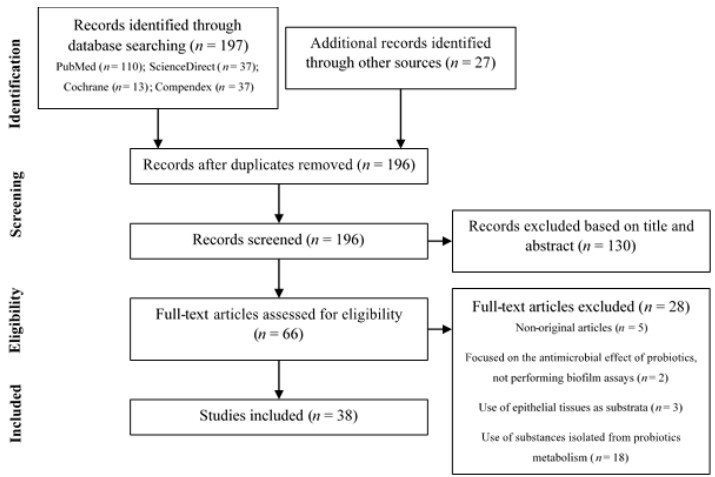
Biofilm-related infections are becoming a major clinical problem due to the increasingly widespread ability of pathogens to develop persistent biofilms in medical devices. The inadequate response of conventional antimicrobial strategies to counteract biofilm development demands urgent alternatives. An increasing interest in promoting a natural approach to health has intensified the research in the field of probiotics to battle pathogenic biofilms. This study aims to summarize the recent evidence supporting the effects of probiotic cells on the control and prevention of medical device-associated biofilms using a PRISMA-oriented (Preferred Reporting Items for Systematic reviews and Meta-Analyses) systematic search. This review demonstrated that probiotic cells have the potential to be used as biocontrol agents against biofilm formation by a broad spectrum of microorganisms. The restriction of biofilm growth caused by probiotics seemed to be strain-specific and independent of the antibiofilm strategy used (displacement, exclusion or competition). Lactobacillus, Lactococcus and Streptococcus were the most studied genus of probiotics and those with the higher capability to hinder biofilm formation, causing reductions up to 99.9%. These findings will pave the way to further experiments on the topic so that probiotic cells may become part of the clinical arsenal for the prevention and treatment of medical device-associated infections.
Citation: Fábio M. Carvalho, Rita Teixeira-Santos, Filipe J. M. Mergulhão, Luciana C. Gomes. Targeting biofilms in medical devices using probiotic cells: a systematic review[J]. AIMS Materials Science, 2021, 8(4): 501-523. doi: 10.3934/matersci.2021031
[1] | Fernanda B Haffner, Roudayna Diab, Andreea Pasc . Encapsulation of probiotics: insights into academic and industrial approaches. AIMS Materials Science, 2016, 3(1): 114-136. doi: 10.3934/matersci.2016.1.114 |
[2] | Ajitanshu Vedrtnam, Kishor Kalauni, Sunil Dubey, Aman Kumar . A comprehensive study on structure, properties, synthesis and characterization of ferrites. AIMS Materials Science, 2020, 7(6): 800-835. doi: 10.3934/matersci.2020.6.800 |
[3] | Sarah Trinschek, Karin John, Uwe Thiele . From a thin film model for passive suspensions towards the description of osmotic biofilm spreading. AIMS Materials Science, 2016, 3(3): 1138-1159. doi: 10.3934/matersci.2016.3.1138 |
[4] | Harold O. Lee III, Sam-Shajing Sun . Properties and mechanisms of iodine doped of P3HT and P3HT/PCBM composites. AIMS Materials Science, 2018, 5(3): 479-493. doi: 10.3934/matersci.2018.3.479 |
[5] | Silvia Colodrero . Conjugated polymers as functional hole selective layers in efficient metal halide perovskite solar cells. AIMS Materials Science, 2017, 4(4): 956-969. doi: 10.3934/matersci.2017.4.956 |
[6] | Noah M. Johnson, Yuriy Y. Smolin, Chris Shindler, Daniel Hagaman, Masoud Soroush, Kenneth K. S. Lau, Hai-Feng Ji . Photochromic dye-sensitized solar cells. AIMS Materials Science, 2015, 2(4): 503-509. doi: 10.3934/matersci.2015.4.503 |
[7] | Avner Neubauer, Shira Yochelis, Gur Mittelman, Ido Eisenberg, Yossi Paltiel . Simple down conversion nano-crystal coatings for enhancing Silicon-solar cells efficiency. AIMS Materials Science, 2016, 3(3): 1256-1265. doi: 10.3934/matersci.2016.3.1256 |
[8] | Takuto Eguchi, Shinya Kato, Naoki Kishi, Tetsuo Soga . Effect of thickness on photovoltaic properties of amorphous carbon/fullerene junction. AIMS Materials Science, 2022, 9(3): 446-454. doi: 10.3934/matersci.2022026 |
[9] | Christina G. Siontorou, Vasileios Th. Keramidas, Georgia-Paraskevi D. Nikoleli, Dimitrios P. Nikolelis, Stephanos Karapetis, Spyridoula Bratakou, Nikolaos Tzamtzis . Nano-enabled medical devices based on biosensing principles: technology basis and new concepts. AIMS Materials Science, 2017, 4(1): 250-266. doi: 10.3934/matersci.2017.1.250 |
[10] | Said Karim Shah, Jahangeer Khan, Irfan Ullah, Yaqoob Khan . Optimization of active-layer thickness, top electrode and annealing temperature for polymeric solar cells. AIMS Materials Science, 2017, 4(3): 789-799. doi: 10.3934/matersci.2017.3.789 |
Biofilm-related infections are becoming a major clinical problem due to the increasingly widespread ability of pathogens to develop persistent biofilms in medical devices. The inadequate response of conventional antimicrobial strategies to counteract biofilm development demands urgent alternatives. An increasing interest in promoting a natural approach to health has intensified the research in the field of probiotics to battle pathogenic biofilms. This study aims to summarize the recent evidence supporting the effects of probiotic cells on the control and prevention of medical device-associated biofilms using a PRISMA-oriented (Preferred Reporting Items for Systematic reviews and Meta-Analyses) systematic search. This review demonstrated that probiotic cells have the potential to be used as biocontrol agents against biofilm formation by a broad spectrum of microorganisms. The restriction of biofilm growth caused by probiotics seemed to be strain-specific and independent of the antibiofilm strategy used (displacement, exclusion or competition). Lactobacillus, Lactococcus and Streptococcus were the most studied genus of probiotics and those with the higher capability to hinder biofilm formation, causing reductions up to 99.9%. These findings will pave the way to further experiments on the topic so that probiotic cells may become part of the clinical arsenal for the prevention and treatment of medical device-associated infections.
Abbreviations: CFU: Colony-Forming Units; EcN: E. coli Nissle 1917; FEP: Fluoroethylenepropylene; N.A.; Not Available; A.: Actinomyces; Ag.: Aggregatibacter; B.; Bifidobacterium; C.: Candida; Ent.: Enterococcus; E.: Escherichia; F.: Fusobacterium; K.: Klebsiella; L.: Lactobacillus; Lact.: Lactococcus; Leuc.: Leuconostoc; P.: Pseudomonas; S.: Staphylococcus; Strep.; Streptococcus; V.; Veillonella
Implantable and prosthetic medical devices, such as intravascular catheters, mechanical heart valves, intrauterine devices and orthopedic inserts, are extensively used in the support and maintenance of healthcare quality in response to the growing prevalence of diseases and the aging population [1,2,3,4,5]. Despite the efforts to maintain sterility, the initial contamination of medical devices occurs by infectious agents from exogenous or endogenous sources, for instance, from the surgical staff or the skin of the patient, respectively [2,6,7,8,9], and disturbs the local host defense mechanisms [10,11]. The access of bacteria to the interior of the human body contributes to its vulnerability to microbial colonization [4,9,12]. Medical device-associated infections account for 50–70% of all healthcare-associated infections (HAIs) [3,8,13], which have a huge impact on healthcare systems by increasing the length of hospital stay, medical costs, and mortality and morbidity rates [4,14,15]. HAIs are estimated to affect approximately 4.1 million patients every year in Europe [16,17], leading to 16 million of extra stay days and approximately €7 billion of direct costs [10]. The degree of infection after the initial contamination of medical devices is affected by several factors such as the local hydrodynamic conditions, physicochemical properties of the surface, host environment (tissues and immune cells), number and species of microorganisms initially present on the device, and indwelling time [7,8]. Biofilm formation on the surface of medical devices represents one of the most threatening complications in the clinical field as it is at the root of many chronic and recurrent infections [2,3,7].
Biofilms are defined as complex communities of microorganisms embedded in a well-organized, structured and self-produced matrix of extracellular polymeric substances (EPS) that can attach to either abiotic and biotic surfaces [18,19,20,21]. This matrix is composed of exopolysaccharides, nucleic acids (extracellular DNA and RNA), proteins and lipids [2,22,23], and facilitates the intercellular communication of bacterial cells through signaling molecules (e.g. autoinducers), a phenomenon known as quorum-sensing (QS) [24,25]. The biofilm matrix acts as a barrier to protect the sessile microorganisms from hostile environmental conditions (pH, mechanical stress, chemical agents or phagocytosis) [4,10,26]. Biofilms may include Gram-positive (such as Staphylococcus aureus and Enterococcus faecalis) and Gram-negative bacteria (such as Escherichia coli and Pseudomonas aeruginosa) and yeasts (essentially Candida spp.), and rapid transit from single-species to multi-species populations [4,7,8]. The microorganisms within biofilms have an altered phenotype which makes the microbial population more resilient than their planktonic counterparts [27,28]. Since biofilms develop significantly increased resistance against antimicrobial therapy, biofilm-related infections became more difficult to eradicate [6,29].
The increasing resistance and inefficacy of antibiotics and other conventional strategies have motivated the search for new alternatives to hamper bacterial adhesion on device surfaces [30,31]. Therefore, a variety of novel emergent strategies have been designed to address this problem, which includes drug-eluting coatings, bactericidal coatings, nanostructured coatings, surface modifications, enzyme-mediated approaches, QS quenchers, phage therapy, and immunotherapy [1,9,29,30,31,32]. However, despite the improvement on the antibiofilm and antimicrobial properties of medical surfaces, there are some reservations about their clinical use: antimicrobial coatings can lose their long-term efficacy and induce microbial resistance; antimicrobial peptides may be toxic at high doses; some anti-adhesive surfaces may have low biocompatibility [30,32]. Thus, although several innovative approaches have been studied, most of them are still premature for clinical application and require a more in-depth validation.
A novel strategy proposed to address these shortcomings involves the use of probiotics as antimicrobial agents since they have demonstrated health-promoting properties and are considered safe for humans and the environment, possessing the status of Generally Regarded as Safe (GRAS) [33,34,35]. Probiotics are defined as (live microorganisms which when administered in adequate amounts confer health benefits on the host) [36] and have been considered as a reliable option to eradicate or delay the incidence of biofilm infections on medical devices [37,38]. An effective probiotic must own the following characteristics: (1) adhere to cells; (2) reduce pathogen adhesion; (3) persist, multiply and produce antimicrobial compounds; (4) be safe, noninvasive, non-carcinogenic, and non-pathogenic; (5) aggregate and form a normal balanced flora [39]. Unlike biofilms that lodge in locations of the human body such as the respiratory tract and oral cavity and cause infections, the biofilm-forming flora of the gastrointestinal tract containing probiotic strains is beneficial and can help in the treatment of bowel diseases [40,41]. High-throughput approaches including transcriptomics, metabolomics, proteomics and metagenomics have demonstrated that probiotics present very low risks for the host [38]. The ability to aggregate and form biofilms enables probiotics to resist environmental conditions, leading to the successful colonization and their longer stability on surfaces such as the intestinal mucosa, helping to exclude pathogenic bacteria by competitive inhibition or steric impediment, and having the possibility of triggering the immune response of host cells [42,43]. Lactobacillus and Bifidobacterium are the most important genera used as probiotics [44,45,46]. These bacterial groups possess the ability to withstand unfavorable conditions in the human body, regulate the action of microbes and exert other biological functions [34,47].
In this work, the currently available published data regarding the potential of probiotic cells for targeting pathogenic biofilms in medical devices by different approaches were systematically reviewed and critically discussed.
The search strategy adopted to systematically review the recent advances on the use of probiotic cells to hinder biofilm formation in medical devices followed the same patterns described by Carvalho et al. [37]. Briefly, a PRISMA-oriented systematic search [48] was conducted for articles published between 1 January 1980 and 13 January 2021 in PubMed, ScienceDirect, Cochrane Library and Compendex databases. The search combined the central keywords "Probiotic", "Biofilm", "Surface" and "Medical devices" with a wide range of terms and combinations adapted for each database. Additionally, the reference lists of all included articles and screened reviews were hand-searched for additional studies that were not identified through the electronic databases. Only articles in the English language were included.
The first screening to identify potentially relevant studies was based on the title and abstract of the selected articles. Afterward, full-text versions of the screened papers were obtained when available and assessed for inclusion according to the pre-established eligibility criteria. All studies were reviewed by one reviewer and any technical uncertainties were resolved by discussion with a second reviewer.
Peer-reviewed full-text articles meeting the following inclusion criteria were considered: (1) studies where probiotic cells are used as main agents to counteract pathogens biofilm formation; (2) the inhibition strategies include displacement, exclusion and competition. The exclusion criteria were: (1) studies focused on the antimicrobial effect of probiotic cells without performing biofilm assays and quantifying the effects on biofilm culturability, viability, biomass, thickness or other property; (2) studies where biotic surfaces, such as epithelial tissues, are used as contact surfaces for biofilm formation; (3) studies where substances isolated from probiotics metabolism are used; (4) non-original articles (including reviews or reports); and (5) unavailability of the full-text version.
For each included study, the following information was extracted and inserted in an electronic spreadsheet: antibiofilm strategy, probiotic strains, biofilm-forming pathogens, surface materials, methodologies (including culture conditions, biofilm platform, and biofilm analysis techniques), and obtained outcomes. Bibliographic details of the studies, such as first author and year of publication were also retrieved. Furthermore, the percentage of reduction of biofilm formation was collected whenever possible. If this result was not described and data was only available in graphical representations or tables, the values were estimated and compared with the control. Because of the high number of results presented in some studies, only the highest reduction value of each probiotic against each pathogen was retrieved (among all strains of the same species and the different methods used for biofilm analysis). When more than one article studied the action of the same probiotic against the same pathogen group, the range with the lower and upper limits was presented.
The search methodology retrieved a total of 197 articles through database searching and 27 articles by reference list searching (Figure 1). After the removal of duplicates, 196 articles advanced to screening. The assessment of titles and abstracts resulted in the exclusion of 130 articles for not accomplishing the inclusion requirements and 66 articles were selected for full-text examination. From these, 28 studies were excluded according to the exclusion criteria: non-original articles (n = 5), focus on the antimicrobial effect of probiotics, not performing biofilm assays (n = 2), use of epithelial tissues as substrata (n = 3), use of substances isolated from probiotics metabolism (n = 18). Therefore, 38 studies were included in this review.
All included articles were in vitro studies performed with probiotic cells. Their effectiveness on surfaces that are identical to those of medical devices, as well as on biofilms of several bacterial and fungal species frequently found in medical devices, was evaluated through at least one of the following strategies—displacement, exclusion and competition (Figure 2)—to simulate the conditions occurred in bacterial communities. The implementation of each strategy depends on the different probiotic mechanisms of interfering with the activity of pathogens. The most common and transversal to all antibiofilm strategies is the modification of the microbial flora through the synthesis and secretion of antimicrobial substances. Probiotics can modify the surrounding environment to make it unfavorable for competitors (for example, by decreasing the pH), hindering their activity and adhesion to surfaces through the release of a variety of growth-inhibiting compounds, such as organic acids (lactic, lipoteichoic, acetic, propionic and succinic acids), diacetyl, enzymes (lipase and amylase), hydrogen sulfide and peroxide, ethanol, carbon dioxide, EPS, deconjugated bile acids, biosurfactants and bacteriocins [33,34,47,49,50,51,52,53,54,55,56,57]. The production of these compounds contributes to a decrease in pathogen cell adherence (exclusion and competition strategies) and leads to their death and dispersion from the biofilms (displacement strategy). Each group of substances has several proposed mechanisms for pathogens' inhibition. For example, organic acids, in particular lactic and acetic acids, are capable of lowering the intracellular pH and disrupt the membranes of pathogens, leading to their death [45,51]. Bacteriocins may permeabilize the bacterial cell membrane, leading to pore formation and cell disruption [37,51]. The combined effect of these substances contributes to the broad spectrum of activity of probiotics.
The displacement strategy implies the contact between probiotic planktonic cells and pathogenic biofilms and focuses on the disruption of the architecture of mature pathogenic biofilms. It may be achieved through the release of antimicrobial substances, like matrix-degrading enzymes, which penetrate the extracellular matrix, interfering with biofilm integrity [30]. Other studies have indicated that probiotics may affect pathogen biofilm formation by secreting molecules that interfere with the expression of genes involved in QS signaling, virulence factors, and biofilm formation [38,41,45]. The suppression of the production of QS molecules might interrupt the QS system and trigger pathogenic biofilm dispersion.
The exclusion strategy consists of pre-coating a surface with probiotic cells to inhibit the adhesion of pathogenic planktonic cells. The main goal of this strategy is to prevent the initial adhesion of pathogens, thus avoiding biofilm development. The ability of probiotics to auto- and co-aggregate allows the formation of non-pathogenic biofilms that can prevent the colonization and biofilm growth of harmful bacteria, working as a protective barrier that reduces the availability of adhesion sites, fencing off the invasion of pathogens [33,40].
Finally, the competition strategy comprises the co-culture of probiotic planktonic cells with pathogenic planktonic cells. The mechanisms of probiotic action associated with this strategy include the competition for binding sites on the surface of medical devices with invading pathogens through the use of adhesins or specific receptors [40,47,51]. This competitive adhesion where probiotics fight for attachment prevents the colonization of harmful bacteria [47]. Probiotics may also limit the growth of pathogens by competition for limited resources [40,51]. For example, the depletion of essential nutrients such as iron might be a critical advantage over other microorganisms [40].
Table 1 summarizes the characteristics of all included studies, which were grouped according to the probiotic species. Additionally, for each probiotic species, the studies were divided into the three antibiofilm strategies previously indicated (displacement, exclusion or competition). Moreover, pathogens were grouped in Gram-positive, Gram-negative, yeasts and multispecies biofilms. Since one article can include more than one antibiofilm strategy, a total of 17 experiments were obtained for displacement, 10 for exclusion, and 24 for competition. Regarding the probiotics tested, Lactobacillus was the most used genus (30/38 studies), followed by Lactococcus and Streptococcus (6/38 studies each), E. coli Nissle 1917 (3/38 studies), and Bifidobacterium, Enterococcus and Leuconostoc (1/38 studies each). Lactobacillus acidophilus, Lactobacillus casei, Lactobacillus fermentum, Lactobacillus paracasei, Lactobacillus rhamnosus and Lactococcus lactis were the most commonly used species. The most used methodologies for biofilm quantification were colony-forming units (CFU) counts and crystal violet (CV) staining, which were employed in 26 and 10 studies, respectively. A wide variety of surface materials were tested, including polystyrene, silicone-based surfaces, hydroxyapatite, denture base resin, bovine enamel, titanium, stainless steel, glass, polyurethane and polypropylene. Furthermore, this review addresses several indwelling medical devices for the application of probiotics, such as urinary tract devices (catheters and stents) [58,59,60,61,62,63,64,65], voice prostheses [66,67,68,69], and dental prostheses [35,70,71,72,73,74,75,76,77,78,79,80,81,82,83,84,85,86,87,88].
Probiotic strains | Strategy | Biofilm-forming pathogens | Percentages of reduction | Ref. | Outcomes |
B. infantis | Displacement | Multispecies | 26% | [68] | Exposure of multispecies biofilms composed by Candida ssp. and streptococcal and staphylococcal strains to B. infantis on silicone rubber did not significantly reduce the number of yeasts in the biofilm. |
E. coli Nissle 1917 (EcN) | Competition | Gram-negative | 78%–93% | [89,90] | EcN was able to decrease the adhesion of S. aureus and S. epidermidis by 99.9%, and of E. coli and P. aeruginosa by 93% and 78%, respectively, on polypropylene after 24 h. |
Gram-positive | 99.9% | [89] | |||
Exclusion | Gram-positive | 99.9% | [58] | Pre-coating of silicone with EcN biofilms reduced the adhesion of Ent. faecalis up to approximately 2 log CFU/cm2 for 11 days. | |
Ent. faecium | Displacement | Multispecies | 16% | [68] | Exposure of multispecies biofilms composed by Candida ssp. and streptococcal and staphylococcal strains to Ent. faecium on silicone rubber did not significantly reduce the number of yeasts in the biofilm. |
L. acidophilus | Competition | Gram-positive | 11%–99% | [62,75,87] | L. acidophilus reduced the adhesion of S. aureus to silicone up to 99% after 1 h and of Strep. mutans in 33% and 61% after 24 and 48 h, respectively. C. albicans adhesion to polystyrene and silicone was reduced by about 47% and 98%, respectively, while C. tropicalis adhesion to silicone was reduced by 72% after 8 days. The adhesion of a streptococcal multispecies biofilm to polystyrene was reduced by 63% after 48 h. |
Yeast | 47%–98% | [69,91] | |||
Multispecies | 63% | [75] | |||
L. acidophilus | Displacement | Gram-negative | 90% | [76] | L. acidophilus was able to displace pre-formed biofilms of Ag. actinomycetemcomitans from polystyrene in 90% after 24 h and of S. aureus from silicone up to 98% after 1 h. C. albicans biofilms formed on polystyrene were removed in 57.5% and 59% after 24 and 48 h of contact. |
Gram-positive | 79%–98% | [62] | |||
Yeast | 57.5%–59% | [77,92] | |||
Exclusion | Gram-negative | 99.9% | [64] | Pre-coating silicone with L. acidophilus biofilms inhibited K. pneumoniae and S. aureus biofilm formation up to 99.9% after 7 days. The total biofilm mass of Strep. mutans and non-mutans streptococci was reduced by 43% and 9%, respectively, after 24 h. | |
Gram-positive | 9%–99.9% | [62,64,87] | |||
L. casei | Competition | Gram-positive | 64%–99% | [62,72,75,80] | L. casei inhibited the onset of biofilms of S. aureus on silicone up to 99% after 1 h and of Strep. mutans on hydroxyapatite (75%) and polystyrene (64%) after 24 and 48 h, respectively. C. albicans adherence to silicone was reduced by 95% after 8 days. The adhesion of multispecies biofilms to hydroxyapatite, polystyrene and silicone was reduced by 52, 72 and 99.9%, respectively. |
Yeast | 95% | [69] | |||
Multispecies | 52%–99.9% | [61,75,80] | |||
Displacement | Gram-negative | 90% | [76] | L. casei displaced up to 98% of S. aureus biofilms formed on silicone after 1 h, and 54% of Ent. faecalis on glass and 31% on FEP after 3 h. Pre-formed biofilms of Ag. actinomycetemcomitans and C. albicans in polystyrene were disrupted in 90% and 56.3% after 24 h. C. albicans biofilm was reduced by 99.9% in a denture base resin for 12 h. A multispecies biofilm was removed from silicone in 61% after 12 days. | |
Gram-positive | 31%–98% | [62,63] | |||
Yeast | 56.3%–99.9% | [81,92] | |||
Multispecies | 61% | [68] | |||
Exclusion | Gram-positive | 70%–99% | [62] | The adhesion of S. aureus to silicone and of C. albicans to denture base resin was reduced up to 99.9% after 1 and 12 h, respectively. | |
Yeast | 99.9% | [81] | |||
L. crispatus | Competition | Yeast | 72%–99% | [69] | L. crispatus decreased the number of biofilm culturable cells of C. albicans and C. tropicalis on silicone rubber by 99% and 72%, respectively, after 8 days. |
L. delbrueckii | Displacement | Gram-negative | 92% | [76] | L. delbrueckii significantly degraded the pre-formed biofilm of Ag. actinomycetemcomitans on polystyrene after 24 h of contact. |
L. fermentum | Competition | Gram-negative | 12%–99% | [59,93] | L. fermentum inhibited P. aeruginosa (12%) and K. pneumoniae (99%) biofilm formation in polystyrene for 7 and 24 h, respectively. The adhesion of S. aureus and mixed cultures to silicone was inhibited up to 99.9% for 1 h and 8 days, respectively. |
Gram-positive | 84%–99% | [62,72] | |||
Multispecies | 77%–99.9% | [61] | |||
Displacement | Gram-negative | 93% | [76] | L. fermentum led to a reduction of pre-formed biofilms of Ag. actinomycetemcomitans of 93% and C. albicans of 68% after 24 and 48 h, respectively. S. aureus and multispecies biofilms were removed from silicone up to 98% and by 33%, respectively, after 12 days of incubation. | |
Gram-positive | 79%–98% | [62] | |||
Yeast | 68% | [79] | |||
Multispecies | 33% | [68] | |||
Exclusion | Gram-positive | 70%–99% | [62] | Silicone pre-coated with L. fermentum reduced S. aureus adhesion up to 99% during 1 h of incubation. | |
Lact. lactis | Competition | Gram-negative | 30%–56% | [83] | Lact. lactis caused a significant decrease in the adhesion of five oral species (A. naeslundii, F. nucleatum, Strep. oralis, Strep. sobrinus and V. dispar) to hydroxyapatite (up to 74% after 40.5 h). Multispecies biofilm formation on polystyrene and hydroxyapatite was inhibited up to 66%. |
Gram-positive | 25%–74% | [83] | |||
Multispecies | 29%–66% | [35,83] | |||
Displacement | Gram-negative | 73% | [76] | Lact. lactis disrupted the pre-formed biofilms of Ag. actinomycetemcomitans in 73% after 24 h of treatment. Additionally, multispecies biofilms were removed from polystyrene (39%) and silicone (up to 96%) after 24 h and 12 days, respectively. | |
Multispecies | 7%–96% | [35,67,68] | |||
Lact. lactis | Exclusion | Gram-negative | 56%–99.9% | [65,83] | Pre-coating hydroxyapatite with Lact. lactis reduced the adhesion of five oral species (A. naeslundii, F. nucleatum, Strep. oralis, Strep. sobrinus and V. dispar) and multispecies biofilm formation up to 85% and 77%, respectively, after 40.5 h. Also, Lact. lactis inhibited the adhesion of E. coli to polystyrene after 24 and 48 h in about 3 and 2 log, respectively. |
Gram-positive | 35%–85% | [83] | |||
Multispecies | 62%–77% | [83] | |||
Multispecies (combinations of L. plantarum, L. helveticus and S. salivarius) | Competition | Yeast | 62%–72% | [84] | The combinations of probiotic species were able to significantly reduce the adherence of C. albicans to polyurethane discs. |
Displacement | Yeast | 63%–80% | [84] | The combinations of probiotic species reduced C. albicans biofilm size by more than 63% after 1 h. | |
Strep. salivarius | Competition | Gram-positive | 74%–87% | [88] | Strep. salivarius was able to interfere with the biofilm formation of Strep. intermedius in a titanium surface during 8 and 20 h of exposure. |
Strep. thermophilus | Competition | Gram-negative | 26% | [83] | Strep. thermophilus caused a decrease in the adhesion of four oral species (A. naeslundii, Strep. oralis, Strep. sobrinus and V. dispar) and multispecies biofilm formation on hydroxyapatite up to 65% and 55%, respectively. |
Gram-positive | 8%–65% | [83] | |||
Multispecies | 23%–55% | [83] | |||
Displacement | Multispecies | 67% | [68] | Strep. thermophilus significantly reduced the prevalence of yeasts in multispecies biofilms formed on silicone during 12 days. | |
Exclusion | Gram-negative | 30% | [83] | Pre-coating hydroxyapatite with Strep. thermophilus reduced the adhesion of four oral species (A. naeslundii, Strep. oralis, Strep. sobrinus and V. dispar) and multispecies biofilm formation up to 50%. | |
Gram-positive | 8%–50% | [83] | |||
Yeast | N.A. | [66] | |||
Multispecies | 26%–49% | [83] | |||
Strep. hyointestinalis | Displacement | Gram-positive | 74%–77% | [63] | Ent. faecalis was displaced by Strep. hyointestinalis from FEP and glass in 74% and 77%, respectively, after 3 h. |
Biofilm-related infections are a critical problem in the medical field due to the high susceptibility of devices to microbial colonization [4]. Previous studies have proposed the use of probiotics as an attractive approach to control biofilm formation and inhibit microbial adhesion to several materials [95,96,97]. In this regard, this review intends to describe and discuss the current findings on the anti-adhesive and antibiofilm activities of probiotic cells against medical device-associated infections. This work succeeds a systematic review and meta-analysis about different strategies using probiotics and their metabolites to curb biofilm formation in medical devices [37]. Since this initial study revealed that probiotics cells can be used to hinder biofilm growth by a broad spectrum of microorganisms, we are now addressing the use of probiotic cells as a potentially effective weapon against biofilm establishment on biomedical devices, highlighting the beneficial effects of each strain against different pathogens.
Results show that the effectiveness of probiotics cells is strain-specific and each strain can have multiple effects on the pathogenic biofilms through different mechanisms, as aforementioned. So far, few studies have investigated the antibiofilm activity of probiotics from the Bifidobacterium, Enterococcus, E. coli, Leuconostoc and Streptococcus genus. Regarding the first two, van der Mei et al. [68] demonstrated that both Bifidobacterium infantis and Enterococcus faecium did not significantly reduce the number of Candida spp. cells in oropharyngeal multispecies biofilms pre-formed on silicone rubber voice prostheses under flow conditions (Table 1). The lack of studies on these probiotics does not allow us to draw more conclusions about their potential use in medical devices.
E. coli strain Nissle 1917 (EcN) is a good biofilm former probiotic described to have beneficial effects on the treatment of some digestive disorders due to its excellent ability to colonize the human intestine and exclude pathogens [58,90]. Only 3 in vitro studies reported the use of this probiotic strain in medical devices, through the competition [89,90] and exclusion strategies [58]. In respect of competition strategy, EcN exhibited a slightly lower reduction of biofilm culturable cells for Gram-negative (78%–93%; E. coli and P. aeruginosa) than for Gram-positive bacteria (99.9%; S. aureus and Staphylococcus epidermidis) (Table 1) [89]. Fang et al. [89] indicated that the secretion of the periplasmic protein DegP by EcN cells to the extracellular space is probably critical to inhibit other biofilms. In the exclusion strategy, Chen et al. [58] used silicone surfaces modified with several mannoside derivatives in order to strengthen the link of EcN biofilms to the surface, since EcN expresses numerous adhesins, such as type 1 fimbriae, that greatly promote biofilm formation, particularly on mannoside surfaces. The authors showed that pre-formed EcN biofilms largely reduced colonization by Ent. faecalis, and strong binding between the EcN biofilms and the biphenyl-mannoside modified silicone was observed (which could withstand shear stresses similar to those generated by urine flow) [58].
The great majority of the studies have reported the effectiveness of Lactobacillus spp. in antagonizing a broad spectrum of microbial biofilms on different abiotic surfaces (Table 1). Overall, it was noted that the action of probiotic cells was independent of the strategy adopted (displacement, exclusion or competition) and varied according to the strain. Indeed, when the same studies compared the effect of Lactobacillus strains using different strategies, similar reductions were observed [62,81,82,84,87,94]. Independently of the strategy used, the pathogens more affected by Lactobacillus spp. were Aggregatibacter actinomycetemcomitans, E. coli, Klebsiella pneumoniae and S. aureus, with reductions higher than 60%. Within each strategy, the groups of pathogens in which lactobacilli exerted higher inhibitory activity were multispecies biofilms for competition, and Gram-negative bacteria and yeasts for displacement and exclusion. Regardless of the strategy used, the probiotic strains that seem to be more promising against the different groups of pathogens were: L. acidophilus, L. casei, and L. rhamnosus for Gram-negative bacteria; L. fermentum and L. paracasei for Gram-positive bacteria; and L. casei and L. rhamnosus for yeasts. The mechanisms by which Lactobacillus strains exert their harmful effects against pathogens are diverse. Song et al. [94] demonstrated that microcapsules containing L. rhamnosus cells disrupted the architecture of E. coli biofilms by decreasing the transcriptional activity of numerous virulence-related genes (luxS, lsrK, and lsrR) that are involved in E. coli QS regulation [94]. Also, Lee et al. [75] and Wu et al. [86] demonstrated that L. rhamnosus and L. salivarius significantly decreased the expression of glucosyltransferase-encoding genes (gtfB, gtfC, and gtfD) of Strep. mutans, that are responsible for the biosynthesis of glucans which play key roles in biofilm formation. Moreover, Matsubara et al. [92] and Rossoni et al. [79] attributed the disruption of C. albicans biofilms by L. acidophilus, L. casei, L. fermentum, L. paracasei and L. rhamnosus to the downregulation of genes involved in biofilm development, DNA replication, translation, glycolysis, and gluconeogenesis. Matsubara et al. [92] also suggested that Lactobacillus strains negatively impacted yeast-to-hyphae differentiation and biofilm formation by cell-cell interactions and production of autoinducers responsible for regulating the production of antimicrobial peptides, such as bacteriocins. This was confirmed by a study developed by Song et al. [81], which reported that the antifungal activity of L. casei and L. rhamnosus is associated with the production of lactic acid that diffuses through the membrane and reduces the cytoplasmic pH, thereby causing loss of viability, and with the production of antifungal peptides that may disrupt the C. albicans membrane or inactivate cytoplasm molecules. The hindrance of pathogenic biofilm formation through the production of antimicrobial agents by lactobacilli was evidenced by several studies, where bacteriocins, biosurfactants, hydrogen peroxide, and lactic acid were the most reported substances [59,71,86]. Ciandrini et al. [82] assessed the interference of L. rhamnosus and L. paracasei on Strep. mutans and Strep. oralis biofilm development by two different strategies and suggested that different mechanisms are involved in each strategy. In displacement, the remarkable biofilm inhibition (99.9%) was probably due to the co-aggregation of Lactobacillus spp. with the streptococci and the production of antimicrobial compounds. However, in competition, it is likely that Lactobacillus spp. links to specific salivary receptors necessary for streptococci. This last mechanism was also proposed by Tahmourespour et al. [87], which suggested that the reduction in the adhesion of streptococci is due to bacterial interactions and pre-colonization of adhesion sites by L. acidophilus. Kıvanç et al. [91] observed the same exclusion activity of L. acidophilus and L. paracasei against Candida spp. biofilms. Finally, Ifeoma et al. [64] immobilized L. acidophilus on urinary catheter samples using sodium alginate and showed its potential to occupy the binding sites for pathogens and produce antibacterial substances.
Concerning the Lactococcus genus, Lact. lactis was the only probiotic tested. Within each strategy, no significant differences were observed between the different groups of pathogenic organisms (Table 1). However, pre-coating the surfaces with Lact. lactis was more effective at inhibiting biofilm proliferation than the other two strategies when comparing the same set of pathogens; for example, the reduction of Gram-negative bacteria and multispecies biofilms using the exclusion strategy was higher than in competition and displacement strategies (Table 1). Comelli et al. [83] investigated the effect of Lact. lactis on the composition of multispecies biofilms and showed that the pre-incubation of hydroxyapatite with this probiotic strain caused a slightly higher decrease in biofilm cell counts than co-incubation. The highest reduction value was reached when a recombinant Lact. lactis strain expressing FimH virulence factor on the cell surface was used against a uropathogenic strain of E. coli (99.9% reduction after 24 and 48 h of exposure) [65]. FimH promotes auto-aggregation and biofilm formation of Lact. lactis, preventing E. coli colonization [65]. Also, Radaic et al. [35] suggested that nisin, a bacteriocin produced by Lact. lactis, plays a critical role in inhibiting the initial biofilm structure and cell viability during biofilm growth. Additionally, van der Mei et al. [67,68] examined the influence of several probiotic bacteria on oropharyngeal biofilms formed on silicone rubber voice prostheses, and in both experiments, Lact. lactis had a higher effect on the number of adhering Candida spp. cells when compared with probiotics from Enterococcus, Lactobacillus and Streptococcus genus. The biosurfactant production was one of the mechanisms proposed to inhibit biofilm development [67]. In turn, Jaffar et al. [76] revealed that Lact. lactis presented lower activity against biofilms formed by periodontal pathogen Ag. actinomycetemcomitans when compared to other Lactobacillus strains. The authors suggest that direct cell contact of aggregated probiotic cells was the physical factor that contributed to biofilm degradation [76].
Regarding Leuconostoc strains, only 1 study [76] evaluated the antibiofilm activity of Leuconostoc fructosum and Leuconostoc mesenteroides (Table 1). Both probiotic strains reduced by 90% the amount of biofilm of Ag. actinomycetemcomitans on polystyrene [76]. However, their future application in the medical context needs more research.
Finally, regarding Streptococcus strains, only three species of this genus were studied: Streptococcus salivarius, Streptococcus thermophilus and Streptococcus hyointestinalis. In general, regardless of pathogenic organisms, the minimal reduction observed for the displacement strategy was substantially higher (67%) than that found for the other two strategies (8%), suggesting that probiotics from the Streptococcus genus may accomplish better results by displacing pre-established biofilms than by preventing the initial attachment of pathogens. This was achieved with Strep. thermophilus, which significantly reduced the prevalence of yeasts in a multispecies biofilm [68], and Strep. hyointestinalis, which displaced Ent. faecalis from glass and fluoroethylenepropylene (77% and 74%, respectively) under flow conditions that mimic the shear rate found in the inner surface of a urinary catheter [63]. The authors speculate that the mechanisms of displacement may involve the production of biosurfactants [63]. Also, Busscher et al. [66] attributed the reduction of both C. albicans and C. tropicalis adhesion to biosurfactant release by Strep. thermophilus. The highest reduction obtained with Streptococcus was observed for Strep. salivarius against Strep. intermedius (87%), where a considerable inhibition of Strep. intermedius luxS gene expression was verified (probably as a result of bacteriocin production) when co-cultured with the probiotic in a dynamic in vitro system simulating a dental implant [88]. The inhibitory activity of Streptococcus strains was also assessed in combination with Lactobacillus strains [84]. Multispecies biofilms composed of Strep. salivarius, Lactobacillus plantarum and Lactobacillus helveticus inhibited the formation of C. albicans biofilms and removed pre-formed biofilms of the same yeast (up to 72% and 80%, respectively) [84].
The characteristics of the studies varied considerably among the articles included in this review. The dissimilarities on the percentages of reduction occasionally verified between studies using the same probiotic and pathogenic species can be associated with many factors: (1) culture medium composition, which has a significant impact on the quantity and diversity of metabolites produced; for example, the concentration of glucose may influence the extent of lactic and acetic acid produced by lactobacilli, affecting their inhibitory activity [72,93]; (2) duration of exposure of pathogens to probiotics (ranged from 1 h to 12 d); (3) duration of biofilm formation by probiotics and pathogens in exclusion and displacement strategies, respectively (ranged from few hours to days, and experiments were performed with biofilms in different growth stages); (4) substratum for biofilm formation, since the initial adhesion of microorganisms is highly influenced by the physicochemical properties of the surface material [37]; (5) concentration of probiotic and pathogenic cells used for biofilm inoculation; (6) hydrodynamic conditions and associated shear forces, that may affect the biofilm amount and structure [98,99]; and (7) methods for biofilm analysis (CFU counts, CV staining, image analysis, real-time PCR, or other), which measure different variables [24,98]. Some researchers tried to increase the predictive value of their studies by using similar conditions, like hydrodynamic, nutritional and temporal conditions, to those found in vivo. For example, van der Mei et al. [69] showed that L. acidophilus, L. casei and Lactobacillus crispatus decreased the biofilm culturable cells of C. albicans and C. tropicalis on silicone rubber during 8 days of feast and famine cycles to mimic the pattern of eating and drinking of a patient, demonstrating their potential against voice prostheses biofilms. In fact, increase the predictive value of in vitro studies is essential to translate their findings into clinical applications.
As aforementioned, exclusion is one of the mechanisms displayed by probiotics to prevent the adhesion of pathogenic strains to surfaces. The most frequent approach employed for covering a surface material with probiotics is through biofilm formation. However, one of the drawbacks of its application in biomedical devices is the easy biofilm detachment over time [100]. Thus, some studies have been making advances towards promoting the adherence and immobilization of probiotic cells to the surface of medical devices, in particular to urinary catheters [100,101,102]. Several strategies were designed based on the ability of probiotics and other non-pathogenic bacteria (like the E. coli 83972 strain) to interact with mannose residues present on the surface of epithelial cells of the intestine [100,101,102]. Some species of probiotics and non-pathogenic bacteria express mannose-specific adhesin proteins or type I fimbriae on the surface of the membrane, which have a high-affinity binding to mannose-presenting surfaces [103,104]. Therefore, the competitive exclusion properties of probiotics associated with their specific adhesion to mannoside derivatives led several authors to address this problem by modifying the silicone catheter surfaces with mannoside ligands, achieving multivalent sites for the adhesion of benign bacteria [58,100,101]. This method of silicone functionalization proved to enhance the attachment and promote faster and stronger probiotic biofilms, which maintained their stability and led to a reduction in the adherence of uropathogenic bacteria when challenged over long periods [58,100,101,102].
Although probiotics are available in a wide variety of formulations, mainly for the treatment of gastrointestinal diseases, there are a few concerns regarding the safety of using live probiotics in clinical practice, particularly in pediatric populations and patients with underlying diseases [41]. For the specific application in medical settings, the main problems could be the risk of biofilm translocation from the application site to the bloodstream, thereby causing bacteremia or sepsis, and the transmission/acquisition of antibiotic resistance genes [105,106]. Thus, the safety aspects of probiotic strains (blood hemolytic activity and resistance to antibiotics) should be evaluated before their translation to the clinical environment. In order to ward off these possibilities, the use of some purified metabolites and the inactivation of probiotic living cells (by heat, chemicals, radiation or sonication) are gaining interest [106]. For example, Tan et al. [105] developed a probiotic-modified implant to prevent methicillin-resistant S. aureus infections and accelerate bone integration by the inactivation of L. casei on the surface of titanium substrates using ultraviolet irradiation. The inactivated probiotic biofilm showed 99.98% antibacterial effectiveness and improved the osseointegration of the implant [105]. Thus, inactivated probiotics can be a potentially safe strategy in the future of medical devices. Although the use of probiotics has some limitations, its benefits largely outweigh the risks (Table 2).
Advantages | Disadvantages |
Generally Regarded as Safe (GRAS) status Withstand unfavorable conditions of the human body Survive, maintain the metabolic activity and grow in the target site Antimicrobial activity Maintain high viability at processing, lyophilization, and storage High biomass amount and productivity of cultures Positive effect on several diseases and illness conditions Increase the efficiency of the immunological system Lower cytotoxicity than conventional antimicrobial agents Wide range of mechanisms of action Both broad and narrow spectrum of action Genetically stable and good in vitro and in vivo growth properties Able to adhere to biotic and abiotic surfaces Natural origin Biodegradable |
Risk of biofilm translocation from the application site to the bloodstream Risk of transmission/acquisition of antibiotic resistance genes Risk of infection and/or morbidity in vulnerable groups Heterogeneous activity (i.e., the same strain may have different behavior under different conditions) Biofilm detachment overtime |
This systematic review showed that probiotic cells are a promising alternative to prevent and control the onset of biofilms of a wide range of pathogenic microorganisms. The relation between the performance of probiotics and the antibiofilm strategy used revealed to be strain-specific since some probiotic strains appear to be better at preventing the initial attachment of pathogens, while others are better at displacing pre-formed biofilms. Considering the percentages of reduction and the number of studies using each probiotic, E. coli Nissle 1917, L. acidophilus, L. casei, L. fermentum, L. paracasei, L. rhamnosus and Lact. lactis seem to be ahead for a possible clinical application since they were responsible for higher biofilm reductions when compared to other probiotic strains addressed in this work. Moreover, coating the surfaces with probiotics to avoid the initial attachment of pathogens seems to be more reliable than removing already established biofilms, as a way to prevent possible irreversible damages on patients. Although it has been shown that a certain degree of protection can be obtained mainly for short time periods, we believe that the future of probiotic cells in fighting device-related infections lies in finding solutions to increase the stability of probiotic biofilms on surfaces and the safety of using live probiotic cells in clinical practice.
This work was financially supported by: national funds of the Portuguese Foundation for Science and Technology (FCT) and Direção Geral do Ensino Superior (DGES) budgets in the scope of the special support "Verão com Ciência"; Base and Programmatic Funding [UIDB/00511/2020] of the Laboratory for Process Engineering, Environment, Biotechnology and Energy-LEPABE-funded by national funds through the FCT/MCTES (PIDDAC) and project PTDC/CTMCOM/4844/2020. The research was also supported by the SurfSAFE project funded by the European Union's Horizon 2020 research and innovation programme under grant agreement No 952471. R.T.-S. acknowledges the receipt of a junior researcher fellowship from the Project PTDC/BII-BIO/29589/2017-POCI-01-0145-FEDER-029589—funded by FEDER funds through COMPETE2020-Programa Operacional Competitividade e Internacionalização (POCI) and by national funds (PIDDAC) through FCT/MCTES. L.C.G. thanks the FCT for financial support of her work contract through the Scientific Employment Stimulus-Individual Call-[CEECIND/01700/2017].
All authors declare no conflicts of interest in this paper.
[1] | Srivastava A, Chandra N, Kumar S (2019) The role of biofilms in medical devices and implants, Biofilms in Human Diseases: Treatment and Control, Springer, Cham, 151-165. |
[2] |
Sun F, Qu F, Ling Y, et al. (2013) Biofilm-associated infections: Antibiotic resistance and novel therapeutic strategies. Future Microbiol 8: 877-886. doi: 10.2217/fmb.13.58
![]() |
[3] |
Khatoon Z, McTiernan CD, Suuronen EJ, et al. (2018) Bacterial biofilm formation on implantable devices and approaches to its treatment and prevention. Heliyon 4: e01067. doi: 10.1016/j.heliyon.2018.e01067
![]() |
[4] |
Percival SL, Suleman L, Vuotto C, et al. (2015) Healthcare-associated infections, medical devices and biofilms: Risk, tolerance and control. J Med Microbiol 64: 323-334. doi: 10.1099/jmm.0.000032
![]() |
[5] |
Van Epps JS, Younger JG (2016) Implantable device-related infection. Shock 46: 597-608. doi: 10.1097/SHK.0000000000000692
![]() |
[6] | Tunney MM, Gorman SP, Patrick S (2002) Infection associated with medical devices. Int J Gen Syst 31: 195-205. |
[7] |
Donlan RM (2001) Biofilms and device-associated infections. Emerg Infect Dis 7: 277-281. doi: 10.3201/eid0702.010226
![]() |
[8] |
Vertes A, Hitchins V, Phillips KS (2012) Analytical challenges of microbial biofilms on medical devices. Anal Chem 84: 3858-3866. doi: 10.1021/ac2029997
![]() |
[9] |
Veerachamy S, Yarlagadda T, Manivasagam G, et al. (2014) Bacterial adherence and biofilm formation on medical implants: A review. P I Mech Eng H 228: 1083-1099. doi: 10.1177/0954411914556137
![]() |
[10] |
Ramstedt M, Ribeiro IAC, Bujdakova H, et al. (2019) Evaluating efficacy of antimicrobial and antifouling materials for urinary tract medical devices: Challenges and recommendations. Macromol Biosci 19: e1800384. doi: 10.1002/mabi.201800384
![]() |
[11] |
Reid G (1999) Biofilms in infectious disease and on medical devices. Int J Antimicrob Ag 11: 223-226. doi: 10.1016/S0924-8579(99)00020-5
![]() |
[12] |
Bryers JD (2008) Medical biofilms. Biotechnol Bioeng 100: 1-18. doi: 10.1002/bit.21838
![]() |
[13] |
Darouiche RO (2004) Treatment of infections associated with surgical implants. N Engl J Med 350: 1422-1429. doi: 10.1056/NEJMra035415
![]() |
[14] |
Darouiche RO (2001) Device-associated infections: A macroproblem that starts with microadherence. Clin Infect Dis 33: 1567-1572. doi: 10.1086/323130
![]() |
[15] |
Campoccia D, Montanaro L, Arciola CR (2006) The significance of infection related to orthopedic devices and issues of antibiotic resistance. Biomaterials 27: 2331-2339. doi: 10.1016/j.biomaterials.2005.11.044
![]() |
[16] |
Azevedo AS, Almeida C, Melo LF, et al. (2017) Impact of polymicrobial biofilms in catheter-associated urinary tract infections. Crit Rev Microbiol 43: 423-439. doi: 10.1080/1040841X.2016.1240656
![]() |
[17] | World Health Organization, Report on the Burden of Endemic Health Care-Associated Infection Worldwide, 2011. Available from: https://apps.who.int/iris/bitstream/handle/10665/80135/9789241501507_eng.pdf;jsessionid=C2A2CD7A9910C3B4AC1FC3B18F1DBD11?sequence=1. |
[18] |
Feneley RCL, Hopley IB, Wells PNT (2015) Urinary catheters: History, current status, adverse events and research agenda. J Med Eng Technol 39: 459-470. doi: 10.3109/03091902.2015.1085600
![]() |
[19] |
Flemming HC, Wingender J (2010) The biofilm matrix. Nat Rev Microbiol 8: 623-633. doi: 10.1038/nrmicro2415
![]() |
[20] |
Rabin N, Zheng Y, Opoku-Temeng C, et al. (2015) Biofilm formation mechanisms and targets for developing antibiofilm agents. Future Med Chem 7: 493-512. doi: 10.4155/fmc.15.6
![]() |
[21] |
Deva AK, Adams WP, Vickery K (2013) The role of bacterial biofilms in device-associated infection. Plast Reconstr Surg 132: 1319-1328. doi: 10.1097/PRS.0b013e3182a3c105
![]() |
[22] |
Garrett TR, Bhakoo M, Zhang Z (2008) Bacterial adhesion and biofilms on surfaces. Prog Nat Sci 18: 1049-1056. doi: 10.1016/j.pnsc.2008.04.001
![]() |
[23] |
Lindsay D, von Holy A (2006) Bacterial biofilms within the clinical setting: What healthcare professionals should know. J Hosp Infect 64: 313-325. doi: 10.1016/j.jhin.2006.06.028
![]() |
[24] |
Corte L, Pierantoni DC, Tascini C, et al. (2019) Biofilm specific activity: A measure to quantify microbial biofilm. Microorganisms 7: 73. doi: 10.3390/microorganisms7030073
![]() |
[25] | Marić S, Vraneš J (2007) Characteristics and significance of microbial biofilm formation. Period Biol 109: 115-121. |
[26] |
Donlan RM (2002) Biofilms: Microbial life on surfaces. Emerg Infect Dis 8: 881-890. doi: 10.3201/eid0809.020063
![]() |
[27] | Vasudevan R (2014) Biofilms: Microbial cities of scientific significance. J Microbiol Exp 1: 84-98. |
[28] | Floyd KA, Eberly AR, Hadjifrangiskou M (2017) Adhesion of bacteria to surfaces and biofilm formation on medical devices, Biofilms and Implantable Medical Devices: Infection and Control, Woodhead Publishing, 47-95. |
[29] |
Chen M, Yu Q, Sun H (2013) Novel strategies for the prevention and treatment of biofilm related infections. Int J Mol Sci 14: 18488-18501. doi: 10.3390/ijms140918488
![]() |
[30] |
Zhu Z, Wang Z, Li S, et al. (2019) Antimicrobial strategies for urinary catheters. J Biomed Mater Res A 107: 445-467. doi: 10.1002/jbm.a.36561
![]() |
[31] | Dwivedi P, Narvi SS, Tewari RP (2013) Application of polymer nanocomposites in the nanomedicine landscape: Envisaging strategies to combat implant associated infections. J Appl Biomater Funct Mater 11: 129-142. |
[32] |
Singha P, Locklin J, Handa H (2017) A review of the recent advances in antimicrobial coatings for urinary catheters. Acta Biomater 50: 20-40. doi: 10.1016/j.actbio.2016.11.070
![]() |
[33] |
Prabhurajeshwar C, Chandrakanth RK (2017) Probiotic potential of lactobacilli with antagonistic activity against pathogenic strains: An in vitro validation for the production of inhibitory substances. Biomed J 40: 270-283. doi: 10.1016/j.bj.2017.06.008
![]() |
[34] |
de Melo Pereira GV, de Oliveira Coelho B, Magalhães Júnior AI, et al. (2018) How to select a probiotic? A review and update of methods and criteria. Biotechnol Adv 36: 2060-2076. doi: 10.1016/j.biotechadv.2018.09.003
![]() |
[35] |
Radaic A, Ye C, Parks B, et al. (2020) Modulation of pathogenic oral biofilms towards health with nisin probiotic. J Oral Microbiol 12: 1809302. doi: 10.1080/20002297.2020.1809302
![]() |
[36] | FAO (2006) Probiotics in food: Health and nutritional properties and guidelines for evaluation, 2006. Available from: http://www.fao.org/3/a-a0512e.pdf. |
[37] | Carvalho FM, Teixeira-Santos R, Mergulhão FJM, et al. (2021) The use of probiotics to fight biofilms in medical devices: A systematic review and meta-analysis. Microorganisms 9: 1-26. |
[38] |
Barzegari A, Kheyrolahzadeh K, Mahdi S, et al. (2020) The battle of probiotics and their derivatives against biofilms. Infect Drug Resist 13: 659-672. doi: 10.2147/IDR.S232982
![]() |
[39] |
Reid G (1999) The scientific basis for probiotic strains of Lactobacillus. Appl Environ Microb 65: 3763-3766. doi: 10.1128/AEM.65.9.3763-3766.1999
![]() |
[40] |
Markowiak P, Ślizewska K (2017) Effects of probiotics, prebiotics, and synbiotics on human health. Nutrients 9: 1021. doi: 10.3390/nu9091021
![]() |
[41] |
Suez J, Zmora N, Segal E, et al. (2019) The pros, cons, and many unknowns of probiotics. Nat Med 25: 716-729. doi: 10.1038/s41591-019-0439-x
![]() |
[42] |
Salas-Jara M, Ilabaca A, Vega M, et al. (2016) Biofilm forming Lactobacillus: New challenges for the development of probiotics. Microorganisms 4: 35. doi: 10.3390/microorganisms4030035
![]() |
[43] |
Saxelin M, Tynkkynen S, Mattila-Sandholm T, et al. (2005) Probiotic and other functional microbes: From markets to mechanisms. Curr Opin Biotech 16: 204-211. doi: 10.1016/j.copbio.2005.02.003
![]() |
[44] | Fioramonti J, Theodorou V, Bueno L (2003) Probiotics: What are they? What are their effects on gut physiology? Best Pract Res Cl Ga 17: 711-724. |
[45] |
Gogineni VK, Morrow LE (2013) Probiotics: Mechanisms of action and clinical applications. J Prob Health 1: 1-11. doi: 10.4172/2329-8901.1000101
![]() |
[46] |
Hemaiswarya S, Raja R, Ravikumar R, et al. (2013) Mechanism of action of probiotics. Braz Arch Biol Techn 56: 113-119. doi: 10.1590/S1516-89132013000100015
![]() |
[47] | Khalighi A, Behdani R, Kouhestani S (2016) Probiotics: A comprehensive review of their classification, mode of action and role in human nutrition, In: Rao V, Rao L, Probiotics and Prebiotics in Human Nutrition and Health, Croatia: InTech, 10: 63646. |
[48] |
Moher D, Liberati A, Tetzlaff J, et al. (2009) Preferred reporting items for systematic reviews and meta-analyses: The PRISMA statement. Plos Med 6: e1000097. doi: 10.1371/journal.pmed.1000097
![]() |
[49] | Nemcová R (1997) Criteria for selection of lactobacilli for probiotic use. Vet Med 42: 19-27. |
[50] |
Kim AR, Ahn KB, Yun CH, et al. (2019) Lactobacillus plantarum lipoteichoic acid inhibits oral multispecies biofilm. J Endod 45: 310-315. doi: 10.1016/j.joen.2018.12.007
![]() |
[51] |
Bermudez-Brito M, Plaza-Díaz J, Muñoz-Quezada S, et al. (2012) Probiotic mechanisms of action. Ann Nutr Metab 61: 160-174. doi: 10.1159/000342079
![]() |
[52] |
Muñoz M, Mosquera A, Alméciga-Díaz CJ, et al. (2012) Fructooligosaccharides metabolism and effect on bacteriocin production in Lactobacillus strains isolated from ensiled corn and molasses. Anaerobe 18: 321-330. doi: 10.1016/j.anaerobe.2012.01.007
![]() |
[53] |
Kechagia M, Basoulis D, Konstantopoulou S, et al. (2013) Health benefits of probiotics: A review. ISRN Nutr 2013: 481651. doi: 10.5402/2013/481651
![]() |
[54] |
Holzapfel WH, Haberer P, Geisen R, et al. (2001) Taxonomy and important features of probiotic microorganisms in food and nutrition. Am J Clin Nutr 73: 365-373. doi: 10.1093/ajcn/73.2.365s
![]() |
[55] |
Carr FJ, Chill D, Maida N (2002) The lactic acid bacteria: A literature survey. Crit Rev Microbiol 28: 281-370. doi: 10.1080/1040-840291046759
![]() |
[56] |
Leroy F, De Vuyst L (2004) Lactic acid bacteria as functional starter cultures for the food fermentation industry. Trends Food Sci Tech 15: 67-78. doi: 10.1016/j.tifs.2003.09.004
![]() |
[57] |
Ng SC, Hart AL, Kamm MA, et al. (2009) Mechanisms of action of probiotics: Recent advances. Inflamm Bowel Dis 15: 300-310. doi: 10.1002/ibd.20602
![]() |
[58] | Chen Q, Zhu Z, Wang J, et al. (2017) Probiotic E. coli Nissle 1917 biofilms on silicone substrates for bacterial interference against pathogen colonization. Acta Biomater 50: 353-360. |
[59] | Maldonado NC, Silva De Ruiz C, Cecilia M, et al. (2007) A simple technique to detect Klebsiella biofilm-forming-strains. Inhibitory potential of Lactobacillus fermentum CRL 1058 whole cells and products, In: Méndez-Vilas A, Communicating Current Research and Educational Topics and Trends in Applied Microbiology, Formatex, 52-59. |
[60] |
McMillan A, Dell M, Zellar MP, et al. (2011) Disruption of urogenital biofilms by lactobacilli. Colloid Surface B 86: 58-64. doi: 10.1016/j.colsurfb.2011.03.016
![]() |
[61] |
Velraeds MMC, van Belt-Gritter B De, Busscher HJ, et al. (2000) Inhibition of uropathogenic biofilm growth on silicone rubber in human urine by lactobacilli—A teleologic approach. World J Urol 18: 422-426. doi: 10.1007/PL00007084
![]() |
[62] |
Reid G, Tieszer C (1994) Use of lactobacilli to reduce the adhesion of Staphylococcus aureus to catheters. Int Biodeter Biodegr 34: 73-83. doi: 10.1016/0964-8305(95)00011-9
![]() |
[63] | Millsap K, Reid G, van der Mei HC, et al. (1994) Displacement of Enterococcus faecalis from hydrophobic and hydrophilic substrata by Lactobacillus and Streptococcus spp. as studied in a parallel plate flow chamber. Appl Environ Microb 60: 1867-1874. |
[64] |
Ifeoma ME, Jennifer UK (2016) Inhibition of biofilms on urinary catheters using immobilized Lactobacillus cells. African J Microbiol Res 10: 920-929. doi: 10.5897/AJMR2016.8056
![]() |
[65] |
Derakhshandeh S, Shahrokhi N, Khalaj V, et al. (2020) Surface display of uropathogenic Escherichia coli FimH in Lactococcus lactis: In vitro characterization of recombinant bacteria and its protectivity in animal model. Microb Pathogensis 141: 103974. doi: 10.1016/j.micpath.2020.103974
![]() |
[66] | Busscher HJ, van Hoogmoed CG, Geertsema-Doornbusch GI, et al. (1997) Streptococcus thermophilus and its biosurfactants inhibit adhesion by Candida spp. on silicone rubber. Appl Environ Microb 63: 3810-3817. |
[67] |
van der Mei HC, van de Belt-Gritter B, van Weissenbruch R, et al. (1999) Effect of consumption of dairy products with probiotic bacteria on biofilm formation on silicone rubber implant surfaces in an artificial throat. Food Bioprod Process 77: 156-158. doi: 10.1205/096030899532303
![]() |
[68] |
van der Mei HC, Free RH, Elving GJ, et al. (2000) Effect of probiotic bacteria on prevalence of yeasts in oropharyngeal biofilms on silicone rubber voice prostheses in vitro. J Med Microbiol 49: 713-718. doi: 10.1099/0022-1317-49-8-713
![]() |
[69] |
van der Mei HC, Buijssen KJDA, van der Laan BFAM, et al. (2014) Voice prosthetic biofilm formation and Candida morphogenic conversions in absence and presence of different bacterial strains and species on silicone-rubber. Plos One 9: e104508. doi: 10.1371/journal.pone.0104508
![]() |
[70] |
Jiang Q, Stamatova I, Kainulainen V, et al. (2016) Interactions between Lactobacillus rhamnosus GG and oral micro-organisms in an in vitro biofilm model. BMC Microbiol 16: 149. doi: 10.1186/s12866-016-0759-7
![]() |
[71] |
Kang MS, Oh JS, Lee HC, et al. (2011) Inhibitory effect of Lactobacillus reuteri on periodontopathic and cariogenic bacteria. J Microbiol 49: 193-199. doi: 10.1007/s12275-011-0252-9
![]() |
[72] |
Teanpaisan R, Piwat S, Dahlén G (2011) Inhibitory effect of oral Lactobacillus against oral pathogens. Lett Appl Microbiol 53: 452-459. doi: 10.1111/j.1472-765X.2011.03132.x
![]() |
[73] | Marttinen AM, Haukioja AL, Keskin M, et al. (2013) Effects of Lactobacillus reuteri PTA 5289 and L. paracasei DSMZ16671 on the adhesion and biofilm formation of Streptococcus mutans. Curr Microbiol 67: 193-199. |
[74] |
Söderling EM, Marttinen AM, Haukioja AL (2011) Probiotic lactobacilli interfere with Streptococcus mutans biofilm formation in vitro. Curr Microbiol 62: 618-622. doi: 10.1007/s00284-010-9752-9
![]() |
[75] |
Lee SH, Kim YJ (2014) A comparative study of the effect of probiotics on cariogenic biofilm model for preventing dental caries. Arch Microbiol 196: 601-609. doi: 10.1007/s00203-014-0998-7
![]() |
[76] |
Jaffar N, Ishikawa Y, Mizuno K, et al. (2016) Mature biofilm degradation by potential probiotics: Aggregatibacter actinomycetemcomitans versus Lactobacillus spp. Plos One 11: e0159466. doi: 10.1371/journal.pone.0159466
![]() |
[77] | Vilela SFG, Barbosa JO, Rossoni RD, et al. (2015) Lactobacillus acidophilus ATCC 4356 inhibits biofilm formation by C. albicans and attenuates the experimental candidiasis in Galleria mellonella. Virulence 6: 29-39. |
[78] |
Krzyściak W, Kościelniak D, Papież M, et al. (2017) Effect of a Lactobacillus salivarius probiotic on a double-species Streptococcus mutans and Candida albicans caries biofilm. Nutrients 9: 1242. doi: 10.3390/nu9111242
![]() |
[79] |
Rossoni RD, de Barros PP, de Alvarenga JA, et al. (2018) Antifungal activity of clinical Lactobacillus strains against Candida albicans biofilms: Identification of potential probiotic candidates to prevent oral candidiasis. Biofouling 34: 212-225. doi: 10.1080/08927014.2018.1425402
![]() |
[80] |
Lin X, Chen X, Tu Y, et al. (2017) Effect of probiotic lactobacilli on the growth of Streptococcus mutans and multispecies biofilms isolated from children with active caries. Med Sci Monit 23: 4175-4181. doi: 10.12659/MSM.902237
![]() |
[81] |
Song YG, Lee SH (2017) Inhibitory effects of Lactobacillus rhamnosus and Lactobacillus casei on Candida biofilm of denture surface. Arch Oral Biol 76: 1-6. doi: 10.1016/j.archoralbio.2016.12.014
![]() |
[82] | Ciandrini E, Campana R, Baffone W (2017) Live and heat-killed Lactobacillus spp. interfere with Streptococcus mutans and Streptococcus oralis during biofilm development on titanium surface. Arch Oral Biol 78: 48-57. |
[83] |
Comelli EM, Guggenheim B, Stingele F, et al. (2002) Selection of dairy bacterial strains as probiotics for oral health. Eur J Oral Sci 110: 218-224. doi: 10.1034/j.1600-0447.2002.21216.x
![]() |
[84] |
James KM, MacDonald KW, Chanyi RM, et al. (2016) Inhibition of Candida albicans biofilm formation and modulation of gene expression by probiotic cells and supernatant. J Med Microbiol 65: 328-336. doi: 10.1099/jmm.0.000226
![]() |
[85] |
Fernández CE, Giacaman RA, Tenuta LM, et al. (2015) Effect of the probiotic Lactobacillus rhamnosus LB21 on the cariogenicity of Streptococcus mutans UA159 in a dual-species biofilm model. Caries Res 49: 583-590. doi: 10.1159/000439315
![]() |
[86] |
Wu CC, Lin CT, Wu CY, et al. (2015) Inhibitory effect of Lactobacillus salivarius on Streptococcus mutans biofilm formation. Mol Oral Microbiol 30: 16-26. doi: 10.1111/omi.12063
![]() |
[87] |
Tahmourespour A, Kermanshahi RK (2011) The effect of a probiotic strain (Lactobacillus acidophilus) on the plaque formation of oral streptococci. Bosn J Basic Med Sci 11: 37-40. doi: 10.17305/bjbms.2011.2621
![]() |
[88] |
Vacca C, Contu MP, Rossi C, et al. (2020) In vitro interactions between Streptococcus intermedius and Streptococcus salivarius K12 on a titanium cylindrical surface. Pathogens 9: 1069. doi: 10.3390/pathogens9121069
![]() |
[89] | Fang K, Jin X, Hong SH (2018) Probiotic Escherichia coli inhibits biofilm formation of pathogenic E. coli via extracellular activity of DegP. Sci Rep 8: 4939. |
[90] |
Hancock V, Dahl M, Klemm P (2010) Probiotic Escherichia coli strain Nissle 1917 outcompetes intestinal pathogens during biofilm formation. J Med Microbiol 59: 392-399. doi: 10.1099/jmm.0.008672-0
![]() |
[91] | Kıvanç M, Er S (2020) Biofilm formation of Candida spp. isolated from the vagina and antibiofilm activities of lactic acid bacteria on the these Candida isolates. Afr Health Sci 20: 641-648. |
[92] |
Matsubara VH, Wang Y, Bandara HMHN, et al. (2016) Probiotic lactobacilli inhibit early stages of Candida albicans biofilm development by reducing their growth, cell adhesion, and filamentation. Appl Microbiol Biot 100: 6415-6426. doi: 10.1007/s00253-016-7527-3
![]() |
[93] | Alexandre Y, Le Berre R, Barbier G, et al. (2014) Screening of Lactobacillus spp. for the prevention of Pseudomonas aeruginosa pulmonary infections. BMC Microbiol 14: 107. |
[94] |
Song H, Zhang J, Qu J, et al. (2019) Lactobacillus rhamnosus GG microcapsules inhibit Escherichia coli biofilm formation in coculture. Biotechnol Lett 41: 1007-1014. doi: 10.1007/s10529-019-02694-2
![]() |
[95] |
Sambanthamoorthy K, Feng X, Patel R, et al. (2014) Antimicrobial and antibiofilm potential of biosurfactants isolated from lactobacilli against multi-drug-resistant pathogens. BMC Microbiol 14: 197. doi: 10.1186/1471-2180-14-197
![]() |
[96] |
Kaur S, Sharma P, Kalia N, et al. (2018) Anti-biofilm properties of the fecal probiotic lactobacilli against Vibrio spp. Front Cell Infect Mi 8: 120. doi: 10.3389/fcimb.2018.00120
![]() |
[97] |
Otero MC, Nader-Macías ME (2006) Inhibition of Staphylococcus aureus by H2O2-producing Lactobacillus gasseri isolated from the vaginal tract of cattle. Anim Reprod Sci 96: 35-46. doi: 10.1016/j.anireprosci.2005.11.004
![]() |
[98] |
Azeredo J, Azevedo NF, Briandet R, et al. (2017) Critical review on biofilm methods. Crit Rev Microbiol 43: 313-351. doi: 10.1080/1040841X.2016.1208146
![]() |
[99] | Gomes M, Gomes LC, Santos RT, et al. (2020) PDMS in urinary tract devices: Applications, problems and potential solutions, In: Carlsen PN, 1st Ed, Polydimethylsiloxane: Structure and Applications, Nova Science Publishers. |
[100] |
Khan F, Tabassum N, Kim YM (2020) A strategy to control colonization of pathogens: Embedding of lactic acid bacteria on the surface of urinary catheter. Appl Microbiol Biot 104: 9053-9066. doi: 10.1007/s00253-020-10903-6
![]() |
[101] |
Zhu Z, Yu F, Chen H, et al. (2017) Coating of silicone with mannoside-PAMAM dendrimers to enhance formation of non-pathogenic Escherichia coli biofilms against colonization of uropathogens. Acta Biomater 64: 200-210. doi: 10.1016/j.actbio.2017.10.008
![]() |
[102] |
Lopez AI, Kumar A, Planas MR, et al. (2011) Biomaterials biofunctionalization of silicone polymers using poly(amidoamine) dendrimers and a mannose derivative for prolonged interference against pathogen colonization. Biomaterials 32: 4336-4346. doi: 10.1016/j.biomaterials.2011.02.056
![]() |
[103] |
Gross G, van Der Meulen J, Snel J, et al. (2008) Mannose-specific interaction of Lactobacillus plantarum with porcine jejunal epithelium. FEMS Immunol Med Mic 54: 215-223. doi: 10.1111/j.1574-695X.2008.00466.x
![]() |
[104] |
Barth KA, Coullerez G, Nilsson LM, et al. (2008) An engineered mannoside presenting platform: Escherichia coli adhesion under static and dynamic conditions. Adv Funct Mater 18: 1459-1469. doi: 10.1002/adfm.200701246
![]() |
[105] | Tan L, Fu J, Feng F, et al. (2020) Engineered probiotics biofilm enhances osseointegration via immunoregulation and anti-infection. Sci Adv 6: eaba5723. |
[106] |
Piqué N, Berlanga M, Miñana-Galbis D (2019) Health benefits of heat-killed (Tyndallized) probiotics: An overview. Int J Mol Sci 20: 2534. doi: 10.3390/ijms20102534
![]() |
1. | David da Silva Barreira, Julie Laurent, Jessica Lourenço, Julia Novion Ducassou, Yohann Couté, Jean Guzzo, Aurélie Rieu, Membrane vesicles released by Lacticaseibacillus casei BL23 inhibit the biofilm formation of Salmonella Enteritidis, 2023, 13, 2045-2322, 10.1038/s41598-023-27959-9 | |
2. | Palkar Omkar Prakash, Keerthi Rayasam, Kolluru Viswanatha Chaitanya, Vidyullatha Peddireddy, 2023, 9780323918060, 131, 10.1016/B978-0-323-91806-0.00017-5 | |
3. | Fábio M. Carvalho, Filipe J. M. Mergulhão, Luciana C. Gomes, Using Lactobacilli to Fight Escherichia coli and Staphylococcus aureus Biofilms on Urinary Tract Devices, 2021, 10, 2079-6382, 1525, 10.3390/antibiotics10121525 | |
4. | Muluneh Assefa, Azanaw Amare, Biofilm-Associated Multi-Drug Resistance in Hospital-Acquired Infections: A Review, 2022, Volume 15, 1178-6973, 5061, 10.2147/IDR.S379502 | |
5. | Suparna Dutta Sinha, Madhumita Choudhuri, Tania Basu, Debkishore Gupta, Alokmay Datta, Decisive Role of Polymer–Bovine Serum Albumin Interactions in Biofilm Substrates on “Philicity” and Extracellular Polymeric Substances Composition, 2022, 38, 0743-7463, 1966, 10.1021/acs.langmuir.1c00187 | |
6. | Fábio M. Carvalho, Rita Teixeira-Santos, Filipe J. M. Mergulhão, Luciana C. Gomes, Effect of Lactobacillus plantarum Biofilms on the Adhesion of Escherichia coli to Urinary Tract Devices, 2021, 10, 2079-6382, 966, 10.3390/antibiotics10080966 | |
7. | Bindu Sadanandan, Vijayalakshmi Vaniyamparambath, K. N. Lokesh, Kalidas Shetty, Amruta P. Joglekar, Priya Ashrit, Beena Hemanth, Candida albicans biofilm formation and growth optimization for functional studies using response surface methodology, 2022, 132, 1365-2672, 3277, 10.1111/jam.15402 | |
8. | Andreia R. Tomé, Fábio M. Carvalho, Rita Teixeira-Santos, Mette Burmølle, Filipe J. M. Mergulhão, Luciana C. Gomes, Use of Probiotics to Control Biofilm Formation in Food Industries, 2023, 12, 2079-6382, 754, 10.3390/antibiotics12040754 | |
9. | Claudia María Bedoya-Correa, Santiago Betancur-Giraldo, John Franco, Santiago Arango-Santander, Probiotic Effect of Streptococcus dentisani on Oral Pathogens: An In Vitro Study, 2024, 13, 2076-0817, 351, 10.3390/pathogens13050351 | |
10. | Abdussalam Adeshina Sulaiman, Adewale Ayodeji Adetoye, Funmilola Abidemi Ayeni, LACTIC ACID BACTERIA AND FERMENTED MAIZE SUPERNATANT (Omidun) HAVE ANTI-BIOFILM PROPERTIES AGAINST STAPHYLOCOCCI AND ENTEROAGGREGATIVE Escherichia coli STRAINS, 2024, 7, 2616-1370, 250, 10.33003/fjs-2023-0706-2134 |
Probiotic strains | Strategy | Biofilm-forming pathogens | Percentages of reduction | Ref. | Outcomes |
B. infantis | Displacement | Multispecies | 26% | [68] | Exposure of multispecies biofilms composed by Candida ssp. and streptococcal and staphylococcal strains to B. infantis on silicone rubber did not significantly reduce the number of yeasts in the biofilm. |
E. coli Nissle 1917 (EcN) | Competition | Gram-negative | 78%–93% | [89,90] | EcN was able to decrease the adhesion of S. aureus and S. epidermidis by 99.9%, and of E. coli and P. aeruginosa by 93% and 78%, respectively, on polypropylene after 24 h. |
Gram-positive | 99.9% | [89] | |||
Exclusion | Gram-positive | 99.9% | [58] | Pre-coating of silicone with EcN biofilms reduced the adhesion of Ent. faecalis up to approximately 2 log CFU/cm2 for 11 days. | |
Ent. faecium | Displacement | Multispecies | 16% | [68] | Exposure of multispecies biofilms composed by Candida ssp. and streptococcal and staphylococcal strains to Ent. faecium on silicone rubber did not significantly reduce the number of yeasts in the biofilm. |
L. acidophilus | Competition | Gram-positive | 11%–99% | [62,75,87] | L. acidophilus reduced the adhesion of S. aureus to silicone up to 99% after 1 h and of Strep. mutans in 33% and 61% after 24 and 48 h, respectively. C. albicans adhesion to polystyrene and silicone was reduced by about 47% and 98%, respectively, while C. tropicalis adhesion to silicone was reduced by 72% after 8 days. The adhesion of a streptococcal multispecies biofilm to polystyrene was reduced by 63% after 48 h. |
Yeast | 47%–98% | [69,91] | |||
Multispecies | 63% | [75] | |||
L. acidophilus | Displacement | Gram-negative | 90% | [76] | L. acidophilus was able to displace pre-formed biofilms of Ag. actinomycetemcomitans from polystyrene in 90% after 24 h and of S. aureus from silicone up to 98% after 1 h. C. albicans biofilms formed on polystyrene were removed in 57.5% and 59% after 24 and 48 h of contact. |
Gram-positive | 79%–98% | [62] | |||
Yeast | 57.5%–59% | [77,92] | |||
Exclusion | Gram-negative | 99.9% | [64] | Pre-coating silicone with L. acidophilus biofilms inhibited K. pneumoniae and S. aureus biofilm formation up to 99.9% after 7 days. The total biofilm mass of Strep. mutans and non-mutans streptococci was reduced by 43% and 9%, respectively, after 24 h. | |
Gram-positive | 9%–99.9% | [62,64,87] | |||
L. casei | Competition | Gram-positive | 64%–99% | [62,72,75,80] | L. casei inhibited the onset of biofilms of S. aureus on silicone up to 99% after 1 h and of Strep. mutans on hydroxyapatite (75%) and polystyrene (64%) after 24 and 48 h, respectively. C. albicans adherence to silicone was reduced by 95% after 8 days. The adhesion of multispecies biofilms to hydroxyapatite, polystyrene and silicone was reduced by 52, 72 and 99.9%, respectively. |
Yeast | 95% | [69] | |||
Multispecies | 52%–99.9% | [61,75,80] | |||
Displacement | Gram-negative | 90% | [76] | L. casei displaced up to 98% of S. aureus biofilms formed on silicone after 1 h, and 54% of Ent. faecalis on glass and 31% on FEP after 3 h. Pre-formed biofilms of Ag. actinomycetemcomitans and C. albicans in polystyrene were disrupted in 90% and 56.3% after 24 h. C. albicans biofilm was reduced by 99.9% in a denture base resin for 12 h. A multispecies biofilm was removed from silicone in 61% after 12 days. | |
Gram-positive | 31%–98% | [62,63] | |||
Yeast | 56.3%–99.9% | [81,92] | |||
Multispecies | 61% | [68] | |||
Exclusion | Gram-positive | 70%–99% | [62] | The adhesion of S. aureus to silicone and of C. albicans to denture base resin was reduced up to 99.9% after 1 and 12 h, respectively. | |
Yeast | 99.9% | [81] | |||
L. crispatus | Competition | Yeast | 72%–99% | [69] | L. crispatus decreased the number of biofilm culturable cells of C. albicans and C. tropicalis on silicone rubber by 99% and 72%, respectively, after 8 days. |
L. delbrueckii | Displacement | Gram-negative | 92% | [76] | L. delbrueckii significantly degraded the pre-formed biofilm of Ag. actinomycetemcomitans on polystyrene after 24 h of contact. |
L. fermentum | Competition | Gram-negative | 12%–99% | [59,93] | L. fermentum inhibited P. aeruginosa (12%) and K. pneumoniae (99%) biofilm formation in polystyrene for 7 and 24 h, respectively. The adhesion of S. aureus and mixed cultures to silicone was inhibited up to 99.9% for 1 h and 8 days, respectively. |
Gram-positive | 84%–99% | [62,72] | |||
Multispecies | 77%–99.9% | [61] | |||
Displacement | Gram-negative | 93% | [76] | L. fermentum led to a reduction of pre-formed biofilms of Ag. actinomycetemcomitans of 93% and C. albicans of 68% after 24 and 48 h, respectively. S. aureus and multispecies biofilms were removed from silicone up to 98% and by 33%, respectively, after 12 days of incubation. | |
Gram-positive | 79%–98% | [62] | |||
Yeast | 68% | [79] | |||
Multispecies | 33% | [68] | |||
Exclusion | Gram-positive | 70%–99% | [62] | Silicone pre-coated with L. fermentum reduced S. aureus adhesion up to 99% during 1 h of incubation. | |
Lact. lactis | Competition | Gram-negative | 30%–56% | [83] | Lact. lactis caused a significant decrease in the adhesion of five oral species (A. naeslundii, F. nucleatum, Strep. oralis, Strep. sobrinus and V. dispar) to hydroxyapatite (up to 74% after 40.5 h). Multispecies biofilm formation on polystyrene and hydroxyapatite was inhibited up to 66%. |
Gram-positive | 25%–74% | [83] | |||
Multispecies | 29%–66% | [35,83] | |||
Displacement | Gram-negative | 73% | [76] | Lact. lactis disrupted the pre-formed biofilms of Ag. actinomycetemcomitans in 73% after 24 h of treatment. Additionally, multispecies biofilms were removed from polystyrene (39%) and silicone (up to 96%) after 24 h and 12 days, respectively. | |
Multispecies | 7%–96% | [35,67,68] | |||
Lact. lactis | Exclusion | Gram-negative | 56%–99.9% | [65,83] | Pre-coating hydroxyapatite with Lact. lactis reduced the adhesion of five oral species (A. naeslundii, F. nucleatum, Strep. oralis, Strep. sobrinus and V. dispar) and multispecies biofilm formation up to 85% and 77%, respectively, after 40.5 h. Also, Lact. lactis inhibited the adhesion of E. coli to polystyrene after 24 and 48 h in about 3 and 2 log, respectively. |
Gram-positive | 35%–85% | [83] | |||
Multispecies | 62%–77% | [83] | |||
Multispecies (combinations of L. plantarum, L. helveticus and S. salivarius) | Competition | Yeast | 62%–72% | [84] | The combinations of probiotic species were able to significantly reduce the adherence of C. albicans to polyurethane discs. |
Displacement | Yeast | 63%–80% | [84] | The combinations of probiotic species reduced C. albicans biofilm size by more than 63% after 1 h. | |
Strep. salivarius | Competition | Gram-positive | 74%–87% | [88] | Strep. salivarius was able to interfere with the biofilm formation of Strep. intermedius in a titanium surface during 8 and 20 h of exposure. |
Strep. thermophilus | Competition | Gram-negative | 26% | [83] | Strep. thermophilus caused a decrease in the adhesion of four oral species (A. naeslundii, Strep. oralis, Strep. sobrinus and V. dispar) and multispecies biofilm formation on hydroxyapatite up to 65% and 55%, respectively. |
Gram-positive | 8%–65% | [83] | |||
Multispecies | 23%–55% | [83] | |||
Displacement | Multispecies | 67% | [68] | Strep. thermophilus significantly reduced the prevalence of yeasts in multispecies biofilms formed on silicone during 12 days. | |
Exclusion | Gram-negative | 30% | [83] | Pre-coating hydroxyapatite with Strep. thermophilus reduced the adhesion of four oral species (A. naeslundii, Strep. oralis, Strep. sobrinus and V. dispar) and multispecies biofilm formation up to 50%. | |
Gram-positive | 8%–50% | [83] | |||
Yeast | N.A. | [66] | |||
Multispecies | 26%–49% | [83] | |||
Strep. hyointestinalis | Displacement | Gram-positive | 74%–77% | [63] | Ent. faecalis was displaced by Strep. hyointestinalis from FEP and glass in 74% and 77%, respectively, after 3 h. |
Advantages | Disadvantages |
Generally Regarded as Safe (GRAS) status Withstand unfavorable conditions of the human body Survive, maintain the metabolic activity and grow in the target site Antimicrobial activity Maintain high viability at processing, lyophilization, and storage High biomass amount and productivity of cultures Positive effect on several diseases and illness conditions Increase the efficiency of the immunological system Lower cytotoxicity than conventional antimicrobial agents Wide range of mechanisms of action Both broad and narrow spectrum of action Genetically stable and good in vitro and in vivo growth properties Able to adhere to biotic and abiotic surfaces Natural origin Biodegradable |
Risk of biofilm translocation from the application site to the bloodstream Risk of transmission/acquisition of antibiotic resistance genes Risk of infection and/or morbidity in vulnerable groups Heterogeneous activity (i.e., the same strain may have different behavior under different conditions) Biofilm detachment overtime |
Probiotic strains | Strategy | Biofilm-forming pathogens | Percentages of reduction | Ref. | Outcomes |
B. infantis | Displacement | Multispecies | 26% | [68] | Exposure of multispecies biofilms composed by Candida ssp. and streptococcal and staphylococcal strains to B. infantis on silicone rubber did not significantly reduce the number of yeasts in the biofilm. |
E. coli Nissle 1917 (EcN) | Competition | Gram-negative | 78%–93% | [89,90] | EcN was able to decrease the adhesion of S. aureus and S. epidermidis by 99.9%, and of E. coli and P. aeruginosa by 93% and 78%, respectively, on polypropylene after 24 h. |
Gram-positive | 99.9% | [89] | |||
Exclusion | Gram-positive | 99.9% | [58] | Pre-coating of silicone with EcN biofilms reduced the adhesion of Ent. faecalis up to approximately 2 log CFU/cm2 for 11 days. | |
Ent. faecium | Displacement | Multispecies | 16% | [68] | Exposure of multispecies biofilms composed by Candida ssp. and streptococcal and staphylococcal strains to Ent. faecium on silicone rubber did not significantly reduce the number of yeasts in the biofilm. |
L. acidophilus | Competition | Gram-positive | 11%–99% | [62,75,87] | L. acidophilus reduced the adhesion of S. aureus to silicone up to 99% after 1 h and of Strep. mutans in 33% and 61% after 24 and 48 h, respectively. C. albicans adhesion to polystyrene and silicone was reduced by about 47% and 98%, respectively, while C. tropicalis adhesion to silicone was reduced by 72% after 8 days. The adhesion of a streptococcal multispecies biofilm to polystyrene was reduced by 63% after 48 h. |
Yeast | 47%–98% | [69,91] | |||
Multispecies | 63% | [75] | |||
L. acidophilus | Displacement | Gram-negative | 90% | [76] | L. acidophilus was able to displace pre-formed biofilms of Ag. actinomycetemcomitans from polystyrene in 90% after 24 h and of S. aureus from silicone up to 98% after 1 h. C. albicans biofilms formed on polystyrene were removed in 57.5% and 59% after 24 and 48 h of contact. |
Gram-positive | 79%–98% | [62] | |||
Yeast | 57.5%–59% | [77,92] | |||
Exclusion | Gram-negative | 99.9% | [64] | Pre-coating silicone with L. acidophilus biofilms inhibited K. pneumoniae and S. aureus biofilm formation up to 99.9% after 7 days. The total biofilm mass of Strep. mutans and non-mutans streptococci was reduced by 43% and 9%, respectively, after 24 h. | |
Gram-positive | 9%–99.9% | [62,64,87] | |||
L. casei | Competition | Gram-positive | 64%–99% | [62,72,75,80] | L. casei inhibited the onset of biofilms of S. aureus on silicone up to 99% after 1 h and of Strep. mutans on hydroxyapatite (75%) and polystyrene (64%) after 24 and 48 h, respectively. C. albicans adherence to silicone was reduced by 95% after 8 days. The adhesion of multispecies biofilms to hydroxyapatite, polystyrene and silicone was reduced by 52, 72 and 99.9%, respectively. |
Yeast | 95% | [69] | |||
Multispecies | 52%–99.9% | [61,75,80] | |||
Displacement | Gram-negative | 90% | [76] | L. casei displaced up to 98% of S. aureus biofilms formed on silicone after 1 h, and 54% of Ent. faecalis on glass and 31% on FEP after 3 h. Pre-formed biofilms of Ag. actinomycetemcomitans and C. albicans in polystyrene were disrupted in 90% and 56.3% after 24 h. C. albicans biofilm was reduced by 99.9% in a denture base resin for 12 h. A multispecies biofilm was removed from silicone in 61% after 12 days. | |
Gram-positive | 31%–98% | [62,63] | |||
Yeast | 56.3%–99.9% | [81,92] | |||
Multispecies | 61% | [68] | |||
Exclusion | Gram-positive | 70%–99% | [62] | The adhesion of S. aureus to silicone and of C. albicans to denture base resin was reduced up to 99.9% after 1 and 12 h, respectively. | |
Yeast | 99.9% | [81] | |||
L. crispatus | Competition | Yeast | 72%–99% | [69] | L. crispatus decreased the number of biofilm culturable cells of C. albicans and C. tropicalis on silicone rubber by 99% and 72%, respectively, after 8 days. |
L. delbrueckii | Displacement | Gram-negative | 92% | [76] | L. delbrueckii significantly degraded the pre-formed biofilm of Ag. actinomycetemcomitans on polystyrene after 24 h of contact. |
L. fermentum | Competition | Gram-negative | 12%–99% | [59,93] | L. fermentum inhibited P. aeruginosa (12%) and K. pneumoniae (99%) biofilm formation in polystyrene for 7 and 24 h, respectively. The adhesion of S. aureus and mixed cultures to silicone was inhibited up to 99.9% for 1 h and 8 days, respectively. |
Gram-positive | 84%–99% | [62,72] | |||
Multispecies | 77%–99.9% | [61] | |||
Displacement | Gram-negative | 93% | [76] | L. fermentum led to a reduction of pre-formed biofilms of Ag. actinomycetemcomitans of 93% and C. albicans of 68% after 24 and 48 h, respectively. S. aureus and multispecies biofilms were removed from silicone up to 98% and by 33%, respectively, after 12 days of incubation. | |
Gram-positive | 79%–98% | [62] | |||
Yeast | 68% | [79] | |||
Multispecies | 33% | [68] | |||
Exclusion | Gram-positive | 70%–99% | [62] | Silicone pre-coated with L. fermentum reduced S. aureus adhesion up to 99% during 1 h of incubation. | |
Lact. lactis | Competition | Gram-negative | 30%–56% | [83] | Lact. lactis caused a significant decrease in the adhesion of five oral species (A. naeslundii, F. nucleatum, Strep. oralis, Strep. sobrinus and V. dispar) to hydroxyapatite (up to 74% after 40.5 h). Multispecies biofilm formation on polystyrene and hydroxyapatite was inhibited up to 66%. |
Gram-positive | 25%–74% | [83] | |||
Multispecies | 29%–66% | [35,83] | |||
Displacement | Gram-negative | 73% | [76] | Lact. lactis disrupted the pre-formed biofilms of Ag. actinomycetemcomitans in 73% after 24 h of treatment. Additionally, multispecies biofilms were removed from polystyrene (39%) and silicone (up to 96%) after 24 h and 12 days, respectively. | |
Multispecies | 7%–96% | [35,67,68] | |||
Lact. lactis | Exclusion | Gram-negative | 56%–99.9% | [65,83] | Pre-coating hydroxyapatite with Lact. lactis reduced the adhesion of five oral species (A. naeslundii, F. nucleatum, Strep. oralis, Strep. sobrinus and V. dispar) and multispecies biofilm formation up to 85% and 77%, respectively, after 40.5 h. Also, Lact. lactis inhibited the adhesion of E. coli to polystyrene after 24 and 48 h in about 3 and 2 log, respectively. |
Gram-positive | 35%–85% | [83] | |||
Multispecies | 62%–77% | [83] | |||
Multispecies (combinations of L. plantarum, L. helveticus and S. salivarius) | Competition | Yeast | 62%–72% | [84] | The combinations of probiotic species were able to significantly reduce the adherence of C. albicans to polyurethane discs. |
Displacement | Yeast | 63%–80% | [84] | The combinations of probiotic species reduced C. albicans biofilm size by more than 63% after 1 h. | |
Strep. salivarius | Competition | Gram-positive | 74%–87% | [88] | Strep. salivarius was able to interfere with the biofilm formation of Strep. intermedius in a titanium surface during 8 and 20 h of exposure. |
Strep. thermophilus | Competition | Gram-negative | 26% | [83] | Strep. thermophilus caused a decrease in the adhesion of four oral species (A. naeslundii, Strep. oralis, Strep. sobrinus and V. dispar) and multispecies biofilm formation on hydroxyapatite up to 65% and 55%, respectively. |
Gram-positive | 8%–65% | [83] | |||
Multispecies | 23%–55% | [83] | |||
Displacement | Multispecies | 67% | [68] | Strep. thermophilus significantly reduced the prevalence of yeasts in multispecies biofilms formed on silicone during 12 days. | |
Exclusion | Gram-negative | 30% | [83] | Pre-coating hydroxyapatite with Strep. thermophilus reduced the adhesion of four oral species (A. naeslundii, Strep. oralis, Strep. sobrinus and V. dispar) and multispecies biofilm formation up to 50%. | |
Gram-positive | 8%–50% | [83] | |||
Yeast | N.A. | [66] | |||
Multispecies | 26%–49% | [83] | |||
Strep. hyointestinalis | Displacement | Gram-positive | 74%–77% | [63] | Ent. faecalis was displaced by Strep. hyointestinalis from FEP and glass in 74% and 77%, respectively, after 3 h. |
Advantages | Disadvantages |
Generally Regarded as Safe (GRAS) status Withstand unfavorable conditions of the human body Survive, maintain the metabolic activity and grow in the target site Antimicrobial activity Maintain high viability at processing, lyophilization, and storage High biomass amount and productivity of cultures Positive effect on several diseases and illness conditions Increase the efficiency of the immunological system Lower cytotoxicity than conventional antimicrobial agents Wide range of mechanisms of action Both broad and narrow spectrum of action Genetically stable and good in vitro and in vivo growth properties Able to adhere to biotic and abiotic surfaces Natural origin Biodegradable |
Risk of biofilm translocation from the application site to the bloodstream Risk of transmission/acquisition of antibiotic resistance genes Risk of infection and/or morbidity in vulnerable groups Heterogeneous activity (i.e., the same strain may have different behavior under different conditions) Biofilm detachment overtime |