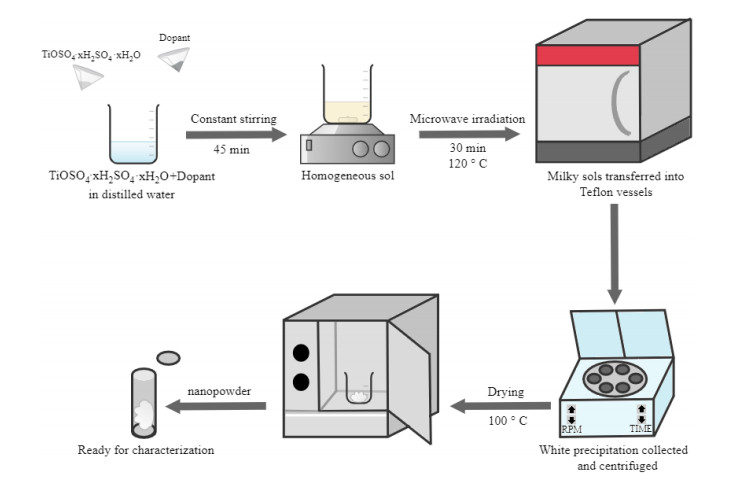
Nanostructured titanium dioxide (TiO2) among other oxides can be used as a prominent photocatalytic nanomaterial with self-cleaning properties. TiO2 is selected in this research, due to its high photocatalytic activity, high stability and low cost. Metal doping has proved to be a successful approach for enhancing the photocatalytic efficiency of photocatalysts. Photocatalytic products can be applied in the building sector, using both building materials as a matrix, but also in fabrics. In this study undoped and Mn-In, Mn-Cu, In-Ni, Mn-Ni bimetallic doped TiO2 nanostructures were synthesized using the microwave-assisted hydrothermal method. Decolorization efficiency of applied nanocoatings on fabrics and 3-D printed sustainable blocks made from recycled building materials was studied, both under UV as well as visible light for Methylene Blue (MB), using a self-made depollution and self-cleaning apparatus. Nanocoated samples showed high MB decolorization and great potential in self-cleaning applications. Results showed that the highest MB decolorization for both applications were observed for 0.25 at% Mn-In doped TiO2. For the application of 3-D printed blocks Mn-In and In-Ni doped TiO2 showed the highest net MB decolorization, 25.1 and 22.6%, respectively. For the application of nanocoated fabrics, three samples (Mn-In, In-Ni and Mn-Cu doped TiO2) showed high MB decolorization (58.1, 52.7 and 47.6%, respectively) under indirect sunlight, while under UV light the fabric coated with Mn-In and In-Ni doped TiO2 showed the highest MB decolorization rate 26.1 and 24.0%, respectively.
Citation: Evangelos Karagiannis, Dimitra Papadaki, Margarita N. Assimakopoulos. Circular self-cleaning building materials and fabrics using dual doped TiO2 nanomaterials[J]. AIMS Materials Science, 2022, 9(4): 534-553. doi: 10.3934/matersci.2022032
[1] | Nhung Thi-Tuyet Hoang, Anh Thi-Kim Tran, Nguyen Van Suc, The-Vinh Nguyen . Antibacterial activities of gel-derived Ag-TiO2-SiO2 nanomaterials under different light irradiation. AIMS Materials Science, 2016, 3(2): 339-348. doi: 10.3934/matersci.2016.2.339 |
[2] | Miguel García-Tecedor, Félix del Prado, Carlos Bueno, G. Cristian Vásquez, Javier Bartolomé, David Maestre, Tomás Díaz, Ana Cremades, Javier Piqueras . Tubular micro- and nanostructures of TCO materials grown by a vapor-solid method. AIMS Materials Science, 2016, 3(2): 434-447. doi: 10.3934/matersci.2016.2.434 |
[3] | Ahmed Al-Ramthan, Ruaa Al Mezrakchi . Investigation of cementitious composites reinforced with metallic nanomaterials, boric acid, and lime for infrastructure enhancement. AIMS Materials Science, 2024, 11(3): 495-514. doi: 10.3934/matersci.2024025 |
[4] | Ahmed Z. Abdullah, Adawiya J. Haider, Allaa A. Jabbar . Pure TiO2/PSi and TiO2@Ag/PSi structures as controllable sensor for toxic gases. AIMS Materials Science, 2022, 9(4): 522-533. doi: 10.3934/matersci.2022031 |
[5] | Iryna Markevich, Tetyana Stara, Larysa Khomenkova, Volodymyr Kushnirenko, Lyudmyla Borkovska . Photoluminescence engineering in polycrystalline ZnO and ZnO-based compounds. AIMS Materials Science, 2016, 3(2): 508-524. doi: 10.3934/matersci.2016.2.508 |
[6] | Anita Haeussler, Stéphane Abanades, Julien Jouannaux, Martin Drobek, André Ayral, Anne Julbe . Recent progress on ceria doping and shaping strategies for solar thermochemical water and CO2 splitting cycles. AIMS Materials Science, 2019, 6(5): 657-684. doi: 10.3934/matersci.2019.5.657 |
[7] | Akira Nishimura, Ryuki Toyoda, Daichi Tatematsu, Masafumi Hirota, Akira Koshio, Fumio Kokai, Eric Hu . Optimum reductants ratio for CO2 reduction by overlapped Cu/TiO2. AIMS Materials Science, 2019, 6(2): 214-233. doi: 10.3934/matersci.2019.2.214 |
[8] | Akira Nishimura, Tadaki Inoue, Yoshito Sakakibara, Masafumi Hirota, Akira Koshio, Fumio Kokai, Eric Hu . Optimum molar ratio of H2 and H2O to reduce CO2 using Pd/TiO2. AIMS Materials Science, 2019, 6(4): 464-483. doi: 10.3934/matersci.2019.4.464 |
[9] | Pham Van Viet, Le Van Hieu, Cao Minh Thi . The directed preparation of TiO2 nanotubes film on FTO substrate via hydrothermal method for gas sensing application. AIMS Materials Science, 2016, 3(2): 460-469. doi: 10.3934/matersci.2016.2.460 |
[10] | Khang Duy Vu Nguyen, Khoa Dang Nguyen Vo . Magnetite nanoparticles-TiO2 nanoparticles-graphene oxide nanocomposite: Synthesis, characterization and photocatalytic degradation for Rhodamine-B dye. AIMS Materials Science, 2020, 7(3): 288-301. doi: 10.3934/matersci.2020.3.288 |
Nanostructured titanium dioxide (TiO2) among other oxides can be used as a prominent photocatalytic nanomaterial with self-cleaning properties. TiO2 is selected in this research, due to its high photocatalytic activity, high stability and low cost. Metal doping has proved to be a successful approach for enhancing the photocatalytic efficiency of photocatalysts. Photocatalytic products can be applied in the building sector, using both building materials as a matrix, but also in fabrics. In this study undoped and Mn-In, Mn-Cu, In-Ni, Mn-Ni bimetallic doped TiO2 nanostructures were synthesized using the microwave-assisted hydrothermal method. Decolorization efficiency of applied nanocoatings on fabrics and 3-D printed sustainable blocks made from recycled building materials was studied, both under UV as well as visible light for Methylene Blue (MB), using a self-made depollution and self-cleaning apparatus. Nanocoated samples showed high MB decolorization and great potential in self-cleaning applications. Results showed that the highest MB decolorization for both applications were observed for 0.25 at% Mn-In doped TiO2. For the application of 3-D printed blocks Mn-In and In-Ni doped TiO2 showed the highest net MB decolorization, 25.1 and 22.6%, respectively. For the application of nanocoated fabrics, three samples (Mn-In, In-Ni and Mn-Cu doped TiO2) showed high MB decolorization (58.1, 52.7 and 47.6%, respectively) under indirect sunlight, while under UV light the fabric coated with Mn-In and In-Ni doped TiO2 showed the highest MB decolorization rate 26.1 and 24.0%, respectively.
Nanotechnology is bringing new materials and new possibilities to industries contributing to an improved living environment and comfort on a daily base. One of these is the construction industry, where nanotechnology can generate products with many unique characteristics that can improve the performance of current construction materials introducing new applications with enhanced sustainable properties [1]. New materials and products based on nanotechnology can be found in building matrices, coatings or insulating applications. Specifically, lighter and stronger structural composites, low maintenance coatings, better cementitious materials, lower thermal transfer rate of fire retardant and insulation, self-cleaning windows, smog-eating concrete, antimicrobial coatings, photovoltaic devices that converts sunlight to electricity and many other advances [2,3,4,5]. The incorporation of recycled construction materials and 3-D printing in construction industry is a further step towards innovation and sustainability. Nonetheless the building industry accounts for 40% of the energy consumption and 50% of the greenhouse gas emissions in Europe [6].
Within the EU more than 50% of all extracted materials are attributed to buildings. The exploitation of natural resources is a serious threat to the natural, social and economic systems in the EU. A transition to both a deep and circular renovation process with the use of recycled materials in the construction industry is necessary to meet the challenge of decarbonization [7]. The use of recycled materials in the construction industry totally alters the value chain linked to all stages of intervention in existing buildings, making the operations sustainable in social, environmental and economic terms [8,9]. Furthermore, the use of innovative, cost effective and time efficient technologies such as 3-D printing technologies, provide state of the art ways to improve the quality of new constructions by minimizing the chance of errors, using highly precise material deposition, reducing on-site construction time by operating at a constant rate and reducing the costs connected to the design and management of buildings and infrastructures, combined with reduced labor requirements, and minimal material usage [10,11,12]. Cleaning of surfaces may be disadvantageous involving the removal of the protection layer, changing the color of pigmented elements, leaving behind chemical contaminants and increasing the risk of rain penetration. Moreover, the pollutants have a major effect on building structures and interior decorative items. For example, black particles and tobacco smoke cause soiling of light-colored surfaces and sulfates/nitrates because of the acid rains enhancing the surface recession [13,14]. A solution to this is the use of photocatalytic nanomaterials. Photocatalytic titanium dioxide nanoparticles with self-cleaning properties can be used as coatings on building blocks or pavements, but also on interior decorative items such as ceiling panels, curtains and wallpapers [15,16,17,18,19].
Nanostructured titanium dioxide is one of the most popular photocatalyst with the characters of long-term photostability, non-toxicity and low-cost availability [20]. However, TiO2 due to wide band-gap (3.2 eV for anatase) exhibits high photocatalytic activity under ultraviolet illumination. There are efforts regarding the improvement of TiO2 photocatalysis by shifting the band gap energy so that it is active under visible light by doping or combining TiO2 with different non-metal or transition metal ions [21,22,23,24,25]. Also, TiO2 can be used as photocatalytic products with antimicrobial properties, or air purifier, waste water treatment cleaning and water disinfection [26,27,28,29].
Specifically, a way to enhance visible light photocatalytic performance of TiO2 nanoparticles is by combining them with conjugated polymers. These nanoparticles can be employed as an efficient photocatalyst to treat the organic pollutants and remove hazardous materials [30,31]. Moreover, doping TiO2 with Nd3+ exhibits simultaneous photocatalytic activity and NIR photoluminescent emission, suitable for applications such as environmental remediation, smart materials, and display technologies [32].
Nowadays the textile industry has become a significant contributor to water pollution, resulting in the generation of huge volumes of wastewater [33]. In the perspective of the state of the art for improved TiO2 based photocatalyst materials through various modification strategies many studies aim to apply them for the removal of contaminants of emerging concerns (CECs), dye degradation and antibacterial activity [33,34,35,36].
In this study, TiO2 dopped with a variety of dopants is being assessed both under UV and Vis light and its depollution is measured on circular 3D printed building materials as well as in fabrics.
Undoped and Mn-In, Mn-Cu, In-Ni, Mn-Ni bimetallic doped TiO2 nanostructures were synthesized using the microwave-assisted hydrothermal method. The microwave-assisted hydrothermal method has unique advantages of uniform and rapid heating in comparison with the conventional one. In addition, this method can significantly reduce the reaction time, leading to the fast crystallization with a narrow size distribution and high purity [37,38,39].
Solution (0.1 M) of Titanium (Ⅳ) oxysulfate-sulfuric acid hydrate (99.95% trace metals basis, Sigma Aldrich), was dissolved into 250 mL of distilled water and were mixed under fast stirring for 45 min. The obtained solution was transferred into four Teflon vessels and irradiated in an advanced flexible microwave synthesis platform (Milestone flexiWAVE), using the high-pressure setup at 120 ℃ for 30 min (max 1500 Watt). When the reaction was finished and cooled to room temperature, the precipitate was collected, centrifuged (1000 rpm for 2 min) and washed several times with ethanol and distilled water (Figure 1).
Mn-In, Mn-Cu, In-Ni and Mn-Ni doped TiO2 nanostructures were prepared following the same experimental procedure used to prepare undoped TiO2 by also adding into the precursor solution, 0.25 at% of indium(Ⅲ) nitrate hydrate (In(NO3)3·H2O, 99.9% Sigma-Aldrich) with nickel(Ⅱ) nitrate hexahydrate (Ni(NO3)2·6H2O, ≥97.0%, Honeywell FlukaTM), manganese(Ⅱ) nitrate tetrahydrate (Mn(NO3)2·4H2O, ≥97.0%, Honeywell FlukaTM) with indium(Ⅲ) nitrate hydrate, manganese(Ⅱ) nitrate tetrahydrate with copper(Ⅱ) nitrate trihydrate (Cu(NO3)2·3H2O, 99–104%, Honeywell FlukaTM) and manganese(Ⅱ) nitrate tetrahydrate with nickel(Ⅱ) nitrate hexahydrate, respectively.
In all cases the microwave was programmed with a first temperature ramp where the target temperature was reached after 15 min. All products were kept at room temperature overnight and totally dried using an oven at 100 ℃ for 1 h.
Their crystallographic structure was examined using a Siemens D5000, X-ray diffractometer, equipped with a Cu-Kα (λ = 0.15405 nm) monochromatic radiation source. The specific surface area of the samples was examined by nitrogen (N2) physisorption using a Micromeritics' Gemini Ⅶ 2390 Surface Area Analyzer. The samples were prior degassed at 100 ℃ for 2 h under continuous nitrogen gas flow using a Micromeritics' FlowPrep 060, in order to remove moisture.
A mix of equal amounts of recycled concrete aggregate and recycled gypsum powder, supplied by ATTICA RECYCLING CD & EW SA, was used for the 3-D printing of blocks (Figure 2a). The dimensions of each block were 5 × 5 × 1 cm and printed with a Z Corporation 3-D printer (ZPrinter 310 Plus). In order to minimize the inconsistencies between the powder samples a reference block was simultaneously printed with the test-sample. Thus, 3-D printed a pair of blocks was delivered, one block served as reference (blank) and one as the test-bed for the circular building materials layered on the top with the nanocoating. The fabric that was used is based on 98% cotton and 2% viscose and is depicted with a grey color (Figure 2b). The dimensions of each fabric sample were 7 × 7 cm. The fabric mass per unit area was 125 g per square meter.
Decolorization of applied coatings was evaluated by the use of a self-made apparatus for the decolorization of Methylene Blue (MB). Degradation of pollutant was assessed by a colorimetric method and by a Varian CARY-5000 DRA 2500 UV-VIS-NIR Spectrophotometer. The color analysis was performed using the CIELAB model.
The 3-D printed blocks were separated into five pairs of blocks, each one consisting of the nanocoated block and its reference (blank) block (Table 1). Each nanocoated block was covered with 0.1 g of a photocatalytic nanomaterial as slurry, which was left to dry. In order to evaluate the decolorization effect, the 3-D printed blocks were dip-coated with a MB solution and after exposed to UV light.
Nanocoated ID | Nanomaterial | Coating | Reference ID | Coating |
TiOP | Undoped TiO2 | 0.004 g/cm2 | Ref1 | Blank |
MnInP | Mn-In/TiO2 | Ref2 | ||
MnNiP | Mn-Ni/TiO2 | Ref3 | ||
InNiP | In-Ni/TiO2 | Ref4 | ||
MnCuP | Mn-Cu/TiO2 | Ref5 |
The exposure to UV light was held into a handmade photocatalytic apparatus (Figure 3). The apparatus consists of a wooden box, which is equipped with two Prinz UV Test lamps (4W, UV-A 366 nm) and a base for supporting the pair of blocks. The horizontal distance of the blocks with the lamps was 3 cm, the height of the base was 3.5 cm while the distance between lamps was 4 cm and between blocks 2 cm.
The decolorization of the MB dye applied to the surfaces of the blocks was monitored by the use of chromatic measurements before UV irradiation and after 1 and 2 h of exposure.
For the decolorization of applied coatings on fabric six samples were used, one for each nanomaterial and one blank as reference (Table 2). Each nanocoated fabric sample was covered with 4 wt% of a photocatalytic nanomaterial as slurry, then pressed with a roller and left to dry. The fabrics were dip-coated with a MB solution and left to dry under indirect sunlight for a day.
Sample ID | Nanomaterial | Coating |
TiOF | Undoped TiO2 | 4 wt% |
MnInF | Mn-In/TiO2 | |
MnNiF | Mn-Ni/TiO2 | |
InNiF | In-Ni/TiO2 | |
MnCuF | Mn-Cu/TiO2 |
The decolorization of the MB dye applied to the surfaces of the fabrics was monitored by the use of chromatic measurements before and after the exposure to indirect sunlight. After that the samples were exposed to UV irradiation. For this purpose, the initial photocatalytic apparatus was modified. The base was replaced by a thread and three hooks to hold each fabric sample in its place (Figure 4). The horizontal distance of the fabrics with the lamps was 4 cm, lamps and fabric samples were respectively 8 cm and 12 cm above the surface of the box, while the distance between lamps was 12 cm and between fabrics 8 cm. The decolorization of the MB dye applied to the surfaces of the fabrics was monitored by a Cary 5000 UV-VIS-NIR spectrophotometer before UV irradiation and after 1 and 2 h of exposure.
The X-ray diffraction patterns of undoped and Mn-In, Mn-Cu, In-Ni and Mn-Ni doped TiO2 nanostructures (Figure 5) only exhibited patterns assigned to the TiO2 anatase phase (JCPDS file card No.-01-071-1166). No signal from the crystalline phase containing metal or metal oxide of the doping elements could be observed indicating that the dopant ions have been successfully doped into the TiO2 lattice. Furthermore, careful analyses of the main peak (101) of the XRD patterns (Figure 5, inset) indicated a slight shift to the higher angle side for Mn-In, Mn-Cu, In-Ni and mainly for Mn-Ni doped TiO2 nanoparticles.
By comparing the ionic radius values [40,41,42,43] of Ti4+ (0.68 Å) to that of In3+ (0.81 Å), Mn2+ (0.80 Å), Ni2+ (0.72Å) and Cu2+ (0.73Å), we hypothesize that some of the doping elements were incorporated into the structures of TiO2, by replacing titanium or oxygen atoms and some occupies the interstitial position, which induced a perturbation in anatase crystal structure [44,45]. Replacement by In3+, Mn2+, Ni2+ and Cu2+ dopant ions may cause expansion/compression of the unit cell resulting to variation in various parameters including micro-strain due to lattice mismatch and distortion. The increase in lattice (micro) strain can be explained by the dopant substitution on the oxygen sites of TiO2 surface.
The lattice constants a, b and c have been calculated from the XRD patterns using the Eq 1 [46].
1d2=(h2+k2)a2+l2c2 | (1) |
where h, k, and l are the Miller indices; a and c are the lattice constants; and d is the interplanar spacing, which can be determined from Bragg's law
2⋅d⋅sinθ=n⋅λ | (2) |
The lattice parameters were calculated for undoped and Mn-In, Mn-Cu, In-Ni and Mn-Ni doped TiO2 nanostructures (Table 3). It is apparent that the lattice parameters are decreased upon addition of different types of dopant ions.
Sample | 2θ [101] (deg) | Lattice parameters | Unit cell volume (Å3) | d spacing [101] (nm) | Crystallite size (nm) | Strain (10−3) | ||
a = b (Å) | c (Å) | Scherrer | SSP | |||||
undoped TiO2 | 25.34 | 3.786 | 9.490 | 136.04 ± 0.07 | 3.512 ± 0.001 | 6.0 | 6.2 | 3.54 |
0.25 at% Mn-In | 25.35 | 3.785 | 9.489 | 135.98 ± 0.07 | 3.510 ± 0.001 | 6.0 | 6.4 | 3.75 |
0.25 at% Mn-Cu | 25.41 | 3.780 | 9.470 | 135.30 ± 0.07 | 3.502 ± 0.001 | 6.1 | 6.4 | 3.45 |
0.25 at% In-Ni | 25.41 | 3.778 | 9.466 | 135.10 ± 0.07 | 3.503 ± 0.001 | 5.9 | 6.1 | 3.11 |
0.25 at% Mn-Ni | 25.56 | 3.771 | 9.439 | 134.25 ± 0.07 | 3.482 ± 0.001 | 5.8 | 6.0 | 3.64 |
The average crystallite sizes of undoped and doped TiO2 samples were also determined (Table 3) by measuring the full width at half-maximum of the prominent peak (101) of anatase, using the Debye-Scherrer method Eq 3 [47].
D=K⋅λβhkl⋅cosθ | (3) |
where D is the average crystallite size; K is the shape factor, usually taken as 0.9; λ is the wavelength of radiation in nanometer (λCuKα = 0.15405 nm); θ is the diffracted angle of the peak; βhkl is the full width at half maximum of the peak in radians.
It is well known that the Debye-Scherrer formula provides only the lower limit of crystallite size [48]. Therefore, the structural properties and XRD peak broadening of the samples were investigated by Size-Strain Plot (SSP) method to evaluate the crystallite sizes and lattice strains [44,45,49].
Size-strain parameters can be obtained from the SSP method. This has a benefit that less importance is given to data from reflections at high angles, where the accuracy and precision are less, than that in lower angles. This is because, at higher angles, XRD data are of lower quality and peaks are generally highly overlapped at higher diffracting angles [50].
In this method, it is assumed that the crystallite size profile is described by a Lorentzian function and that the strain profile is described by a Gaussian function [50,51,52]. Hence we have,
(dhkl⋅βhkl⋅cosθ)2=K⋅λD⋅(d2hkl+βhkl⋅cosθ)+ε24 | (4) |
The Size-Strain plots of undoped and bimetallic doped TiO2 nanostructures (Figure 6) are plotted with the term (dhkl·βhkl·cosθ)2 along Y-axis against the term (dhkl2 + βhkl·cosθ) along x-axis as per Eq 4. The size and strain for each sample have been calculated from the slope and intercept of the graphs, respectively (Table 3).
The average crystallite size calculated by Size-Strain Plot method was found to increase from 6.2 nm for the undoped TiO2 to 6.4 nm for the Mn-In, Mn-Cu doped TiO2 and decrease to 6.1 and 6.0 nm for the In-Ni, Mn-Ni doped TiO2, respectively. Moreover, the lattice strain ε was found to increase with the Mn-In, Mn-Ni doping and decrease with the Mn-Cu, In-Ni doping. In this work, average crystallite sizes calculated from the Debye-Scherrer method and SSP method is found to be comparable with each other.
The photocatalytic activity of nanoparticles is highly related to their surface properties such as surface area. The selection of dopants plays a significant role on the surface area of the nanomaterial. More specifically, undoped TiO2 showed the surface area of 96.7 m2/g, the TiO2 doped with Mn-Cu and In-Ni revealed higher surface area of 98.8 and 98.4 m2/g respectively, while Mn-In and Mn-Ni displayed lower surface areas accounting to 81.5 and 94.1 m2/g respectively (Figure 7). Lower surface area may be justified by aggregation of nanostructures or because of partial pore blockages from dopants and framework defects [53]. It is well demonstrated that the nature of dopant ions is affecting the BET-specific surface area. Each curve has a point of inflection called a "knee" around P/P0 = 0.1 (Figure 7). This point indicates that monolayer adsorption is complete and multilayer formation starts to take place. Over this point the adsorbed volume was increased predominantly, which means that there are also mesopores on doped and undoped TiO2 nanostructures [54].
The decolorization of the MB dye applied to the surfaces of the 3-D printed blocks under UV exposure, was monitored by the use of chromatic measurements. For better evaluation of the initial conditions of the substrate and the effect of color differences that may occur between the analyzed surfaces before the test, the decolorization values (D) were normalized with respect to the original aspect of substrate before deposition of MB dye. The decolorization D has been calculated using Eq 5.
D(%)=ΔE(t)ΔE(0)⋅100 | (5) |
where ΔE(t) is the total color difference of chromatic coordinates at a specific time under UV irradiation with respect to the sample before the UV exposure, and ΔE(0) is the total color difference of the sample prior to UV exposure with respect to the sample before the deposition of MB dye (original block).
The decolorization of nanocoated 3-D printed blocks, after 120 min of UV irradiation, were found to be 44.5, 31.6, 34.4, 34.1 and 19.6% for sample TiOP, InNiP, MnInP, MnNiP and MnCuP, respectively (Figure 8). While the decolorization of the reference 3-D printed blocks, after 120 min of UV irradiation, were 23.8, 9.3, 16.0, 9.0 and 7.7% for sample Ref1, Re2, Ref3, Ref4 and Ref5, respectively (Figure 8). The decolorization of reference 3-D printed blocks is mainly caused by the sorption (absorption and adsorption) of MB dye onto the blocks.
It is important to compare each pair of blocks in order to distinguish the sorption processes from the decolorization of each nanomaterial applied. The net decolorization (ND = DNanocoated − DReference) of blocks caused by the nanomaterials used for the coating of the 3-D printed blocks, after 2 h of UV light exposure were found to be 20.7, 25.1, 18.1, 22.6 and 12.0% for sample TiOP, InNiP, MnInP, MnNiP and MnCuP, respectively (Table 4). The sample coated with 0.25 at% Mn-In doped TiO2 showed the highest decolorization rate of MB dye (Figure 9).
Time (min) | NDTiOP (%) | NDMnInP (%) | NDMnNiP (%) | NDInNiP (%) | NDMnCuP (%) |
60 | 18.8 | 23.2 | 14.1 | 15.1 | 6.2 |
120 | 20.7 | 25.1 | 18.1 | 22.6 | 12.0 |
Indirect sunlight exposure: For the case of indirect sunlight exposure, the decolorization percentage values of fabrics was calculated using the Eq 5. The decolorization of reference and nanocoated fabrics, after indirect sunlight exposure, are 10.8, 13.3, 52.7, 58.1, 18.6 and 47.6% for sample Ref, TiOF, InNiF, MnInF, MnNiF and MnCuF, respectively (Table 5).
Exposure | DRef (%) | DTiOF (%) | DInNiF (%) | DMnInF (%) | DMnNiF (%) | DMnCuF (%) |
Indirect sunlight | 10.8 | 13.3 | 52.7 | 58.1 | 18.6 | 47.6 |
The fabrics coated with Mn-In, In-Ni and Mn-Cu doping showed great decolorization under sunlight (Figure 10). The fabric coated with Mn-In doping showed the best decolorization of MB dye, 47.3% more than the decolorization of the reference fabric, while fabric coated with undoped TiO2 showed the smallest decolorization, 2.5% more than the decolorization of the reference fabric.
UV light exposure: The decolorization of fabrics was determined according to the Beer-Lambert law at the maximum absorbance (λmax = 670 nm) using a UV-Vis spectrophotometer (Figure 11). The decolorization percentage D, was calculated using Eq 6.
D(%)=(A0−At)A0⋅100 | (6) |
where A0 and At stand for the absorbance before UV exposure and after a specific time under UV irradiation, respectively.
The decolorization of reference and nanocoated fabrics, after 120 min of UV irradiation, are 3.9, 15.9, 18.4, 26.1, 24.0 and 20.4% for sample Ref, TiOF, InNiF, MnInF, MnNiF and MnCuF, respectively (Table 6). The fabric coated with Mn-In doping showed the best decolorization, 22.2% more than the decolorization of the reference fabric, while the fabric coated with undoped TiO2 and Mn-Ni doping showed the smallest decolorization, 12.0% and 14.5% more than the decolorization of the reference fabric, respectively (Table 6). The absorption spectra of fabrics nanocoated with Mn-Ni and Mn-Cu doping for higher wavelengths than 700 nm shows a maximum MB degradation, so we can assume that there will be no more decolorization after 120 min of UV irradiation (Figure 11).
Time (min) | DRef (%) | DTiOF (%) | DMnNiF (%) | DMnInF (%) | DInNiF (%) | DMnCuF (%) |
60 | 2.2 | 8.8 | 9.9 | 14.9 | 12.8 | 12.2 |
120 | 3.9 | 15.9 | 18.4 | 26.1 | 24.0 | 20.4 |
Doping with different non-metal or transition metal ions can alter the crystallite size, lattice stress, porosity and surface area, affecting both energy gap and the recombination rate of photogenerated electron-hole pairs [43,55]. Undoped and Mn-In, Mn-Cu, In-Ni and Mn-Ni doped TiO2 nanostructures were measured to have small crystallite size, large lattice strain and specific surface area. As a result to these characteristics, the nanocoatings showed high decolorization rate of MB dye. Mn-In doped TiO2 nanocoatings showed the highest MB decolorization for both applications. The Mn-In doping of TiO2 enhanced the MB decolorization rate in several ways. The lattice strain of Mn-In doped TiO2 was measured to be the highest of all samples, while the surface area was the lowest probably due to partial pore blockages from dopants and framework defects [53]. These characteristics can reduce the adsorption strength of the MB onto the nanocoating, increasing the probability for the reactive oxygen species (ROS) to react with the MB since the nanocoating is able to absorb light more effectively [56]. In addition, the excess surface defects created by Mn-In dopant can suppress the recombination rate of photogenerated electron-hole pairs, and then react with H2O2, OH– and O2 to generate OH and O2– radicals, which results in greater ROS generation [53].
Nanocoatings applied on the 3-D printed blocks are heavily substrate dependent [4]. Mn-Cu doped TiO2 nanocoating couldn't incorporate successfully with the 3-D printed block. This was due to the appearance of a black-green coloration on block's surface probably produced by the photochemical reaction of copper. Furthermore, a poor dispersion of the Mn-Cu doped TiO2 nanoparticles onto the block was observed. This problem can be solved with the help of any additional dispersing agent [4].
Compared to the application of 3-D printed blocks, nanocoatings showed better incorporation and dispersion onto the fabrics, resulting in the improvement of the MB decolorization rate. Doping enhanced the decolorization of MB under visible light, probably due to the reduction of the bandgap energy so that it can absorb energy from a major portion of visible light. Specifically, the fabrics coated with Mn-In, In-Ni and Mn-Cu doped TiO2 showed great MB decolorization rate under indirect sunlight.
In the present work, synthesis of undoped and Mn-In, Mn-Cu, In-Ni and Mn-Ni doped TiO2 nanostructures were conducted and a low temperature anatase phase formation was successfully achieved using a green microwave assisted technique. The structure of nanoparticles was investigated by X-ray Diffraction analysis (XRD) and the specific surface area by the BET analysis. The XRD results revealed the shift in diffraction peak positions toward higher 2θ angles and the alteration in lattice parameters between undoped and Mn-In, Mn-Cu, In-Ni and Mn-Ni doped TiO2 nanostructures, indicating TiO2 lattice compression and production of lattice strain due to formation of defects on the TiO2 surface. BET-specific area analysis revealed high surface area values for the undoped and Mn-In, Mn-Cu, In-Ni and Mn-Ni doped TiO2 nanostructures associated with the microporous nature of the structures. The decolorization of the applied nanocoatings of 3-D printed blocks and fabrics was studied. Substrates were made of a mix of equal amounts of recycled concrete aggregate and recycled gypsum powder, while the fabrics mainly of cotton. Due to the high porosity of substrates, nanoparticles showed good adhesion. The nanocoated samples showed high MB decolorization and great potential in self-cleaning applications. The highest decolorization of MB for both applications were observed for 0.25 at% Mn-In doped TiO2, probably because of the high lattice strain, which has been reported to be beneficial for photocatalytic activity with TiO2. Moreover, the results of nanocoated fabrics under exposure to indirect sunlight provide a potential breakthrough in the modern textile industry.
The authors would like to acknowledge the late professor Emmanuel Syskakis and his team, especially the PhD candidate Dimitra Manousou from the National and Kapodistrian University of Athens for their precious assistance in this work.
The authors declare that they have no known conflict for financial interests or personal relationships that could have appeared to influence the work reported in this paper.
[1] | Papadaki D, Kiriakidis G, Tsoutsos T (2018) Applications of nanotechnology in construction industry, In: Barhoum A, Abdel Salam HM, Fundamentals of Nanoparticles, Elsevier, 343-370. https://doi.org/10.1016/B978-0-323-51255-8.00011-2 |
[2] |
Boostani H, Modirrousta S (2016) Review of nanocoatings for building application. Procedia Eng 145: 1541-1548. https://doi.org/10.1016/j.proeng.2016.04.194 doi: 10.1016/j.proeng.2016.04.194
![]() |
[3] |
Jesus MAMLD, Neto JTDS, Timò G, et al. (2015) Superhydrophilic self-cleaning surfaces based on TiO2 and TiO2/SiO2 composite films for photovoltaic module cover glass. Appl Adhes Sci 3: 5. https://doi.org/10.1186/s40563-015-0034-4 doi: 10.1186/s40563-015-0034-4
![]() |
[4] |
Luna M, Delgado J, Gil M, et al. (2018) TiO2-SiO2 coatings with a low content of AuNPs for producing self-cleaning building materials. Nanomaterials 8: 177. https://doi.org/10.3390/nano8030177 doi: 10.3390/nano8030177
![]() |
[5] |
Ünlü B, Özacar M (2020) Effect of Cu and Mn amounts doped to TiO2 on the performance of DSSCs. Sol Energy 196: 448-456. https://doi.org/10.1016/j.solener.2019.12.043 doi: 10.1016/j.solener.2019.12.043
![]() |
[6] |
Papadaki D, Foteinis S, Binas V, et al. (2019) A life cycle assessment of PCM and VIP in warm Mediterranean climates and their introduction as a strategy to promote energy savings and mitigate carbon emissions. AIMS Mater Sci 6: 944-959. https://doi.org/10.3934/matersci.2019.6.944 doi: 10.3934/matersci.2019.6.944
![]() |
[7] |
Tisov A, Kuusk K, Escudero MN, et al. (2020) Driving decarbonisation of the EU building stock by enhancing a consumer centred and locally based circular renovation process. E3S Web of Conferences 172: 18006. https://doi.org/10.1051/e3sconf/202017218006 doi: 10.1051/e3sconf/202017218006
![]() |
[8] | Cheshire D (2019) Building Revolutions: Applying the Circular Economy to the Built Environment, RIBA Publishing. https://doi.org/10.4324/9780429346712 |
[9] |
Mangialardo A, Micelli E (2018) Rethinking the construction industry under the circular economy: Principles and case studies. International conference on Smart and Sustainable Planning for Cities and Regions, 333-344. https://doi.org/10.1007/978-3-319-75774-2_23 doi: 10.1007/978-3-319-75774-2_23
![]() |
[10] |
Gao W, Zhang Y, Ramanujan D, et al. (2015) The status, challenges, and future of additive manufacturing in engineering. Comput Aided Design 69: 65-89. https://doi.org/10.1016/j.cad.2015.04.001 doi: 10.1016/j.cad.2015.04.001
![]() |
[11] |
Xia M, Sanjayan J (2016) Method of formulating geopolymer for 3D printing for construction applications. Mater Design 110: 382-390. https://doi.org/10.1016/j.matdes.2016.07.136 doi: 10.1016/j.matdes.2016.07.136
![]() |
[12] |
Wangler T, Lloret E, Reiter L, et al. (2016) Digital Concrete: Opportunities and Challenges. RILEM Tech Lett 1: 67. https://doi.org/10.21809/rilemtechlett.2016.16 doi: 10.21809/rilemtechlett.2016.16
![]() |
[13] |
Mansour AMH, Al-Dawery SK (2018) Sustainable self-cleaning treatments for architectural facades in developing countries. Alex Eng J 57: 867-873. https://doi.org/10.1016/j.aej.2017.01.042 doi: 10.1016/j.aej.2017.01.042
![]() |
[14] |
Li Y (2019) Study on the weathering mechanism and protection technology of stone archives. IOP Conf Ser Mater Sci Eng 490: 062032. https://doi.org/10.1088/1757-899X/490/6/062032 doi: 10.1088/1757-899X/490/6/062032
![]() |
[15] | Janus M, Zając K (2019) Self-cleaning efficiency of nanoparticles applied on facade bricks, In: Pacheco-Torgal F, Diamanti MV, Nazari A, et al., Nanotechnology in Eco-efficient Construction: Materials, Processes and Applications, 2Eds., Elsevier, 591-618. https://doi.org/10.1016/B978-0-08-102641-0.00024-4 |
[16] | Diamanti MV, Pedeferri M (2019) Photocatalytic performance of mortars with nanoparticles exposed to the urban environment, In: Pacheco-Torgal F, Diamanti MV, Nazari A, et al., Nanotechnology in Eco-efficient Construction: Materials, Processes and Applications, 2Eds., Elsevier, 527-555. https://doi.org/10.1016/B978-0-08-102641-0.00022-0 |
[17] |
Sikora P, Horszczaruk E, Rucinska T (2015) The effect of nanosilica and titanium dioxide on the mechanical and self-cleaning properties of waste-glass cement mortar. Procedia Eng 108: 146-153. https://doi.org/10.1016/j.proeng.2015.06.130 doi: 10.1016/j.proeng.2015.06.130
![]() |
[18] |
Abbas M, Iftikhar H, Malik M, et al. (2018) Surface coatings of TiO2 nanoparticles onto the designed fabrics for enhanced self-cleaning properties. Coatings 8: 35. https://doi.org/10.3390/coatings8010035 doi: 10.3390/coatings8010035
![]() |
[19] |
Giesz P, Celichowski G, Puchowicz D, et al. (2016) Microwave-assisted TiO2: anatase formation on cotton and viscose fabric surfaces. Cellulose 23: 2143-2159. https://doi.org/10.1007/s10570-016-0916-z doi: 10.1007/s10570-016-0916-z
![]() |
[20] |
Humayun M, Raziq F, Khan A, et al. (2018) Modification strategies of TiO2 for potential applications in photocatalysis: a critical review. Green Chem Letter Rev 11: 86-102. https://doi.org/10.1080/17518253.2018.1440324 doi: 10.1080/17518253.2018.1440324
![]() |
[21] |
Nurdin M, Ramadhan LOAN, Darmawati D, et al. (2018) Synthesis of Ni, N co-doped TiO2 using microwave-assisted method for sodium lauryl sulfate degradation by photocatalyst. J Coat Technol Res 15: 395-402. https://doi.org/10.1007/s11998-017-9976-8 doi: 10.1007/s11998-017-9976-8
![]() |
[22] | Moma J, Baloyi J (2019) Modified titanium dioxide for photocatalytic applications, In: Khan SB, Akhta K, Photocatalysts: Applications and Attributes, IntechOpen. https://doi.org/10.5772/intechopen.79374 |
[23] |
Maragatha J, Rajendran S, Endo T, et al. (2017) Microwave synthesis of metal doped TiO2 for photocatalytic applications. J Mater Sci-Mater El 28: 5281-5287. https://doi.org/10.1007/s10854-016-6185-7 doi: 10.1007/s10854-016-6185-7
![]() |
[24] |
Singh I, Birajdar B (2017) Synthesis, characterization and photocatalytic activity of mesoporous Na-doped TiO2 nano-powder prepared via a solvent-controlled non-aqueous sol-gel route. RSC Adv 7: 54053-54062. https://doi.org/10.1039/C7RA10108B doi: 10.1039/C7RA10108B
![]() |
[25] |
Qi H, Lee J, Wang L, et al. (2016) Photocatalytic performance of titanium dioxide nanoparticles doped with multi-metals. J Adv Oxid Technol 19: 302-309. https://doi.org/10.1515/jaots-2016-0214 doi: 10.1515/jaots-2016-0214
![]() |
[26] |
Gnanaprakasam A, Sivakumar VM, Thirumarimurugan M (2015) Influencing parameters in the photocatalytic degradation of organic effluent via nanometal oxide catalyst: A review. Indian J Mater Sci 2015: 1-16. https://doi.org/10.1155/2015/601827 doi: 10.1155/2015/601827
![]() |
[27] |
Colangiuli D, Calia A, Bianco N (2015) Novel multifunctional coatings with photocatalytic and hydrophobic properties for the preservation of the stone building heritage. Constr Build Mater 93: 189-196. https://doi.org/10.1016/j.conbuildmat.2015.05.100 doi: 10.1016/j.conbuildmat.2015.05.100
![]() |
[28] | Matoh L, Žener B, Korošec RC, et al. (2019) Photocatalytic water treatment, In: Pacheco-Torgal F, Diamanti MV, Nazari A, et al., Nanotechnology in Eco-efficient Construction: Materials, Processes and Applications, 2Eds., Elsevier, 675-702. https://doi.org/10.1016/B978-0-08-102641-0.00027-X |
[29] | Janjua ZA (2019) Icephobic nanocoatings for infrastructure protection, In: Pacheco-Torgal F, Diamanti MV, Nazari A, et al., Nanotechnology in Eco-efficient Construction: Materials, Processes and Applications, 2Eds., Elsevier, 281-302. https://doi.org/10.1016/B978-0-08-102641-0.00013-X |
[30] |
Bai W, Tian X, Yao R, et al. (2020) Preparation of nano-TiO2@polyfluorene composite particles for the photocatalytic degradation of organic pollutants under sunlight. Sol Energy 196: 616-624. https://doi.org/10.1016/j.solener.2019.12.066 doi: 10.1016/j.solener.2019.12.066
![]() |
[31] |
Bai W, Yao R, Tian X, et al. (2018) Sunlight highly photoactive TiO2@poly-p-phenylene composite microspheres for malachite green degradation. J Taiwan Inst Chem E 87: 112-116. https://doi.org/10.1016/j.jtice.2018.03.018 doi: 10.1016/j.jtice.2018.03.018
![]() |
[32] |
Colomer MT, Roa C, Ortiz AL, et al. (2020) Influence of Nd3+ doping on the structure, thermal evolution and photoluminescence properties of nanoparticulate TiO2 xerogels. J Alloy Compd 819: 152972. https://doi.org/10.1016/j.jallcom.2019.152972 doi: 10.1016/j.jallcom.2019.152972
![]() |
[33] |
Pipil H, Yadav S, Chawla H, et al. (2022) Comparison of TiO2 catalysis and Fenton's treatment for rapid degradation of Remazol Red Dye in textile industry effluent. Rend Lincei-Sci Fis 33: 105-114. https://doi.org/10.1007/s12210-021-01040-x doi: 10.1007/s12210-021-01040-x
![]() |
[34] |
Anucha CB, Altin I, Bacaksiz E, et al. (2022) Titanium dioxide (TiO2)-based photocatalyst materials activity enhancement for contaminants of emerging concern (CECs) degradation: In the light of modification strategies. Chem Eng J Adv 10: 100262. https://doi.org/10.1016/j.ceja.2022.100262 doi: 10.1016/j.ceja.2022.100262
![]() |
[35] |
Dharma HNC, Jaafar J, Widiastuti N, et al. (2022) A review of titanium dioxide (TiO2)-based photocatalyst for oilfield-produced water treatment. Membranes 12: 345. https://doi.org/10.3390/membranes12030345 doi: 10.3390/membranes12030345
![]() |
[36] |
Shimi AK, Ahmed HM, Wahab M, et al. (2022) Synthesis and applications of green synthesized TiO2 nanoparticles for photocatalytic dye degradation and antibacterial activity. J Nanomater 2022: 1-9. https://doi.org/10.1155/2022/7060388 doi: 10.1155/2022/7060388
![]() |
[37] |
Bregadiolli BA, Fernandes SL, Graeff CFDO (2017) Easy and fast preparation of TiO2-based nanostructures using microwave assisted hydrothermal synthesis. Mater Res 20: 912-919. https://doi.org/10.1590/1980-5373-mr-2016-0684 doi: 10.1590/1980-5373-mr-2016-0684
![]() |
[38] |
Dhilip Kumar R, Andou Y, Sathish M, et al. (2016) Synthesis of nanostructured Cu-WO3 and CuWO4 for supercapacitor applications. J Mater Sci-Mater El 27: 2926-2932. https://doi.org/10.1007/s10854-015-4111-z doi: 10.1007/s10854-015-4111-z
![]() |
[39] |
Jaafar NF, Marfur NA, Jusoh NWC, et al. (2019) Synthesis of mesoporous nanoparticles via microwave-assisted method for photocatalytic degradation of phenol derivatives. Malaysian J Anal Sci 23: 462-471. https://doi.org/10.17576/mjas-2019-2303-10 doi: 10.17576/mjas-2019-2303-10
![]() |
[40] | Rohrer GS (2001) Structure and Bonding in Crystalline Materials, Cambridge University Press. https://doi.org/10.1017/CBO9780511816116 |
[41] | Pauling L (1960) The nature of the chemical bond and the structure of molecules and crystals: An introduction to modern structural chemistry, George Fisher Baker nonresident lectureship in chemistry at Cornell University, 3 Eds., Ithaca: Cornell University Press. |
[42] | Galasso FS (1970) Structure and properties of inorganic solids, In: Francis S. Galasso. Illustrated by W. Darby. Pergamon Press Oxford, New York, 1st ed edition. |
[43] |
Papadaki D, Mhlongo GH, Motaung DE, et al. (2019) Hierarchically porous Cu-, Co-, and Mn-doped platelet-like ZnO nanostructures and their photocatalytic performance for indoor air quality control. ACS Omega 4: 16429-16440. https://doi.org/10.1021/acsomega.9b02016 doi: 10.1021/acsomega.9b02016
![]() |
[44] |
Amreetha S, Dhanuskodi S, Nithya A, et al. (2016) Three way electron transfer of a C-N-S tri doped two-phase junction of TiO2 nanoparticles for efficient visible light photocatalytic dye degradation. RSC Adv 6: 7854-7863. https://doi.org/10.1039/C5RA25017J doi: 10.1039/C5RA25017J
![]() |
[45] |
Belekbir S, el Azzouzi M, el Hamidi A, et al. (2020) Improved photocatalyzed degradation of phenol, as a model pollutant, over metal-impregnated nanosized TiO2. Nanomaterials 10: 996. https://doi.org/10.3390/nano10050996 doi: 10.3390/nano10050996
![]() |
[46] |
Wilson GJ, Matijasevich AS, Mitchell DRG, et al. (2006) Modification of TiO2 for enhanced surface properties: Finite ostwald ripening by a microwave hydrothermal process. Langmuir 22: 2016-2027. https://doi.org/10.1021/la052716j doi: 10.1021/la052716j
![]() |
[47] |
El Mragui A, Logvina Y, Pinto da Silva L, et al. (2019) Synthesis of Fe- and Co-doped TiO2 with improved photocatalytic activity under visible irradiation toward carbamazepine degradation. Materials 12: 3874. https://doi.org/10.3390/ma12233874 doi: 10.3390/ma12233874
![]() |
[48] |
Irfan H, Racik KM, Anand S (2018) Microstructural evaluation of CoAl2O4 nanoparticles by Williamson-Hall and size-strain plot methods. J Asian Ceram Soc 6: 54-62. https://doi.org/10.1080/21870764.2018.1439606 doi: 10.1080/21870764.2018.1439606
![]() |
[49] |
Ghasemifard M, Abrishami ME, Iziy M (2015) Effect of different dopants Ba and Ag on the properties of SrTiO3 nanopowders. Result Phys 5: 309-313. https://doi.org/10.1016/j.rinp.2015.10.005 doi: 10.1016/j.rinp.2015.10.005
![]() |
[50] |
Nath D, Singh F, Das R (2020) X-ray diffraction analysis by Williamson-Hall, Halder-Wagner and size-strain plot methods of CdSe nanoparticles-a comparative study. Mater Chem Phys 239: 122021. https://doi.org/10.1016/j.matchemphys.2019.122021 doi: 10.1016/j.matchemphys.2019.122021
![]() |
[51] |
Dey PCh, Sarkar S, Das R (2020) X-ray diffraction study of the elastic properties of jagged spherical CdS nanocrystals. Mater Sci-Poland 38: 271-278. https://doi.org/10.2478/msp-2020-0032 doi: 10.2478/msp-2020-0032
![]() |
[52] |
Rabiei M, Palevicius A, Monshi A, et al. (2020) Comparing methods for calculating nano crystal size of natural hydroxyapatite using X-ray diffraction. Nanomaterials 10: 1627. https://doi.org/10.3390/nano10091627 doi: 10.3390/nano10091627
![]() |
[53] |
Gao X, Zhou B, Yuan R (2015) Doping a metal (Ag, Al, Mn, Ni and Zn) on TiO2 nanotubes and its effect on Rhodamine B photocatalytic oxidation. Environ Eng Res 20: 329-335. https://doi.org/10.4491/eer.2015.062 doi: 10.4491/eer.2015.062
![]() |
[54] |
Lee H-M, Kang H-R, An K-H, et al. (2013) Comparative studies of porous carbon nanofibers by various activation methods. Carbon Lett 14: 180-185. https://doi.org/10.5714/CL.2013.14.3.180 doi: 10.5714/CL.2013.14.3.180
![]() |
[55] |
Moradi H, Eshaghi A, Hosseini SR, et al. (2016) Fabrication of Fe-doped TiO2 nanoparticles and investigation of photocatalytic decolorization of reactive red 198 under visible light irradiation. Ultrason Sonochem 32: 314-319. https://doi.org/10.1016/j.ultsonch.2016.03.025 doi: 10.1016/j.ultsonch.2016.03.025
![]() |
[56] |
Iqbal A, Ibrahim NH, Rahman NRA, et al. (2020) ZnO surface doping to enhance the photocatalytic activity of lithium titanate/TiO2 for methylene blue photodegradation under visible light irradiation. Surfaces 3: 301-318. https://doi.org/10.3390/surfaces3030022 doi: 10.3390/surfaces3030022
![]() |
1. | Yang Cao, Hongte Wu, Preparation and performance analysis of polycarboxylate superplasticizer modified with fluorine-containing monomer and silicon dioxide nanoparticles, 2024, 14, 2158-5849, 605, 10.1166/mex.2024.2665 |
Nanocoated ID | Nanomaterial | Coating | Reference ID | Coating |
TiOP | Undoped TiO2 | 0.004 g/cm2 | Ref1 | Blank |
MnInP | Mn-In/TiO2 | Ref2 | ||
MnNiP | Mn-Ni/TiO2 | Ref3 | ||
InNiP | In-Ni/TiO2 | Ref4 | ||
MnCuP | Mn-Cu/TiO2 | Ref5 |
Sample ID | Nanomaterial | Coating |
TiOF | Undoped TiO2 | 4 wt% |
MnInF | Mn-In/TiO2 | |
MnNiF | Mn-Ni/TiO2 | |
InNiF | In-Ni/TiO2 | |
MnCuF | Mn-Cu/TiO2 |
Sample | 2θ [101] (deg) | Lattice parameters | Unit cell volume (Å3) | d spacing [101] (nm) | Crystallite size (nm) | Strain (10−3) | ||
a = b (Å) | c (Å) | Scherrer | SSP | |||||
undoped TiO2 | 25.34 | 3.786 | 9.490 | 136.04 ± 0.07 | 3.512 ± 0.001 | 6.0 | 6.2 | 3.54 |
0.25 at% Mn-In | 25.35 | 3.785 | 9.489 | 135.98 ± 0.07 | 3.510 ± 0.001 | 6.0 | 6.4 | 3.75 |
0.25 at% Mn-Cu | 25.41 | 3.780 | 9.470 | 135.30 ± 0.07 | 3.502 ± 0.001 | 6.1 | 6.4 | 3.45 |
0.25 at% In-Ni | 25.41 | 3.778 | 9.466 | 135.10 ± 0.07 | 3.503 ± 0.001 | 5.9 | 6.1 | 3.11 |
0.25 at% Mn-Ni | 25.56 | 3.771 | 9.439 | 134.25 ± 0.07 | 3.482 ± 0.001 | 5.8 | 6.0 | 3.64 |
Time (min) | NDTiOP (%) | NDMnInP (%) | NDMnNiP (%) | NDInNiP (%) | NDMnCuP (%) |
60 | 18.8 | 23.2 | 14.1 | 15.1 | 6.2 |
120 | 20.7 | 25.1 | 18.1 | 22.6 | 12.0 |
Exposure | DRef (%) | DTiOF (%) | DInNiF (%) | DMnInF (%) | DMnNiF (%) | DMnCuF (%) |
Indirect sunlight | 10.8 | 13.3 | 52.7 | 58.1 | 18.6 | 47.6 |
Time (min) | DRef (%) | DTiOF (%) | DMnNiF (%) | DMnInF (%) | DInNiF (%) | DMnCuF (%) |
60 | 2.2 | 8.8 | 9.9 | 14.9 | 12.8 | 12.2 |
120 | 3.9 | 15.9 | 18.4 | 26.1 | 24.0 | 20.4 |
Nanocoated ID | Nanomaterial | Coating | Reference ID | Coating |
TiOP | Undoped TiO2 | 0.004 g/cm2 | Ref1 | Blank |
MnInP | Mn-In/TiO2 | Ref2 | ||
MnNiP | Mn-Ni/TiO2 | Ref3 | ||
InNiP | In-Ni/TiO2 | Ref4 | ||
MnCuP | Mn-Cu/TiO2 | Ref5 |
Sample ID | Nanomaterial | Coating |
TiOF | Undoped TiO2 | 4 wt% |
MnInF | Mn-In/TiO2 | |
MnNiF | Mn-Ni/TiO2 | |
InNiF | In-Ni/TiO2 | |
MnCuF | Mn-Cu/TiO2 |
Sample | 2θ [101] (deg) | Lattice parameters | Unit cell volume (Å3) | d spacing [101] (nm) | Crystallite size (nm) | Strain (10−3) | ||
a = b (Å) | c (Å) | Scherrer | SSP | |||||
undoped TiO2 | 25.34 | 3.786 | 9.490 | 136.04 ± 0.07 | 3.512 ± 0.001 | 6.0 | 6.2 | 3.54 |
0.25 at% Mn-In | 25.35 | 3.785 | 9.489 | 135.98 ± 0.07 | 3.510 ± 0.001 | 6.0 | 6.4 | 3.75 |
0.25 at% Mn-Cu | 25.41 | 3.780 | 9.470 | 135.30 ± 0.07 | 3.502 ± 0.001 | 6.1 | 6.4 | 3.45 |
0.25 at% In-Ni | 25.41 | 3.778 | 9.466 | 135.10 ± 0.07 | 3.503 ± 0.001 | 5.9 | 6.1 | 3.11 |
0.25 at% Mn-Ni | 25.56 | 3.771 | 9.439 | 134.25 ± 0.07 | 3.482 ± 0.001 | 5.8 | 6.0 | 3.64 |
Time (min) | NDTiOP (%) | NDMnInP (%) | NDMnNiP (%) | NDInNiP (%) | NDMnCuP (%) |
60 | 18.8 | 23.2 | 14.1 | 15.1 | 6.2 |
120 | 20.7 | 25.1 | 18.1 | 22.6 | 12.0 |
Exposure | DRef (%) | DTiOF (%) | DInNiF (%) | DMnInF (%) | DMnNiF (%) | DMnCuF (%) |
Indirect sunlight | 10.8 | 13.3 | 52.7 | 58.1 | 18.6 | 47.6 |
Time (min) | DRef (%) | DTiOF (%) | DMnNiF (%) | DMnInF (%) | DInNiF (%) | DMnCuF (%) |
60 | 2.2 | 8.8 | 9.9 | 14.9 | 12.8 | 12.2 |
120 | 3.9 | 15.9 | 18.4 | 26.1 | 24.0 | 20.4 |