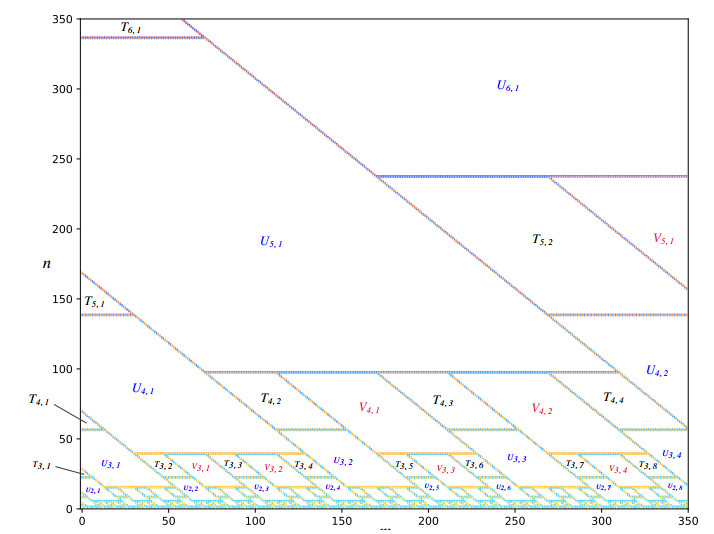
Let τ be the substitution 1→101 and 0→1 on the alphabet {0,1}. The fixed point of τ obtained starting from 1, denoted by s, is a Sturmian sequence. We first give a characterization of s using f-representation. Then we show that the distribution of zeros in the determinants induces a partition of integer lattices in the first quadrant. Combining those properties, we give the explicit values of the Hankel determinants Hm,n of s for all m≥0 and n≥1.
Citation: Haocong Song, Wen Wu. Hankel determinants of a Sturmian sequence[J]. AIMS Mathematics, 2022, 7(3): 4233-4265. doi: 10.3934/math.2022235
[1] | Mohammad Faisal Khan, Jongsuk Ro, Muhammad Ghaffar Khan . Sharp estimate for starlikeness related to a tangent domain. AIMS Mathematics, 2024, 9(8): 20721-20741. doi: 10.3934/math.20241007 |
[2] | Abdullah Özbekler, Kübra Uslu İşler, Jehad Alzabut . Sturmian comparison theorem for hyperbolic equations on a rectangular prism. AIMS Mathematics, 2024, 9(2): 4805-4815. doi: 10.3934/math.2024232 |
[3] | Muhammmad Ghaffar Khan, Wali Khan Mashwani, Jong-Suk Ro, Bakhtiar Ahmad . Problems concerning sharp coefficient functionals of bounded turning functions. AIMS Mathematics, 2023, 8(11): 27396-27413. doi: 10.3934/math.20231402 |
[4] | Muhammmad Ghaffar Khan, Wali Khan Mashwani, Lei Shi, Serkan Araci, Bakhtiar Ahmad, Bilal Khan . Hankel inequalities for bounded turning functions in the domain of cosine Hyperbolic function. AIMS Mathematics, 2023, 8(9): 21993-22008. doi: 10.3934/math.20231121 |
[5] | Muhammad Ghaffar Khan, Sheza.M. El-Deeb, Daniel Breaz, Wali Khan Mashwani, Bakhtiar Ahmad . Sufficiency criteria for a class of convex functions connected with tangent function. AIMS Mathematics, 2024, 9(7): 18608-18624. doi: 10.3934/math.2024906 |
[6] | Muhammad Ghaffar Khan, Nak Eun Cho, Timilehin Gideon Shaba, Bakhtiar Ahmad, Wali Khan Mashwani . Coefficient functionals for a class of bounded turning functions related to modified sigmoid function. AIMS Mathematics, 2022, 7(2): 3133-3149. doi: 10.3934/math.2022173 |
[7] | Wenzheng Hu, Jian Deng . Hankel determinants, Fekete-Szegö inequality, and estimates of initial coefficients for certain subclasses of analytic functions. AIMS Mathematics, 2024, 9(3): 6445-6467. doi: 10.3934/math.2024314 |
[8] | Huo Tang, Shahid Khan, Saqib Hussain, Nasir Khan . Hankel and Toeplitz determinant for a subclass of multivalent q-starlike functions of order α. AIMS Mathematics, 2021, 6(6): 5421-5439. doi: 10.3934/math.2021320 |
[9] | Tomomichi Hagiwara, Masaki Sugiyama . L2/L1 induced norm and Hankel norm analysis in sampled-data systems. AIMS Mathematics, 2024, 9(2): 3035-3075. doi: 10.3934/math.2024149 |
[10] | Daud Mohamad, Nur Hazwani Aqilah Abdul Wahid, Nurfatin Nabilah Md Fauzi . Some properties of a new subclass of tilted star-like functions with respect to symmetric conjugate points. AIMS Mathematics, 2023, 8(1): 1889-1900. doi: 10.3934/math.2023097 |
Let τ be the substitution 1→101 and 0→1 on the alphabet {0,1}. The fixed point of τ obtained starting from 1, denoted by s, is a Sturmian sequence. We first give a characterization of s using f-representation. Then we show that the distribution of zeros in the determinants induces a partition of integer lattices in the first quadrant. Combining those properties, we give the explicit values of the Hankel determinants Hm,n of s for all m≥0 and n≥1.
Let s=(sj)j≥0 be an integer sequence. For all m≥0, n≥1, the (m,n)-order Hankel matrix of s is
Mm,n:=(sm+i+j)0≤i,j≤n−1=(smsm+1⋯sm+n−1sm+1sm+2⋯sm+n⋮⋮⋱⋮sm+n−1sm+n⋯sm+2n−2.). |
The (m,n)-order Hankel determinant of s is Hm,n=detMm,n.
Hankel determinants of automatic sequences have been widely studied, due to its application to the study of irrationality exponent of real numbers; see for example [1,3,5,6,7,9,15] and references therein. In 2016, Han [10] introduced the Hankel continued fraction which is a powerful tool for evaluating Hankel determinants. By using the Hankel continued fractions, Bugeaud, Han Wen and Yao [4] characterized the irrationality exponents of values of certain degree two Mahler functions at rational points. Recently, Guo, Han and Wu [8] fully characterized apwenian sequences, that is ±1 sequences whose Hankel determinants H0,n satisfying H0,n/2n−1≡1(mod2) for all n≥1.
However, the Hankel determinants of other low complexity sequences, such as Sturmian sequences, are rarely known. Kamae, Tamura and Wen [11] explicitly evaluated the Hankel determinants of the Fibonacci word. Tamura [13] extended this result to infinite words generated by the substitutions a→akb,b→a (k≥1). In this paper, we study the Hankel determinants of the sequence generated by the substitution
τ:1→101,0→1. |
Denote by s=(sn)n≥0=limn→∞τn(1) the fixed point of τ. Since τn+1(1)=τn(τ(1))=τn(1)τn(0)τn(1), the word τn(1) is a prefix of s. The first values of s can be obtained by finding τn(1). For example,
s0s1⋯s6=τ2(1)=τ(101)=1011101 |
and
s0s1⋯s17=τ3(1)=τ(τ2(1))=10111011011011101. |
It follows from [14,Proposition 2.1] that s is a Sturmian sequence. See also the sequence A104521 in [12].
We give the explicit values of Hankel determinants Hm,n for the sequence s for all m≥0 and n≥1. In Figure 1, we use the color at the point (m,n) to indicate the value of Hm,n. For example, if Hm,n≠Hm′,n′, then points (m,n) and (m′,n′) will be marked by different colors. In particular, if Hm,n=0, then the point (m,n) is marked by white. Then we can see the distribution of first values of Hm,n from Figure 1 and the collection of all the points (m,n) with Hm,n=0 are the union of disjoint parallelograms. These parallelograms (together with their boundaries) are divided into parallelograms of three types, labelled by Uk,i, Vk,i and Tk,i where k≥0 and i≥1 (for detailed definitions, see Section 3).
For all k≥0 and i≥1, Theorem 1.1 (resp. Theorem 1.2, Theorem 1.3) gives the exact value of Hm,n for all (m,n)∈Uk,i (resp. Vk,i, Tk,i); see Figure 2. Since for k≥0 and i≥1, the parallelograms Uk,i, Vk,i and Tk,i are disjoint and they tile the lattices in the first quadrant; see Proposition 3.1 in Section 3. Combing this nice property and Theorem 1.1, 1.2 and 1.3, we obtain the values of Hm,n for all m≥0 and n≥1.
To state our results, we need four (technically defined) integer sequences (fn)n≥0, (αi)i≥1, (βi)i≥1 and (γi)i≥1; see Section 3 for their definitions. These four sequences are used to locate the corners of parallelograms Uk,i, Vk,i and Tk,i. Our main results are the following.
Theorem 1.1. Let k≥0 and Uk=∪i≥1Uk,i. For all (m,n)∈Uk,
1. when n=f2k+3−1, Hm,n=(−1)k+1(−1)f2k+52−Φk+1(m+n)⋅f2k+12;
2. when n=f2k, Hm,n=(−1)k+1(−1)f2k+2−12⋅f2k+12;
3. when f2k<n<f2k+3−1, if m+n=αi−f2k+2+1 or αi for some i≥1, then
Hm,n=−(−1)(f2k+3−n)k(−1)(f2k+3−1−n)(f2k+3−2−n)2⋅f2k+12; |
otherwise Hm,n=0.
Theorem 1.2. Let k≥0 and Vk=∪i≥1Vk,i. For all (m,n)∈Vk,
1. when n=f2k+2−1, Hm,n=(−1)f2k+2+f2k+1−32⋅f2k+12;
2. when n=f2k, Hm,n=(−1)k+1(−1)f2k+2−12⋅f2k+12;
3. when f2k<n<f2k+2−1, if m+n=βi+1 or βi+f2k+1 for some i≥1, then
Hm,n=−(−1)(f2k+2−n)(k+1)(−1)(f2k+2−1−n)(f2k+2−2−n)2⋅f2k+12; |
otherwise Hm,n=0.
Theorem 1.3. Let k≥0 and Tk=∪i≥1Tk,i. For all (m,n)∈Tk,
1. when n=f2k+2−1, Hm,n=(−1)f2k−12⋅f2k;
2. when n=f2k+1, Hm,n=(−1)Φk(m+n)−f2k+12−1⋅f2k;
3. when f2k<n<f2k+2−1, if m+n=γi−f2k+1 or γi for some i≥1, then
Hm,n=(−1)(f2k+2−1−n)k(−1)(f2k+2−1−n)(f2k+2−2−n)2(−1)f2k−12⋅f2k; |
otherwise Hm,n=0.
Sketch of proofs of main results
The computational result indicates that the collection of points (m,n) such that the Hankel determinant Hm,n=0 is covered by disjoint parallelograms of three different types, denoted by U∗,∗,V∗,∗ and T∗,∗; see Figure 1. This inspires us to calculate the Hankel determinant Hm,n in each parallelogram (according to the location of (m,n)). Then connect the values of Hankel determinants in different parallelograms.
Step 1 Locate the parallelograms.
We first find that the second coordinates of the corners of those parallelograms can be expressed in terms of an integer sequence (fn)n≥0 (see Figure 2 for example). Then we see that the first coordinates of corners of three types of parallelograms are determined by three integer sequences (αi)i≥1, (βi)i≥1 and (γi)i≥1 introduced in Section 3. Then we characterize the parallelograms (observed in Figure 1) by (3.1). Proposition 3.1 showed that parallelograms defined by (3.1) tile the first quadrant.
Step 2 Calculate Hm,n for (m,n) inside the parallelograms Uk,∗, Vk,∗ and Tk,∗.
Lemma 4.1 show that Hm,n=0 for all (m,n) which are not on the boundary of those parallelograms. Now the white part in Figure 1 is clear.
Step 3 Reduction on the boundary of Uk,∗, Vk,∗ and Tk,∗.
● By Lemma 4.2 and Lemma 4.6 (resp. Lemma 4.8, Lemma 4.10), calculating Hm,n for all (m,n) on the boundary of Uk,∗ (resp. Vk,∗, Tk,∗) is reduced to calculate the determinant Hm,n for only one point (m,n) on its boundary. See Figure 3.
● Lemma 4.3 connects the values of Hm,n for (m,n) on the lower edge of Uk,∗ and Vk,∗. Lemma 4.4 builds similar connections for the values of Hm,n for (m,n) on the lower edge of Tk,i and Tk,i+1. See Figure 4.
Therefore, to obtain the values of Hm,n for all (m,n) on the boundary of all Uk,∗ and Vk,∗, we only need to calculate Hm,n for one point (m,n) on the boundary of Uk,1.
Step 4 Reduction on k. Lemma 5.1 enable us to calculate Hm,n for (m,n) on the boundary of Uk+1,∗ by using the values of Hm,n on the boundary of Uk,∗ and Tk,∗. Lemma 5.2 enable us to calculate Hm,n for (m,n) on the boundary of Tk,∗ by using the values of Hm,n on the boundary of Uk,∗ and Uk−1,∗. See Figure 4.
Step 5 According to step 3 and step 4, to obtain the value of Hm,n for all (m,n), we only need to calculate Hm,n for (m,n) on the boundary of Uk,1, Vk,1 and Tk,2 for all k. These have been done by Theorem 5.3, Corollary 5.4 and Corollary 5.5.
The paper is organized as follows. In Section 2, we introduce the f-representation of positive integers and give a criterion (Proposition 2.4) to determine sn according the f-representation of n. This criterion leads us to the key ingredient (Theorem 2.5) in calculating the Hankel determinants. Then we introduce the truncated f-representation which is essential in describing the parallelograms. In Section 3, we show that the parallelograms Uk,i, Vk,i and Tk,i tile all the integer points in the first quadrant. In Section 4, we first show that the Hankel determinants vanish when (m,n) is inside a parallelogram of those three types. Next we show the relations of Hankel determinants Hm,n on the boundary of a parallelogram Uk,i (or Vk,i, Tk,i) for a given k and i. Finally, for any k≥0, we describe the relation of values of Hankel determinants for parallelograms Uk,i for all i≥1. In Section 5, we give the expressions for Hankel determinants on the boundary of Uk,i (or Vk,i, Tk,i) for all k≥0. In the last section, we formulate and prove our main results.
In this section, we first introduce the f-representation of positive integers according to the sequence (fn)n≥0. By understanding the occurrences of 0s in the sequence s, we prove a key result (Proposition 2.4) which can determine sn according to the f-representation of n. Then we give the essential result (Theorem 2.5). In subsection 2.3, we introduce the truncated f-representation which is useful in determining the parallelograms. In section 2.4, we investigate to some sub-sequences of (fn)n≥0 which are need in evaluating the coefficients of the Hankel determinants. Then we characterize two sub-sequences of s which helps us understand Hm,n.
We introduce an auxiliary sequence (fn)n≥0 to determine the positions of 0's. For all n≥0, we define
f2n=|τn(1)|andf2n+1=|τn(10)|. |
Then f0=1, f1=2, and for all n≥0,
{f2n+2=f2n+f2n+1,f2n+3=f2n+f2n+2. | (2.1) |
The first values are
(fn)n≥0=(1,2,3,4,7,10,17,24,41,58,99,140,239,338,577,816,…). |
Remark 2.1. The sequence (fn)n≥0 can be expressed in terms of Pell numbers (pn)n≥0 defined by the recurrence p0=1, p1=2 and pn+2=2pn+1+pn for all n≥0. See the sequence A000129 in [12]. Indeed, pn (resp. pn−1) is the number of 1's (resp. 0's) in τn(1). It is easy to verify that f2n=pn+1−pn and f2n+1=2pn.
Since (fn)n≥0 is an increasing non-negative integer sequence, it is a numeration system in the following sense.
Lemma 2.2 (Theorem 3.1.1 [2]). Let u0<u1<u2<… be an increasing sequence of integers with u0=1. Every non-negative integer N has exactly one representation of the form ∑0≤i≤raiui where ar≠0, and for i≥0, the digits ai are non-negative integers satisfying the inequality
a0u0+a1u1+⋯+aiui<ui+1. |
Proposition 2.3. Every integer n≥0 can be uniquely expressed as n=∑0≤i≤raifi with ai∈{0,1}, ar≠0, and
{aiai+1=0for all0≤i<r,aiai+2=0for all even numbers0≤i<r−1. | (2.2) |
Proof. Suppose ai∈{0,1}. By Lemma 2.2, we only need to show that a0f0+a1f1+⋯+atft<ft+1 for all t if and only if the condition (2.2) holds.
The 'only if' part. Suppose there is an index i such that aiai+1=1. Then
a0f0+a1f1+⋯+aifi+ai+1fi+1≥fi+fi+1≥fi+2, |
which is a contradiction for t=i+1. Suppose there is an even index i such that aiai+2=1. Then
a0f0+a1f1+⋯+aifi+ai+1fi+1+ai+2fi+2≥fi+fi+2=fi+3, |
which is a contradiction for t=i+2.
The 'if' part. Suppose the condition (2.2) holds. When t is odd, the maximum possible value of a0f0+a1f1+⋯+atft occurs when atat−1…a0=1010…10, and this maximum value f1+f3+⋯+ft−2+ft=ft+1−1. When t is even, the maximum possible value of a0f0+a1f1+⋯+atft occurs when atat−1…a0=1001010…10. In this case, the maximum value is f1+f3+⋯+ft−5+ft−3+ft=ft+1−1.
Definition (f-representation). Let n≥0 be an integer. We call the representation n=∑0≤i≤raifi in Proposition 2.3 the f-representation of n. We also write n=∑+∞i=0aifi where ai=0 for all i>r. In the case that we need to emphasize that ai depends on n, we write ai=ai(n) as a function of n.
Proposition 2.4. For any integer n≥0 with the f-representation ∑ri=0ai(n)fi, we have sn=0 if and only if a0(n)=1.
Proof. One can verify directly that the result holds for all n<f4=7. Assume that the result holds for n<f2k where k≥2. We only need to prove it for all f2k≤n<f2k+2.
Suppose f2k≤n<f2k+1. One has a2k(n)=1 and hence a0(n−f2k)=a0(n). Note that f2k=|τk(1)| and
s0s1…sf2k+2−1=τk+1(1)=τk(1)τk(0)τk(1). | (2.3) |
We see that sn is the (n+1)-th letter of τk+1(1) and it is also the (n+1−f2k)-th letter of τk(0)=τk−1(1). Consequently, sn=sn−f2k. Since
n−f2k<f2k+1−f2k=f2k−2, |
by the inductive assumption, we have sn−f2k=0 if and only if a0(n−f2k)=1. Therefore, sn=0 if and only if a0(n)=1.
Suppose f2k+1≤n<f2k+2. In this case a2k+1(n)=1 and a0(n−f2k+1)=a0(n). Since |τk(10)|=f2k+1, it follows from (2.3) that sn=sn−f2k+1. Note that
n−f2k+1<f2k+2−f2k+1=f2k. |
By the inductive assumption, sn−f2k+1=0 if and only if a0(n−f2k+1)=1 which implies the result also holds for all f2k+1≤n<f2k+2.
We introduce the truncated f-representations (of positive integers) which are useful in telling two digits with a fixed gap in s are equal or not.
Definition. (Truncated f-representation) Let n≥0 be an integer with the f-representation ∑+∞i=0ai(n)fi. For all integers k≥0, the truncated f-representation of n is
Φk(n):=2k+2∑i=0ai(n)fi. |
The next lemma gives a criterion that when two digits (with a fixed gap) in s are equal by using their positions.
Theorem 2.5. Let n≥0 be an integer with the f-representation ∑+∞i=0ai(n)fi. Then
(i) for all k≥0, sn+f2k≠sn if and only if Φk(n)∈{f2k+12,f2k+12−1};
(ii) for all k≥1, sn+f2k+1≠sn if and only if Φk(n)∈{f2k+32,f2k+32−1,f2k+32+f2k,f2k+32+f2k−1}.
Proof. (ⅰ) We prove by induction on k. When k=0, by Proposition 2.3, there are only four possible values for a0(n)a1(n)a2(n). By Eq (2.1), we have
Φ0(n) | 0 | 1 | 2 | 3 |
a0(n)a1(n)a2(n) | 000 | 100 | 010 | 001 . |
a0(n+f0) | 1 | 0 | 0 | 0 |
Then we see that a0(n)≠a0(n+f0) if and only if Φ0(n)=0 or 1. The result holds for k=0.
When k=1, note that Φ1(n)≤∑4i=0fi<f5=10. We see
Φ1(n) | 0 | 1 | 2 | 3 | 4 | 5 | 6 | 7 | 8 | 9 |
a0(n)…a4(n) | 00000 | 10000 | 01000 | 00100 | 00010 | 10010 | 01010 | 00001 | 10001 | 01001 . |
a0(n+f2) | 0 | 0 | 1 | 0 | 0 | 1 | 0 | 0 | 1 | 0 |
Then we have a0(n)≠a0(n+f2) if and only if Φ1(n)=1 or 2, that is f32−1 or f32. The result also holds for k=1.
Now assume that the result holds for all 0≤k<ℓ with ℓ≥2. We prove it for k=ℓ. Let w=a2ℓ−2(n)a2ℓ−1(n)…a2ℓ+2(n) and v=a2ℓ−2(n+f2ℓ)a2ℓ−1(n+f2ℓ)…a2ℓ+1(n+f2ℓ). According to Proposition 2.3, w can take only 10 different values.
While w≠01000, one can determine v directly using Eq (2.1); thus in these cases, ai(n+f2ℓ)=ai(n) for all 0≤i≤2ℓ−3; see Table 1. For instance, when w=10010,
n+f2ℓ=(2ℓ−3∑i=0ai(n)fi+f2ℓ−2+f2ℓ+1++∞∑i=2ℓ+3ai(n)fi)+f2ℓ=(2ℓ−3∑i=0ai(n)fi+f2ℓ−2)+(f2ℓ+2++∞∑i=2ℓ+3ai(n)fi). |
w | 00000 | 10000 | 01000 | 00100 | 00010 | 10010 | 01010 | 00001 | 10001 | 01001 |
v | 0010 | 0001 | ? | 0101 | 0000 | 1000 | 0100 | 0000 | 1000 | 0100 |
Hence one can see that a0(n+f2ℓ)=a0(n).
When w=01000, set n′=∑2ℓ−2i=0ai(n)fi. Then a0(n)=a0(n′) and
n+f2ℓ=(n′+f2ℓ−1++∞∑i=2ℓ+3ai(n)fi)+f2ℓ=(n′+f2ℓ−4)+f2ℓ+1++∞∑i=2ℓ+3ai(n)fi.(by Eq. 2.1) |
Noticing that n′+f2ℓ−4<f2ℓ−2, we have ai(n+f2ℓ)=ai(n′+f2ℓ−4) for all 0≤i≤2ℓ−2. In particular, a0(n+f2ℓ)=a0(n′+f2ℓ−4). By Proposition 2.4 and the inductive assumption,
a0(n+f2ℓ)≠a0(n)⟺a0(n′+f2ℓ−4)≠a0(n′)⟺n′∈{f2ℓ−32,f2ℓ−32−1}⟺Φℓ(n)=n′+f2ℓ−1∈{f2ℓ−32+f2ℓ−1,f2ℓ−32+f2ℓ−1−1}. |
By Eq (2.1), we have
f2ℓ+12=(f2ℓ−2+f2ℓ)/2=(f2ℓ−2+f2ℓ−2+f2ℓ−1)/2=f2ℓ−2+f2ℓ−12=f2ℓ−2+f2ℓ−4+f2ℓ−32=f2ℓ−1+f2ℓ−32. | (2.4) |
Then we obtain that
a0(n+f2ℓ)≠a0(n)⟺Φℓ(n)∈{f2ℓ+12,f2ℓ+12−1}. |
It follows from Proposition 2.4 that the result holds for k=ℓ.
(ⅱ) For any k≥1, let u=a2k(n)a2k+1(n)a2k+2(n). It follows from Proposition 2.4 that u∈{100,010,001}. The proof is divided into the following three cases.
● When u=001, we also have a2k+3(n)=a2k+4(n)=0. So
n+f2k+1=(2k−1∑i=0ai(n)fi+f2k+2++∞∑i=2k+5ai(n)fi)+f2k+1=(2k−1∑i=0ai(n)fi+f2k−2)+(f2k+3++∞∑i=2k+5ai(n)fi). |
Let n′=∑2k−1i=0ai(n)fi. Then a0(n)=a0(n′). Since n′<f2k and n′+f2k−2<f2k+1, we have ai(n′+f2k−2)=ai(n+f2k+1) for all 0≤i≤2k. Thus
a0(n+f2k+1)≠a0(n)⟺ai(n′+f2k−2)≠a0(n′)⟺n′=2k∑i=0ai(n′)fi∈{f2k−12,f2k−12−1} |
where in the last step we use Theorem 2.5(ⅰ). By Eq (2.1) and Eq (2.4),
f2k−12+f2k+2=f2k−12+f2k+1+f2k=f2k+32+f2k. |
So when u=001, a0(n+f2k+1)≠a0(n) if and only if
Φk(n)=n′+f2k+2∈{f2k+32+f2k,f2k+32+f2k−1}. |
● Suppose u=010. Applying Eq (2.1) twice, we obtain that
2f2k+1=f2k−2+f2k+f2k+1=f2k−2+f2k+2. |
Then
n+f2k+1=(2k−1∑i=0ai(n)fi+f2k+1++∞∑i=2k+3ai(n)fi)+f2k+1=(2k−1∑i=0ai(n)fi+f2k−2)+(f2k+2++∞∑i=2k+3ai(n)fi). |
Let n′=∑2k−1i=0ai(n)fi. Using Theorem 2.5(ⅰ), the same argument as in the case u=001 leads us to the fact that
a0(n+f2k+1)≠a0(n)⟺n′=2k∑i=0ai(n′)fi∈{f2k−12,f2k−12−1}⟺Φk(n)=n′+f2k+1∈{f2k+32,f2k+32−1}. |
● When u=100, we have a2k−2(n)=a2k−1(n)=0. Then
n+f2k+1=(2k−3∑i=0ai(n)fi+f2k++∞∑i=2k+3ai(n)fi)+f2k+1=(2k−3∑i=0ai(n)fi)+(f2k+2++∞∑i=2k+3ai(n)fi) |
which implies that a0(n+f2k+1)=a0(n).
To apply Theorem 2.5, we need to investigate the integers of the same truncated f-representations. The following two lemmas (Lemma 2.6 and Lemma 2.8) serve for this purpose.
For all k≥0, denote
E′k={x∈N:Φk(x)=f2k+32}andEk″ |
Let E_k = E'_k\cup E''_k = (x^{(k)}_j)_{j\ge 1} where x^{(k)}_1 < x^{(k)}_2 < x^{(k)}_3 < \dots . The first values of E_k are
\begin{align*} (x^{(k)}_j)_{j\ge 1} = & \left(\frac{f_{2k+3}}{2}, \, \frac{f_{2k+3}}{2}+f_{2k}, \, \frac{f_{2k+3}}{2}+f_{2k+3}, \, \frac{f_{2k+3}}{2}+f_{2k+4}, \, \frac{f_{2k+3}}{2}+f_{2k+5}, \, \right.\\ & \qquad\left.\frac{f_{2k+3}}{2}+f_{2k}+f_{2k+5}, \, \frac{f_{2k+3}}{2}+f_{2k+3}+f_{2k+5}, \, \frac{f_{2k+3}}{2}+f_{2k+6}, \, \dots\right). \end{align*} |
Lemma 2.6. Let k\ge 0 and x\in E'_k with x = x^{(k)}_j for some j\ge 2 . Then x-f_{2k+2} = x^{(k)}_{j-1}\in E_k .
Proof. Let x\in E''_{k} with x = x_{j}^{(k)} for some j\ge 2 . Note that \frac{f_{2k+3}}{2}+f_{2k} = f_{2k+2}+\frac{f_{2k-1}}{2} . By Proposition 2.3, have a_{2k+3}(x) = a_{2k+4}(x) = 0 . When 0 < b < f_{2k}-\frac{f_{2k-1}}{2} , we see
\Phi_{k}(x+b) = \left(\frac{f_{2k+3}}{2}+f_{2k}\right)+b < f_{2k+3} |
which implies that x+b\notin E_k . When f_{2k}-\frac{f_{2k-1}}{2}\leq b < f_{2k+2} ,
\Phi_{k}(x+b) = \left(\frac{f_{2k+3}}{2}+f_{2k}\right)+b - f_{2k+3} < \frac{f_{2k+3}}{2}. |
So x+b\notin E_k . Since \Phi_{k}(x+f_{2k+2}) = \frac{f_{2k+3}}{2} , we have x+f_{2k+2} = x_{j+1}^{(k)}\in E'_k .
Let x\in E'_{k} with x = x_{j}^{(k)} for some j\ge 1 . According to Proposition 2.3, a_{2k+3}(x)a_{2k+4}(x) = 00 , 10 or 01 , which can be divided into two sub-cases.
● a_{2k+3}(x)a_{2k+4}(x) = 00 . For 0 < b\leq f_{2k} , we see \Phi_{k}(x+b) = \Phi_{k}(x)+b = \frac{f_{2k+3}}{2}+b . Thus x+f_{2k} = x_{j+1}^{(k)}\in E''_k .
● a_{2k+3}(x)a_{2k+4}(x) = 01 or 10 . For 0 < b < f_{2k} , we see
\Phi_{k}(x+b) = \Phi_{k}(x)+b = \frac{f_{2k+3}}{2}+b < \frac{f_{2k+3}}{2}+f_{2k}. |
Thus x+b\notin E_k . For f_{2k}\leq b < f_{2k+2} , we have
\Phi_{k}(x+b) = \frac{f_{2k+3}}{2}+b-f_{2k+2}\in \left(\frac{f_{2k-1}}{2}, \, \frac{f_{2k+3}}{2}\right) |
which yields that x+b\notin E_k . Noting that \Phi_k(x+f_{2k+2}) = \Phi_k(x) = \frac{f_{2k+3}}{2} , we obtain that x+f_{2k+2} = x_{j+1}^{(k)}\in E'_k .
From the above argument, we see that if x = x_{j}^{(k)}\in E'_k for some j\ge 2 , then either x-f_{2k+2} = x_{j-1}^{(k)}\in E''_k or x-f_{2k+2} = x_{j-1}^{(k)}\in E'_k with a_{2k+3}(x-f_{2k+2})a_{2k+4}(x-f_{2k+2})\neq 00 . The result holds.
Remark 2.7. From the proof of Lemma 2.6, we see the gaps between two adjacent elements in E_k are f_{2k} and f_{2k+2} . That is x_{j+1}^{(k)}-x_{j}^{(k)} = f_{2k} or f_{2k+2} for all j\ge 1 . Moreover, the gaps between two adjacent elements in E'_k are f_{2k+2} and f_{2k+3} .
For all k\ge 0 , let
F_k = \left\{y\in\mathbb{N}\, :\, \Phi_{k}(y) = \frac{f_{2k+1}}{2}\right\} = (y^{(k)}_j)_{j\ge 1}\quad\text{and}\quad F'_k = \left\{y\in\mathbb{N}\, :\, \Phi_{k+1}(y) = \frac{f_{2k+1}}{2}\right\} |
where y^{(k)}_1 < y^{(k)}_2 < y^{(k)}_3 < \dots . Write F''_k = F_k-F'_k . The first values of F_k are
\begin{align*} (y_{j}^{(k)})_{j\ge 1} = & \left(\frac{f_{2k+1}}{2}, \, \frac{f_{2k+1}}{2}+f_{2k+3}, \, \frac{f_{2k+1}}{2}+f_{2k+4}, \, \frac{f_{2k+1}}{2}+f_{2k+5}, \, \right. \\ &\qquad \left.\frac{f_{2k+1}}{2}+f_{2k+5}+f_{2k+3}, \, \frac{f_{2k+1}}{2}+f_{2k+6}, \, \dots \right). \end{align*} |
Lemma 2.8. For any y\in F_k with y = y^{(k)}_j for some j\ge 1 , we have
y^{(k)}_{j+1} = \begin{cases} y+f_{2k+3}, & \mathit{\text{if}}\;y\in F'_{k};\\ y+f_{2k+2}, & \mathit{\text{if}}\;y\in F''_{k}. \end{cases} |
Proof. We prove the result by giving the construction of F_k . It clear that y_1^{(k)} = \frac{f_{2k+1}}{2} . Now suppose y = y_{j}^{(k)}\in F_k where j\ge 1 . According to Proposition 2.3, we see a_{2k+3}(y)a_{2k+4}(y) = 00 , 01 or 01 .
● a_{2k+3}(y)a_{2k+4}(y) = 00 , i.e., y\in F'_{k} . Note that \frac{f_{2k+1}}{2} = f_{2k-1}+\frac{f_{2k-3}}{2} . For 0 < b < f_{2k+3}-\frac{f_{2k+1}}{2} , we have \Phi_k(y+b) = \frac{f_{2k+1}}{2}+b , so y+b\notin F_k . For f_{2k+3}-\frac{f_{2k+1}}{2}\leq b < f_{2k+3} ,
\Phi_k(y+b) = \frac{f_{2k+1}}{2}+b-f_{2k+3} < \frac{f_{2k+1}}{2}, |
so y+b\notin F_{k} . Since \Phi_{k}(y+f_{2k+3}) = \Phi_{k}(y) , we obtain that y+f_{2k+3} = y_{j+1}^{(k)}\in F_{k}-F_{k}' .
● a_{2k+3}(y)a_{2k+4}(y) = 10 or 01 . For 0 < b < f_{2k+2}-\frac{f_{2k+1}}{2} , we have \Phi_k(y+b) = \frac{f_{2k+1}}{2}+b , so y+b\notin F_k . For f_{2k+2}-\frac{f_{2k+1}}{2}\leq b < f_{2k+2} , since
\Phi_k(y+b) = \frac{f_{2k+1}}{2}+b-f_{2k+2} < \frac{f_{2k+1}}{2}, |
we also have y+b\notin F_{k} . It follows from \Phi_k(y+f_{2k+2}) = \Phi_{k}(y) that y+f_{2k+2} = y_{j+1}^{(k)}\in F_k .
The result follows from the above two sub-cases.
Remark 2.9. From the proof of Lemma 2.8, we see the gaps between two adjacent elements in F_k are f_{2k+2} and f_{2k+3} . That is y_{j+1}^{(k)}-y_{j}^{(k)} = f_{2k+2} or f_{2k+3} for all j\ge 1 . Moreover, the gaps between two adjacent elements in F''_k are f_{2k+2} and f_{2k+4} .
The subsequences (s_{\frac{f_{2k+1}}{2}})_{k\ge 0} and (s_{\frac{f_{2k+1}}{2}-1})_{k\ge 0} can be determined according to the parity of k ; see Lemma 2.11. We start with an auxiliary lemma which concerns the parity of \frac{f_{2k+1}}{2} .
Lemma 2.10. For all k\ge 0 ,
(i) f_{2k}\equiv\begin{cases} 1, & \mathit{\text{if}}\; k\equiv 0 \; \mathit{\text{or}}\; 3 \pmod 4, \\ 3, & \mathit{\text{if}}\; k\equiv 1 \; \mathit{\text{or}}\; 2 \pmod 4, \end{cases}\pmod 4 ,
(ii) f_{2k+1}\equiv \begin{cases} 2, & \mathit{\text{if}}\;k\;\mathit{\text{is even}}, \\ 0, & \mathit{\text{if}}\;k\;\mathit{\text{is odd}}, \\ \end{cases}\pmod 4 .
Proof. (ⅰ) Note that f_0 = 1 and f_{2} = 3 . Since f_{2n} is odd for all n\ge 0 , using Eq. (2.1) twice, we have for all k\ge 2 ,
\begin{align*} f_{2k} & = f_{2k-2} + f_{2k-1} = 2f_{2k-2} + f_{2k-4} \equiv 2 + f_{2(k-2)}\pmod 4. \end{align*} |
The result follows by induction on k .
(ⅱ) The initial value is f_1 = 2 . Using Eq. (2.1) and the previous result (ⅰ), we have for all k\ge 1 ,
f_{2k+1} = f_{2k}+f_{2k-2}\equiv \begin{cases} 2, & k\equiv 0, 2 \pmod 4, \\ 0, & k\equiv 1, 3 \pmod 4, \end{cases}\pmod 4 |
which is the desired result.
In the calculation of H_{m, n} , we need to know s_n explicitly for some n . The next lemma determines the values of two sub-sequences {\bf{s}} .
Lemma 2.11. For all k\ge 0 ,
s_{\frac{f_{2k+1}}{2}} = \begin{cases} 1, & \mathit{\text{if}} \;k\; \mathit{\text{is odd}}, \\ 0, & \mathit{\text{if}} \;k \;\mathit{\text{is even}}, \end{cases}\quad \mathit{\text{and}}\quad s_{\frac{f_{2k+1}}{2}-1} = \begin{cases} 0, & \mathit{\text{if}}\; k\; \mathit{\text{is odd}}, \\ 1, & \mathit{\text{if}} \;k \;\mathit{\text{is even}}. \end{cases} |
Proof. By Eq (2.1), we obtain that for all k\ge 0 ,
\begin{align} \frac{f_{2k+1}}{2} & = (f_{2k-2} + f_{2k})/2 \\ & = (f_{2k-2} + f_{2k-2} + f_{2k-1})/2\\ & = f_{2k-2} + \frac{f_{2k-1}}{2} = \cdots = \sum\limits_{i = 0}^{k-1}f_{2i} + \frac{f_1}{2}. \end{align} | (2.5) |
When k is odd,
\begin{align} \frac{f_{2k+1}}{2} & = (f_{2k-2}+f_{2k-4}) + (f_{2k-6}+f_{2k-8})+\dots+(f_{4}+f_{2}) + f_0 + \frac{f_1}{2}\\ & = f_{2k-1} + f_{2k-5} +\dots +f_5 + f_1\quad \text{(by Eq. (2.1))}\\ & = \sum\limits_{i = 0}^{\frac{k-1}{2}}f_{4i+1}. \end{align} | (2.6) |
When k\ge 2 is even,
\begin{align} \frac{f_{2k+1}}{2} & = (f_{2k-2}+f_{2k-4}) + (f_{2k-6}+f_{2k-8})+\dots+(f_{2}+f_{0}) + \frac{f_1}{2}\\ & = f_{2k-1} + f_{2k-5} +\dots +f_3 + \frac{f_1}{2}\quad \text{(by Eq. (2.1))}\\ & = \sum\limits_{i = 0}^{\frac{k-2}{2}}f_{4i+3}+ \frac{f_1}{2} = \sum\limits_{i = 0}^{\frac{k-2}{2}}f_{4i+3} + f_0. \end{align} | (2.7) |
It follows from (2.6) and (2.7) that for all k\geq 0 ,
\begin{equation*} a_0\left(\frac{f_{2k+1}}{2}\right) = \begin{cases} 0, & \text{ if } k \text{ is odd}, \\ 1, & \text{ if } k \text{ is even}, \end{cases} \end{equation*} |
and
\begin{equation*} a_0\left(\frac{f_{2k+1}}{2}-1\right) = \begin{cases} 1, & \text{ if } k \text{ is odd}, \\ 0, & \text{ if } k \text{ is even}. \end{cases} \end{equation*} |
Then by Proposition 2.4, the result follows.
According to the values of the Hankel determinants of {\bf{s}} , we tile the integer lattice using the following parallelograms. Given a k\ge 0 , write the elements in E'_{k+1} , F''_{k} and E'_k in ascending order as follows:
E'_{k+1} = (\alpha_i)_{i\ge 1}, \quad F''_{k} = (\beta'_{i})_{i\ge 1}, \quad E'_{k} = (\gamma_{i})_{i\ge 1}. |
Moreover, let \beta_i = \beta'_{i}+f_{2k} for all i\ge 1 . We define three different types of parallelograms: for i\ge 1 ,
\begin{equation} \begin{aligned} U_{k, i} & = \left\{(m, n)\in\mathbb{N}^2\, :\, \, f_{2k}\leq n < f_{2k+3}, \, \alpha_{i}-f_{2k+2} < n+m\leq \alpha_i\right\}, \\ V_{k, i} & = \left\{(m, n)\in\mathbb{N}^2\, :\, \, f_{2k}\leq n < f_{2k+2}, \, \beta_{i} < n+m\leq \beta_{i}+f_{2k+1}\right\}, \\ T_{k, i} & = \left\{(m, n)\in\mathbb{N}^2\, :\, \, f_{2k+1}\leq n < f_{2k+2}, \, \gamma_{i}-f_{2k} < n+m\leq \gamma_{i}\right\}; \end{aligned} \end{equation} | (3.1) |
see Figure 1. Let U_k = \cup_{i\ge 1}U_{k, i} , V_{k} = \cup_{i\ge 1}V_{k, i} and T_{k} = \cup_{i\ge 1}T_{k, i} .
Proposition 3.1. The parallelograms \{U_{k, i}\} , \{V_{k, i}\} , and \{T_{k, i}\} introduce a partition of pairs of positive integers. Namely, \mathbb{N}\times\mathbb{N}_{\ge 1} = \bigsqcup_{k\geq 0} (U_{k}\sqcup V_{k}\sqcup T_{k}) where \sqcup denotes the disjoint union.
Proof. Let m\ge 0 and n\ge 1 be two integers. Since (f_k)_{k\ge 0} and (\gamma_{k})_{k\ge 1} are two increasing unbounded non-negative integer sequences, there exist k\ge 0 and \ell\ge 1 such that f_{2k}\le n < f_{2k+2} and \gamma_{\ell-1} < n+m\leq \gamma_{\ell} where \gamma_0: = 0 . The result clearly holds when \ell = 1 . Now we assume that \ell\ge 2 . From the proof of Lemma 2.6 we see that \gamma_{\ell}-\gamma_{\ell-1} = f_{2k+2} or f_{2k+3} for all \ell\ge 2 . When \gamma_{\ell}-f_{2k} < n+m\leq \gamma_{\ell} , we have
(m, n)\in\begin{cases} U_{k-1}, & \text{ if }f_{2k}\leq n < f_{2k+1};\\ T_{k}, & \text{ if }f_{2k+1}\leq n < f_{2k+2}; \end{cases} |
see also Figure 5. When \gamma_{\ell-1} < n+m\leq \gamma_{\ell}-f_{2k} , we have the following two cases:
Case 1: \gamma_{\ell}-\gamma_{\ell-1} = f_{2k+2} . In this case, we shall verify that (m, n)\in V_k . To do this, we only need to show that \gamma_{\ell-1}-f_{2k}\in F''_{k} . Since \gamma_{\ell-1}\in E'_{k} , we have \Phi_{k}(\gamma_{\ell-1}) = \frac{f_{2k+3}}{2} and \Phi_{k}(\gamma_{\ell-1}-f_{2k}) = \frac{f_{2k+3}}{2}-f_{2k} = \frac{f_{2k+1}}{2} . So (\gamma_{\ell-1}-f_{2k})\in F_{k} . Suppose on the contrary that (\gamma_{\ell-1}-f_{2k})\in F'_{k} . Then \Phi_{k+1}(\gamma_{\ell-1}-f_{2k}) = \frac{f_{2k+1}}{2} and \Phi_{k+1}(\gamma_{\ell-1}) = \frac{f_{2k+3}}{2} . This implies \Phi_{k}(\gamma_{\ell-1}+f_{2k+2}) = \frac{f_{2k+1}}{2} and (\gamma_{\ell-1}+f_{2k+2})\notin E'_k . Note that in this case \gamma_{\ell} = \gamma_{\ell-1}+f_{2k+2} . We conclude that \gamma_{\ell}\notin E'_{k} which is a contradiction. Hence, (\gamma_{\ell-1}-f_{2k})\in F''_{k} . The result follows.
Case 2: \gamma_{\ell}-\gamma_{\ell-1} = f_{2k+3} . We assert that, in this case, \gamma_{\ell-1}-f_{2k}\in F'_{k} . Since \gamma_{\ell-1}\in E'_{k} , we have \Phi_{k}(\gamma_{\ell-1}) = \frac{f_{2k+3}}{2} . Consequently, \Phi_{k}(\gamma_{\ell-1}-f_{2k}) = \frac{f_{2k+1}}{2} and (\gamma_{\ell-1}-f_{2k})\in F_{k} . Suppose (\gamma_{\ell-1}-f_{2k})\in F''_{k} . Then \Phi_{k}(\gamma_{\ell-1}) = \frac{f_{2k+3}}{2} and \Phi_{k+1}(\gamma_{\ell-1})\neq \frac{f_{2k+3}}{2} . It follows that \Phi_{k}(\gamma_{\ell-1}+f_{2k+3}) = \frac{f_{2k+3}}{2}+f_{2k} . Since \gamma_{\ell-1}+f_{2k+3} = \gamma_{\ell} , we obtain that \gamma_{\ell}\notin E'_{k} which is a contradiction. Now we have \gamma_{\ell-1}-f_{2k}\in F'_{k} . This yields that \Phi_{k+1}(\gamma_{\ell-1}) = \frac{f_{2k+3}}{2} . Observing that \Phi_{k+1}(\gamma_{\ell}-f_{2k}) = \Phi_{k+1}(\gamma_{\ell-1}+f_{2k+2}) = \frac{f_{2k+5}}{2} , we see \gamma_{\ell}-f_{2k}\in E'_{k+1} . So (m, n)\in U_k .
In this section, we use the Theorem 2.5 to show the determinant value inside U_k , V_k , T_k is 0. For some integer k\ge 0 , we prove the relationship between the determinant value of the boundary of U_k , V_k , T_k . We assert that as long as we know one value of U_k ( V_k or T_k ), we can know all its values.
The Hankel determinant H_{m, n} vanishes if (m, n) is not on the boundary of any parallelogram U_{k, i} , V_{k, i} or T_{k, i} .
Lemma 4.1. Let m\ge 1 and n\ge 0 be two integer.
(i) If (m, n) is inside V_{k, i} for some k\ge 0 and i\ge 1 , i.e.,
\begin{cases} f_{2k}+1\leq n < f_{2k+2}-1, \\ \beta_i + 1 < n+m \leq \beta_i + f_{2k+1} -1, \end{cases} |
then H_{m, n} = 0 .
(ii) If (m, n) is inside T_{k, i} for some k\ge 0 and i\ge 1 , i.e.,
\begin{cases} f_{2k+1}+1\leq n < f_{2k+2}-1, \\ \gamma_i - f_{2k} + 1 < n+m \leq \gamma_i -1, \end{cases} |
then H_{m, n} = 0 .
(iii) If (m, n) is inside U_{k, i} for some k\ge 0 and i\ge 1 , i.e.,
\begin{cases} f_{2k}+1\leq n < f_{2k+3}-1, \\ \alpha_i - f_{2k+2} + 1 < n+m \leq \alpha_i -1, \end{cases} |
then H_{m, n} = 0 .
Proof. Let A_{m+i} be the i -th row of H_{m, n} . Then
\begin{align*} H_{m, n}& = \det \begin{pmatrix} s_m & s_{m+1}& \cdots & s_{m+n-1}\\ s_{m+1} & s_{m+2} &{\mathinner{\mkern2mu\raise1pt\hbox{.}\mkern2mu \raise4pt\hbox{.}\mkern2mu\raise7pt\hbox{.}\mkern1mu}} & \vdots \\ \vdots & {\mathinner{\mkern2mu\raise1pt\hbox{.}\mkern2mu \raise4pt\hbox{.}\mkern2mu\raise7pt\hbox{.}\mkern1mu}} & {\mathinner{\mkern2mu\raise1pt\hbox{.}\mkern2mu \raise4pt\hbox{.}\mkern2mu\raise7pt\hbox{.}\mkern1mu}} &\vdots \\ s_{m+n-1}& \cdots & \cdots & s_{m+2n-2} \end{pmatrix} = \det \begin{pmatrix} A_m\\ A_{m+1}\\ \vdots\\ A_{m+n-1} \end{pmatrix}. \end{align*} |
(ⅰ) When m\leq \beta'_i+1 , recall that \beta'_i = \beta_i-f_{2k}\in F''_{k} . Since n\leq f_{2k+2}-2 , by Lemma 2.8, we have \Phi_{k}(\beta'_k + j)\neq \frac{f_{2k+1}}{2} or \frac{f_{2k+1}}{2}-1 for all 1\leq j\leq n . Then it follows from Theorem 2.5(ⅰ) that
\begin{align*} A_{\beta'_i+1}& = (s_{\beta'_i+1}, \, s_{\beta'_i+2}, \, \dots, \, s_{\beta'_i+n})\\ & = (s_{\beta_i+1}, \, s_{\beta_i+2}, \, \dots, \, s_{\beta_i+n}) = A_{\beta_i+1}, \end{align*} |
which gives H_{m, n} = 0 . When m > \beta'_i+1 , note that n+m\leq \beta'_k + f_{2k+2}-1 . By Lemma 2.8, we have \Phi_{k}(m + j)\neq \frac{f_{2k+1}}{2} or \frac{f_{2k+1}}{2}-1 for all 1\leq j\leq n . Then it follows from Theorem 2.5(ⅰ) that
\begin{align*} A_{m}& = (s_{m}, \, s_{m+1}, \, \dots, \, s_{m+n-1})\\ & = (s_{m+f_{2k}}, \, s_{m+f_{2k}+1}, \, \dots, \, s_{m+f_{2k}+n-1}) = A_{m+f_{2k}}. \end{align*} |
So H_{m, n} = 0 .
(ⅱ) Recall that \gamma_i\in E'_k and by Lemma 2.6, \gamma_i and \gamma_i-f_{2k+2} are adjacent elements in E_k . Let
r = \begin{cases} \gamma_i - f_{2k+2} + 1 - m, & \text{ if } m\le \gamma_i - f_{2k+2} + 1, \\ 0, & \text{ if } m > \gamma_i - f_{2k+2} + 1. \end{cases} |
Combining Lemma 2.6 and Theorem 2.5(ⅱ), we have A_{m+r} = A_{m+r+f_{2k+1}} which means H_{m, n} = 0 .
(ⅲ) Recall that \alpha_i\in E'_{k+1} and by Lemma 2.6, \alpha_i and \alpha_i-f_{2k+4} are adjacent elements in E_{k+1} . When m\le \alpha_i - f_{2k+4} + 1 , note that \alpha_i-f_{2k+4}+n < \alpha_i - f_{2k+2} - 1 . By Theorem 2.5(ⅱ), we have
\begin{align*} A_{\alpha_i-f_{2k+4}+1} & = (s_{\alpha_i-f_{2k+4}+1}, \, s_{\alpha_i-f_{2k+4}+2}, \, \dots, \, s_{\alpha_i-f_{2k+4}+n})\\ & = (s_{\alpha_i-f_{2k+2}+1}, \, s_{\alpha_i-f_{2k+2}+2}, \, \dots, \, s_{\alpha_i-f_{2k+2}+n}) = A_{\alpha_i-f_{2k+2}+1}. \end{align*} |
Thus H_{m, n} = 0 . When m > \alpha_i - f_{2k+4} + 1 , since n+m-1\leq \alpha_i-2 , by Theorem 2.5(ⅱ), we obtain that
\begin{align*} A_{m} & = (s_{m}, \, s_{m+1}, \, \dots, \, s_{m+n-1})\\ & = (s_{m+f_{2k+3}}, \, s_{m+f_{2k+3}+1}, \, \dots, \, s_{m+f_{2k+3}+n-1}) = A_{m+f_{2k+3}} \end{align*} |
which also implies H_{m, n} = 0 .
We first deal with the Hankel determinants H_{m, n} on the horizontal edges with n = f_{2k} and f_{2k+1} where k\ge 0 .
Lemma 4.2. Let k\ge 0 and i\ge 1 .
(i) \textrm{(Bottom edge of $ V_{k, i} $)} H_{\beta'_i+r, \, f_{2k}} = H_{\beta'_i+1, \, f_{2k}} for all 1\leq r\leq f_{2k+1} .
(ii) \textrm{(Bottom edge of $ U_{k, i} $)} H_{\alpha_i-f_{2k+3}+r, \, f_{2k}} = H_{\alpha_i-f_{2k}, \, f_{2k}} for all 1\leq r\leq f_{2k+2} .
(iii) \textrm{(Bottom edge of $ T_{k, i} $)} H_{\gamma_{i}-f_{2k+2}+r, \, f_{2k+1}} = (-1)^{r+1}H_{\gamma_{i}-f_{2k+1}, \, f_{2k+1}} for all 1\leq r \leq f_{2k} with \gamma_{i}-f_{2k+2}+r\ge 0 .
Proof. (ⅰ) Let A_j = (s_{\beta'_i+j}, \, s_{\beta'_i+j+1}, \, \dots, \, s_{\beta'_i+j+f_{2k}-1}) . Then for 1\leq j < f_{2k+1} ,
H_{\beta'_i+j, \, f_{2k}} = \det\begin{pmatrix} A_j\\ A_{j+1}\\ \vdots\\ A_{f_{2k}+j-1} \end{pmatrix}\quad\text{and}\quad H_{\beta'_i+j+1, \, f_{2k}} = \det\begin{pmatrix} A_{j+1}\\ A_{j+2}\\ \vdots\\ A_{f_{2k}+j} \end{pmatrix}. |
Recall that \beta'_i\in F''_k . By Lemma 2.8, since j+f_{2k}-1\leq f_{2k+2}-2 , we see \Phi_k(\beta'_i+\ell)\neq \frac{f_{2k+1}}{2} or \frac{f_{2k+1}}{2}-1 for all 1\leq \ell \leq f_{2k+2}-2 . Applying Theorem 2.5(ⅰ), we have
\begin{align*} A_j & = (s_{\beta'_i+j}, \, s_{\beta'_i+j+1}, \, \dots, \, s_{\beta'_i+j+f_{2k}-1})\\ & = (s_{\beta'_i+j+f_{2k}}, \, s_{\beta'_i+j+1+f_{2k}}, \, \dots, \, s_{\beta'_i+j+2f_{2k}-1}) = A_{f_{2k}+j}. \end{align*} |
Therefore, for 1\leq j < f_{2k+1} ,
H_{\beta'_i+j, \, f_{2k}} = \begin{vmatrix} A_j\\ A_{j+1}\\ \vdots\\ A_{f_{2k}+j-1} \end{vmatrix} = \begin{vmatrix} A_{f_{2k}+j}\\ A_{j+1}\\ \vdots\\ A_{f_{2k}+j-1} \end{vmatrix} = (-1)^{f_{2k}-1} \begin{vmatrix} A_{j+1}\\ A_{j+2}\\ \vdots\\ A_{f_{2k}+j} \end{vmatrix} = H_{\beta'_i+j+1, \, f_{2k}} |
where the last equality follows from Lemma 2.10(ⅰ).
(ⅱ) Recall that \alpha_i\in E'_{k+1} and \Phi_{k+1}(\alpha_i) = \frac{f_{2k+5}}{2} . Let y = \alpha_i-f_{2k+3} . Then \Phi_{k+1}(y) = \frac{f_{2k+1}}{2} and y\in F'_k . Let B_j = (s_{y+j}, \, s_{y+j+1}, \, \dots, \, s_{y+j+f_{2k}-1}) . Then for 1\leq j < f_{2k+2} ,
H_{y+j, \, f_{2k}} = \det\begin{pmatrix} B_j\\ B_{j+1}\\ \vdots\\ B_{f_{2k}+j-1} \end{pmatrix}\quad\text{and}\quad H_{y+j+1, \, f_{2k}} = \det\begin{pmatrix} B_{j+1}\\ B_{j+2}\\ \vdots\\ B_{f_{2k}+j} \end{pmatrix}. |
Since j+f_{2k}-1\leq f_{2k+3}-2 , by Lemma 2.8 and Theorem 2.5(ⅰ),
\begin{align*} B_j & = (s_{y+j}, \, s_{y+j+1}, \, \dots, \, s_{y+j+f_{2k}-1})\\ & = (s_{y+j+f_{2k}}, \, s_{y+j+1+f_{2k}}, \, \dots, \, s_{y+j+2f_{2k}-1}) = B_{f_{2k}+j}. \end{align*} |
Therefore, for 1\leq j < f_{2k+2} ,
H_{y+j, \, f_{2k}} = (-1)^{f_{2k}-1}H_{y+j+1, \, f_{2k}} = H_{y+j+1, \, f_{2k}} |
where the last equality follows from Lemma 2.10(ⅰ).
(ⅲ) Recall that \gamma_i\in E'_{k} . By Lemma 2.6, g: = \gamma_i-f_{2k+2}\in E_{k} . Write
A_{g+j} = (s_{g+j}, \, s_{g+j+1}, \, \dots, \, s_{g+j+f_{2k+1}-1}). |
For 1\leq r < f_{2k} ,
H_{g+r, \, f_{2k+1}} = \begin{vmatrix} A_{g+r}\\ A_{g+r+1}\\ \vdots\\ A_{g+r+f_{2k+1}-1} \end{vmatrix}\quad\text{and}\quad H_{g+r+1, \, f_{2k+1}} = \begin{vmatrix} A_{g+r+1}\\ A_{g+r+2}\\ \vdots\\ A_{g+r+f_{2k+1}} \end{vmatrix}. |
By Theorem 2.5, A_{g+r} = A_{g+r+f_{2k+1}} . Then using Lemma 2.10, for all 1\leq r < f_{2k} ,
H_{g+r, \, f_{2k+1}} = (-1)^{f_{2k+1}-1}H_{g+r+1, \, f_{2k+1}} = -H_{g+r+1, \, f_{2k+1}} |
and H_{g+r, \, f_{2k+1}} = (-1)^{f_{2k}-r}H_{g+r+f_{2k}, \, f_{2k+1}} = (-1)^{1+r}H_{g+r+f_{2k}, \, f_{2k+1}}.
In fact, for all i\ge 1 , the Hankel determinants on the bottom of U_{k, i} and V_{k, i} take the same value which depends only on k . The following lemma helps us to connect the determinants on the bottom of U_{k, *} and V_{k, *} .
Lemma 4.3. Let k\ge 0 and i\ge 1 . If \gamma_{i+1}-\gamma_i = f_{2k+3} , then H_{\gamma_i+f_{2k}+1, \, f_{2k}} = H_{\gamma_{i+1}-f_{2k}+1, \, f_{2k}} . If \gamma_{i+1}-\gamma_i = f_{2k+2} , then H_{\gamma_i+1, \, f_{2k}} = H_{\gamma_{i+1}-f_{2k}+1, \, f_{2k}} .
Proof. Suppose \gamma_{i+1}-\gamma_i = f_{2k+3} . Then \Phi_{k}(\gamma_{i}+f_{2k}) = \frac{f_{2k+3}}{2}+f_{2k} . Since 3f_{2k} = f_{2k+2}+f_{2k-1} < f_{2k+3} , by Theorem 2.5(ⅱ), we have
\begin{align*} \begin{pmatrix} s_{\gamma_i+f_{2k}+1} & \cdots & s_{\gamma_i+3f_{2k}-1} \end{pmatrix} & = \begin{pmatrix} s_{\gamma_i+f_{2k}+1+f_{2k+1}} & \cdots & s_{\gamma_i+3f_{2k}-1+f_{2k+1}}\end{pmatrix}\\ & = \begin{pmatrix} s_{\gamma_{i+1}-f_{2k}+1} & \cdots & s_{\gamma_{i+1}+f_{2k}-1} \end{pmatrix}. \end{align*} |
Therefore
\begin{align*} H_{\gamma_i+f_{2k}+1, \, f_{2k}}& = \begin{vmatrix} s_{\gamma_i+f_{2k}+1} & \cdots & s_{\gamma_i+2f_{2k}}\\ \vdots & \vdots & \vdots\\ s_{\gamma_i+2f_{2k}} & \cdots & s_{\gamma_i+3f_{2k}-1} \end{vmatrix} = \begin{vmatrix} s_{\gamma_{i+1}-f_{2k}+1} & \cdots & s_{\gamma_{i+1}}\\ \vdots & \vdots & \vdots\\ s_{\gamma_{i+1}} & \cdots & s_{\gamma_{i+1}+f_{2k}-1} \end{vmatrix}\\ & = H_{\gamma_{i+1}-f_{2k}+1, \, f_{2k}}. \end{align*} |
When \gamma_{i+1}-\gamma_i = f_{2k+2} , we have \Phi_{k}(\gamma_i) = \frac{f_{2k+3}}{2} . By Theorem 2.5(ⅱ),
\begin{align*} \begin{pmatrix} s_{\gamma_i+1} & \cdots & s_{\gamma_i+2f_{2k}-1} \end{pmatrix} & = \begin{pmatrix} s_{\gamma_i+1+f_{2k+1}} & \cdots & s_{\gamma_i+2f_{2k}-1+f_{2k+1}}\end{pmatrix}\\ & = \begin{pmatrix} s_{\gamma_{i+1}-f_{2k}+1} & \cdots & s_{\gamma_{i+1}+f_{2k}-1} \end{pmatrix}. \end{align*} |
So H_{\gamma_i+1, \, f_{2k}} = H_{\gamma_{i+1}-f_{2k}+1, \, f_{2k}} .
Next we give the connection between T_{k, i} and T_{k, i+1} .
Lemma 4.4. For all i\ge 1 , H_{\gamma_i-f_{2k+1}, \, f_{2k+1}} = H_{\gamma_{i+1}-f_{2k+1}, \, f_{2k+1}} .
Proof. If \gamma_{i+1}-\gamma_i = f_{2k+3} , then \Phi_{k+1}(\gamma_i) = \frac{f_{2k+3}}{2} and \Phi_{k+1}(\gamma_i+f_{2k+1}) < \frac{f_{2k+5}}{2} . By Theorem 2.5(ⅱ), we have
\begin{align*} \begin{pmatrix} s_{\gamma_{i}-f_{2k+1}} & \cdots & s_{\gamma_{i}+f_{2k+1}-2} \end{pmatrix} & = \begin{pmatrix} s_{\gamma_{i}-f_{2k+1}+f_{2k+3}} & \cdots & s_{\gamma_{i}+f_{2k+1}-2+f_{2k+3}} \end{pmatrix} \\ & = \begin{pmatrix} s_{\gamma_{i+1}-f_{2k+1}} & \cdots & s_{\gamma_{i+1}+f_{2k+1}-2} \end{pmatrix}. \end{align*} |
Consequently, H_{\gamma_i-f_{2k+1}, \, f_{2k+1}} = H_{\gamma_{i+1}-f_{2k+1}, \, f_{2k+1}} .
If \gamma_{i+1}-\gamma_i = f_{2k+2} , then \Phi_{k+1}(\gamma_i) = \frac{f_{2k+3}}{2}+f_{2k+3} or \frac{f_{2k+3}}{2}+f_{2k+4} . By Theorem 2.5(ⅰ), we have
\begin{align*} \begin{pmatrix} s_{\gamma_{i}-f_{2k+1}} & \cdots & s_{\gamma_{i}+f_{2k+1}-2} \end{pmatrix} & = \begin{pmatrix} s_{\gamma_{i}-f_{2k+1}+f_{2k+2}} & \cdots & s_{\gamma_{i}+f_{2k+1}-2+f_{2k+2}} \end{pmatrix} \\ & = \begin{pmatrix} s_{\gamma_{i+1}-f_{2k+1}} & \cdots & s_{\gamma_{i+1}+f_{2k+1}-2} \end{pmatrix}. \end{align*} |
Consequently, H_{\gamma_i-f_{2k+1}, \, f_{2k+1}} = H_{\gamma_{i+1}-f_{2k+1}, \, f_{2k+1}} .
According to Lemma 4.3 and Lemma 4.4, the values of the determinants on the bottom edges of U_{k, i} and V_{k, i} only depends on k . We improve Lemma 4.2 to the following proposition.
Proposition 4.5. Let k\ge 0 . For all i\ge 1 ,
(i) \textrm{(Bottom edges of $ U_{k, i} $ and $ V_{k, i} $)} for all 1\leq r\leq f_{2k+1} and 1\leq r'\leq f_{2k+2} ,
H_{\alpha_i-f_{2k+3}+r', \, f_{2k}} = H_{\beta'_i+r, \, f_{2k}} = H_{\alpha_1-f_{2k}, \, f_{2k}}; |
(ii) \textrm{(Bottom edge of $ T_{k, i} $)} H_{\gamma_{i}-f_{2k+2}+r, \, f_{2k+1}} = (-1)^{r+1}H_{\gamma_{1}-f_{2k+1}, \, f_{2k+1}} for all 1\leq r \leq f_{2k} with \gamma_{i}-f_{2k+2}+r\ge 0 .
Proof. Since \alpha_i-f_{2k+2}\in E'_k and \beta'_i+f_{2k}\in E'_k , Lemma 4.3 shows that the values of two determinants on the bottom edge of two adjacent parallelograms in \{U_{k, j}\}_{j\ge 1}\cup\{V_{k, j}\}_{j\ge 1} are the same. Then Lemma 4.2 implies the result (ⅰ). The result (ⅱ) follows from Lemma 4.2(ⅲ) and Lemma 4.4.
Lemma 4.6. Let k\ge 0 and i\ge 1 . For all 0\leq r\leq f_{2k+2}-1 with \alpha_i-f_{2k+4}+2+r\ge 0 ,
(i) \textrm{(Right edge of $ U_{k, i} $)} H_{\alpha_i-f_{2k+3}+1+r, f_{2k+3}-1-r} = (-1)^{rk}(-1)^{\frac{r(r-1)}{2}}H_{\alpha_i-f_{2k+3}+1, f_{2k+3}-1} ,
(ii) \textrm{(Left edge of $ U_{k, i} $)} H_{\alpha_i-f_{2k+4}+2+r, f_{2k+3}-1-r} = (-1)^{rk}(-1)^{\frac{r(r-1)}{2}}H_{\alpha_i-f_{2k+3}+1, f_{2k+3}-1} ,
(iii) \textrm{(Upper edge of $ U_{k, i} $)} H_{\alpha_i-f_{2k+4}+2+r, f_{2k+3}-1} = (-1)^r H_{\alpha_i-f_{2k+3}+1, f_{2k+3}-1} .
Proof. Write y = \alpha_i-f_{2k+3} . Recall that \alpha_i\in E'_{k+1} . So \Phi_{k+1}(y) = \frac{f_{2k+1}}{2} and y\in F'_{k} .
(ⅰ) For 0\le r < f_{2k+2} , let A_{y+r+j} be the j -th column of M_{y+1+r, \, f_{2k+3}-1-r} . Applying Lemma 2.8 and Theorem 2.5(ⅰ), we see s_{y+r+\ell} = s_{y+r+\ell+f_{2k}} for 1\le \ell \le f_{2k+3}-r-2 and s_{y+f_{2k+3}-1}\neq s_{y+f_{2k+3}-1+f_{2k}} . Then Proposition 2.4 and Lemma 2.11 yields s_{y+f_{2k+3}-1}-s_{y+f_{2k+3}-1+f_{2k}} = (-1)^{k} . Therefore,
A_{y+r+1} -A_{y+r+f_{2k}} = \begin{pmatrix} s_{y+r+1}\\ s_{y+r+2} \\ \vdots\\ s_{y+f_{2k+3}-2}\\ s_{y+f_{2k+3}-1} \end{pmatrix}-\begin{pmatrix} s_{y+r+1+f_{2k}}\\ s_{y+r+2+f_{2k}} \\ \vdots\\ s_{y+f_{2k+3}-2+f_{2k}}\\ s_{y+f_{2k+3}-1+f_{2k}} \end{pmatrix} = \begin{pmatrix} 0\\ 0\\ \vdots\\ 0\\ (-1)^{k} \end{pmatrix} |
and
\begin{align} H_{y+1+r, \, f_{2k+3}-1-r} & = \begin{vmatrix} A_{y+r+1} & A_{y+r+2} & \dots & A_{y+f_{2k+3}-1} \end{vmatrix}\\ & = \begin{vmatrix} (A_{y+r+1}-A_{y+r+f_{2k}}) & A_{y+r+2} & \dots & A_{y+f_{2k+3}-1} \end{vmatrix}\\ & = \begin{vmatrix} {\bf{0}}_{ f_{2k+3}-2-r , 1}& M_{y+r+2, f_{2k+3}-r-2}\\ (-1)^k& * \end{vmatrix}\\ & = (-1)^k(-1)^{1+f_{2k+3}-1-r}H_{y+r+2, f_{2k+3}-r-2}\\ & = (-1)^{k+r}H_{y+r+2, f_{2k+3}-r-2} \end{align} | (4.1) |
where in the last equality we apply Lemma 2.10 and {\bf{0}}_{i, j} denotes the i\times j zero matrix. It follows from Eq (4.1) that
\begin{align*} H_{y+1, \, f_{2k+3}-1} & = (-1)^{k}H_{y+2, \, f_{2k+3}-2} = (-1)^{k}(-1)^{k+1}H_{y+3, \, f_{2k+3}-3} \\ & = (-1)^{k}(-1)^{k+1}\dots(-1)^{k+r-1}H_{y+1+r, \, f_{2k+3}-1-r}\\ & = (-1)^{rk}(-1)^{\frac{r(r-1)}{2}}H_{y+1+r, \, f_{2k+3}-1-r}. \end{align*} |
(ⅱ) Let B_{y-f_{2k+2}+1+r+j} be the j -th row of M_{y-f_{2k+2}+2+r, f_{2k+3}-1-r} . Combining Lemma 2.8, Theorem 2.5(ⅰ), Proposition 2.4 and Lemma 2.11, a similar argument as above yields
\begin{align} H_{y-f_{2k+2}+2+r, f_{2k+3}-1-r} & = \begin{vmatrix} B_{y-f_{2k+2}+1+r+1}\\ \vdots\\ B_{y}\\ \vdots\\ B_{y+f_{2k}} \end{vmatrix} = \begin{vmatrix} B_{y-f_{2k+2}+1+r+1}\\ \vdots\\ B_{y}\\ \vdots\\ B_{y+f_{2k}}-B_{y} \end{vmatrix}\\ & = \begin{vmatrix} * & M_{y-f_{2k+2}+2+(r+1), f_{2k+3}-1-(r+1)}\\ (-1)^k& {\bf{0}}_{ 1 , f_{2k+3}-2-r} \end{vmatrix}\\ & = (-1)^{k+r}H_{y-f_{2k+2}+2+(r+1), f_{2k+3}-1-(r+1)}. \end{align} | (4.2) |
Applying Eq (4.2), we have
\begin{align*} H_{y-f_{2k+2}+2+r, f_{2k+3}-1-r} & = (-1)^{k+r}(-1)^{k+r+1}\cdots(-1)^{k+f_{2k+2}-2}H_{y+1, \, f_{2k}}\\ & = (-1)^{\frac{(2k+r+f_{2k+2}-2)(f_{2k+2}-r-1)}{2}}H_{y+1, \, f_{2k}}\\ & = (-1)^{\frac{(2k+r+f_{2k+2}-2)(f_{2k+2}-r-1)}{2}}H_{y+f_{2k+2}, \, f_{2k}}\;\;\;\;\;\;\;\;\;\;\text{(by Lemma 4.2(ii))}\\ & = (-1)^{rk}(-1)^{\frac{r(r-1)}{2}}H_{y+1, \, f_{2k+3}-1}.\;\;\;\;\;\;\;\;\;\;\;\;\;\;\;\;\; \text{(by Lemma 4.6(i))} \end{align*} |
(ⅲ) Let C_j be the j -th column of M_{y-f_{2k+2}+2+r, f_{2k+3}-1} . Then
\begin{align*} H_{y-f_{2k+2}+2+r, f_{2k+3}-1} & = \det(C_1, C_2, \dots, C_{f_{2k+3}-1})\\ & = \det(C_1, C_2, \dots, C_{f_{2k+3}-1-r}, C'_{1}, C'_{2}, \dots, C'_{r}) \end{align*} |
where C'_{p} = C_{f_{2k+3}-1-r+p}-C_{f_{2k+3}-1-r+p-f_{2k}} for 1\le p \le r . According to Lemma 2.8, Theorem 2.5(ⅰ), we have s_{y+\ell} = s_{y+\ell+f_{2k}} for all 1\leq \ell \leq f_{2k+3}-2 and f_{2k+3}+1\leq \ell \leq f_{2k+3}+r-2 . By Proposition 2.4 and Lemma 2.11, we obtain that s_{y+f_{2k+3}-1+f_{2k}}-s_{y+f_{2k+3}-1} = (-1)^{k+1} and s_{y+f_{2k+3}+f_{2k}}-s_{y+f_{2k+3}} = (-1)^{k} . Thus
(C'_{1}, C'_{2}, \dots, C'_{r}) = \begin{pmatrix} {\bf{0}}_{ f_{2k+3}-1-r, r}\\ X \end{pmatrix} |
where X is the r\times r matrix
\begin{pmatrix} 0 & \cdots & 0 & (-1)^{k+1}\\ \vdots & {\mathinner{\mkern2mu\raise1pt\hbox{.}\mkern2mu \raise4pt\hbox{.}\mkern2mu\raise7pt\hbox{.}\mkern1mu}} & {\mathinner{\mkern2mu\raise1pt\hbox{.}\mkern2mu \raise4pt\hbox{.}\mkern2mu\raise7pt\hbox{.}\mkern1mu}} & (-1)^k\\ \vdots & {\mathinner{\mkern2mu\raise1pt\hbox{.}\mkern2mu \raise4pt\hbox{.}\mkern2mu\raise7pt\hbox{.}\mkern1mu}} & {\mathinner{\mkern2mu\raise1pt\hbox{.}\mkern2mu \raise4pt\hbox{.}\mkern2mu\raise7pt\hbox{.}\mkern1mu}} & \vdots\\ (-1)^{k+1} & (-1)^k & \cdots & 0 \end{pmatrix}. |
Expanding by the last r columns, we have
\begin{align*} H_{y-f_{2k+2}+2+r, f_{2k+3}-1} & = \det\begin{pmatrix} M_{y-f_{2k+2}+2+r, f_{2k+3}-1-r} & {\bf{0}}_{f_{2k+3}-1-r, r}\\ * & X \end{pmatrix}\\ & = (-1)^{(k+1)r}(-1)^{\frac{(r-1)r}{2}}H_{y-f_{2k+2}+2+r, f_{2k+3}-1-r}\\ & = (-1)^{(k+1)r}(-1)^{\frac{(r-1)r}{2}}(-1)^{rk}(-1)^{\frac{r(r-1)}{2}}H_{y+1, f_{2k+3}-1} \;\;\;\;\;\;\text{(by Lemma 4.6(ii))}\\ & = (-1)^r H_{y+1, f_{2k+3}-1}. \end{align*} |
Remark 4.7. From Proposition 4.5(ⅰ) and Lemma 4.6, Hankel determinants on the boundary of U_{k, i} can be determined by H_{\alpha_1-f_{2k+3}+1, f_{2k+3}-1} = H_{\frac{f_{2k+1}}{2}+1, f_{2k+3}-1} (the upper right corner of U_{k, 1} ).
Lemma 4.8. Let k\ge 0 and i\ge 1 . For all 0\leq r\leq f_{2k+1}-1 ,
(i) \textrm{(Left edge of $ V_{k, i} $)} H_{\beta'_i-f_{2k+1}+2+r, f_{2k+2}-1-r} = (-1)^{rk}(-1)^{\frac{r(r+1)}{2}}H_{\beta'_i-f_{2k+1}+2, f_{2k+2}-1} ,
(ii) \textrm{(Right edge of $ V_{k, i} $)} H_{\beta'_i+1+r, f_{2k+2}-1-r} = (-1)^{rk}(-1)^{\frac{r(r+1)}{2}}H_{\beta'_i+1, f_{2k+2}-1} ,
(iii) \textrm{(Upper edge of $ V_{k, i} $)} H_{\beta'_i-f_{2k+1}+2+r, f_{2k+2}-1} = H_{\beta'_i+1, f_{2k+2}-1} .
Proof. (ⅰ) Denote by A_{\beta'_i-f_{2k+1}+1+r+j} the j -th row of M_{\beta'_i-f_{2k+1}+2+r, f_{2k+2}-1-r} . Then
\begin{align*} H_{\beta'_i-f_{2k+1}+2+r, f_{2k+2}-1-r}& = \det \begin{pmatrix} A_{\beta'_i-f_{2k+1}+2+r}\\ A_{\beta'_i-f_{2k+1}+2+r+1}\\ \vdots\\ A_{\beta'_i+f_{2k}} \end{pmatrix} = \det \begin{pmatrix} A_{\beta'_i-f_{2k+1}+2+r}\\ A_{\beta'_i-f_{2k+1}+2+r+1}\\ \vdots\\ A_{\beta'_i+f_{2k}} - A_{\beta'_i} \end{pmatrix}. \end{align*} |
From Lemma 2.8, Theorem 2.5 and Lemma 2.11, we have
A_{\beta'_i+f_{2k}}-A_{\beta'_i} = ((-1)^k, \, 0 , \, \cdots , \, 0). |
For 0\leq r\leq f_{2k+1}-1 ,
\begin{align*} H_{\beta'_i-f_{2k+1}+2+r, f_{2k+2}-1-r}& = \begin{pmatrix} * & M_{\beta'_i-f_{2k+1}+2+(r+1), f_{2k+2}-1-(r+1)}\\ (-1)^k& {\bf{0}}_{1 , f_{2k+2}-2-r} \end{pmatrix}\\ & = (-1)^k(-1)^{1+f_{2k+2}-1-r}H_{\beta'_i-f_{2k+1}+2+(r+1), f_{2k+2}-1-(r+1)}\\ & = (-1)^{k+1+r}H_{\beta'_i-f_{2k+1}+2+(r+1), f_{2k+2}-1-(r+1)}. \;\;\;\;\;\;\;\;\;\;\;\;\text{(by Lemma 2.10(i))} \end{align*} |
Thus
\begin{align*} H_{\beta'_i-f_{2k+1}+2+r, f_{2k+2}-1-r}& = (-1)^{k+1+r-1}(-1)^{k+1+r-2}\cdots(-1)^{k+1}H_{\beta'_i+2-f_{2k+1}, f_{2k+2}-1}\\ & = (-1)^{r(k+1)}(-1)^{\frac{r(r-1)}{2}}H_{\beta'_i+2-f_{2k+1}, f_{2k+2}-1}. \end{align*} |
(ⅱ) Let B_{\beta'_i+r+j} be the j -th column of M_{\beta'_i+1+r, f_{2k+2}-1-r} .
\begin{align*} H_{\beta'_i+1+r, f_{2k+2}-1-r}& = \det\begin{pmatrix} s_{\beta'_i+1+r} & s_{\beta'_i+2+r} & \cdots & s_{\beta'_i+f_{2k+2}-1}\\ \vdots & \vdots & {\mathinner{\mkern2mu\raise1pt\hbox{.}\mkern2mu \raise4pt\hbox{.}\mkern2mu\raise7pt\hbox{.}\mkern1mu}} & \vdots\\ s_{\beta'_i+f_{2k+2}-1} & s_{\beta'_i+f_{2k+2}} & \cdots & s_{\beta'_i+2f_{2k+2}-3-r} \end{pmatrix}\\ & = \det\begin{pmatrix} B_{\beta'_i+1+r} & B_{\beta'_i+2+r} & \cdots & B_{\beta'_i+f_{2k+2}-1} \end{pmatrix}\\ & = \det\begin{pmatrix} B_{\beta'_i+1+r}-B_{\beta'_i+1+r+f_{2k}} & B_{\beta'_i+2+r} & \cdots & B_{\beta'_i+f_{2k+2}-1} \end{pmatrix}. \end{align*} |
Recall that \beta'_i\in F''_k . By Lemma 2.8, \beta'_i and \beta'_i+f_{2k+2} are adjacent elements in F_k . It follows from Theorem 2.5(ⅰ) and Lemma 2.11 that
\begin{align*} H_{\beta'_i+1+r, f_{2k+2}-1-r}& = \det\begin{pmatrix} {\bf{0}}_{f_{2k+2}-2-r , 1}& M_{\beta'_i+1+(r+1), f_{2k+2}-1-(r+1)}\\ (-1)^k & * \end{pmatrix}\\ & = (-1)^k(-1)^{1+f_{2k+2}-1-r}H_{\beta'_i+1+(r+1), f_{2k+2}-1-(r+1)}\\ & = (-1)^{k+1+r}H_{\beta'_i+1+(r+1), f_{2k+2}-1-(r+1)}.\;\;\;\;\;\;\;\;\;\;\;\;\;\;\text{(by Lemma 2.10(i))} \end{align*} |
Hence H_{\beta'_i+1+r, f_{2k+2}-1-r} = (-1)^{r(k+1)}(-1)^{\frac{r(r-1)}{2}}H_{\beta'_i+1, f_{2k+2}-1} .
(ⅲ) Let C_{\beta'_i-f_{2k+1}+1+r+j} be the j -th column of M_{\beta'_i-f_{2k+1}+2+r, f_{2k+2}-1} . Then
\begin{align*} H_{\beta'_i-f_{2k+1}+2+r, f_{2k+2}-1} & = \det \begin{pmatrix} C_{\beta'_i-f_{2k+1}+2+r} & C_{\beta'_i-f_{2k+1}+2+r+1} & \cdots & C_{\beta'_i+f_{2k}+r} \end{pmatrix}\\ & = \det \begin{pmatrix} C_{\beta'_i-f_{2k+1}+2+r} & \cdots & C_{\beta'_i+f_{2k}} & C'_{1}& \cdots & C'_{r} \end{pmatrix} \end{align*} |
where C'_{p} = C_{\beta'_i+f_{2k}+p}-C_{\beta'_i+p} for 1\leq p \leq r . By Lemma 2.8 and Theorem 2.5(ⅰ), we have s_{\beta'_i+\ell} = s_{\beta'_i+\ell+f_{2k}} for 1\leq \ell \leq f_{2k+2}-2 and f_{2k+2}+1\leq \ell \leq r+f_{2k+2}-1 . Moreover, by Proposition 2.4 and Lemma 2.11, we have s_{\beta'_i+f_{2k}+f_{2k+2}-1}-s_{\beta'_i+f_{2k+2}-1} = (-1)^{k+1} and s_{\beta'_i+f_{2k}+f_{2k+2}}-s_{\beta'_i+f_{2k+2}} = (-1)^{k} . Thus
\begin{align*} \begin{pmatrix} C'_{1}& \cdots & C'_{r} \end{pmatrix} & = \begin{pmatrix} {\bf{0}}_{f_{2k+2}-1-r, \, r}\\ X \end{pmatrix} \end{align*} |
where X is the r\times r matrix
\begin{pmatrix} 0 & \cdots & 0 & (-1)^{k+1}\\ \vdots & {\mathinner{\mkern2mu\raise1pt\hbox{.}\mkern2mu \raise4pt\hbox{.}\mkern2mu\raise7pt\hbox{.}\mkern1mu}} & {\mathinner{\mkern2mu\raise1pt\hbox{.}\mkern2mu \raise4pt\hbox{.}\mkern2mu\raise7pt\hbox{.}\mkern1mu}} & (-1)^k\\ \vdots & {\mathinner{\mkern2mu\raise1pt\hbox{.}\mkern2mu \raise4pt\hbox{.}\mkern2mu\raise7pt\hbox{.}\mkern1mu}} & {\mathinner{\mkern2mu\raise1pt\hbox{.}\mkern2mu \raise4pt\hbox{.}\mkern2mu\raise7pt\hbox{.}\mkern1mu}} & \vdots\\ (-1)^{k+1} & (-1)^k & \cdots & 0 \end{pmatrix}. |
Now expanding H_{\beta'_i-f_{2k+1}+2+r, f_{2k+2}-1} by its last r columns, we obtain that for 0\leq r \leq f_{2k+1}-1 ,
\begin{align*} H_{\beta'_i-f_{2k+1}+2+r, f_{2k+2}-1}& = \det \begin{pmatrix} M_{\beta'_i-f_{2k+1}+2+r, f_{2k+2}-1-r}& {\bf{0}}_{f_{2k+2}-1-r, \, r}\\ * & X \end{pmatrix} \\ & = (-1)^{(k+1)r}(-1)^{\frac{(r-1)r}{2}}H_{\beta'_i-f_{2k+1}+2+r, f_{2k+2}-1-r}\\ & = H_{\beta'_i-f_{2k+1}+2, f_{2k+2}-1}. \;\;\;\;\;\;\;\;\;\;\;\;\;\;\;\;\;\;\text{(by Lemma 4.8(i))} \end{align*} |
Remark 4.9. From Proposition 4.5(ⅰ) and Lemma 4.8, Hankel determinants on the boundary of V_{k, i} can be determined by H_{\frac{f_{2k+1}}{2}+f_{2k+3}+1, f_{2k}} (the lower left corner of V_{k, 1} ).
Lemma 4.10. Let k\ge 0 and i\ge 1 .
(i) \textrm{(Left edge of $ T_{k, i} $)} For all 0\le r\le f_{2k}-1 with \gamma_i-f_{2k+3}+2+r\ge 0 ,
H_{\gamma_i-f_{2k+3}+2+r, \, f_{2k+2}-1-r} = (-1)^{rk}(-1)^{\frac{r(r-1)}{2}}H_{\gamma_i-f_{2k+3}+2, \, f_{2k+2}-1}. |
(ii) \textrm{(Right edge of $ T_{k, i} $)} For all 0\le r\le f_{2k}-1 with \gamma_i-f_{2k+2}+1+r\ge 0 ,
H_{\gamma_i-f_{2k+2}+1+r, \, f_{2k+2}-1-r} = (-1)^{rk}(-1)^{\frac{r(r-1)}{2}}H_{\gamma_i-f_{2k+2}+1, \, f_{2k+2}-1}. |
(iii) \textrm{(Upper edge of $ T_{k, i} $)} For all 0\le r\le f_{2k}-1 with \gamma_i-f_{2k+3}+2+r\ge 0 ,
H_{\gamma_i-f_{2k+3}+2+r, \, f_{2k+2}-1} = H_{\gamma_i-f_{2k+3}+2, \, f_{2k+2}-1}. |
Proof. To shorten the notation, write x = \gamma_i-f_{2k+3}+2 and x' = \gamma_i-f_{2k+2}+1 .
(ⅰ) Let \max\{0, -x\}\leq \ell \leq f_{2k}-1 and let A_{j} be the j th row of H_{x+\ell, f_{2k+2}-1-\ell} . By Theorem 2.5(ⅱ) and Lemma 2.11, we see
A_{f_{2k+2}-1-\ell}-A_{f_{2k}-1-\ell} = \begin{pmatrix} (-1)^{k+1} & 0 & \dots & 0 \end{pmatrix}. |
Then for \max\{0, -x\}\leq \ell\leq f_{2k}-1 ,
\begin{align*} H_{x+\ell, f_{2k+2}-1-\ell} & = \det\begin{pmatrix} A_1\\ A_2\\ \vdots\\ A_{f_{2k+2}-2-\ell}\\ A_{f_{2k+2}-1-\ell} \end{pmatrix} = \det\begin{pmatrix} A_1\\ A_2\\ \vdots\\ A_{f_{2k+2}-2-\ell}\\ A_{f_{2k+2}-1-\ell}-A_{f_{2k}-1-\ell} \end{pmatrix}\\ & = \begin{vmatrix} * & M_{x+(\ell+1), f_{2k+2}-1-(\ell+1)}\\ (-1)^{k+1}& {\bf{0}}_{1, f_{2k+2}-2-\ell} \end{vmatrix}\\ & = (-1)^{k+1}(-1)^{1+f_{2k+2}-1-\ell}H_{x+(\ell+1), f_{2k+2}-1-(\ell+1)}\\ & = (-1)^{k+\ell}H_{x+(\ell+1), f_{2k+2}-1-(\ell+1)}. \;\;\;\;\;\;\;\;\;\;\;\;\;\;\;\;\;\;\;\;\;\;\text{(by Lemma 2.10(i))} \end{align*} |
Applying the above equality r times, one has
\begin{align*} H_{x+r, f_{2k+2}-1-r}& = (-1)^{k+r-1}(-1)^{k+r-2}\cdots(-1)^{k}H_{x, f_{2k+2}-1}\\ & = (-1)^{rk}(-1)^{\frac{r(r-1)}{2}}H_{x, f_{2k+2}-1}. \end{align*} |
(ⅱ) Let \max\{0, -x'\}\leq \ell \leq f_{2k}-1 and let B_j be the j th column of H_{x'+\ell, f_{2k+2}-1-\ell} . By Theorem 2.5(ⅱ) and Lemma 2.11, we see
B_1-B_{1+f_{2k+1}} = \begin{pmatrix} 0\\ \vdots\\ 0 \\ (-1)^{k+1} \end{pmatrix}. |
Therefore, for \max\{0, -x'\}\leq \ell \leq f_{2k}-1 ,
\begin{align*} H_{x'+\ell, f_{2k+2}-1-\ell} & = \det\begin{pmatrix} B_1 & B_2 & \cdots & B_{f_{2k+2}-1-\ell} \end{pmatrix}\\ & = \det\begin{pmatrix} B_1-B_{1+f_{2k+1}} & B_2 & \cdots & B_{f_{2k+2}-1-\ell} \end{pmatrix}\\ & = \det \begin{pmatrix} {\bf{0}}_{ f_{2k+2}-2-\ell, 1} & M_{x'+(\ell+1), f_{2k+2}-1-(\ell+1)}\\ (-1)^{k+1}& *\\ \end{pmatrix}\\ & = (-1)^{k+1}(-1)^{1+f_{2k+2}-1-\ell}H_{x'+(\ell+1), f_{2k+2}-1-(\ell+1)}\\ & = (-1)^{k+\ell}H_{x'+(\ell+1), f_{2k+2}-1-(\ell+1)}. \;\;\;\;\;\;\;\;\;\;\;\;\;\;\;\;\;\;\;\;\; \;\text{(by Lemma 2.10(i))} \end{align*} |
Applying the above equality r times, one has
\begin{align*} H_{x'+r, f_{2k+2}-1-r}& = (-1)^{k+r-1}(-1)^{k+r-2}\cdots(-1)^{k}H_{x', f_{2k+2}-1}\\ & = (-1)^{rk}(-1)^{\frac{r(r-1)}{2}}H_{x', f_{2k+2}-1}. \end{align*} |
(ⅲ) Let \max\{0, -x\}\leq r \leq f_{2k}-1 and let C_j be the j th column of M_{x+r, f_{2k+2}-1} . Then
\begin{align*} H_{x+r, f_{2k+2}-1} & = \det\begin{pmatrix} C_1 & C_2 & \cdots & C_{f_{2k+2}-1} \end{pmatrix}\\ & = \det\begin{pmatrix} C_1 & C_2 & \cdots & C_{f_{2k+2}-r-1} & C'_1 & \cdots & C'_r \end{pmatrix} \end{align*} |
where C'_p = C_{f_{2k+2}-r-1+p}-C_{f_{2k}-r-1+p} for 1\le p \le r . Note that
\begin{pmatrix} C'_1 & \cdots & C'_r \end{pmatrix} = \begin{pmatrix} s_{\gamma_i-f_{2k}+1} & \cdots & s_{\gamma_i-f_{2k}+r}\\ \vdots & \ddots & \vdots\\ s_{\gamma_i+f_{2k+1}-1} & \cdots & s_{\gamma_i+f_{2k+1}+r-2} \end{pmatrix}- \begin{pmatrix} s_{\gamma_i-f_{2k+2}+1} & \cdots & s_{\gamma_i-f_{2k+2}+r}\\ \vdots & \ddots & \vdots\\ s_{\gamma_i-1} & \cdots & s_{\gamma_i+r-2} \end{pmatrix}. |
By Lemma 2.6 and Theorem 2.5(ⅱ), for 1\leq q\leq f_{2k+2}-2 and f_{2k+2}+1\leq q \leq f_{2k+2}+r-2 ,
s_{\gamma_i-f_{2k}+q} = s_{\gamma_i-f_{2k+2}+q}. |
Moreover, by Lemma 2.11, s_{\gamma_i+f_{2k+1}-1}-s_{\gamma_i-1} = (-1)^{k} and s_{\gamma_i+f_{2k+1}}-s_{\gamma_i} = (-1)^{k+1} . Then
\begin{pmatrix} C'_1 & \cdots & C'_r \end{pmatrix} = \begin{pmatrix} {\bf{0}}_{f_{2k+2}-1-r, \, r}\\ X \end{pmatrix} |
where X is the r\times r matrix
\begin{pmatrix} 0 & \cdots & 0 & (-1)^{k}\\ \vdots & {\mathinner{\mkern2mu\raise1pt\hbox{.}\mkern2mu \raise4pt\hbox{.}\mkern2mu\raise7pt\hbox{.}\mkern1mu}} & {\mathinner{\mkern2mu\raise1pt\hbox{.}\mkern2mu \raise4pt\hbox{.}\mkern2mu\raise7pt\hbox{.}\mkern1mu}} & (-1)^{k+1}\\ \vdots & {\mathinner{\mkern2mu\raise1pt\hbox{.}\mkern2mu \raise4pt\hbox{.}\mkern2mu\raise7pt\hbox{.}\mkern1mu}} & {\mathinner{\mkern2mu\raise1pt\hbox{.}\mkern2mu \raise4pt\hbox{.}\mkern2mu\raise7pt\hbox{.}\mkern1mu}} & \vdots\\ (-1)^{k} & (-1)^{k+1} & \cdots & 0 \end{pmatrix}. |
Therefore,
\begin{align*} H_{x+r, f_{2k+2}-1} & = \det \begin{pmatrix} M_{x+r, f_{2k+2}-1-r}& {\bf{0}}_{f_{2k+2}-1-r, \, r}\\ * & X \end{pmatrix}\\ & = (-1)^{kr}(-1)^{\frac{(r-1)r}{2}}H_{x+r, f_{2k+2}-1-r}\\ & = H_{x, f_{2k+2}-1}. \;\;\;\;\;\;\;\;\;\;\;\;\;\;\;\;\;\;\;\;\;\;\;\;\;\;\;\;\;\;\;\;\;\;\;\;\;\;\text{(by Lemma 4.10(i))} \end{align*} |
Remark 4.11. From Proposition 4.5(ⅱ) and Lemma 4.10, Hankel determinants on the boundary of T_{k, i} can be determined by H_{\frac{f_{2k+3}}{2}+f_{2k}+1, f_{2k+1}} (the lower left corner of T_{k, 2} ).
In section 4, we show that for any k\ge 0 , to know all the determinants on the boundary of U_{k, i} (resp. V_{k, i} , T_{k, i} ) for all i , it is enough to know the value of one determinant on the boundary U_{k, i} (resp. V_{k, i} or T_{k, i} ) for some i . In this section, for certain i , we shall give the expression of a determinant on the boundary U_{k, i} (resp. V_{k, i} or T_{k, i} ) for all k .
The next result allows us to determine the determinant on the lower left corner of U_{k, i} by using the determinants on the boundary of U_{k-1, *} and T_{k-1, *} .
Lemma 5.1. \textrm{(Lower left corner of $ U_{k, i} $)} For all k\ge 1 and i\ge 1 ,
H_{\alpha_i-f_{2k+3}+1, \, f_{2k}} = (-1)^{k}(H_{\alpha_i-f_{2k+3}+2, \, f_{2k}-1}-H_{\alpha_i-f_{2k+3}+1, \, f_{2k}-1}). |
Proof. Let y = \alpha_i-f_{2k+3} and let A_j be the j th column of H_{y+1, f_{2k}} . Then
\begin{align*} H_{y+1, f_{2k}} & = \begin{vmatrix} s_{y+1} & s_{y+2} & \cdots & s_{y+f_{2k}}\\ \vdots & \vdots & {\mathinner{\mkern2mu\raise1pt\hbox{.}\mkern2mu \raise4pt\hbox{.}\mkern2mu\raise7pt\hbox{.}\mkern1mu}} & \vdots\\ s_{y+f_{2k}} & s_{y+f_{2k}+1} & \cdots & s_{y+2f_{2k}-1} \end{vmatrix} = \det \begin{pmatrix} A_1 & A_2 & \cdots & A_{f_{2k}-1} \end{pmatrix}. \end{align*} |
Recall that \alpha_i\in E'_{k+1} . Then \Phi_{k+1}(y) = \frac{f_{2k+1}}{2} and \Phi_{k-1}(y+f_{2k}) = \frac{f_{2k-1}}{2} . This implies y\in F'_k and y+f_{2k}\in F_{k-1} . By Lemma 2.8 and Theorem 2.5(ⅰ), the fact y+f_{2k}\in F_{k-1} yields that s_{y+\ell} = s_{y+f_{2k-2}+\ell} for 1\leq \ell \leq f_{2k}-2 . By Lemma 2.11, s_{y+f_{2k}-1}-s_{y+f_{2k}+f_{2k-2}-1} = (-1)^{k+1} and s_{y+f_{2k}}-s_{y+f_{2k}+f_{2k-2}} = (-1)^{k} . So
\begin{align} H_{y+1, f_{2k}} & = \det \begin{pmatrix} A_1-A_{1+f_{2k-2}} & A_2 & \cdots & A_{f_{2k}-1} \end{pmatrix}\\ & = \begin{vmatrix} 0 & s_{y+2} & \cdots & s_{y+f_{2k}}\\ \vdots & \vdots & {\mathinner{\mkern2mu\raise1pt\hbox{.}\mkern2mu \raise4pt\hbox{.}\mkern2mu\raise7pt\hbox{.}\mkern1mu}} & \vdots\\ 0 & s_{y+f_{2k}-1} & \cdots & s_{y+2f_{2k}-3}\\ (-1)^{k+1} & s_{y+f_{2k}} & \cdots & s_{y+2f_{2k}-2}\\ (-1)^{k} & s_{y+f_{2k}+1} & \cdots & s_{y+2f_{2k}-1} \end{vmatrix}\\ & = (-1)^{k}(-1)^{1+f_{2k}} H_{y+2, f_{2k}-1} + (-1)^{k+1}(-1)^{f_{2k}}X \end{align} | (5.1) |
where
X = \begin{vmatrix} s_{y+2} & \cdots & s_{y+f_{2k}}\\ \vdots & {\mathinner{\mkern2mu\raise1pt\hbox{.}\mkern2mu \raise4pt\hbox{.}\mkern2mu\raise7pt\hbox{.}\mkern1mu}} & \vdots\\ s_{y+f_{2k}-1} & \cdots & s_{y+2f_{2k}-3}\\ s_{y+f_{2k}+1} & \cdots & s_{y+2f_{2k}-1} \end{vmatrix} = (-1)^{f_{2k}-2}\begin{vmatrix} s_{y+f_{2k}+1} & \cdots & s_{y+2f_{2k}-1}\\ s_{y+2} & \cdots & s_{y+f_{2k}}\\ \vdots & {\mathinner{\mkern2mu\raise1pt\hbox{.}\mkern2mu \raise4pt\hbox{.}\mkern2mu\raise7pt\hbox{.}\mkern1mu}} & \vdots\\ s_{y+f_{2k}-1} & \cdots & s_{y+2f_{2k}-3} \end{vmatrix}. |
Since y+f_{2k}\in F'_{k} , by Lemma 2.8 and Theorem 2.5(ⅰ), we see
\begin{pmatrix} s_{y+f_{2k}+1} & \cdots & s_{y+2f_{2k}-1} \end{pmatrix} = \begin{pmatrix} s_{y+1} & \cdots & s_{y+f_{2k}-1} \end{pmatrix} |
and X = (-1)^{f_{2k}-2}H_{y+1, f_{2k}-1} . Then the result follows from Eq (5.1) and Lemma 2.10.
Now we show how to obtain the determinant on the lower left corner of T_{k, i} by using determinants on the boundary of U_{k, i-1} and U_{k-1, i+1} .
Lemma 5.2. \textrm{(Lower left corner of $ T_{k, i}) $} For all k\ge 1 and i\ge 2 ,
H_{\gamma_i-f_{2k+2}+1, \, f_{2k+1}} = (-1)^{k}(H_{\gamma_i-f_{2k+2}+2, \, f_{2k+1}-1}+H_{\gamma_i-f_{2k+2}+1, \, f_{2k+1}-1}). |
Proof. Let y = \gamma_i-f_{2k+2} and let A_j be the j th column of H_{y+1, f_{2k+1}} . Then
\begin{align*} H_{y+1, f_{2k+1}} & = \begin{vmatrix} s_{y+1} & s_{y+2} & \cdots & s_{y+f_{2k+1}}\\ \vdots & \vdots & {\mathinner{\mkern2mu\raise1pt\hbox{.}\mkern2mu \raise4pt\hbox{.}\mkern2mu\raise7pt\hbox{.}\mkern1mu}} & \vdots\\ s_{y+f_{2k+1}} & s_{y+f_{2k+1}+1} & \cdots & s_{y+2f_{2k+1}-1} \end{vmatrix} = \det \begin{pmatrix} A_1 & A_2 & \cdots & A_{f_{2k+1}-1} \end{pmatrix}. \end{align*} |
Recall that \gamma_i\in E'_{k} . By Lemma 2.6, y\in E_k and \Phi_{k}(y+f_{2k+1}) = \frac{f_{2k+1}}{2} . This implies y+f_{2k+1}\in F_{k} . By Lemma 2.8 and Theorem 2.5(ⅰ), the fact y+f_{2k+1}\in F_{k} yields that s_{y+\ell} = s_{y+f_{2k}+\ell} for 1\leq \ell \leq f_{2k+1}-2 . By Lemma 2.11, s_{y+f_{2k+1}-1}-s_{y+f_{2k+1}+f_{2k}-1} = (-1)^{k} and s_{y+f_{2k+1}}-s_{y+f_{2k+1}+f_{2k}} = (-1)^{k+1} . So
\begin{align} H_{y+1, f_{2k+1}} & = \det \begin{pmatrix} A_1-A_{1+f_{2k}} & A_2 & \cdots & A_{f_{2k+1}-1} \end{pmatrix}\\ & = \begin{vmatrix} 0 & s_{y+2} & \cdots & s_{y+f_{2k+1}}\\ \vdots & \vdots & {\mathinner{\mkern2mu\raise1pt\hbox{.}\mkern2mu \raise4pt\hbox{.}\mkern2mu\raise7pt\hbox{.}\mkern1mu}} & \vdots\\ 0 & s_{y+f_{2k+1}-1} & \cdots & s_{y+2f_{2k+1}-3}\\ (-1)^{k} & s_{y+f_{2k+1}} & \cdots & s_{y+2f_{2k+1}-2}\\ (-1)^{k+1} & s_{y+f_{2k+1}+1} & \cdots & s_{y+2f_{2k+1}-1} \end{vmatrix}\\ & = (-1)^{k+1}(-1)^{1+f_{2k+1}} H_{y+2, f_{2k+1}-1} + (-1)^{k}(-1)^{f_{2k+1}}X \end{align} | (5.2) |
where
X = \begin{vmatrix} s_{y+2} & \cdots & s_{y+f_{2k+1}}\\ \vdots & {\mathinner{\mkern2mu\raise1pt\hbox{.}\mkern2mu \raise4pt\hbox{.}\mkern2mu\raise7pt\hbox{.}\mkern1mu}} & \vdots\\ s_{y+f_{2k+1}-1} & \cdots & s_{y+2f_{2k+1}-3}\\ s_{y+f_{2k+1}+1} & \cdots & s_{y+2f_{2k+1}-1} \end{vmatrix} = (-1)^{f_{2k+1}-2}\begin{vmatrix} s_{y+f_{2k+1}+1} & \cdots & s_{y+2f_{2k+1}-1}\\ s_{y+2} & \cdots & s_{y+f_{2k+1}}\\ \vdots & {\mathinner{\mkern2mu\raise1pt\hbox{.}\mkern2mu \raise4pt\hbox{.}\mkern2mu\raise7pt\hbox{.}\mkern1mu}} & \vdots\\ s_{y+f_{2k+1}-1} & \cdots & s_{y+2f_{2k+1}-3} \end{vmatrix}. |
Since y\in E_{k} , by Lemma 2.6 and Theorem 2.5(ⅱ), we see
\begin{pmatrix} s_{y+f_{2k+1}+1} & \cdots & s_{y+2f_{2k+1}-1} \end{pmatrix} = \begin{pmatrix} s_{y+1} & \cdots & s_{y+f_{2k+1}-1} \end{pmatrix} |
and X = (-1)^{f_{2k+1}-2}H_{y+1, f_{2k+1}-1} . Then the result follows from Eq (5.2) and Lemma 2.10.
Now We are able to give the exact value of the Hanker determinant on the upper right corner of U_{k, i} , and hence we know all the determinants on the boundary of U_{k, i} .
Theorem 5.3. \textrm{(Upper right corner of $ U_{k, 1} $)} Let k\ge 1 . Then
H_{\frac{f_{2k+1}}{2}+1, \, f_{2k+3}-1} = (-1)^{k+1}\frac{f_{2k+1}}{2}. |
Proof. We can check directly that the result holds for k = 1, 2 . Now suppose k\ge 3 . Let h_{k} = H_{\frac{f_{2k+1}}{2}+1, \, f_{2k+3}-1} . Then
\begin{align*} h_{k} & = (-1)^{(f_{2k+2}-1)k}(-1)^{\frac{(f_{2k+2}-1)(f_{2k+2}-2)}{2}} H_{\frac{f_{2k+5}}{2}-f_{2k}, f_{2k}} \;\;\;\;\;\;\;\;\;\;\;\;\;\;\;\;\;\;\;\;\;\text{(by Lemma 4.6(i))}\\ & = (-1)^{\frac{f_{2k+2}-1}{2}} H_{\frac{f_{2k+1}}{2}+1, f_{2k}} \;\;\;\;\;\;\;\;\;\;\;\;\;\;\;\;\;\;\;\;\;\;\;\;\;\;\;\text{(by Lemma 2.10 and Lemma 4.2(ii))}\\ & = (-1)^{\frac{f_{2k+2}-1}{2}}(-1)^{k}(H_{\frac{f_{2k+1}}{2}+2, \, f_{2k}-1}-H_{\frac{f_{2k+1}}{2}+1, \, f_{2k}-1})\;\;\;\;\;\;\;\;\;\;\;\;\;\;\;\;\text{(by Lemma 5.1)}. \end{align*} |
Applying Lemma 4.6(ⅰ) for k-1 * and r = f_{2k-2} ,
*We need to mention that \alpha_i 's depend also on k . For example, \alpha_1^{(k-1)} = \frac{f_{2k+3}}{2} and \alpha_1^{(k)} = \frac{f_{2k+5}}{2} .
\begin{align*} H_{\frac{f_{2k+1}}{2}+1, \, f_{2k}-1} & = (-1)^{f_{2k-2}(k-1)}(-1)^{\frac{f_{2k-2}(f_{2k-2}-1)}{2}}H_{\frac{f_{2k-1}}{2}+1, \, f_{2k+1}-1}\\ & = (-1)^{k-1}(-1)^\frac{f_{2k-2}-1}{2}h_{k-1}.\;\;\;\;\;\;\;\;\;\;\;\;\;\;\;\;\text{(by Lemma 2.10)} \end{align*} |
Applying Lemma 4.10(ⅰ) for k-1 and r = f_{2k-2} ,
\begin{align*} H_{\frac{f_{2k+1}}{2}+2, \, f_{2k}-1} & = (-1)^{(f_{2k-2}-1)(k-1)}(-1)^{\frac{(f_{2k-2}-1)(f_{2k-2}-2)}{2}}H_{\frac{f_{2k+1}}{2}+f_{2k-2}+1, \, f_{2k-1}}\\ & = (-1)^{\frac{f_{2k-2}-1}{2}}H_{\frac{f_{2k+1}}{2}+f_{2k-2}+1, \, f_{2k-1}}\;\;\;\;\;\;\;\;\;\;\;\;\;\;\;\;\;\;\;\;\;\;\;\;\;\;\;\;\;\;\;\;\;\;\;\;\;\;\;\;\;\;\;\;\;\;\;\;\;\;\;\;\;\;\;\;\;\;\;\;\;\;\;\; \;\text{(by Lemma 2.10)}\\ & = (-1)^{\frac{f_{2k-2}-1}{2}}(-1)^{k-1}\left(H_{\frac{f_{2k+1}}{2}+f_{2k-2}+2, \, f_{2k-1}-1}+H_{\frac{f_{2k+1}}{2}+f_{2k-2}+1, \, f_{2k-1}-1}\right)\;\;\;\;\;\;\;\;\;\;\;\;\text{(by Lemma 5.2)}\\ & = (-1)^{\frac{f_{2k-2}-1}{2}}(-1)^{k-1}\left(h_{k-2}+H_{\frac{f_{2k+1}}{2}+f_{2k-2}+1, \, f_{2k-1}-1}\right) \;\;\;\;\;\;\;\;\;\;\;\;\;\;\;\;\;\;\;\;\;\;\;\;\;\;\;\;\;\text{(by Lemma 4.6(iii))}\\ & = (-1)^{\frac{f_{2k-2}-1}{2}}(-1)^{k-1}\left(h_{k-2}-h_{k-1}\right). \;\;\;\;\;\;\;\;\;\;\;\;\;\;\;\;\;\;\;\;\;\;\;\;\;\;\;\;\;\;\;\;\text{(by Lemma 4.6(i) and Lemma 2.10)} \end{align*} |
Combing previous equations, we have
\begin{align*} h_{k} & = (-1)^{\frac{f_{2k+2}-1}{2}}(-1)^{k}(H_{\alpha_1-f_{2k+3}+2, \, f_{2k}-1}-H_{\alpha_1-f_{2k+3}+1, \, f_{2k}-1})\\ & = (-1)^{\frac{f_{2k+2}-1}{2}}(-1)^{k}(-1)^{\frac{f_{2k-2}-1}{2}}(-1)^{k-1}(h_{k-2}-2h_{k-1})\\ & = h_{k-2}-2h_{k-1}. \;\;\;\;\;\;\;\;\;\;\;\;\;\;\;\;\;\;\;\;\;\;\;\;\;\;\;\;\;\;\;\;\;\;\;\;\;\;\;\;\;\;\;\;\;\;\;\;\text{(by Lemma 2.10)} \end{align*} |
The initial values are h_1 = 2 , h_2 = -5 . The result follows from the recurrence relation of h_k and its initial values.
Corollary 5.4. \textrm{(Lower left corner of $ V_{k, 1} $)} For all k\ge 1 ,
H_{\frac{f_{2k+1}}{2}+f_{2k+3}+1, \, f_{2k}} = (-1)^{k+\frac{f_{2k+2}+1}{2}}\frac{f_{2k+1}}{2}. |
Proof. By Proposition 4.5,
\begin{align*} H_{\frac{f_{2k+1}}{2}+f_{2k+3}+1, \, f_{2k}} & = H_{\frac{f_{2k+5}}{2}-f_{2k}, \, f_{2k}}\\ & = (-1)^{(f_{2k+2}-1)k}(-1)^{\frac{(f_{2k+2}-1)(f_{2k+2}-2)}{2}}H_{\frac{f_{2k+1}}{2}+1, f_{2k+3}-1}\;\;\;\;\;\;\;\;\;\;\text{(by Lemma 4.6(i))}\\ & = (-1)^{\frac{f_{2k+2}-1}{2}}H_{\frac{f_{2k+1}}{2}+1, f_{2k+3}-1}\;\;\;\;\;\;\;\;\;\;\;\;\;\;\;\;\;\;\;\;\;\;\;\;\;\;\;\;\;\;\;\;\;\;\;\; \text{(by Lemma 2.10)}\\ & = (-1)^{k+\frac{f_{2k+2}+1}{2}}\frac{f_{2k+1}}{2}. \;\;\;\;\;\;\;\;\;\;\;\;\;\;\;\;\;\;\;\;\;\;\;\;\;\;\;\;\;\;\;\;\;\;\;\;\;\;\;\;\;\;\;\;\;\;\text{(by Theorem 5.3)} \end{align*} |
Corollary 5.5. \textrm{(Lower left corner of $ T_{k, 2} $)} For all k\ge 1 , H_{\frac{f_{2k+3}}{2}+f_{2k}+1, f_{2k+1}} = f_{2k} .
Proof. From Lemma 5.2, we have
\begin{align} H_{\frac{f_{2k+3}}{2}+f_{2k}+1, f_{2k+1}}& = (-1)^k(H_{\frac{f_{2k+3}}{2}+f_{2k}+2, f_{2k+1}-1}+H_{\frac{f_{2k+3}}{2}+f_{2k}+1, f_{2k+1}-1}). \end{align} | (5.3) |
Note that H_{\frac{f_{2k+3}}{2}+f_{2k}+2, f_{2k+1}-1} is on the upper left corner of U_{k-1, 2} . By Lemma 4.6(ⅲ),
H_{\frac{f_{2k+3}}{2}+f_{2k}+2, f_{2k+1}-1} = H_{\frac{f_{2k-1}}{2}+f_{2k+3}+1, f_{2k+1}-1}. |
According to Proposition 4.5 and Lemma 4.6, the determinants on the upper left corner of U_{k-1, 1} and U_{k-1, 1} are equal. Namely, H_{\frac{f_{2k-1}}{2}+f_{2k+3}+1, f_{2k+1}-1} = H_{\frac{f_{2k-1}}{2}+1, f_{2k+1}-1} . Therefore,
\begin{equation} H_{\frac{f_{2k+3}}{2}+f_{2k}+2, f_{2k+1}-1} = H_{\frac{f_{2k-1}}{2}+1, f_{2k+1}-1}. \end{equation} | (5.4) |
It follows from Lemma 4.6(ⅰ) and Lemma 2.10 that
\begin{align} H_{\frac{f_{2k+3}}{2}+f_{2k}+1, , \, f_{2k+1}-1} & = (-1)^{2f_{2k}k}(-1)^{\frac{2f_{2k}(2f_{2k}-1)}{2}}H_{\frac{f_{2k+1}}{2}+1, \, f_{2k+3}-1}\\ & = - H_{\frac{f_{2k+1}}{2}+1, \, f_{2k+3}-1}. \end{align} | (5.5) |
Using Eq (5.3), Eq (5.4) and Eq (5.5), we have
\begin{align*} H_{\frac{f_{2k+3}}{2}+f_{2k}+1, f_{2k+1}} & = (-1)^{k}\left(H_{\frac{f_{2k-1}}{2}+1, f_{2k+1}-1}-H_{\frac{f_{2k+1}}{2}+1, \, f_{2k+3}-1}\right)\\ & = (-1)^k\left((-1)^k\frac{f_{2k-1}}{2}-(-1)^{k+1}\frac{f_{2k+1}}{2}\right)\;\;\;\;\;\;\;\;\;\;\text{(by Theorem 5.3)}\\ & = f_{2k}. \end{align*} |
Proof of Theorem 1.1. Suppose (m, n)\in U_{k, i} for some i . Then \alpha_{i}-f_{2k+2} < n+m\leq \alpha_i and m = \alpha_i-f_{2k+2}+1-n+r where 0\leq r < f_{2k+2} .
Case 1: n = f_{2k+3}-1 . Applying Lemma 4.6(ⅰ), Proposition 4.5 and then Lemma 4.6(ⅰ) again, we have
\begin{equation} H_{\alpha_i-f_{2k+3}+1, f_{2k+3}-1} = H_{\alpha_1-f_{2k+3}+1, f_{2k+3}-1} = (-1)^{k+1}\frac{f_{2k+1}}{2}. \end{equation} | (6.1) |
where the last equality follows from Theorem 5.3. Since r = \alpha_i-m-n = \frac{f_{2k+5}}{2}-\Phi_{k+1}(m+n) , by Lemma 4.6(ⅲ),
\begin{align*} H_{m, n}& = H_{\alpha_i-f_{2k+4}+2+r, f_{2k+3}-1}\\ & = (-1)^r H_{\alpha_i-f_{2k+3}+1, f_{2k+3}-1}\\ & = (-1)^{\frac{f_{2k+5}}{2}-\Phi_{k+1}(m+n)}H_{\alpha_i-f_{2k+3}+1, f_{2k+3}-1}\\ & = (-1)^{k+1}(-1)^{\frac{f_{2k+5}}{2}-\Phi_{k+1}(m+n)}\frac{f_{2k+1}}{2} \end{align*} |
where the last equality follows from Eq (6.1).
Case 2: n = f_{2k} . By Proposition 4.5, we have
\begin{align*} H_{m, n}& = H_{\alpha_1-f_{2k}, \, f_{2k}} \\ & = (-1)^{\ell k}(-1)^{\frac{\ell(\ell-1)}{2}}H_{\alpha_1-f_{2k+3}+1, f_{2k+3}-1} \;\;\;\;\;\;\;\;\;\;\;\;\text{(by Lemma 4.6(iii))}\\ & = (-1)^{k+1}(-1)^{\frac{f_{2k+2}-1}{2}}\cdot \frac{f_{2k+1}}{2} \;\;\;\;\;\;\;\;\;\;\;\;\;\;\;\;\;\;\;\;\;\;\;\;\text{(by Theorem 5.3)} \end{align*} |
where \ell = f_{2k+3}-1-f_{2k} is even by Lemma 2.10.
Case 3: f_{2k} < n < f_{2k+3}-1 . If m+n = \alpha_i (or \alpha_i-f_{2k+2}+1 ), then applying Lemma 4.6(ⅰ) (or Lemma 4.6(ⅱ)) and then Eq (6.1), we have
\begin{align*} H_{m, n} & = (-1)^{\ell k}(-1)^{\frac{\ell(\ell-1)}{2}}H_{\alpha_i-f_{2k+3}+1, f_{2k+3}-1}\\ & = (-1)^{\ell k}(-1)^{\frac{\ell(\ell-1)}{2}}(-1)^{k+1}\frac{f_{2k+1}}{2} \end{align*} |
where \ell = f_{2k+3}-1-n . If \alpha_i-f_{2k+2}+1 < m+n < \alpha_i , then Lemma 4.1 yields H_{m, n} = 0 .
Proof of Theorem 1.2. Suppose (m, n)\in V_{k, i} for some i . Then \beta_i < n+m\leq \beta_i+f_{2k+1} .
Case 1: n = f_{2k+2}-1 . By Lemma 4.8(ⅲ) & (ⅰ), we have
\begin{align} H_{m, n} & = H_{\beta'_i-f_{2k+1}+2, \, f_{2k+2}-1} \\ & = (-1)^{(f_{2k+1}-1)k}(-1)^{\frac{(f_{2k+1}-1)f_{2k+1}}{2}}H_{\beta'_i+1, \, f_{2k}}. \end{align} | (6.2) |
According to Proposition 4.5(ⅰ) and Corollary 5.4,
\begin{equation} H_{\beta'_i+1, \, f_{2k}} = H_{\beta'_1+1, \, f_{2k}} = (-1)^{k+\frac{f_{2k+2}+1}{2}}\frac{f_{2k+1}}{2}. \end{equation} | (6.3) |
The result follows from Eq (6.2) and Eq (6.3).
Case 2: n = f_{2k} . By Proposition 4.5, H_{m, n} = H_{\alpha_1-f_{2k}, \, f_{2k}} . Then the result follows from Theorem 1.1(ⅱ).
Case 3: f_{2k} < n < f_{2k+2}-1 . If m+n = \beta_i+1 (or \beta_i+f_{2k+1} ), then by Lemma 4.8(ⅰ) (or Lemma 4.8(ⅱ)), we have
H_{m, n} = -(-1)^{(f_{2k+2}-n)(k+1)}(-1)^{\frac{(f_{2k+2}-1-n)(f_{2k+2}-2-n)}{2}} \frac{f_{2k+1}}{2}. |
If \beta_i+1 < m+n < \beta_i+f_{2k+1} , then Lemma 4.1 shows H_{m, n} = 0 .
Proof of Theorem 1.3. Suppose (m, n)\in T_{k, i} for some i . Then \gamma_i-f_{2k} < n+m\leq \gamma_i .
Case 1: n = f_{2k+2}-1 . By Lemma 4.10(ⅲ) & (ⅰ),
\begin{align} H_{m, n}& = H_{\gamma_i-f_{2k+3}+2, \, f_{2k+2}-1} \\ & = (-1)^{(f_{2k}-1)k}(-1)^{\frac{(f_{2k}-1)(f_{2k}-2)}{2}}H_{\gamma_i-f_{2k+2}+1, \, f_{2k+1}}\\ & = (-1)^{\frac{f_{2k}-1}{2}}H_{\gamma_i-f_{2k+2}+1, \, f_{2k+1}}. \end{align} | (6.4) |
where the last equality follows from Lemma 2.10(ⅰ). Using Proposition 4.5(ⅱ) and Corollary 5.5,
\begin{equation} H_{\gamma_i-f_{2k+2}+1, \, f_{2k+1}} = H_{\gamma_2-f_{2k+2}+1, \, f_{2k+1}} = f_{2k}. \end{equation} | (6.5) |
The result follows from Eq (6.4) and Eq (6.5).
Case 2: n = f_{2k+1} . Write m = \gamma_i-f_{2k+2}+r with 1\leq r\leq f_{2k} . By Proposition 4.5(ⅱ) and Corollary 5.5,
\begin{align*} H_{m, n} & = (-1)^{r+1}H_{\gamma_{2}-f_{2k+2}+1, \, f_{2k+1}} = (-1)^{r+1}f_{2k}. \end{align*} |
Note that \gamma_i = m+n+f_{2k}-r and 1\leq r\leq f_{2k} . Consequently,
\frac{f_{2k+3}}{2} = \Phi_k(\gamma_i) = \Phi_{k}(m+n+f_{2k}-r) = \Phi_{k}(m+n)+f_{2k}-r |
which gives r = \Phi_{k}(m+n)-\frac{f_{2k+1}}{2} . Then the result follows.
Case 3: f_{2k} < n < f_{2k+2}-1 . If m+n = \gamma_i-f_{2k}+1 (or \gamma_i ), then by Lemma 4.10(ⅰ) (or Lemma 4.10(ⅱ)),
H_{m, n} = (-1)^{(f_{2k+2}-1-n)k}(-1)^{\frac{(f_{2k+2}-1-n)(f_{2k+2}-2-n)}{2}}(-1)^{\frac{f_{2k}-1}{2}}\cdot f_{2k}. |
If \gamma_i-f_{2k}+1 < m+n < \gamma_i , then Lemma 4.1 yields H_{m, n} = 0 .
In this paper, we study the Hankel determinants H_{m, n} of the Sturmian sequence {\bf{s}} = \tau^{\infty}(1) . In Theorem 1.1, 1.2 and 1.3, we give the closed form of the Hankel determinants H_{m, n} for all m\ge 0 and n\ge 1 . To extend the results to other Sturmian sequences, the difficulty is to locate the parallelograms that are composed by (m, n) 's such that H_{m, n} = 0 . This will need further effort.
We thank the referees for their valuable comments and suggestions. This work was supported by Guangzhou Science and Technology program (202102020294), Guangdong Basic and Applied Basic Research Foundation (2021A1515010056) and the Fundamental Research Funds for the Central Universities from SCUT (2020ZYGXZR041).
The authors declare that they have no conflict of interest.
[1] | J. P. Allouche, J. Peyrière, Z. X. Wen, Z. Y. Wen, Hankel determinants of the Thue-Morse sequence, Ann. Inst. Fourier, Grenoble, 48 (1998), 1–27. |
[2] | J. P. Allouche, J. Shallit, Automatic sequences: Theory and Applications, Cambridge University Press, 2003. |
[3] |
Y. Bugeaud, On the rational approximation to the Thue-Morse-Mahler numbers, Ann. Inst. Fourier, 61 (2011), 2065–2076. https://doi.org/10.5802/aif.2666 doi: 10.5802/aif.2666
![]() |
[4] | Y. Bugeaud, G. N. Han, Z. Y. Wen, J. Y. Yao, Hankel Determinants, Padé Approximations, and Irrationality Exponents, Int. Math. Res. Notices (IMRN), 5 (2016), 1467–1496. |
[5] |
M. Coons, On the rational approximation of the sum of the reciprocals of the Fermat numbers, Ramanujan J., 30 (2013), 39–65. https://doi.org/10.1007/s11139-012-9410-x doi: 10.1007/s11139-012-9410-x
![]() |
[6] | H. Fu, G. N. Han, Computer assisted proof for Apwenian sequences, Proc. ISSAC 2016 Conference, Waterloo, Ontario, Canada, 2016. |
[7] |
R. J. Fokkink, C. Kraaikamp, J. Shallit. Hankel matrices for the period-doubling sequence. Indagationes Mathematicae, 28(1) (2017), 108-119. https://doi.org/10.1016/j.indag.2016.11.008 doi: 10.1016/j.indag.2016.11.008
![]() |
[8] |
Y. J. Guo, G. N. Han, W. Wu. Criterions for apwenian sequences, Adv. Math., 389 (2021), 107899. https://doi.org/10.1016/j.aim.2021.107899 doi: 10.1016/j.aim.2021.107899
![]() |
[9] |
Y. J. Guo, Z. X. Wen, W. Wu, On the irrationality exponent of the regular paperfolding numbers, Linear Algebra Appl, , 446 (2014), 237–264. https://doi.org/10.1016/j.laa.2013.12.023 doi: 10.1016/j.laa.2013.12.023
![]() |
[10] | G. N. Han, Hankel continued fraction and its applications, Adv. Math., 303 (2016), 295–321. |
[11] |
T. Kamae, J. Tamura, Z. Y. Wen, Hankel determinants for the Fibonacci word and Padé approximation, Acta Arithmetica, 89 (1999), 123–161. https://doi.org/10.4064/aa-89-2-123-161 doi: 10.4064/aa-89-2-123-161
![]() |
[12] | N. J. A. Sloane, The On-Line Encyclopedia of Integer Sequences, (2009). Available from: https://oeis.org/. |
[13] | J. Tamura, Padé approximation for the infinite words generated by certain substitutions and Hankel determinants, Number Theory and Its Applications, Kluwer Academic Publishers. (1990), 309–346. |
[14] |
B. Tan, Z. Y. Wen, Invertible substitutions and Sturmian sequences, European J. Combin., 24 (2003), 983–1002. https://doi.org/10.1016/S0195-6698(03)00105-7 doi: 10.1016/S0195-6698(03)00105-7
![]() |
[15] |
K. Väänänen, On rational approximations of certain Mahler functions with a connection to the Thue-Morse sequence, Int. J. Number Theory, 11 (2015), 487–493. https://doi.org/10.1142/S1793042115500244 doi: 10.1142/S1793042115500244
![]() |
\Phi_{0}(n) | 0 | 1 | 2 | 3 |
a_0(n)a_1(n)a_2(n) | 000 | 100 | 010 | 001 . |
a_0(n+f_0) | 1 | 0 | 0 | 0 |
\Phi_{1}(n) | 0 | 1 | 2 | 3 | 4 | 5 | 6 | 7 | 8 | 9 |
a_0(n)\dots a_4(n) | 00000 | 10000 | 01000 | 00100 | 00010 | 10010 | 01010 | 00001 | 10001 | 01001 . |
a_0(n+f_2) | 0 | 0 | 1 | 0 | 0 | 1 | 0 | 0 | 1 | 0 |
w | 00000 | 10000 | 01000 | 00100 | 00010 | 10010 | 01010 | 00001 | 10001 | 01001 |
v | 0010 | 0001 | ? | 0101 | 0000 | 1000 | 0100 | 0000 | 1000 | 0100 |
\Phi_{0}(n) | 0 | 1 | 2 | 3 |
a_0(n)a_1(n)a_2(n) | 000 | 100 | 010 | 001 . |
a_0(n+f_0) | 1 | 0 | 0 | 0 |
\Phi_{1}(n) | 0 | 1 | 2 | 3 | 4 | 5 | 6 | 7 | 8 | 9 |
a_0(n)\dots a_4(n) | 00000 | 10000 | 01000 | 00100 | 00010 | 10010 | 01010 | 00001 | 10001 | 01001 . |
a_0(n+f_2) | 0 | 0 | 1 | 0 | 0 | 1 | 0 | 0 | 1 | 0 |
w | 00000 | 10000 | 01000 | 00100 | 00010 | 10010 | 01010 | 00001 | 10001 | 01001 |
v | 0010 | 0001 | ? | 0101 | 0000 | 1000 | 0100 | 0000 | 1000 | 0100 |