β | DUTS-TE | |
Max-F↑ | MAE↓ | ||
10 | 25 | 0.739 | 0.071 |
10 | 40 | 0.743 | 0.071 |
10 | 55 | 0.740 | 0.072 |
15 | 25 | 0.746 | 0.068 |
15 | 40 | 0.749 | 0.067 |
15 | 55 | 0.733 | 0.077 |
20 | 25 | 0.746 | 0.070 |
20 | 40 | 0.737 | 0.075 |
20 | 55 | 0.735 | 0.076 |
IOU | Image Num | MAE |
0.8–1.0 | 5871 | 0.098 |
0.6–0.8 | 3338 | 0.110 |
0.4–0.6 | 125 | 0.173 |
0.2–0.4 | 403 | 0.171 |
0.0–0.2 | 816 | 0.201 |
Supervision | Method | DUTS-TE | PASCAL-S | ECSSD | HKU-IS | |||||||
Max-F↑ | MAE↓ | Max-F↑ | MAE↓ | Max-F↑ | MAE↓ | Max-F↑ | MAE↓ | |||||
Fully supervised | SAMNet[61] | 0.836 | 0.058 | 0.856 | 0.113 | 0.927 | 0.051 | 0.914 | 0.044 | |||
PAGRN[62] | 0.778 | 0.055 | 0.765 | 0.151 | 0.871 | 0.064 | 0.863 | 0.045 | ||||
PFAN[63] | 0.764 | 0.060 | 0.754 | 0.137 | 0.875 | 0.046 | 0.871 | 0.042 | ||||
UCF[64] | 0.663 | 0.112 | 0.787 | 0.140 | 0.844 | 0.070 | 0.818 | 0.062 | ||||
Bounding-box | WSB[19] | 0.736 | 0.079 | - | - | 0.860 | 0.072 | 0.853 | 0.058 | |||
Subitizing | SOS[51] | - | - | 0.803 | 0.131 | 0.858 | 0.108 | 0.882 | 0.080 | |||
Scribble | SAE[23] | 0.746 | 0.062 | 0.788 | 0.139 | 0.865 | 0.061 | 0.857 | 0.047 | |||
LDS[52] | 0.755 | 0.066 | 0.793 | 0.094 | 0.873 | 0.056 | 0.860 | 0.048 | ||||
Category | WSS[25] | 0.630 | 0.099 | 0.697 | 0.184 | 0.823 | 0.109 | 0.821 | 0.084 | |||
ASMO[20] | 0.614 | 0.116 | 0.752 | 0.145 | 0.837 | 0.112 | 0.846 | 0.088 | ||||
MSW[53] | 0.684 | 0.091 | 0.713 | 0.133 | 0.840 | 0.096 | 0.814 | 0.084 | ||||
MFNet[21] | 0.710 | 0.076 | 0.751 | 0.115 | 0.854 | 0.084 | 0.851 | 0.059 | ||||
NSAL[22] | 0.729 | 0.074 | 0.753 | 0.111 | 0.853 | 0.081 | 0.859 | 0.053 | ||||
Ours | 0.749 | 0.067 | 0.785 | 0.125 | 0.871 | 0.063 | 0.861 | 0.049 |
Metrics | MDN-SP | MDN-MP | MDN-Edge | MDN-Update |
Max-F↑ | 0.675 | 0.718 | 0.722 | 0.749 |
MAE↓ | 0.118 | 0.096 | 0.077 | 0.067 |
Network | Parameter | DUTS-TE | |
Max-F↑ | MAE↓ | ||
MDN-SP | 0.675 | 0.118 | |
MDN-MP | λ=2.0×10−5, λ2=1.5×10−5 | 0.710 | 0.099 |
λ=2.0×10−5, λ2=2.0×10−5 | 0.702 | 0.108 | |
λ=2.0×10−5, λ2=2.5×10−5 | 0.718 | 0.096 |
θ | Training image num | DUTS-TE | |
Max-F↑ | MAE↓ | ||
0.8 | 6464 | 0.637 | 0.124 |
0.6 | 9209 | 0.718 | 0.096 |
0.4 | 9335 | 0.679 | 0.099 |
0.2 | 9447 | 0.661 | 0.103 |
α | β | DUTS-TE | |
Max-F↑ | MAE↓ | ||
10 | 25 | 0.739 | 0.071 |
10 | 40 | 0.743 | 0.071 |
10 | 55 | 0.740 | 0.072 |
15 | 25 | 0.746 | 0.068 |
15 | 40 | 0.749 | 0.067 |
15 | 55 | 0.733 | 0.077 |
20 | 25 | 0.746 | 0.070 |
20 | 40 | 0.737 | 0.075 |
20 | 55 | 0.735 | 0.076 |