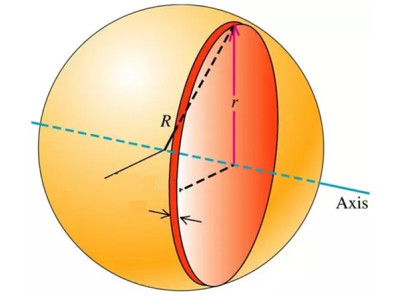
Citation: Alaa. K. Khamis, Allal Bakali, A. A. El-Bary, Haitham. M. Atef. The effect of modified Ohm’s and Fourier’s laws in generalized magneto-thermo viscoelastic spherical region[J]. AIMS Materials Science, 2020, 7(4): 381-398. doi: 10.3934/matersci.2020.4.381
[1] | Julien G. Mahy, Carole Carcel, Michel Wong Chi Man . Evonik P25 photoactivation in the visible range by surface grafting of modified porphyrins for p-nitrophenol elimination in water. AIMS Materials Science, 2023, 10(3): 437-452. doi: 10.3934/matersci.2023024 |
[2] | Gashaw Beyene, Gamachis Sakata, Teshome Senbeta, Belayneh Mesfin . Effect of core size/shape on the plasmonic response of spherical ZnO@Au core-shell nanostructures embedded in a passive host-matrices of MgF2. AIMS Materials Science, 2020, 7(6): 705-719. doi: 10.3934/matersci.2020.6.705 |
[3] | Cabinet Chivimbiso Musuna-Garwe, Netai Mukaratirwa-Muchanyereyi, Mathew Mupa, Courtie Mahamadi, Munyaradzi Mujuru . Preparation and characterization of nanocarbons from Nicotiana tabacum stems. AIMS Materials Science, 2018, 5(6): 1242-1254. doi: 10.3934/matersci.2018.6.1242 |
[4] | Erabhoina Hari Mohan, Varma Siddhartha, Raghavan Gopalan, Tata Narasinga Rao, Dinesh Rangappa . Urea and sucrose assisted combustion synthesis of LiFePO4/C nano-powder for lithium-ion battery cathode application. AIMS Materials Science, 2014, 1(4): 191-201. doi: 10.3934/matersci.2014.4.191 |
[5] | Gashaw Beyene Kassahun . Effect of spacer on size dependent plasmonic properties of triple layered spherical core-shell nanostructure. AIMS Materials Science, 2020, 7(6): 788-799. doi: 10.3934/matersci.2020.6.788 |
[6] | Jing-Fung Lin, Jer-Jia Sheu, Xin-Rong Qiu . Magnetic retardance and magnetic heating in dextran-citrate coated ferrofluids. AIMS Materials Science, 2017, 4(1): 231-249. doi: 10.3934/matersci.2017.1.231 |
[7] | Álvaro Guzmán Aponte, María A Llano Ramírez, Yuliana Cadavid Mora, Juan F Santa Marín, Robison Buitrago Sierra . Cerium oxide nanoparticles for color removal of indigo carmine and methylene blue solutions. AIMS Materials Science, 2020, 7(4): 468-485. doi: 10.3934/matersci.2020.4.468 |
[8] | Qiu-bo Wang, Chao Xu, Yi-bao Jiang, Xian Zhang, Jin-shui Yao, Cong-de Qiao, Qin-ze Liu, Yuan-hong Zhang . The synthesis of conjugated polymers with different length side chains and the effect on their nanoparticles. AIMS Materials Science, 2018, 5(4): 770-780. doi: 10.3934/matersci.2018.4.770 |
[9] | HN Girish, P Madhusudan, CP Sajan, BV Suresh Kumar, K Byrappa . Supercritical hydrothermal synthesis of polycrystalline gadolinium aluminum perovskite materials (GdAlO3, GAP). AIMS Materials Science, 2017, 4(3): 540-550. doi: 10.3934/matersci.2017.3.540 |
[10] | Inês Teixeira, Inês Castro, Violeta Carvalho, Cristina Rodrigues, Andrews Souza, Rui Lima, Senhorinha Teixeira, João Ribeiro . Polydimethylsiloxane mechanical properties: A systematic review. AIMS Materials Science, 2021, 8(6): 952-973. doi: 10.3934/matersci.2021058 |
Abbreviations: λe,μe: Lame elastic constants; ρ: Density; CE: Specific heat at constant strain; K: Thermal conductivity; αt: Coefficient of linear thermal expansion; γe: (3λe+2μe)αt; γo: (3λeαo+2μeα1)αt/γe; αo,α1: Viscoelastic relaxation time; t: Time; qi: Components of heat flux vector; σij: Components of stress tensor; eij: Components of strain tensor; ui: Components of displacement vector; To: Reference temperature; θ: Temperature increment; δij: Kronicker delta; e: Cubical dilatation; R: Radius of the shell; μo: Magnetic permittivity; E: Electric displacement vector; J: Current density vector; H: Total magnetic intensity vector; h: Induced magnetic field vector; Ho: Initial uniform magnetic field; Fi: Components of Lorentz body force; πo: Coefficient connecting the current density with the heat flow density; ko: Coefficient connecting the temperature gradient and electric current density
The traditional uncoupled hypothesis of thermo elasticity predicts two marvels not good with physical perceptions. In the first place, the condition of heat conduction of this hypothesis doesn't contain any elastic terms; second, the heat condition is of an parabolic kind, anticipating unending paces of spread for heat waves.
Biot [1] presented the hypothesis of coupled thermo elasticity to conquer the principal weakness. The overseeing conditions for this hypothesis are coupled, dispensing with the main oddity of the old style hypothesis. In any case, the two hypotheses share the second inadequacy since the heat equation for the coupled hypothesis is likewise parabolic.
Two generalizations to the coupled hypothesis were presented. The first is because of Lord and Shulman [2], who acquired a wave-type heat equation by proposing another law of heat equation to supplant the old style Fourier's law. Since the heat equation of this hypothesis is of the wave type, it consequently guarantees limited velocities of spread for heat and elastic waves. The staying administering equations for this hypothesis, to be specific, the equations of motion and constitutive relations, continue as before as those for the coupled and the uncoupled speculations. The second speculation to the coupled hypothesis of elasticity is what is known as the hypothesis of thermo elasticity with two relaxation times or the hypothesis of temperature-rate-dependent thermo elasticity. Müller [3], in a survey of the thermodynamics of thermo elasticity solids, proposed an entropy creation imbalance, with the assistance of which he thought about limitations on a class of constitutive equations. A generalization of this imbalance was proposed by Green and Laws [4]. Green and Lindsay got an express form of the constitutive equations in [5]. These equations were additionally gotten autonomously by Shuhubi [6] has acquired the fundamental solution for this hypothesis. This hypothesis contains two constants that go about as relaxation times and alter all the equation of the coupled hypothesis, not just the heat equation. The old style Fourier's law of heat equation isn't disregarded if the medium viable has a focal point of balance.
Later Green and Naghdi [7,8,9] proposed three hypotheses of generalized thermo elasticity. The primary model (G-N Ⅰ) is actually equivalent to Biot's hypothesis [1]. The second and third models are named as G-N Ⅱ and G-N Ⅲ model. In G-N Ⅱ and G-N Ⅲ models, the thermal wave engenders with limited rates which concur with physical circumstances. A significant component of G-N Ⅱ hypothesis is that this hypothesis doesn't suits dissipation of thermal energy though G-N Ⅲ hypothesis obliges dissemination of dissipation of thermal energy.
With the quick advancement of polymer science and plastic industry, just as the wide utilization of materials under high temperature in present day innovation and use of science and topography in designing, the hypothetical examination and applications in viscoelastic material has become a significant errand for strong mechanics.
The hypothesis of thermo-viscoelasticity and the solutions of some boundary value problems of thermo-viscoelasticity were researched by Illyushin's and Pobedria [10]. Crafted by Biot [11,12], Morland and Lee [13] and Tanner [14] mate extraordinary walks in the most recent decade in discovering answers for limit esteem issues for linear viscoelasticity materials including temperature varieties for both semi static and dynamic issues. Drozdov [15] inferred a constitutive model in thermo-viscoelasticity which represents changes in elastic moduli and relaxation times. Tasteless [16] connected the arrangement of linear viscoelasticity issues to comparing linear elastic solutions. Lion [17] studied the large deformation behavior of reinforced rubber at different temperatures. Thermo-viscoelastic experimental characterization and numerical modelling of VHB polymer were induced by Liao et al. [18].
The hypothesis of electro-magneto-thermo-viscoelasticity has stimulated a lot of enthusiasm for some mechanical applications especially in atomic gadget. Where there exists an essential magnetic field. Different examinations have been completed by thinking about the connection between attractive, magnetic, thermal and strain fields. Examinations of such issue additionally impact different applications in biomedical building just as in various geometric investigations. Fish et al. [19] has studied modeling and simulation of nonlinear electro-thermo-mechanical continua with application to shape memory polymeric medical devices.
Numerous applications of state space approach created for various sort of issues in electro-magneto-thermo-viscoelasticity [20,21,22,23,24,25,26,27,28,29,30,31,32,33,34].
We shall consider a homogeneous isotropic thermo-viscoelastic medium occupying the region R≪r<∞ of a perfect electrically conductivity permeated by an initial constant magnetic field Ho, where R is the radius of the shell.
Due to the effect of this magnetic field there arises in the conducting medium an induced magnetic field h and induced electric field E. Also, there arises a force F (the Lorentz Force). Due to the effect of this force, points of the medium undergo a displacementu, which gives rise to a temperature.
The linearized equations of electromagnetism for slowly moving media are:
curlh=J+ϵo∂E∂t | (1) |
curlE=−μo∂E∂t | (2) |
B=μoH | (3) |
divB=0 | (4) |
The above field equations are supplemented by constitutive equations which consist first of modified ohm's law:
E=−μo∂u∂t×Ho+kogradθ | (5) |
The second constitutive equation is the one for the Lorenz force which is
F=J×B | (6) |
The third constitutive equation is the stress-displacement-temperature relation for viscoelastic medium of Kelvin–Voigt type:
σij=2μe(1+α1∂∂t)eij+λe(1+αo∂∂t)eδij−γe(1+γo∂∂t)θδij | (7) |
The equation of motion is given by:
ρ∂2ui∂2t=(2μe(1+α1∂∂t)+λe(1+αo∂∂t))uj,ij−γe(1+γo∂∂t)θ,i+μo(J×Ho) | (8) |
The generalized heat conduction equation is given by
Kθ,ii+K∗˙θ,ii=ρCE¨θ+γeTo(1+γo∂∂t)¨e+πodivJ | (9) |
The strain displacement relation is given by
eij=12(ui,j+uj,i) | (10) |
Together with the previous equations, constitute a complete system of generalized-magneto-thermo-viscoelasticity equations for a medium with a perfect conductivity.
Let (r,ψ,φ) denote the radial coordinates, the co-latitude, and the longitude of a spherical coordinates system, respectively. Due to spherical symmetry, all the considered function will be functions of r and t only.
The components of the displacement vector will be taken the form:
ur=u(r,t), uψ=uφ=0 | (11) |
The strain tensor components are thus given by
err=∂u∂r,eψψ=eφφ=ur,erφ=eφψ=0 | (12) |
e=∂u∂r+2ur=1r2∂(r2u)∂r | (13) |
From Eq 7 we obtain the components of the stress tensor as
σrr=2μe(1+α1∂∂t)∂u∂r+λe(1+αo∂∂t)e−γe(1+γo∂∂t)θ | (14) |
σφφ=σψψ=2μe(1+α1∂∂t)ur+λe(1+αo∂∂t)e−γe(1+γo∂∂t)θ | (15) |
σrφ=σrψ=σψφ=0 | (16) |
Assume now that the initial magnetic field acts in the φ-direction and has the components(0,0,Ho). The induced magnetic field h will have one component h in the φ-direction, while the induced electric field E will have one component E in the ψ-direction.
Then, Eqs 1, 2 and 5 yield
J=Ho∂e∂r+koμo∂θ∂r | (17) |
h=−Ho(∂u∂r+ur)−koμo∂θ∂r | (18) |
E=μoHo∂u∂t+ko∂θ∂r | (19) |
From Eqs 17 and 6, we get that the Lorentz force has only one component Fr in the r-direction:
Fr=μoH2o∂e∂r+koHo∂θ∂r | (20) |
Also, we arrived at
ρ∂2u∂t2=(2μe(1+α1∂∂t)+2λe(1+αo∂∂t)+μoH2o)∂e∂r−γe(1+γo∂∂t)∂e∂r+koHo∂θ∂r | (21) |
Equation 21 is to be supplemented by the constitutive Eq 13 and the heat conduction equation
K∇2θ+K∗∇2˙θ=ρCE¨θ+γeTo(1+γo∂∂t)¨e+πodivJ | (22) |
Where ∇2 is Laplaces operator in spherical coordinates which is given by
∇2=1r2∂∂r(r2∂∂r)+1r2sinψ(sinψ∂∂ψ)+1r2sin2ψ∂2∂φ2 | (23) |
In case of dependence on r only, this reduce to
∇2=1r2∂∂r(r2∂∂r) | (24) |
Now, we shall use the following non dimensional variables:
ˊr=C1ηr,ˊu=C1ηu,ˊt=C21ηt,´γo=C21ηγo,´αo=C21ηαo,´α1=C21ηα1, |
´σij=σijμe,ˊθ=θTo,ˊh=hHo,ˊE=EμoHoC1,ˊJ=JηHoC1 | (25) |
Equations 14–19, 21 and 22 take the following form (dropping the primes for convenience).
J=∂e∂r+A∂θ∂r | (26) |
h=−(∂u∂r+ur)−A∂θ∂r | (27) |
E=∂u∂t+A∂θ∂r | (28) |
σrr=2μeλe+2μe(1+α1∂∂t)∂u∂r+λeλe+2μe(1+αo∂∂t)eγeθoλe+2μe(1+γo∂∂t)θ | (29) |
σφφ=σψψ=2μeλe+2μe(1+α1∂∂t)ur+λeλe+2μe(1+αo∂∂t)e-γeθoλe+2μe(1+γo∂∂t)θ | (30) |
σrφ=σrψ=σφψ=0 | (31) |
∂2u∂t2=(1+A+μoH2o+λeαo+2μeα1ρC21∂∂t)∂e∂r−γeθoρC21(1+A+γo∂∂t)∂θ∂r | (32) |
∇2θ+K∗C21ηK∇2˙θ=ηC21¨θ+γeC21K(1+γo∂∂t)¨e | (33) |
Where, η=ρCEK,C21=λe+2μeρ, A=koμoHo.
Equation 32 can be written in the form:
¨e=(1+A+μoH2o+λeαo+2μeα1ρC21∂∂t)∇2e−γeθoρC21(1+A+γo∂∂t)∇2θ | (34) |
Taking the Laplace transform of Eqs 26–31, 33 and 34 by using homogeneous initial conditions, defined and denoted as
−f(s)∫∞0e−stf(t)dt,s>0 | (35) |
We obtain
−J=d−edr+Ad−θdr | (36) |
−h=−(d−udr+−ur)−Ad−θdr | (37) |
−E=s−u+Ad−θdr | (38) |
∇2−θ=L1−θ+L1−e | (39) |
−σrr=a1d−udr+a2−e−a3−θ | (40) |
−σφφ=−σθθ=a1−ur+a2−e−a3−θ | (41) |
∇2−e=M1−θ+M1−e | (42) |
where
L1=KηC21s2K+A+K∗ηC21s2,l2=γeC21(1+γos)s2K+A+K∗ηC21s2,a1=2μeλe+2μe(1+A+α1s), a2=λeλe+2μe(1+A+αos),
a3=γeθoλe+2μe(1+A+γos),M1=γeθo(1+A+γos)L1μoH2oρC21+ρC21+(λeαo+2μeα1)s, M1=ρC21s2+γeθo(1+A+γos)L2μoH2oρC21+ρC21+(λeαo+2μeα1)s.
Choosing as state variables the temperature of heat conduction −θand the strain components −e then Eqs 35 and 38 can be written in the matrix form
∇2V(r,s)=A(s)−V(r,s) | (43) |
where −V(r,s)=[−θ(r,s)−e(r,s)],A(s)=[L1L2M1M2].
The formal solution of Eq 43 can be written in the form
−V(r,s)=Ce−√A(s)rr+Be−√A(s)rr | (44) |
For bounded solution with large r, we have canceled the exponential part has positive power. And at r=R the value of C is given by C=R−V(R,s)e√A(s)R, then Eq 44 reduces to
−V(r,s)=Rr−V(R,s)e−√A(s)R,r≫R | (45) |
We will use the will-known Cayley–Hamiltonian theorem to find the form of the matrix exp(−√A(s)(r−R)). The characteristic equation of the matrix A(s) can be written as
k2−(L1+M2)k+(L1M2−L2M1) | (46) |
The roots of this equation namely k1 and k2 satisfy the relations
k1+k2=L1+M2 | (47) |
k1k2=L1M2−L2M1 | (48) |
The Tailor's series expansion for the matrix exponential of exp(−√A(s)(r−R)) is given by
exp(−√A(s)(r−R))=∑∞n=0[−√A(s)(r−R)]nn! | (49) |
Using Cayley–Hamiltonian theorem, we can express A2 and higher orders of the matrix A in terms of I and A where I is the unit matrix of second order.
Thus, the infinite series in Eq 49 can be reduced to
exp(−√A(s)(r−R))=bo(r,s)I+b1(r,s)A | (50) |
where bo and b1 are some coefficients depending on s and r.
By Cayley–Hamiltonian theorem, the characteristic roots k1 and k2 of the matrix A must satisfy Eq 50, thus we have
exp(−√k1(r−R))=bo+b1k1 | (51) |
exp(−√k2(r−R))=bo+b1k2 | (52) |
Solving the above linear system of equations, we get
bo=k1e−√k2(r−R)−k2e−√k1(r−R)k1−k2 | (53) |
b1=e−√k1(r−R)−e−√k2(r−R)k1−k2 | (54) |
Hence, we have
exp(−√A(s)(r−R))=Lij(r,s),i,j=1,2 | (55) |
where
L11=e−√k2(r−R)(k1−L1)+e−√k1(r−R)(L1−k2)k1−k2 | (56) |
L12=L2e−√k1(r−R)−L1e−√k2(r−R)k1−k2 | (57) |
L21=M1e−√k1(r−R)−M2e−√k1(r−R)k1−k2 | (58) |
L22=e−√k1(r−R)(M2−k2)+e−√k2(r−R)(k1−M2)k1−k2 | (59) |
In order to evaluate the unknown parameters−θo(r,s)and−eo(r,s), we shall use the boundary conditions on the internal surface of the shell, r=R which are given by:
(Ⅰ) Thermal boundary condition at r=R, θ(R,t)=θo
Taking the Laplace transform, this is defined as following:
−θo(r,s)=θos | (60) |
(Ⅱ) Mechanical boundary condition
The internal surface r=R has a rigid foundation, which is rigid enough to prevent any strain e(R,t)=0. Taking the Laplace transform, this is defined as following:
−e(R,s)=−eo=0 | (61) |
Using the Eq 60 and 61 into Eq 45 and using Eqs 56–59, we get
−θ(r,s)=Rθos(k1−k2)r[(k1−L2)e−√k2(r−R)+(k2−L1)e−√k2(r−R)] | (62) |
−e(r,s)=RM1θos(k1−k2)r[(k2−L1)e−√k1(r−R)+(k1−L2)e−√k2(r−R)] | (63) |
To find the displacement, taking Laplace transform for Eq 32 using Eqs 62 and 63, we get
−u(r,s)=Rθosr2(k1−k2)r(((1+r√k1)(B5(L1−k2))−B4M1)e−√k1(r−R)+((1+r√k2)(B4M1+B5(k1−L1)))e−√k2(r−R)) | (64) |
To find the radial stress, from Eq 40 and Eqs 62–64 we get
−σrr=Rθosr2(k1−k2)r{e−√k1(r−R)(M1(B1+B3)r2+2B1B4M1(1+r√k1)−(L1−k2)(B3r2+2B1B5(1+r√k1)))+e−√k2(r−R)(−M1(B1+B2)r2−2B1B4M1(1+r√k1)+(L1−k2)(B3r2+2B1B5(1+r√k2))+)} | (65) |
where B4=1s2+λeαo+2μeα1ρC21s2, B5=γeθo(1+γos)ρC21s2.
In order to invert the Laplace transforms in the above equations we shall use a numerical technique based on Fourier expansions of functions. Let −g(s) be the Laplace transform of a given function g(t). The inversion formula of Laplace transforms states that
g(t)=12πi∫d+i∞d−i∞est−g(s)ds | (66) |
where d is an arbitrary positive constant greater than all the real parts of the singularities of −g(s). Taking s=d+iy, we get
g(t)=edt2π∫∞−∞eiky−g(d+iy)dy | (67) |
This integral can be approximated by
g(t)=edt2π∑∞k=−∞eikt∆y−g(d+ik∆y)∆y | (68) |
Taking ∆y=πt1we obtain:
g(t)=edtt1(12−g(d)+Re(∑∞k=1eiktπ/t1−g(d+ikπ/t1))) | (69) |
For numerical purposes this is approximated by the function
gN(t)=edtt1(12−g(d)+Re(∑Nk=1eiktπ/t1−g(d+ikπ/t1))) | (70) |
where N is a sufficiently large integer chosen such that
edtt1Re(eiNπt/t1−g(d+iNπ/t1))<η | (71) |
where η is a reselected small positive number that corresponds to the degree of accuracy to be achieved Eq 69 is the numerical inversion formula valid for0≤t≤t1. In particular, we chooset=t1, getting
gN(t)=edtt1(12−g(d)+Re(∑Nk=1(−1)k−g(d+ikπ/t1))) | (72) |
The copper material was chosen for purposes of numerical evaluations and constants of the problem were taken as following (35) in SI units: K=386N/Ks,αt=17.8(10)−5K−1,CE=383.1m2/K,To=293K,ρ=8954kg/m2, μe=3.86(10)10N/m2,λe=7,76(10)10N/m2,α1=3.25(10)−2,αo=3.25(10)−2, R=1,θo=1.
In order to study the effect of time t and study the comparison between two models on temperature, radial stress, shear stress, displacement and strain, we now present our results in the form of graphs (Figures 2–9).
Green and Naghdi [7,8,9] proposed three new thermoelastic theories based on entropy equality rather than the usual entropy inequality. The constitutive assumptions for the heat flux vector are different in each theory. Thus, they obtained three theories they called thermoelasticity of type Ⅰ, thermoelasticity of type Ⅱ and thermoelasticity of type Ⅲ. When the type Ⅰ theory is linearized we obtain the classical system of thermo-elasticity. The type Ⅱ theory (is a limiting case of type Ⅲ) does not admit energy dissipation.
Figure 2 is plotted to show the variation of temperature θ against rfor wide range of r (1≤r≤3) at small time(t=0.07) for two theories (G-N Ⅲ) and (G-N Ⅱ). It is observed from this figure the magnitude of the temperature is greater for (G-N Ⅲ) model than (G-N Ⅱ). It can be noted that the speed of propagation of temperature is finite and coincide with the physical behavior of viscoelastic material. Also, we can see from this figure that the boundary condition (60) is satisfied.
Figure 3 shows variation of temperature θ for the coefficient of Ohm and Fourier laws. It is noticed that the modified Fourier and Ohm laws influence is significant, the temperature in the modified model records value higher than these in the old model.
Figure 4 is plotted to show the variation of the radial stress σrr against rfor wide range of r(1≤r≤10), at small time(t=0.07), for two theories (G-N Ⅲ) and (G-N Ⅱ). It is observed from this figure the magnitude of the radial stress is greater for (G-N Ⅲ) model than (G-N Ⅱ). It can be noted that the speed of propagation of stress is finite and coincide with the physical behavior of viscoelastic material.
Figure 5 shows variation of radial stress for the coefficient of Ohm and Fourier laws. It is noticed that the modified Fourier's and Ohm's model effects on the radial stress by increasing their values.
Figure 6 is plotted to show the variation of strain eagainst rfor wide range of(1≤r≤3), at small time(t=0.07), for two theories (G-N Ⅲ) and (G-N Ⅱ). It is observed from this figure the magnitude of the strain is greater for (G-N Ⅲ) model than (G-N Ⅱ). It can be noted that the speed of propagation of strain is finite and coincide with the physical behavior of viscoelastic material. Also, we can see from this figure that the boundary condition (61) is satisfied.
Figure 7 shows variation of strain e for the coefficient of Ohm and Fourier laws. It is noticed that the modified Fourier and Ohm laws influence is significant, the strain in the modified model records value higher than these in the old model.
Figure 8 is plotted to show the variation of displacement uagainst rfor wide range of(1≤r≤3), at small time(t=0.07), for two theories (G-N Ⅲ) and (G-N Ⅱ). It is observed from this figure the magnitude of the displacement is greater for (G-N Ⅲ) model than (G-N Ⅱ). It can be noted that the speed of propagation of displacement is finite and coincide with the physical behavior of viscoelastic material.
Figure 9 shows the variation of displacement uagainst rfor wide range ofr (1≤r≤3), for different values of time(t=0.0,t=0.5,t=0.1). And we have noticed that, the time thas significant effects on displacementu. The increasing of the value of tcauses increasing of the value of displacement u, and displacement uvanishes more rapidly.
Green and Naghdi [7,8,9] developed a generalized theory of thermoelasticity which involves thermal displacement gradient as one of the constitutive variables in contrast to the classical coupled thermoelasticity which includes temperature gradient as one of the constitutive variables. An important feature of this theory is that it does not accommodate dissipation of thermal energy. On this theory the characterization of material response to a thermal phenomenon is based on three types of constitutive response functions. The nature of those three types of constitutive response functions is such that when the respective theories are linearized, type Ⅰ is same as classical heat conduction equation (based on Fourier's law), whereas type Ⅱ, the internal rate of production of entropy is taken to be identically zero, implying no dissipation of thermal energy. This model is known as the theory of thermoelasticity without energy dissipation. Type Ⅲ involves the previous two models as special cases, and admits dissipation of energy in general, in this model, introducing the temperature gradient and thermal displacement gradient as the constitutive variables.
From the above discussion, one can reason that the new model of generalized magneto-thermo-viscoelasticity predicts new qualities for the temperature, displacement, stresses and strain. The impact of the temperature gradient at adequately low temperature may cause new sorts of magneto-thermo-viscoelastic wave with explicit stability properties. The expansion in the estimations of temperature might be clarified as the lost heat producing from the development of electric flow; this heat might be the fundamental motivation behind why the deformation of the medium will in general be ordinary and the magnetic field records esteems more prominent than the qualities in the old model. As indicated by this work, numerous specialists in the field of generalized thermo elasticity have applied Green–Naghdi hypothesis (Ⅲ-Ⅱ) for thermo elastic problem and not many of them can effectively applied for magneto thermo Viscoelastic issue. Right now conclude that the greatness of the every single physical amount is more noteworthy for (G-N Ⅲ) model than (G-N Ⅱ). It very well may be noticed that the speed of spread of every single physical amount is limited and match with the physical conduct of Viscoelastic material.
The authors wish to acknowledge the approval and the support of this research study by the Project NO. SCI-2018-3-9-F-7795 from the Deanship of Scientific Research in Northern Border University, Arar, Saudi Arabia.
The authors declare that they have no known competing financial interests or personal relationships that could have appeared to influence the work reported in this paper.
[1] |
Biot MA (1956) Thermo elasticity and irreversible thermo-dynamics. J Appl Phys 27: 240-253. doi: 10.1063/1.1722351
![]() |
[2] | Lord HW, Shulman Y (1976) A generalized dynamical theory of thermo elasticity. J Mech Phys Solid 15: 299-309. |
[3] |
Müller MM, Kaiser E, Bauer P, et al. (1976) Lipid composition of the rat kidney. Nephron 17: 41-50. doi: 10.1159/000180709
![]() |
[4] |
Green AE, Laws N (1972) On the entropy production inequality. Arch Ration Mech An 45: 47-53. doi: 10.1007/BF00253395
![]() |
[5] |
Green AE, Lindsay KA (1972) Thermo elasticity. J Elasticity 2: 1-7. doi: 10.1007/BF00045689
![]() |
[6] | Shuhubi E (1957) Thermo elastic solid, In: Eringen AC, Continuum Physics, New York: Academic Press. |
[7] | Green AE, Naghdi PM (1991) A re-examination of the basic postulate of thermo-mechanics. P Roy Soc A-Math Phy 432: 171-194. |
[8] |
Green AE, Naghdi PM (1993) Thermoelasticity without energy dissipation. J Elasticity 31: 189-208. doi: 10.1007/BF00044969
![]() |
[9] |
Green AE, Naghdi PM (1992) An unbounded heat wave in an elastic solid. J Therm Stresses 15: 253-264. doi: 10.1080/01495739208946136
![]() |
[10] | Illyushin AA, Pobedria BE (1970) Fundamentals of the mathematical theory of thermal viscoelasticity. |
[11] |
Biot MA (1954) Theory of stress-strain relations in anisotropic viscoelasticity and relaxation phenomena. J Appl Phys 25: 1385-1391. doi: 10.1063/1.1721573
![]() |
[12] |
Biot MA (1955) Variational principles in irreversible thermodynamics with application to viscoelasticity. Phys Rev 97: 1463-1469. doi: 10.1103/PhysRev.97.1463
![]() |
[13] | Morland LW, Lee EH (1960) Stress analysis for linear viscoelastic materials with temperature variation. J Rheol 4: 233-263. |
[14] | Tanner RI (1988) Engineering Rheology, Oxford: Oxford University Press. |
[15] |
Drozdov AD (1996) A constitutive model in thermoviscoelasticity. Mech Res Commun 23: 543-548. doi: 10.1016/0093-6413(96)00055-9
![]() |
[16] | Bland DR (1960) The Theory of Linear Viscoelasticity, Oxford: Pergamon Press. |
[17] |
Lion A (1997) On the large deformation behavior of reinforced rubber at different temperatures. J Mech Phys Solids 45: 1805-1834. doi: 10.1016/S0022-5096(97)00028-8
![]() |
[18] |
Liao Z, Hossain M, Yao X, et al. (2020) Thermo-viscoelastic experimental characterization and numerical modelling of VHB polymer. Int J Nonlin Mech 118: 103263. doi: 10.1016/j.ijnonlinmec.2019.103263
![]() |
[19] |
Niyonzima I, Jiao Y, Fish J (2019) Modeling and simulation of nonlinear electro-thermo-mechanical continua with application to shape memory polymeric medical devices. Comput Method Appl M 350: 511-534. doi: 10.1016/j.cma.2019.03.003
![]() |
[20] |
Mehnert M, Hossain M, Steinmann P (2017) Towards a thermo-magneto-mechanical coupling framework for magneto-rheological elastomers. Int J Solids Struct 128: 117-132. doi: 10.1016/j.ijsolstr.2017.08.022
![]() |
[21] |
Mehnert M, Hossain M, Steinmann P (2016) On nonlinear thermo-electro-elasticity. P Roy Soc A-Math Phys 472: 20160170. doi: 10.1098/rspa.2016.0170
![]() |
[22] |
Youssef HM, El-Bary AA, Elsibai KA (2014) Vibration of gold nano beam in context of two-temperature generalized thermoelasticity subjected to laser pulse. Lat Am J Solids Stru 11: 2460-2482. doi: 10.1590/S1679-78252014001300008
![]() |
[23] |
Ezzat MA, El-Bary AA (2014) Two-temperature theory of magneto-thermo-viscoelasticity with fractional derivative and integral orders heat transfer. J Electromagnet Wave 28: 1985-2004. doi: 10.1080/09205071.2014.953639
![]() |
[24] | Ezzat MA, El-Bary AA (2015) State space approach to two-dimensional magneto-thermoelasticity with fractional order heat transfer in a medium of perfect conductivity. Int J Appl Electrom 49: 607-625. |
[25] |
Ismail MAH, Khamis AK, El-Bary AA, et al. (2017) Effect of rotation of generalized thermoelastic layer subjected to harmonic heat: state-space approach. Microsyst Technol 23: 3381-3388. doi: 10.1007/s00542-016-3137-3
![]() |
[26] |
Khamis AK, Ismail AH, Youssef HM, et al. (2017) Thermal shock problem of two-temperature generalized thermoelasticity without energy dissipation with rotation. Microsyst Technol 23: 4831-4839. doi: 10.1007/s00542-017-3279-y
![]() |
[27] |
Youssef HM, Elsibai KA, El-Bary AA (2017) Effect of the speed, the rotation and the magnetic field on the Q-factor of an axially clamped gold micro-beam. Meccanica 52: 1685-1694. doi: 10.1007/s11012-016-0498-8
![]() |
[28] |
Ezzat MA, El-Karamany AS, El-Bary AA (2017) Thermoelectric viscoelastic materials with memory-dependent derivative. Smart Struct Sys 19: 539-551. doi: 10.12989/sss.2017.19.5.539
![]() |
[29] |
El-Karamany AS, Ezzat MA, El-Bary AA (2018) Thermodiffusion with two time delays and Kernel functions. Math Mech Solids 23: 195-208. doi: 10.1177/1081286516676870
![]() |
[30] |
Ezzat MA, El-Bary AA (2018) Thermoelectric spherical shell with fractional order heat transfer. Microsyst Technol 24: 891-899. doi: 10.1007/s00542-017-3400-2
![]() |
[31] |
El-Bary AA, Atef H (2016) On effect of viscous fractional parameter on infinite thermo Viscoelastic medium with a spherical cavity. Journal of computational and theoretical. Nanoscience 13: 1-5. doi: 10.1166/jctn.2016.4099
![]() |
[32] |
El-Bary AA, Atef M (2016) Modified approach for stress strain equation in the linear Kelvin-Voigt solid based on fractional order. J Comput Theor Nanos 13: 1027-1036. doi: 10.1166/jctn.2016.4332
![]() |
[33] |
Amin MM, El-Bary A, Atef H (2018) Effect of viscous fractional parameter on generalized magneto thermo-viscoelastic thin slim strip exposed to moving heat source. Mater Focus 7: 814-823. doi: 10.1166/mat.2018.1591
![]() |
[34] |
Amin MM, El-Bary AA, Atef HM (2018) Modification of Kelvin-Voigt model in fractional order for thermoviscoelastic isotropic material. Mater Focus 7: 824-832. doi: 10.1166/mat.2018.1592
![]() |
[35] |
Sharma K, Kumar P (2013) Propagation of plane waves and fundamental solution in thermoviscoelastic medium with voids. J Therm Stresses 36: 94-111. doi: 10.1080/01495739.2012.720545
![]() |
1. | Farshad Shakeriaski, Maryam Ghodrat, Juan Escobedo-Diaz, Masud Behnia, Recent advances in generalized thermoelasticity theory and the modified models: a review, 2021, 8, 2288-5048, 15, 10.1093/jcde/qwaa082 | |
2. | Pooja Kadian, Sunil Kumar, Neelam Hooda, Monika Sangwan, Reflection of plane waves in a non-local viscothermoelastic half-space under variable thermal conductivity and microelongation effects, 2024, 0149-5739, 1, 10.1080/01495739.2024.2415025 | |
3. | Manuel Rivas, Manuel Reina, Covariant Formulation of the Brain’s Emerging Ohm’s Law, 2024, 16, 2073-8994, 1570, 10.3390/sym16121570 |