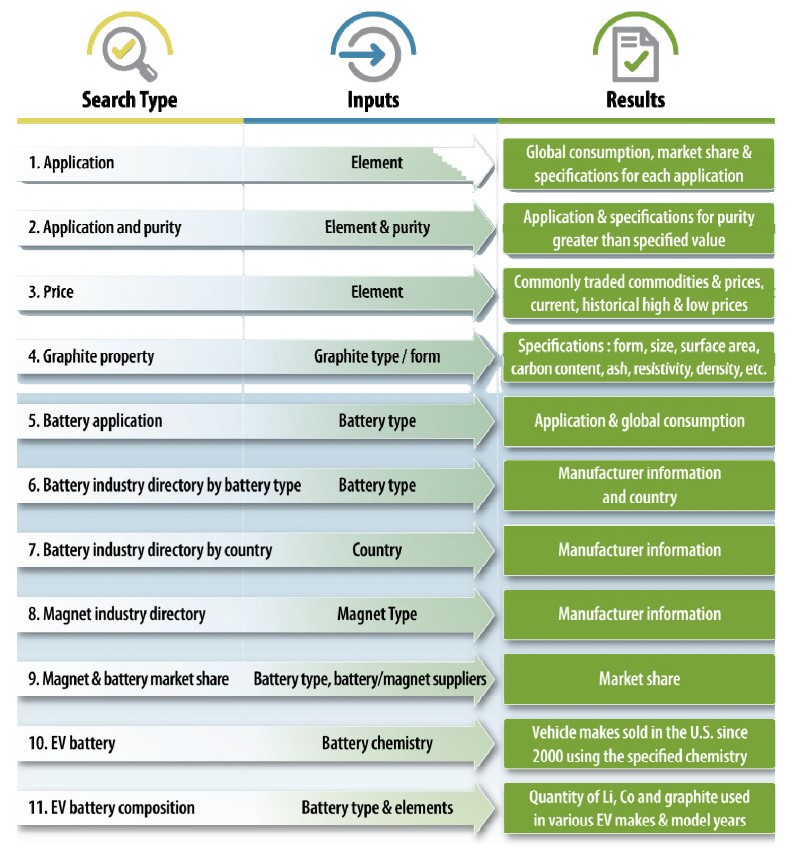
Material databases are important tools to provide and store information from material research. Rising concerns about supply-chain risks to raw materials presents a need to incorporate raw-material market and end-use application data, beyond basic chemical and physical properties, into a material database. One key challenge for researchers working on critical materials is information scarcity and inconsistency. This paper introduces, as a result of a two-year project, a critical-material commodity database (CMCD) incorporated with a low-code web-based platform that allows easy access for users and simple updates for the authors. The main goal of this project was to educate material scientists on the applications having the most impact on the supply chain and current industrial specifications/markets for each application. The objective was to provide material researchers with harmonized information so that they could gain a better understanding of the market, focus their technologies on an application with a high potential for commercialization, and better contribute to supply-chain risk reduction. While the goal was met with high receptivity, several limitations stemmed from query design, distribution platform, and quality of data source. To overcome some of these limitations and expand on CMCD's potential, we are building a public webpage with an improved interface, better data organization, and higher extensibility.
Citation: Ruby T. Nguyen, Ange-Lionel Toba, Michael H. Severson, Ethan M. Woodbury, Austin R. Carey, D. Devin Imholte. A market-oriented database design for critical material research[J]. Clean Technologies and Recycling, 2021, 1(1): 34-49. doi: 10.3934/ctr.2021002
[1] | Majid Alipanah, Sunday Oluwadamilola Usman, Apurba Kumar Saha, Hongyue Jin . Designing profitable supply chains for lithium-ion battery recycling in the United States. Clean Technologies and Recycling, 2024, 4(1): 22-42. doi: 10.3934/ctr.2024002 |
[2] | Dieuwertje L. Schrijvers, Philippe Loubet, Guido W. Sonnemann . The influence of market factors on the potential environmental benefits of the recycling of rare earth elements. Clean Technologies and Recycling, 2022, 2(1): 64-79. doi: 10.3934/ctr.2022004 |
[3] | Apurba Kumar Saha, Nighat Afroz Chowdhury, Qian Zhang, Denis Prodius, Priyesh Wagh, Hongyue Jin . Critical materials for low carbon society. Clean Technologies and Recycling, 2022, 2(4): 279-281. doi: 10.3934/ctr.2022014 |
[4] | Yi Ji, Edwin E. Kpodzro, Chad T. Jafvert, Fu Zhao . Direct recycling technologies of cathode in spent lithium-ion batteries. Clean Technologies and Recycling, 2021, 1(2): 124-151. doi: 10.3934/ctr.2021007 |
[5] | Majid Alipanah, Apurba Kumar Saha, Ehsan Vahidi, Hongyue Jin . Value recovery from spent lithium-ion batteries: A review on technologies, environmental impacts, economics, and supply chain. Clean Technologies and Recycling, 2021, 1(2): 152-184. doi: 10.3934/ctr.2021008 |
[6] | Muhammad Ali Saqib, Muhammad Sohail Abbas, Hiroyuki Tanaka . Sustainability and innovation in 3D printing: Outlook and trends. Clean Technologies and Recycling, 2024, 4(1): 1-21. doi: 10.3934/ctr.2024001 |
[7] | Shadia Moazzem, Delwyn Jones, Mathilde Vlieg, Direshni Naiker . Inaccurate polyester textile environmental product declarations. Clean Technologies and Recycling, 2022, 2(1): 47-63. doi: 10.3934/ctr.2022003 |
[8] | Andrea Di Maria, Annie Levasseur, Karel Van Acker . Assessing the long term effects on climate change of metallurgical slags valorization as construction material: a comparison between static and dynamic global warming impacts. Clean Technologies and Recycling, 2021, 1(1): 88-111. doi: 10.3934/ctr.2021005 |
[9] | Muhammad Mansoor Uz Zaman Siddiqui, Syed Amir Iqbal, Ali Zulqarnain, Adeel Tabassum . An investigative study on the parameters optimization of the electric discharge machining of Ti6Al4V. Clean Technologies and Recycling, 2024, 4(1): 43-60. doi: 10.3934/ctr.2024003 |
[10] | Chukwuebuka C. Okafor, Christian N. Madu, Charles C. Ajaero, Juliet C. Ibekwe, Chinelo A. Nzekwe . Sustainable management of textile and clothing. Clean Technologies and Recycling, 2021, 1(1): 70-87. doi: 10.3934/ctr.2021004 |
Material databases are important tools to provide and store information from material research. Rising concerns about supply-chain risks to raw materials presents a need to incorporate raw-material market and end-use application data, beyond basic chemical and physical properties, into a material database. One key challenge for researchers working on critical materials is information scarcity and inconsistency. This paper introduces, as a result of a two-year project, a critical-material commodity database (CMCD) incorporated with a low-code web-based platform that allows easy access for users and simple updates for the authors. The main goal of this project was to educate material scientists on the applications having the most impact on the supply chain and current industrial specifications/markets for each application. The objective was to provide material researchers with harmonized information so that they could gain a better understanding of the market, focus their technologies on an application with a high potential for commercialization, and better contribute to supply-chain risk reduction. While the goal was met with high receptivity, several limitations stemmed from query design, distribution platform, and quality of data source. To overcome some of these limitations and expand on CMCD's potential, we are building a public webpage with an improved interface, better data organization, and higher extensibility.
Databases are a tool commonly used by industry, academia, and governmental agencies to store and retrieve material information. Many online material databases exist; these consist of free and paid subscription services for different purposes [1]. For example, Total Materia is a paid service offering physical and mechanical properties, as well as chemical compositions, of over 350,000 materials [2]. Another paid service is Ansys Granta, a material database consisting of physical and mechanical properties that is integrable to computer-aided drawing, computer-aided experimentation, and product lifetime-management software packages [3]. MatWeb provides a free searchable database of ~140,000 engineering materials that includes their chemical, physical, mechanical, and thermal properties [4]. Materials Project [5] is another open-access online database that virtually delivers a collection of material properties to scientists for battery, solar cell, and computer-chip innovation [6]. Matmatch [7] and MatNavi [8] are also free databases that offer structures and properties for various materials, including polymers and others. Besides the elemental property levels, databases created by the United States Geological Survey (USGS) provide information regarding mineral consumption, production, price trends, price predictions, and major and minor suppliers [9]. These have served as an information centers on flows of minerals and materials essential to the U.S. economy, national security, and environmental protection.
The field of material research has changed significantly within the past decade, mainly due to the rare-earth price spike in 2010 [10]. This event attracted international attention and prompted the formation of various critical-material research consortia and initiatives globally—e.g., the European Innovation Partnership [11], European Institute of Innovation, Technology on Raw Materials (EIT Raw Materials) [12], and Critical Materials Institute (CMI) in the United States [13]. It should be noted that the focus of this research aligns with CMI's agenda as it is the funding agency. To build on this point, aggressive research agendas were introduced by CMI in the form of three focus areas: diversifying supply, developing substitutions, and improving reuse and recycling [14]. Within CMI, the author's research group focuses on economic and supply-chain analysis for CMI research in all three focus areas to quantify the research impacts of CMI projects on the respective supply-chains. To enable the work outlined in this paper, a techno-economic assessment (TEA) needs to be conducted for the project of interest based on researchers' expected outcomes for their technology. A TEA describes the technique of evaluating the overall value of a product or service regarding its economic viability [15]. Next, the authors educate researchers on market needs and requirements, which will help modify their TEAs, and evaluate potential market penetration of the technology. During this process, researchers might want to adjust their research design to increase the likelihood to be commercialized.
It is important to emphasize that our database provides input for the TEA toward market demand and requirements. Without conducting a TEA, it would be difficult for a researcher to understand the value proposition to industry and change their research design accordingly. One example scenario is a technology that can recycle batteries to produce oxides. The database shows that battery production requires sulfates as material input. Only after having a TEA done can the researcher decide whether they want to upgrade their process to produce sulfates or sell oxides at a lower price without the upgrade.
With CMI's research goal of reducing supply-chain risk and material criticality, there is a need for material databases to build knowledge about the use of critical materials and provide both supply-chain and market information. This will help bridge the gap of data harmonization and dataset connection for critical materials [16]. One example is the Raw Materials Information System (RMIS) developed by the European Commission (EC). The RMIS provides a visualization of raw-material supply-chain networks, connecting countries that supply materials to end users with additional information on products, applications, and sectors [17]. This information system includes data pertaining to resources and reserves, supply, demand, raw-material supply chain, markets, research and development, and environmental- and social-sustainability aspects. Currently, the RMIS covers 14 raw materials: aluminum, borates, chromium, cobalt, coking coal, iridium, iron, lithium, natural graphite, natural rubber, palladium, platinum, rhodium, and ruthenium. Additionally, the RMIS database contains another module detailing raw materials in batteries, battery weights per application, commodities per battery chemistry, and commodities in electric mobility.
Although substantial information can be obtained from the RMIS, this platform does not provide information for researchers to make decisions about their research and development technologies at an individual-project level. From the author's observations of CMI researchers through frequent collaboration and interaction, there is a disconnect between their research and potential supply-chain and market outcomes. More specifically, commercialization of a substitution research technology will impact the supply chains of existing and substituted raw materials, which might or might not reduce criticality. Researchers focus most of their effort on developing novel technologies (such as solvent extraction, biosorption, etc.) while paying little attention to product requirements in terms of purity, downstream applications (e.g., magnets, batteries, catalysts) in which the product may be used, and price implications. For example, rare earths are usually extracted in the form of mixed rare-earth oxides. Separating these rare earths will require additional steps that fall beyond the scope of extractive hydrometallurgy. It is vital that critical-materials researchers developing these novel processes understand the different specifications of the critical-material compounds they may be generating and refining (e.g., mischmetal vs. rare-earth oxides). With this knowledge, they will know how their technology can fit with commercial processes and what value proposition they bring to industry.
With the goal of educating material researchers, starting with CMI researchers, we designed a critical material commodity database (CMCD) to answer three key questions:
(1) For a given element, what are the applications, their corresponding market shares, and their required product specifications?
(2) Given that different applications require different purities, can purity be used to help critical-materials researchers make economic decisions regarding potential market applications for their technologies?
(3) Is there a simple way to estimate economic value of a research product (i.e., a commodity not traded commercially) based on commonly traded commodities?
The objective of the first question is to understand the main uses of each selected element so that researchers can focus on applications that have the greatest impact on reducing criticality. Purity and impurity levels help researchers understand industry requirements. From there, they can decide whether additional processes are needed for their current products to meet market requirements. As a result, we pose the second research question to find applications based on purity levels. The third question aims to support the economic assessment that eventually would help researchers make decisions about the next technology readiness level (TRL) of their technologies. For example, commodity prices are usually reported for commonly traded products, leaving gaps for obtaining prices or quantifying economic value for less-common products. In addition, many CMI researchers focus more on the novelty of their process or products, and less on economics unless they are encouraged to commercialize the technologies. This new request for commercialization creates a challenge for researchers that the CMCD aims to solve.
In addition to general queries to address the three aforementioned research questions, eight queries are included to answer specific CMI project needs, including graphite properties, magnet and battery markets, and battery types used in different electric vehicles by make and model year. In the next section, the components and structure of our database are detailed. Visual illustrations of the interface are depicted, and results are reported. Finally, user feedback, limitations, prospects, and lessons learned from our two-year effort are discussed.
The starting point for the list of critical materials implemented in our database comes from the results of an annual criticality assessment conducted by the Colorado School of Mines (CSM), a CMI member [18]. In the first five years of CMI, a main research focus was on rare-earth elements (REEs). In the second five years, CMI wanted to expand the scope of critical materials beyond REEs. From 32 elements identified as critical in the Department of Energy's 2015 Quadrennial Technology Review [19], CSM researchers shortened the list using three focus strategies: (1) material criticality to energy technologies; (2) consideration of emerging technology trends alongside their effects on material supply chains; and (3) inadequate extraction efficiency of joint or co-production of materials [18]. To further explain the third strategy, many critical materials are obtained through joint production that could be in short supply if the economics of the main product are not viable and the extraction efficiency of the joint product is low. Their final list consists of REEs, battery materials (lithium, cobalt, graphite, manganese), gallium, indium, platinum-group metals, and vanadium. Based on the CMI research portfolio, we added arsenic, copper, nickel, and tellurium to their list to support the economic assessment of critical material extraction and recovery through co-production of specific CMI projects. A total of 29 elements comprise our final list: arsenic, cerium, cobalt, copper, dysprosium, erbium, europium, gallium, gadolinium, germanium, graphite, holmium, indium, iridium, lanthanum, lithium, manganese, neodymium, nickel, palladium, praseodymium, platinum, rhodium, ruthenium, samarium, terbium, tellurium, vanadium, and yttrium. Although scandium is an REE, it is not included because it is a niche market with very small demand.
Within the scope of this work, search type is defined as a query with specific inputs to display outputs in a meaningful way. In other words, each combination of inputs and outputs within our database is called a search type. Defining search types is the most-critical step in designing a database because it affects data organization and query logic. Driven by the research questions, this step starts by specifying user inputs, desired outputs, and intermediate data layers to achieve those outputs. Next, data gathering is conducted to obtain the needed data.
To give an example, the first research question aims to solve the question of an element's applications, market shares, and product specifications. "Elements" are selected as inputs and outputs are "applications, market shares, and specifications" for each application, as shown in Figure 1 for Search Type 1. Data on annual market share for major applications and annual global consumption of the 29 specified elements are compiled. Within each application, information on industrial specifications is gathered, including purity level and accepted thresholds of impurities. Data sources include manufacturer websites and datasheets (reporting elemental purities, related applications, etc.), government agency websites (such as the USGS, which provides element-application information), and free public market-research reports (e.g., Johnson Matthey, with consumption data) [15,20,21,22,23,24,25,26,27,28,29,30,31,32,33,34,35,36,37]. The compilation of these various sources provides immense value to any critical-materials researcher by reducing the time expenditure to gather these data.
Inputs and outputs for the second question are shown in Figure 1 as Search Type 2. Because applications for lower purity levels outnumber higher purity levels, the query is designed to return applications with a higher purity than user inputs to shorten the list of results. Users can always reduce purity input if query results are too few. In addition, although high-purity materials can be used in applications requiring lower purity, this choice does not make economic sense because the cost of purifying outweighs the prices that lower-purity applications are willing to pay. Although this query seems to be the reverse of the first one, it employs the same dataset.
The third research question involves estimating economic value of research products. In this paper, research products are defined as the chemical forms of materials produced from a specific technology. For example, if a technology can produce rare-earth oxides from end-of-life magnets, the research products are rare-earth oxides. As shown in Figure 1, the structure of Search Type 3 aims to provide baseline prices for researchers to further calculate the values of research products. The main data source for this search type is Argus Media [38].
In addition to searches addressing the three research questions, we included eight more queries in this CMCD to support a broader research portfolio of CMI (Figure 1). Projects within CMI have different knowledge needs, and the CMCD attempts to address several projects' individual needs. For example, Search Type 4 provides specifications for a project working on synthesizing graphite using a low-temperature process. The CMCD allows this individual project to examine the current market of graphite to find an applicable area of market penetration should the technology prove successful. Search Types 5, 6, and 7 support projects that develop recycling and extracting technologies from virgin minerals for supply to the battery markets. While Search Type 5 shows how different battery types and chemistries are used in various applications, Search Types 6 and 7 yield manufacturers for each battery type and the countries in which those manufacturers are located. Specific data for these search types and the associated sources can be found in the Supplementary. Search Type 8 returns magnet companies from an input of magnet type, country of manufacture, and role in the supply chain—i.e., manufacturer, distributor, recycler, or retailer. Market share of both magnet and battery types can be found from Search Type 9. This search also offers values in the import and export of magnets in the global market and market shares of major electric vehicle (EV)-battery suppliers.
For researchers working on recycling technologies, EV battery-composition information is important. To address this need for EV batteries, Search Type 10 gives battery information for each vehicle model and model year. Search Type 11 returns the content of cobalt, graphite, or lithium in each vehicle model and model year. The main cathode chemistries considered in Search Type 10 are nickel-metal hydride (NiMH, NiOH2), lithium manganese oxide (LMO, LiMn2O4), lithium iron phosphate (LFP, LiFePO4), lithium nickel cobalt aluminum oxide (NCA, LiNiCoAlO2), and lithium manganese cobalt oxide mixed with lithium manganese oxide (NMC-LMO). Major EV types include most battery electric vehicles (BEVs), plug-in hybrids (PHEVs) and hybrids for vehicles sold in the U.S. from 1999 to 2017 [39]. Identification and analysis from a larger pool of data show that the Nissan Leaf, Tesla Model S, Tesla Model X, BMW i3, and Chevy Bolt account for 80% of total sold BEVs while Toyota Prius plug-in, Chevy Volt, Ford Fusion Energi, and Ford C-Max Energi account for 67% of sold PHEVs [39]. The ten hybrid models occupying 88% of sold HEVs include the Toyota Prius hybrid, Toyota Camry hybrid, Honda Civic hybrid, Ford Fusion hybrid, Lexus RX 400h/450h, Toyota Prius C, Toyota Highlander hybrid, Toyota Prius V, Ford Escape hybrid, and Hyundai Sonata hybrid [39]. The highlighted vehicles show the majority stakeholders in respective categories; however, the database also includes a more comprehensive list of vehicles for assessment beyond the majority stakeholders. This selection was gathered from data from the Alternative Fuels Data Center [40]. The material quantities within each vehicle model's battery pack were calculated by considering cell chemistry, cell capacity (Ah), and number of cells. This calculation method mostly relies on the Bat Pac model [41]. Battery information was collected from the online datasheets of vehicle manufacturers, news articles, previously published academic papers, and reports on EVs. More details can be found in the Supplementary.
To create the database, we used Caspio, a cloud-based platform, with low-code requirements and a low-cost option to execute user queries. Caspio uses Microsoft Standard Query Language (SQL) Server, a relational database management system (RDBMS) to compile tables and store procedures [42]. The RDBMS serves as an interface between the database and end users, allowing them to perform mainly data definition, update, and retrieval [43].
In order to execute the interface between the database and end users, two main components are required: tables and data pages. These are interlinked as information stored in tables is captured by data pages, which are application interfaces used to display, visualize, and edit data, as well as provide query capability. They can be used to build both user interfaces and links between data pages.
Interface building: Data pages can represent data in different formats, including forms, reports, and charts. Results from queries are displayed through reports and charts while queries are performed via forms. Different searches will call for different types of data pages. For instance, to show the yearly consumption of lithium for a given application, we built a data page of the type chart for visual display.
Data page linkages: Each data page contains an embedded link that helps users navigate between multiple data pages. Once deployed, the link can also be copied and placed on any website or in a document or an email for sharing. In that sense, adding the link for Data Page 2 on Data Page 1 would enable users to access information on Data Page 2 by following the link. For example, to find all applications of lithium, we may need a data page of type report. On that data page, we may add a link to a data page of type chart to visualize lithium global consumption over time.
The test CMCD was shared with over 100 CMI researchers from all research focus areas including (1) diversifying supply; (2) developing substitutions; (3) improving reuse and recycling; and (4) cross-cutting research. First, a demonstration webinar was presented to each focus-area group. After that, a user's manual was distributed to all meeting attendees. A focus group of 10 people was selected from the original group to go over each search type and document their experiences. Feedback was collected via email for evaluation and improvements.
The first search type of the commodity database answers the first research question, which was to establish applications and corresponding market shares. In this case, application information for lithium is chosen (Figure 2). After an elemental symbol is selected (top left corner), a report table shows up with five columns. The first column lists applications. The second column provides a link to "Element Types and Purities" for each application. The third and fourth column lists links to "Percent Global Market" and "Global Consumption" per application, respectively. The last column provides sources of information. When links are clicked in the second column, a new report appears with information on "Element Type", "Purity", and "Chemical Form". To obtain additional information, users can click on the "View Details" link (top right corner of Figure 2). To view charts of market share and global consumption, users can click on links provided in the third and fourth columns (bottom right charts of Figure 2).
Applications and their market share are a straightforward query to set up. The only challenge was gathering data. Most publicly available data do not provide a comprehensive time-series collection of elemental consumption. However, select subscription services and sources possess more comprehensive data, but it would be costly to obtain annual consumption for roughly 30 elements, and obtaining permissions to use data from subscription services in this database would be difficult.
It is possible to find applications based on purity. Figure 3 shows an example for dysprosium. Except in the case of ferro-dysprosium, other applications require a purity of 99% or higher, and the chemical form could be metal or oxide. The metal form is listed twice because it is used for two different applications that have different specifications. When "Application" is selected, more information on application type can be found, with corresponding application market share and global consumption, similar to Figure 2. Details on required specifications can be found by following "View Details". More specifically, metal dysprosium at 99% purity can be used in magnets or Terfenol-D [20], and dysprosium oxide at 99% purity can be used to dope various ceramics and glasses [20,44].
Estimating prices for research products based on posted prices of commonly traded commodities proves to be challenging due to the opaqueness of some critical-materials markets, including REEs. To address this problem, a possible solution can be found within the mining industry. In this industry, basket price is commonly used to calculate a weighted price of the product based on its composition given known commodity prices [45]. This basket-price approach could also be used to estimate the highest value of research products for which there is no established price. While this is a commonly used method for estimating the value of mining products, it does not account for additional processing costs and can overvalue a given product. For example, a mixed rare-earth oxide is recovered as a product having a composition of 15% lanthanum, 26% cerium, 2% praseodymium, 17% neodymium, 2% samarium, 1% europium, 3% gadolinium, 3% dysprosium, 1% holmium, 2% erbium, 2% ytterbium, and 26% yttrium. Of those 12 elements, lanthanum, cerium, and yttrium are major compositions, but their prices are quite low. As a result, the major elements making up the price of the mixed oxide are praseodymium, neodymium, and dysprosium. Given the prices of these elements as of February 2021, the recovered-product maximum prices could be priced at $32.86/kg at the highest because oxide prices for high purity of more than 99% were used for basket price.
This section highlights two additional features of the CMCD that are not mentioned in the research questions. The first one is the ability to search battery types used in each EV make. As shown in Figure 4, when NCA is selected from the drop-down menu for battery types, nine common EV makes sold in the U.S. since 2000 that have used this battery type are listed [37]. Besides NCA, other battery types used in these nine makes are also tabulated by model year to give some historical perspectives of battery-chemistry evolution. For example, the Toyota Prius used NiMH batteries between 2000 and 2015. Starting in 2016, NCA was used in this make. This evolution shows the improvements that have occurred in EV-battery technology and the potential availability of different battery chemistries as a recycling feedstock. The first EV to use NCA was the Tesla Roadster in 2008. In the case of the Toyota Rav4, NCA was used in 2013, but model 2014 observed a switch to NMC-LMO. Additional links are provided to the right of the main report table shown in Figure 4 to view details on the battery type used in the first model year of each make.
The next key feature is the ability to search for cobalt, lithium, or graphite content in batteries of various EV makes and model years. In the example shown in Figure 5, cobalt content in NCA batteries is selected. Results are returned for seven EV makes using NCA batteries, including Mercedes B-Class Electric/B250e, Tesla Model 3, Tesla Model S, Tesla Model X, Tesla Roadster, Toyota Prius (plug-in), and Toyota Rav4. Clicking on "Element Quantity per Car" for a specific make (e.g., Tesla Model S) causes a chart to display cobalt content for this make over time in kg/car (top right corner). This chart shows a trend of reduced cobalt content in NCA chemistry over time. If users want to know the total cobalt content in all Tesla Model S vehicles sold in the U.S. since 2012, they can follow the link "Element Consumption" to view a chart shown in the bottom right of Figure 5. These numbers simply represent cobalt content per vehicle multiplied by total annual sales of that make.
Researchers across CMI are receptive to the CMCD because it allows more-informed decisions regarding their technologies and projects. The three most-used searches are application by element, application based on purity, and price. Since CMCD was made available, researchers have paid more attention to the product specifications to improve their technology economics. CMI researchers using element specification and purity levels in their work can compare data they customarily used with data from CMCD, and they find those to be similar, making CMCD their go-to source. Feedback included suggestion that we expand the list of critical materials and add recycling share of total material supply.
At the interactivity level, researchers encounter some inconveniences caused by the interface. For example, when following a link to access more information from the main result page, users have to open a new tab. Otherwise, they cannot navigate back to the main result page. Another comment regarded information type to be displayed; more specifically, some researchers would like to see suppliers and prices of an element while viewing the specifications for a specific application. In other words, they would like to combine application, price, and supplier searches into one search return. There is also a request to see all critical elements used in a specific application or search by application instead of by element. For visual purposes, some users would like to see application market share and global-consumption charts on the same page. While some of these shortcomings can be overcome by changing query design (e.g., search by application instead of by element), other changes are not possible due to Caspio's design. Because each query in Caspio is represented by a separate link, it is unable to follow that link and come back to the main result page without opening it in a new tab. Similarly, charts of market share and global consumption are from two separate queries and cannot be displayed on the same page. Regarding the display of suppliers, prices, and specifications on the same page, the collected data are not sufficient to display suppliers by element, given that only magnet- and battery-supplier data were collected. This feature will be improved in the next version of the database. Listing all elements used in an application or search by application is a good suggestion that we will include in the next version.
There are two main limitations to the database. The first limitation lies within the distribution method. Because the database uses a low-code platform, each search is represented by an individual web link which prompts for inputs upon opening, and any user who has access to that link can perform the query. However, Caspio does not enable a home page to list all search types with detailed descriptions so that users can navigate from one query to another. To overcome this distributed manner, we have created a user's manual and distributed it to CMI researchers. However, this distribution method is still limited, not able to reach other users who may find the database useful. The second limitation pertains to the quality of data sources. In the critical-materials research domain, market information and material standards are scarce, even with subscriptions to reputable supply-chain intelligence such as Roskill and ASTM Compass. This is the only limitation that not yet been overcome.
Given the positive feedback, there is a potential to expand this effort. In its current form, the CMCD only considers raw materials, without their downstream applications, which have a more-complex supply-chain structure. For example, magnets can go into motors, which can go into different applications like wind turbines, electric vehicles, etc. The CMCD could be expanded to (1) follow various supply chains using the same raw material (e.g., supply chains for all cobalt applications); (2) all materials contained in a specific application; and (3) all supply chains supporting one application (e.g., solar energy). In addition, to making the CMCD more centralized, we are in the process of building a public webpage. This effort will take more resources, but it will offer broader access, a better interface, and more flexibility in query design and interactivity.
Along with the listed limitations and prospects, we faced challenges and gathered some lessons learned. The first issue with CMCD has to do with maintenance of the database and element prices. CMCD will have to be maintained regularly for data updates. Currently, prices are updated every two weeks, and we anticipate the effort for this task will grow substantially given calls from researchers to increase the number of elements. Because most price data are obtained from subscription, maintaining the CMCD would become costly over time.
The second issue involves organizing data. Hosting large quantities of materials data, compiling into tables, and linking them via a relational database system require effort. CMCD designers had to develop logical data structures in a way that minimized the number of tables in order to achieve simplicity and clarity while ensuring efficient architecture to return query results in a minimal number of queries. This required rigorous tracking of identifiers in tables to build relationships between existing tables and facilitating expansion to future ones, if needed.
Concerns regarding global supply-chain risks create a need for a market-oriented critical-materials commodity database that helps shed light onto the most-impactful applications, as well as industry specifications, prices, product composition, and global consumption for each element. In this paper, we described our effort to construct such a database using a low-code web-browsing platform for query formulation and distribution. While we attempted to answer three research questions, two of them could be addressed directly using the database format—namely, application by element and application by purity. The third question, regarding research product-value determination, required a basket-price calculation approach that takes into account known commodity prices and product composition. The database enabled users to obtain known commodity prices as inputs for research-product valuation. In general, CMI researchers were receptive to the database and would like to see us expand its content. Inconveniences came from the interface, which is constrained by the cloud platform that we used. While the CMCD proved to be useful, maintaining it would be costly in terms of both the effort to update data and access to data sources. To overcome the CMCD limitations and expand its potential, we are building a public website. With this new version, the CMCD will be able to reach a broader audience and provide more supply-chain information for downstream applications.
This work is supported by the Critical Materials Institute, an Energy Innovation Hub funded by the U.S. Department of Energy, Office of Energy Efficiency and Renewable Energy, Advanced Manufacturing Office under Grant AL-12-350-001. This manuscript has been authored by Battelle Energy Alliance, LLC under Contract No. DE-AC07-05ID14517 with the U.S. Department of Energy. The United States Government retains and the publisher, by accepting the article for publication, acknowledges that the United States Government retains a nonexclusive, paid-up, irrevocable, world-wide license to publish or reproduce the published form of this manuscript, or allow others to do so, for United States Government purposes.
The authors declare no competing interest.
[1] | Toulas B, Material Database - Top 10 Engineering Materials Resources on the Web. Engineering Clicks, 2018. Available from: https://www.engineeringclicks.com/material-database/. |
[2] | Total Materia, The world's most comprehensive materials database. Total Materia, 2020. Available from: https://www.totalmateria.com/page.aspx?ID=Home&LN=EN. |
[3] | Ansys Granta, Ansys Granta EduPack: Enhance your Materials Teaching. Ansys Granta, 2021. Available from: https://www.ansys.com/products/materials/granta-edupack. |
[4] | MatWeb, Online Materials Information Resource - MatWeb. MatWeb, 2020. Available from: http://www.matweb.com/. |
[5] | Department of Energy, Lawrence Berkley National Laboratory, National Science Foundation, About the Materials Project. Department of Energy, n.d. Available from: https://materialsproject.org/about. |
[6] | Duque T, Making a Material World Better, Faster Now: Q & A With Materials Project Director Kristin Persson. Berkley Lab News Center, 2020. Available from: https://newscenter.lbl.gov/2020/05/08/materials-project-qa-persson/. |
[7] | Matmatch, The World's Leading Material Connection Platform. Matmatch, 2020. Available from: https://matmatch.com/. |
[8] | MatNavi, NIMS Materials Database. MatNavi, 2020. Available from: https://mits.nims.go.jp/en/. |
[9] | USGS. National Minerals Information Center. USGS, n.d. Available from: https://www.usgs.gov/centers/nmic. |
[10] |
Sprecher B, Daigo I, Spekkink W, et al. (2017) Novel indicators for the quantification of resilience in critical material supply chains, with a 2010 rare earth crisis case study. Environ Sci Technol 51: 3860-3870. doi: 10.1021/acs.est.6b05751
![]() |
[11] | European Commission, Policy and strategy for raw materials. European Commission, n.d. Available from: https://ec.europa.eu/growth/sectors/raw-materials/policy-strategy_en. |
[12] | EIT, EIT RawMaterials: Developing raw materials into a major strength for Europe. European Institute of Innovation & Technology, 2020. Available from: https://ec.europa.eu/growth/sectors/raw-materials/policy-strategy_en. |
[13] | Matulka R, Increasing Access to Materials Critical to the Clean Energy Economy. Department of Energy, 2013. Available from: https://www.energy.gov/articles/increasing-access-materials-critical-clean-energy-economy. |
[14] | DOE, Critical Materials Strategy. Department of Energy, 2011. Available from: https://www.energy.gov/sites/prod/files/2016/12/f34/2011%20Critical%20Materials%20Strategy%20Report.pdf. |
[15] | Acharya M, Techno-Economic Analysis (TEA). ARPA-E, 2021. Available from: https://arpa-e.energy.gov/sites/default/files/7%20TEA%20for%20REFUEL%20-%20ACHARYA.pdf. |
[16] | León MFG, Dewulf J (2020) Data quality assessment framework for critical raw materials. The case of cobalt. Resour Conserv Recycl 157: 104564. |
[17] | European Commission, Whole Supply Chain Network. European Commission, 2018. Available from: https://rmis.jrc.ec.europa.eu/apps/scv/#/. |
[18] | Eggert R (2018) Material Criticality and Carbon Abatement. Golden, CO: Critical Materials Institute, In Press. |
[19] | Department of Energy, An assessment of Energy Technologies and Research Opportunities. Department of Energy, 2015. Available from: https://www.energy.gov/quadrennial-technology-review-2015. |
[20] | METALL The Rare Earth Company, Rare Earth Products. METALL Rare Earth Limited, n.d. Available from: http://metall.com.cn/. |
[21] | Johnson Matthey, Market Research - PGM. Johnson Matthey, n.d. Available from: http://www.platinum.matthey.com/services/market-research. |
[22] |
Wang X, Lei Y, Ge J, et al. (2015) Production forecast of China's rare earths based on the Generalized Weng model and policy recommendations. Resour Policy 43: 11-18. doi: 10.1016/j.resourpol.2014.11.002
![]() |
[23] | Nornickel, Platinum - Market overview - Nornickel Annual Report 2017. Nornickel, 2018. Available from: https://ar2017.nornickel.com/metals-market/platinum. |
[24] | Nornickel, Palladium - Global metals market - Strategic report - Nornickel Annual report 2016. Nornickel, 2017. Available from: https://ar2016.nornik.ru/en/strategy/metals-market/palladium. |
[25] |
Rioja RJ, Liu J (2012) The Evolution of Al-Li Base Products for Aerospace and Space Applications. Metall Mater Trans A 43: 3325-3337. doi: 10.1007/s11661-012-1155-z
![]() |
[26] | Revert Alloys, Metals, Metals & Alloys. Revert Alloys and Metals, n.d. Available from: http://www.revertalloysandmetals.com/alloys/. |
[27] | Baylis R, Constantinides S, High Performance Magnetics. Arnold Magnetics, 2017. Available from: https://www.arnoldmagnetics.com/wp-content/uploads/2017/10/Cobalt-Essential-to-High-Performance-Magnetics-Baylis-and-Constantinides-Cobalt-Conference-2012.-psn-hi-res.pdf. |
[28] | Livent, High-Performance Lithium Products & Solutions. Livent, 2021. Available from: https://livent.com/market-products/. |
[29] | IARC, Metallic Cobalt Particles (With or Without Tungsten Carbide). Volume 86 ed: IARC Monographs. IARC, 2018. Available from: https://monographs.iarc.who.int/wp-content/uploads/2018/06/mono86-6.pdf. |
[30] | Reade Advanced Materials, Specialty chemical solids distributor. Reade, 2020. Available from: https://reade.com/products. |
[31] | Asbury Carbons, Graphite. Asbury Carbons, 2019. Available from: https://asbury.com/materials/graphite/. |
[32] | Indium Corporation, Product Data Sheet Library. Indium Corporation, n.d. Available from: https://www.indium.com/technical-documents/product-data-sheets/english/. |
[33] | Sharp Ferro Alloys Limited, Silico Magnanese. Sharp Ferro Alloys Limited, 2014. Available from: http://www.sharpferro.com/silico-manganese.html. |
[34] | ESPI Metals, Palladium. ESPI Metals, 2021. Available from: https://espimetals.com/shop/elements/palladium. |
[35] | Copper Development Association Inc., Copper Alloys Advanced Search for Wrought and Cast Copper Alloys. Copper Development Association Inc., 2021. Available from: https://alloys.copper.org/. |
[36] | Battery University, Nickel-based Batteries. CADEX, 2021. Available from: https://batteryuniversity.com/learn/article/nickel_based_batteries. |
[37] | American Elements, The Advanced Materials Manufacturer. AmericanElement, n.d. Available from: https://www.americanelements.com/. |
[38] | ArgusMedia, Argus Metal Prices. Argus Media, 2019. Available from: http://www.argusmedia.com/metals/argus-metal-prices/. |
[39] | InsideEVs, Historical U.S. EV sales. InsideEVs, 2018. Available from: https://insideevs.com/monthly-plug-in-sales-scorecard/. |
[40] | Alternative Fuels Data Center Vehicles, AFVs and HEVs. AFDC, 2019. Available from: https://afdc.energy.gov/data/. |
[41] | Argonne National Laboratory, BatPac Home. Argonne National Laboratory, n.d. Available from: https://www.anl.gov/partnerships/batpac-battery-manufacturing-cost-estimation. |
[42] | Varga S, Cherry D, D'Antoni J (2016) Introducing Microsoft SQL Server 2016: Mission-Critical Applications, Deeper Insights, Hyperscale Cloud, Redmond: Microsoft Press. |
[43] | Coronel C, Morris S (2016) Database systems: design, implementation, & management, Boston: Cengage Learning. |
[44] | Goonan T, Rare Earth Elements - End Use and Recyclability. United States Geological Service, 2011. https://pubs.usgs.gov/sir/2011/5094/pdf/sir2011-5094.pdf. |
[45] | Hykawy J, Chudnovsky T, Rare Earths Update 2020: Things are not Great, but They Will Get Better. Stormcrow, 2020. Available from: https://static1.squarespace.com/static/535e7e2de4b088f0b623c597/t/5e4162ff4b6b8940eedf177a/1581343504580/20200210-Stormcrow-REE+Report+2020+v3.pdf. |
![]() |
![]() |
1. | Apurba Kumar Saha, Nighat Afroz Chowdhury, Qian Zhang, Denis Prodius, Priyesh Wagh, Hongyue Jin, Critical materials for low carbon society, 2022, 2, 2770-4580, 279, 10.3934/ctr.2022014 |