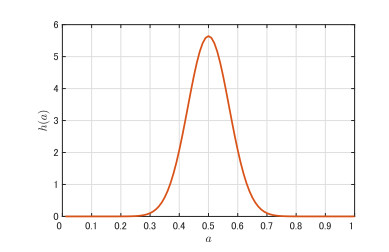
Citation: Jacqueline Jerney, Magdalena Mayr, Michael Schagerl. Biofilm scrubbing for restoration—algae community composition and succession in artificial streams[J]. AIMS Environmental Science, 2016, 3(3): 560-581. doi: 10.3934/environsci.2016.3.560
[1] | A. Vinodkumar, T. Senthilkumar, S. Hariharan, J. Alzabut . Exponential stabilization of fixed and random time impulsive delay differential system with applications. Mathematical Biosciences and Engineering, 2021, 18(3): 2384-2400. doi: 10.3934/mbe.2021121 |
[2] | Eduardo Liz, Alfonso Ruiz-Herrera . Delayed population models with Allee effects and exploitation. Mathematical Biosciences and Engineering, 2015, 12(1): 83-97. doi: 10.3934/mbe.2015.12.83 |
[3] | Paolo Fergola, Marianna Cerasuolo, Edoardo Beretta . An allelopathic competition model with quorum sensing and delayed toxicant production. Mathematical Biosciences and Engineering, 2006, 3(1): 37-50. doi: 10.3934/mbe.2006.3.37 |
[4] | Yoichi Enatsu, Yukihiko Nakata, Yoshiaki Muroya . Global stability for a class of discrete SIR epidemic models. Mathematical Biosciences and Engineering, 2010, 7(2): 347-361. doi: 10.3934/mbe.2010.7.347 |
[5] | Xinran Zhou, Long Zhang, Tao Zheng, Hong-li Li, Zhidong Teng . Global stability for a class of HIV virus-to-cell dynamical model with Beddington-DeAngelis functional response and distributed time delay. Mathematical Biosciences and Engineering, 2020, 17(5): 4527-4543. doi: 10.3934/mbe.2020250 |
[6] | Hongjing Shi, Wanbiao Ma . An improved model of t cell development in the thymus and its stability analysis. Mathematical Biosciences and Engineering, 2006, 3(1): 237-248. doi: 10.3934/mbe.2006.3.237 |
[7] | Marion Weedermann . Analysis of a model for the effects of an external toxin on anaerobic digestion. Mathematical Biosciences and Engineering, 2012, 9(2): 445-459. doi: 10.3934/mbe.2012.9.445 |
[8] | Kalyan Manna, Malay Banerjee . Stability of Hopf-bifurcating limit cycles in a diffusion-driven prey-predator system with Allee effect and time delay. Mathematical Biosciences and Engineering, 2019, 16(4): 2411-2446. doi: 10.3934/mbe.2019121 |
[9] | C. Connell McCluskey . Global stability of an SIR epidemic model with delay and general nonlinear incidence. Mathematical Biosciences and Engineering, 2010, 7(4): 837-850. doi: 10.3934/mbe.2010.7.837 |
[10] | Jinliang Wang, Hongying Shu . Global analysis on a class of multi-group SEIR model with latency and relapse. Mathematical Biosciences and Engineering, 2016, 13(1): 209-225. doi: 10.3934/mbe.2016.13.209 |
In this paper, we study the following general class of functional differential equations with distributed delay and bistable nonlinearity,
{x′(t)=−f(x(t))+∫τ0h(a)g(x(t−a))da,t>0,x(t)=ϕ(t),−τ≤t≤0. | (1.1) |
Many mathematical models issued from ecology, population dynamics and other scientific fields take the form of Eq (1.1) (see, e.g., [1,2,3,4,5,6,7,8,9,10,11,12,13,14,15] and references therein). When a spatial diffusion is considered, many models are studied in the literature (see, e.g., [16,17,18,19,20,21,22]).
For the case where g(x)=f(x) has not only the trivial equilibrium but also a unique positive equilibrium, Eq (1.1) is said to be a problem with monostable nonlinearity. In this framework, many authors studied problem (1.1) with various monostable nonlinearities such as Blowflies equations, where f(x)=μx and g(x)=βxe−αx, and Mackey-Glass equations, where f(x)=μx and g(x)=βx/(α+xn) (see, e.g., [1,3,6,7,9,10,11,12,13,21,23,24,25,26,27]).
When g(x) allows Eq (1.1) to have two positive equilibria x∗1 and x∗2 in addition to the trivial equilibrium, Eq (1.1) is said to be a problem with bistable nonlinearity. In this case, Huang et al. [5] investigated the following general equation:
x′(t)=−f(x(t))+g(x(t−τ)). |
The authors described the basins of attraction of equilibria and obtained a series of invariant intervals using the decomposition domain. Their results were applied to models with Allee effect, that is, f(x)=μx and g(x)=βx2e−αx. We point out that the authors in [5] only proved the global stability for x∗2≤M, where g(M)=maxs∈R+g(s). In this paper, we are interested in the dynamics of the bistable nonlinearity problem (1.1). More precisely, we will present some attracting intervals which will enable us to give general conditions on f and g that ensure global asymptotic stability of equilibria x∗1 and x∗2 in the both cases x∗2<M and x∗2≥M.
The paper is organized as follows: in Section 2, we give some preliminary results including existence, uniqueness and boundedness of the solution as well as a comparison result. We finish this section by proving the global asymptotic stability of the trivial equilibrium. Section 3 is devoted to establish the attractive intervals of solutions and to prove the global asymptotic stability of the positive equilibrium x∗2. In Section 4, we investigate an application of our results to a model with Allee effect. In Section 5, we perform numerical simulation that supports our theoretical results. Finally, Section 6 is devoted to the conclusion.
In the whole paper, we suppose that the function h is positive and
∫τ0h(a)da=1. |
We give now some standard assumptions.
(T1) f and g are Lipschitz continuous with f(0)=g(0)=0 and there exists a number B>0 such that maxv∈[0,s]g(v)<f(s) for all s>B.
(T2) f′(s)>0 for all s≥0.
(T3) g(s)>0 for all s>0 and there exists a unique M>0 such that g′(s)>0 for 0<s<M, g′(M)=0 and g′(s)<0 for s>M.
(T4) There exist two positive constants x∗1 and x∗2 such that g(x)<f(x) if x∈(0,x∗1)∪(x∗2,∞) and g(x)>f(x) if x∈(x∗1,x∗2) and g′(x∗1)>f′(x∗1).
Let C:=C([−τ,0],R) be the Banach space of continuous functions defined in [−τ,0] with ||ϕ||=supθ∈[−τ,0]|ϕ(θ)| and C+={ϕ∈C;ϕ(θ)≥0,−τ≤θ≤0} is the positive cone of C. Then, (C,C+) is a strongly ordered Banach space. That is, for all ϕ,ψ∈C, we write ϕ≥ψ if ϕ−ψ∈C+, ϕ>ψ if ϕ−ψ∈C+∖{0} and ϕ>>ψ if ϕ−ψ∈Int(C+). We define the following ordered interval:
C[ϕ,ψ]:={ξ∈C;ϕ≤ξ≤ψ}. |
For any χ∈R, we write χ∗ for the element of C satisfying χ∗(θ)=χ for all θ∈[−τ,0]. The segment xt∈C of a solution is defined by the relation xt(θ)=x(t+θ), where θ∈[−τ,0] and t≥0. In particular, x0=ϕ. The family of maps
Φ:[0,∞)×C+→C+, |
such that
(t,ϕ)→xt(ϕ) |
defines a continuous semiflow on C+ [28]. For each t≥0, the map Φ(t,.) is defined from C+ to C+ which is denoted by Φt:
Φt(ϕ)=Φ(t,ϕ). |
The set of equilibria of the semiflow, which is generated by (1.1), is given by
E={χ∗∈C+;χ∈Randg(χ)=f(χ)}. |
In this section, we first provide existence, uniqueness and boundedness of solution to problem (1.1). We then present a Lyapunov functional and show the global asymptotic stability of the trivial equilibrium. We begin by recalling a useful theorem related to a comparison principle (see Theorem 1.1 in page 78 in [29]).
We consider the following problem:
{x′(t)=F(xt),t>0,x(t)=ϕ(t),−τ≤t≤0, | (2.1) |
where F:Ω→R is continuous on Ω, which is an open subset of C. We write x(t,ϕ,F) for the maximal defined solution of problem (2.1). When we need to emphasize the dependence of a solution on initial data, we write x(t,ϕ) or x(ϕ).
Theorem 2.1. Let f1, f2 :Ω→R be continuous, Lipschitz on each compact subset of Ω, and assume that either f1 or f2 is a nondecreasing function, with f1(ϕ)≤f2(ϕ) for all ϕ∈Ω. Then
x(t,ϕ,f1)≤x(t,ϕ,f2), |
holds for all t≥0, for which both are defined.
The following lemma states existence, uniqueness and boundedness of the positive solution to problem (1.1). For the proof, see [15,30,31].
Lemma 2.2. Suppose that (T1) holds. For any ϕ∈C+, the problem (1.1) has a unique positive solution x(t):=x(t,ϕ) on [0,∞) satisfying x0=ϕ, provided ϕ(0)>0. In addition, we have the following estimate:
0≤lim supt→∞x(t)≤B. |
Moreover, the semiflow Φt admits a compact global attractor, which attracts every bounded set in C+.
The following lemma can be easily proved (see also the proof of Theorem 1.1 in [29]).
Lemma 2.3. Let ϕ∈C be a given initial condition, x(ϕ) be the solution of problem (1.1) and xϵ(ϕ), ϵ>0 be the solution of problem (1.1) when replacing f by f±ϵ. Then
xϵ(t,ϕ)→x(t,ϕ) as ϵ→0, for all t∈[0,∞). |
We focus now on the global stability of the trivial equilibrium. For this purpose, we suppose that
f(s)>g(s) for all s∈(0,x∗1) and f(x∗1)=g(x∗1). | (2.2) |
Lemma 2.4. Assume that (T1) and condition (2.2) hold. For a given ϕ∈C[0,x∗1]∖{x∗1}, the solution x(ϕ) of problem (1.1) satisfies
lim supt→∞x(t)<x∗1, | (2.3) |
provided that one of the following hypotheses holds:
(i) g is a nondecreasing function over (0,x∗1).
(ii) f is a nondecreasing function over (0,x∗1).
Proof. Without loss of generality, we assume that ϕ(0)<x∗1. Suppose that (ⅰ) holds. We first claim that x(t)<x∗1 for all t≥0. Let xϵ(ϕ) be the solution of problem (1.1) by replacing f by f+ϵ. We prove that xϵ(t):=xϵ(t,ϕ)≤x∗1 for all t>0. Suppose, on the contrary, that there exists t1>0 such that xϵ(t1)=x∗1, xϵ(t)≤x∗1 for all t≤t1 and x′ϵ(t1)≥0. Then, using the equation of xϵ(t1), we have
0≤x′ϵ(t1)=−f(xϵ(t1))−ϵ+∫τ0h(a)g(xϵ(t1−a))da,<−f(x∗1)+g(x∗1)=0, |
which leads to a contradiction. Now, applying Lemma 2.3, we obtain x(t)≤x∗1 for all t≥0. Next, set X(t)=x∗1−x(t). We then have
X′(t)=f(x(t))−f(x∗1)+∫τ0h(a)(g(x∗1)−g(x(t−a)))da. |
Since x(t)≤x∗1 for all t≥0 and g is a nondecreasing function, we have, for t≥τ,
X′(t)≥−LX(t), |
where L is the Lipschitz constant of f. Consequently, X(t)≥X(0)e−Lt and the claim is proved. Finally, to prove inequality (2.3), we suppose, on the contrary, that there exist an increasing sequence (tn)n, tn→∞, t0≥τ and a nondecreasing sequence (x(tn))n, such that x(tn)→x∗1 as tn→∞, x(tn)<x∗1 for some n and x(tn)=maxt∈[0,tn]x(t). Then, in view of condition (2.2), the equation of x(tn) satisfies
0≤x′(tn)=−f(x(tn))+∫τ0h(a)g(x(tn−a))da,≤−f(x(tn))+g(x(tn))<0, |
which is a contradiction.
Suppose now that (ⅱ) holds. Let xϵ(t) be the solution of problem (1.1) by replacing f(s) by f(s)+ϵ and g(s) by g+(s)=maxσ∈[0,s]g(σ).
Then, again by contradiction, suppose that there exists t1>0 such that xϵ(t1)=x∗1, xϵ(t)≤x∗1 for all t≤t1 and x′ϵ(t1)≥0. Then, arguing as above, we obtain
0≤x′ϵ(t1)=−f(xϵ(t1))−ϵ+∫τ0h(a)g+(xϵ(t−a))da,<−f(x∗1)+g+(x∗1). | (2.4) |
Since f is a nondecreasing function and g+ is the smallest nondecreasing function that is greater than g, we obtain f(s)>g+(s) for all s∈(0,x∗1). This contradicts with inequality (2.4). Further, by combining Theorem 2.1 and Lemma 2.3, we get x(t)≤x∗1 for all t≥0. Finally, following the same arguments as in the first part of this proof, the lemma is proved.
We now prove the global asymptotic stability of the trivial equilibrium.
Theorem 2.5. Assume that (T1) and condition (2.2) hold. Suppose also that ϕ∈C[0,x∗1]∖{x∗1}. The trivial equilibrium is globally asymptotically stable if one of the following hypotheses holds:
(i) g is a nondecreasing function over (0,x∗1).
(ii) f is a nondecreasing function over (0,x∗1).
Proof. Suppose that (ⅰ) holds. Let V be the Lyapunov functional defined by
V(s)=s+∫τ0ψ(a)g(ϕ(−a))da, | (2.5) |
where ψ(a)=∫τah(σ)dσ. The derivative of V along the solution of problem (1.1) gives
dV(xt)dt=g(x(t))−f(x(t))∀t>0. |
From condition (2.2) and Lemma 2.4, we have dV(xt)/dt<0 and thus, the result is reached by classical Lyapunov theorem (see [6]). Next, suppose that (ⅱ) holds. Let the function V be defined in (2.5) by replacing g(s) by g+(s):=maxσ∈[0,s]g(σ). Since g+(s)<f(s) for all s∈(0,x∗1), we can employ the same argument as above to get dV(xt)/dt<0. Finally the result is obtained by applying Theorem 2.1 and Lemma 2.4. This completes the proof.
Now, suppose that
f(s)>g(s) for all s>0. | (2.6) |
Using the same proof as in Theorem 2.5, we immediately obtain the following theorem.
Theorem 2.6. Assume that (T1) and condition (2.6) hold. The trivial equilibrium is globally asymptotically stable for all ϕ∈C+.
The following theorem concerns the global stability of x∗2 in the case where g is a nondecreasing function.
Theorem 3.1. Suppose that ϕ∈C[x∗1,sups∈[−τ,0]ϕ]∖{x∗1} and g is a nondecreasing function. Assume also that (T1) and (T4) hold. Then, the positive equilibrium x∗2 is globally asymptotically stable.
Proof. Without loss of generality, suppose that ϕ(0)>x∗1. We first claim that lim inft→∞x(t)>x∗1. For this, let xϵ(t):=xϵ(t;ϕ) be the solution of problem (1.1) when replacing f by f−ϵ. To reach the claim, we begin by proving that xϵ(t)>x∗1 for all t≥0. Otherwise, there exists t1>0 such that xϵ(t1)=x∗1, xϵ(t)≥x∗1 for all t≤t1 and x′ϵ(t1)≤0. Then, the equation of xϵ(t1) satisfies
0≥x′ϵ(t1)=−f(xϵ(t1))+ϵ+∫τ0h(a)g(xϵ(t1−a))da>−f(x∗1)+g(x∗1)=0. |
This reaches a contradiction and thus xϵ(t)>x∗1 for all t>0. This result, together with Lemma 2.3, gives x(t)≥x∗1 for all t≥0.
Next, for X(t)=x(t)−x∗1 and since f(x∗1)=g(x∗1), the equation of X(t) satisfies
X′(t)=f(x∗1)−f(x(t))+∫τ0h(a)(g(x(t−a))−g(x∗1))da, |
this leads to
X′(t)≥−LX(t), |
where L is the Lipschitz constant of f. Consequently x(t)>x∗1 for all t>0.
Now, suppose that there exist an increasing sequence (tn)n, tn→∞, t0≥τ and a nonincreasing sequence (x(tn))n, such that x(tn)→x∗1 as tn→∞, x(tn)>x∗1 for some n and x(tn)=mint∈[0,tn]x(t). In view of (T4), the equation of x(tn) satisfies
0≥x′(tn)=−f(x(tn))+∫τ0h(a)g(x(tn−a))da,≥−f(x(tn))+g(x(tn))>0, |
which is a contradiction. The claim is proved.
To prove that x∗2 is globally asymptotically stable, we consider the following Lyapunov functional
V(ϕ)=∫ϕ(0)x∗2[g(s)−g(x∗2)]ds+12∫τ0ψ(a)[g(ϕ(−a))−g(x∗2)]2da, |
with ψ(a)=∫τah(σ)dσ. By a straightforward computation, the derivative of V along the solution of problem (1.1) gives, for all t>0,
dV(xt)dt=[g(x(t))−g(x∗2)]x′(t)−12∫τ0ψ(a)∂∂a[g(x(t−a))−g(x∗2)]2da=[g(x(t))−g(x∗2)][−f(x(t))+∫τ0h(a)g(x(t−a))da]−12{ψ(τ)[g(x(t−τ))−g(x∗2)]2−ψ(0)[g(x(t))−g(x∗2)]2−∫τ0ψ′(a)[g(x(t−a))−g(x∗2)]2da}=[g(x(t))−g(x∗2)][−f(x(t))+g(x(t))]+[g(x(t))−g(x∗2)][−g(x(t))+∫τ0h(a)g(x(t−a))da]+12∫τ0h(a)da[g(x(t))−g(x∗2)]2−12∫τ0h(a)[g(x(t−a))−g(x∗2)]2da=[g(x(t))−g(x∗2)][−f(x(t))+g(x(t))]+12∫τ0h(a){2[g(x(t))−g(x∗2)][−g(x(t))+g(x(t−a))]+[g(x(t))−g(x∗2)]2−[g(x(t−a))−g(x∗2)]2}da=[g(x(t))−g(x∗2)][−f(x(t))+g(x(t))]−12∫τ0h(a)[g(x(t))−g(x(t−a))]2da. |
Note that ψ(τ)=0. In view of (T4), we have dV(xt)/dt≤0. If g is an increasing function, then the result is reached by using a classical Lyapunov theorem (see, e.g., [30]). If g is a nondecreasing function, then the result is proved by using the same argument as in the proof of Theorem 2.6 in [31]. This completes the proof.
We focus now on the case where g is non-monotone. Suppose that there exists ˆG(x) such that ˆG(x)=ˆg−1og(x), where ˆg(.) denotes the restriction of g to the interval [M,∞). Then, ˆG(x)=x for x∈[M,∞) and ˆG(x)>M>x for x∈[0,M).
Lemma 3.2. Assume that (T1)–(T4) hold. For a given ϕ∈C[x∗1,ˆG(x∗1)]∖{x∗1}, let x be the solution of problem (1.1). Then, the following assertions hold:
(i) if x∗2<M, then x∗1<x(t)<ˆG(x∗1) for all t>0.
(ii) if x∗2≥M and f(ˆG(x∗1))>g(M), then x∗1<x(t)<ˆG(x∗1) for all t>0.
Proof. Without loss of generality, suppose that x∗1<ϕ(0)≤ˆG(x∗1). First, observe that, for a given ϕ∈C[x∗1,ˆG(x∗1)]∖{x∗1}, we have f(ˆG(x∗1))>f(x∗1)=g(x∗1)=g(ˆG(x∗1)), and thus, there exists ϵ>0 such that f(ˆG(x∗1))−ϵ>g(ˆG(x∗1)). We begin by proving that x∗1≤x(t)≤ˆG(x∗1) for all t>0. To this end, in view of Lemma 2.3, we only need to prove that x∗1<xϵ(t):=xϵ(t;ϕ)≤ˆG(x∗1) for all t>0, where xϵ(t):=xϵ(t;ϕ) is the solution of problem (1.1) when replacing f by f−ϵ. Let yϵ:=yϵ(ϕ) be the solution of
{y′ϵ(t)=−f(yϵ(t))+ϵ+∫τ0h(a)g+(yϵ(t−a))da,t>0,yϵ(t)=ϕ(t),−τ≤t≤0. | (3.1) |
with g+(s)=maxσ∈[0,s]g(σ). Since f is an increasing function, we have f(ˆG(x∗1))−ϵ>g+(ˆG(x∗1)). Accordingly, the function ˆG(x∗1) is a super-solution of problem (3.1). Finally, in view of Theorem 2.1, we obtain xϵ(t)≤yϵ(t)≤ˆG(x∗1) for all t≥0.
We now prove that xϵ(t)>x∗1. Suppose, on the contrary, that there exists t1>0 such that xϵ(t1)=x∗1, xϵ(t)≥x∗1 for all t≤t1, and thus, x′ϵ(t1)≤0. Then, the equation of xϵ(t1) satisfies
x′ϵ(t1)=−f(xϵ(t1))+ϵ+∫τ0h(a)g(xϵ(t1−a))da>−f(x∗1)+g(x∗1)=0, |
since g(s)≥g(x∗1) for all s∈[x∗1,ˆG(x∗1)]. This reaches a contradiction. Further, from Lemma 2.3, we obtain x(t)≥x∗1. The claim is proved.
Next, let X(t)=x(t)−x∗1. Since f(x∗1)=g(x∗1), the equation of X(t) satisfies
X′(t)=f(x∗1)−f(x(t))+∫τ0h(a)(g(x(t−a))−g(x∗1))da. |
We know that g(s)≥g(x∗1) for all s∈[x∗1,ˆG(x∗1)]. We then have
X′(t)≥−LX(t), |
where L is the Lipschitz constant of f. Consequently x(t)>x∗1 for all t>0.
Next, we prove that x(t)<ˆG(x∗1) for all t>0. Suppose, on the contrary, that there exists t1>0 such that x(t1)=ˆG(x∗1), x(t)≤ˆG(x∗1) for all t≤t1 and x′(t1)≥0. Then
0≤x′(t1)=−f(x(t1))+∫τ0h(a)g(x(t1−a))da,≤−f(ˆG(x∗1))+g(M). | (3.2) |
Since f is an increasing function and ˆG(x∗1)>M, we have
0≤x′(t1)≤−f(M)+g(M). | (3.3) |
Now, the assertion x∗2<M implies that g(M)<f(M), which leads to a contradiction.
When x∗2≥M, inequality (3.2) gives a contradiction by hypothesis. The Lemma is proved.
Using Lemma 3.2, we next prove the following lemma.
Lemma 3.3. Suppose that ϕ∈C[x∗1,ˆG(x∗1)]∖{x∗1}. Assume also that (T1)–(T4) hold. Let x be the solution of problem (1.1). Then, we have
lim inft→∞x(t)>x∗1, |
provided that one of the following assertions holds:
(i) x∗2<M.
(ii) x∗2≥M and f(ˆG(x∗1))>g(M).
Proof. Firstly, from Lemma 3.2, both assertions imply that x∗1<x(t)<ˆG(x∗1) for all t≥0. Next, observe that there exists ϵ>0 such that
g(s)>f(x∗1+ϵ)for alls∈[x∗1+ϵ,θϵ], |
with θϵ∈(M,ˆG(x∗1)) and g(θϵ)=g(x∗1+ϵ). Indeed, in view of (T3), minσ∈[x∗1+ϵ,θϵ]g(σ)=g(θϵ)=g(x∗1+ϵ) and from (T4), we have g(x∗1+ϵ)>f(x∗1+ϵ).
Now, consider the following nondecreasing function:
g_(s)={g(s),for 0<s<ˉm,f(x∗1+ϵ),for ˉm<s<ˆG(x∗1), | (3.4) |
where x∗1<ˉm<x∗1+ϵ, which is a constant satisfying g(ˉm)=f(x∗1+ϵ). From (T2) and (T4), we get g_(s)>f(s) for x∗1<s<x∗1+ϵ and g_(s)<f(s) for s>x∗1+ϵ. Let y(ϕ) be the solution of problem (1.1) when replacing g by g_. Then, according to Theorem 2.1, we have y(t;ϕ)≤x(t;ϕ). In addition, using Theorem 3.1, we obtain
x∗1<limt→∞y(t;ϕ)=x∗1+ϵ≤lim inft→∞x(t;ϕ). |
The lemma is proved.
Under Lemma 3.3, we prove the following theorem on the global asymptotic stability of the positive equilibrium x∗2<M.
Theorem 3.4. Suppose that ϕ∈C[x∗1,ˆG(x∗1)]∖{x∗1} and x∗2<M. Assume also that (T1)–(T4) hold. Then, the positive equilibrium x∗2 is globally asymptotically stable.
Proof. We first claim that there exists T>0 such that x(t)≤M for all t≥T. Indeed, let xϵ:=xϵ(ϕ) be the solution of problem (1.1) when replacing f by f+ϵ. First, suppose that there exists T>0 such that xϵ(t)≥M for all t≥T. So, since x∗2<M, we have from (T4) that g(M)<f(M). Combining this with (T2), the equation of xϵ satisfies
x′ϵ(t)≤−f(M)−ϵ+g(M)≤−ϵ, |
which contradicts with xϵ(t)≥M. Hence there exists T>0 such that xϵ(T)<M. We show that xϵ(t)<M for all t≥T. In fact, at the contrary, if there exists t1>T such that xϵ(t1)=M and so x′ϵ(t1)≥0 then,
x′ϵ(t1)=−f(M)−ϵ+g(M)<0, |
which is a contradiction. Further, according to Lemma 2.3, there exists T>0 such that x(t)≤M for all t≥T and the claim is proved. Finally, since g is a nondecreasing function over (0,M), the global asymptotic stability of x∗2 is proved by applying Theorem 3.1.
Remark 3.5. In the case where g(s)>g(x∗1) for all s>x∗1, the above theorem holds true. In fact, it suffices to replace ˆG(x∗1) in C[x∗1,ˆG(x∗1)]∖{x∗1} by sups∈[−τ,0]ϕ(s).
We focus now on the case where x∗2≥M. To this end, suppose that there exists a unique constant A such that
A≥M and g(A)=f(M). | (3.5) |
Lemma 3.6. Assume that (T1)–(T4) hold. Suppose also that x∗2≥M and f(A)≥g(M). For a given ϕ∈C[x∗1,ˆG(x∗1)]∖{x∗1} and the solution x:=x(ϕ) of problem (1.1), there exists T>0 such that
M≤x(t)≤A for all t≥T. |
Proof. We begin by claiming that x∗2≤A. On the contrary, suppose that x∗2>A≥M. Then, due to (T2) and (T3), we have g(x∗2)<g(A)=f(M)<f(x∗2)=g(x∗2), which is a contradiction. The claim is proved.
Next, for a given ϕ∈C[x∗1,ˆG(x∗1)]∖{x∗1}, let xϵ:=xϵ(ϕ) be the solution of problem (1.1) when replacing f by f+ϵ. Since xϵ converges to x as ϵ tends to zero, we only need to prove that there exists T>0 such that xϵ(t)<A for all t≥T. To this end, let yϵ:=yϵ(ϕ) be the solution of problem (1.1), when replacing f by f+ϵ and g by g+ with g+(s)=maxσ∈[0,s]g(σ). By Theorem 2.1, we have xϵ(t)≤yϵ(t) for all t≥0, and thus, we only need to show that yϵ(t)<A for all t≥T. On the contrary, we suppose that yϵ(t)≥A for all t>0. Then, combining the equation of yϵ and the fact that g+(s)=g(M) for all s≥M, we obtain
y′ϵ(t)≤g(M)−f(A)−ϵ<0, |
which is a contradiction. Then, there exists T>0 such that yϵ(T)<A. We further claim that yϵ(t)<A for all t≥T. Otherwise, there exists t1>T such that yϵ(t1)=A and y′ϵ(t1)≥0. Substituting yϵ(t1) in Eq (1.1), we get
0≤y′ϵ(t1)=−f(yϵ(t1))−ϵ+∫τ0h(a)g+(yϵ(t1−a))da,<−f(A)+g(M)≤0, |
which is a contradiction. Consequently, by passing to the limit in ϵ, we obtain that x(t)≤A for all t≥T.
We focus now on the lower bound of x. First, define the function
g_(s)={g(s),for 0<s<ˉm,f(M),for ˉm<s≤A, | (3.6) |
where x∗1<ˉm<M, which is the constant satisfying g(ˉm)=f(M). Note that, due to (T2)–(T4) and condition (3.5), the function g_ is nondecreasing over (0,A) and satisfies
{g_(s)≤g(s),0≤s≤A,g_(s)>f(s),x∗1<s<M,g_(s)<f(s),M<s≤A. |
Let y(ϕ) be the solution of problem (1.1) when replacing g by g_. From Theorem 2.1, we have y(t;ϕ)≤x(t;ϕ) for all t>0, and from Theorem 3.1, we have limt→∞y(t;ϕ)=M. Consequently, lim inft→∞x(t)≥M. In addition, if, for all T>0, there exists (tn)n such that tn>T, y(tn)=M, ˉm<y(s)≤A for all 0<s≤tn and y′(tn)<0, then, for tn>T+τ,
y′(tn)=−f(M)+f(M)=0, |
which is a contradiction. The lemma is established.
Denote ˉf=f|[M,A] the restriction function of f over [M,A] and G(s):=ˉf−1(g(s)) for s∈[M,A]. Now, we are ready to state our main theorem related to x∗2≥M.
Theorem 3.7. Under the assumptions of Lemma 3.6, the positive equilibrium x∗2 of problem (1.1) is globally asymptotically stable, provided that one of the following conditions holds:
(H1) fg is a nondecreasing function on [M,A].
(H2) f+g is a nondecreasing function over [M,A].
(H3) (GoG)(s)s is a nonincreasing function over [M,x∗2],
(H4) (GoG)(s)s is a nonincreasing function over [x∗2,A],
Proof. Denote x∞:=lim inft→∞x(t) and x∞:=lim supt→∞x(t). First, suppose that either x∞≤x∗2 or x∞≥x∗2. For x∞≤x∗2, we introduce the following function:
g_(s)={minσ∈[s,x∗2]g(σ)forx∗1<s<x∗2,g(x∗2)forx∗2≤s≤A. |
Let y(ϕ) be the solution of problem (1.1) when replacing g by g_. Since g_(s)≤g(s) for all x∗1≤s≤x∗2, we have y(t)≤x(t) for all t>0. Further, in view of Theorem 3.1, we obtain
limt→∞y(t)=x∗2≤lim supt→∞x(t)=x∞≤x∗2. |
The local stability is obtained by using the same idea as in the proof of Theorem 2.6 in [31].
Now, for x∞≥x∗2, we introduce the function
ˉg(s)={minσ∈[s,x∗2]g(σ)forx∗1<s≤x∗2,maxσ∈[x∗2,s]g(σ)forx∗2<s≤A, | (3.7) |
and let y(ϕ) be the solution of problem (1.1) when replacing g by ˉg. As above, we have x(t)≤y(t) for all t>0 and y(t) converges to x∗2 as t goes to infinity. Next, we suppose that x∞<x∗2<x∞ and we prove that it is impossible. Indeed, according to Lemma 3.6, we know that, for every solution x of problem (1.1), we have M≤x∞≤x∞≤A.
Now, using the fluctuation method (see [28,32]), there exist two sequences tn→∞ and sn→∞ such that
limn→∞x(tn)=x∞, x′(tn)=0, ∀n≥1, |
and
limn→∞x(sn)=x∞, x′(sn)=0, ∀n≥1. |
Substituting x(tn) in problem (1.1), it follows that
0=−f(x(tn))+∫τ0h(a)g(x(tn−a))da. | (3.8) |
Since g is nonincreasing over [M,A], we obtain, by passing to the limit in Eq (3.8), that
f(x∞)≤g(x∞). | (3.9) |
Similarly, we obtain
f(x∞)≥g(x∞). | (3.10) |
Multiplying the expression (3.9) by g(x∞) and combining with inequality (3.10), we get
f(x∞)g(x∞)≤f(x∞)g(x∞). |
This fact, together with the hypothesis (H1), gives x∞≤x∞, which is a contradiction. In a similar way, we can conclude the contradiction for (H2). Now, suppose that (H3) holds. First, notice that G makes sense, that is, for all s∈[M,A], the range of g is contained in [ˉf(M),ˉf(A)] since ˉf is strictly increasing over [M,A]. In fact, for all s∈[M,A] and since g is non-increasing over [M,A], we have g(A)≤g(s)≤g(M). Now, using the fact that f(A)≥f(M), we show that
ˉf(M)≤g(s)≤ˉf(A)for alls∈[M,A]. |
Therefore, the function G is nonincreasing and maps [M,A] to [M,A]. In view of inequalities (3.9) and (3.10) and the monotonicity of ˉf, we arrive at
x∞≤G(x∞), | (3.11) |
and
x∞≥G(x∞), | (3.12) |
with G(s)=ˉf−1(g(s)). Now, applying the function G to inequalities (3.11) and (3.12), we find
x∞≥G(x∞)≥(GoG)(x∞), |
which gives
(GoG)(x∞)x∞≤1=(GoG)(x∗2)x∗2. | (3.13) |
Due to (H3), it ensures that x∗2≤x∞, which is impossible. Using the same arguments as above, we obtain a contradiction for (H4). The theorem is proved.
Remark 3.8. In the case where g(s)>g(A) for all s>M, the two above results hold true. In fact, it suffices to replace A in Lemma 3.6 and Theorem 3.7 by B defined in (T1).
For the tangential case where two positive equilibria x∗1 and x∗2 are equal, we have the following theorem
Theorem 3.9. Suppose that (T1)–(T3) hold. Suppose that, in addition to the trivial equilibrium, problem (1.1) has a unique positive equilibrium x∗1. Then
(i) for ϕ∈C+, there exists T>0 such that 0≤x(t)≤M for all t≥T.
(ii) ϕ∈C[x∗1,ˆG(x∗1)]∖{x∗1} implies that x∗1<x(t)≤M for all t≥T.
(iii) if ϕ∈C[x∗1,ˆG(x∗1)], then x∗1 attracts every solution of problem (1.1) and x∗1 is unstable.
Proof. The uniqueness of the positive equilibrium implies that x∗1≤M and g(x)<f(x) for all x≠x∗1. For (ⅰ), suppose that there exists t0>0 such that x(t)≥M for all t≥t0. Then, by substituting x in Eq (1.1), we get
x′(t)≤−f(M)+g(M)<0. |
This is impossible and then there exists T>0 such that x(T)<M. Next, if there exists t1>T such that x(t1)=M and x(t)≤M for all t≤t1, then
0≤x′(t1)≤−f(M)+g(M)<0. |
Consequently (ⅰ) holds. We argue as in the proof of Lemma 3.2 (ⅰ), to show (ⅱ). Concerning (ⅲ), we consider the following Lyapunov functional
V(ϕ)=∫ϕ(0)x∗1(g(s)−g(x∗1))ds+12∫τ0ψ(a)(g(ϕ(−a))−g(x∗1))2da, |
where ψ(a)=∫τah(σ)dσ. As in the proof of Theorem 3.1, the derivative of V along the solution of problem (1.1) gives
dV(xt)dt=−12∫τ0h(a)[g(x(t))−g(x(t−a))]2da+[g(x(t))−g(x∗1)][g(x(t))−f(x(t))],∀t>0. |
Since g(s)<f(s) for all s≠x∗1 and g(s)≥g(x∗1) for all s∈[x∗1,M], we have dV(xt)/dt≤0. By LaSalle invariance theorem, x∗1 attracts every solution x(ϕ) of problem (1.1) with ϕ∈C[x∗1,ˆG(x∗1)]. From Theorems 2.5 and 3.4, we easily show that x1 is unstable.
In this section, we apply our results to the following distributed delay differential equation:
x′(t)=−μx(t)+∫τ0h(a)kx2(t−a)1+2x3(t−a)da, for t≥0, | (4.1) |
where μ, k are positive constants. The variable x(t) stands for the maturated population at time t and τ>0 is the maximal maturation time of the species under consideration. h(a) is the maturity rate at age a. In this model, the death function f(x)=μx and the birth function g(x)=kx2/(1+2x3) reflect the so called Allee effect. Obviously, the functions f and g satisfy the assumptions (T1)–(T3) and g reaches the maximum value k/3 at the point M=1. The equilibria of Eq (4.1) satisfies the following equation:
μx=kx21+2x3. | (4.2) |
Analyzing Eq (4.2), we obtain the following proposition.
Proposition 4.1. Equation (4.1) has a trivial equilibrium x=0. In addition
(i) if μ>2133k, then Eq (4.1) has no positive equilibrium;
(ii) if μ=2133k, then Eq (4.1) has exactly one positive equilibrium x∗1;
(iii) if 0<μ<2133k, then Eq (4.1) has exactly two positive equilibria x∗1<x∗2. Moreover,
(iii)-a if k3<μ<2133k, then 0<x∗1<x∗2<1;
(iii)-b if 0<μ≤k3, then 0<x∗1<1≤x∗2.
Using Theorem 2.6, we obtain
Theorem 4.2. If μ>2133k, then the trivial equilibrium of Eq (4.1) is globally asymptotically stable for all ϕ∈C+.
For the case (ⅲ)-a in Proposition 4.1 we have the following result
Theorem 4.3. Suppose that k3<μ<2133k. Then
(i) The trivial equilibrium of Eq (4.1) is globally asymptotically stable for all ϕ∈C[0,x∗1]∖{x∗1}.
(ii) The positive equilibrium x∗1 is unstable and the positive equilibrium x∗2 is globally asymptotically stable for all ϕ∈C[x∗1,ˆG(x∗1)]∖{x∗1}, where ˆG(x∗1)∈[1,∞) satisfies g(ˆG(x∗1))=g(x∗1).
(iii) There exists two heteroclinic orbits X(1) and X(2) connecting 0 to x∗1 and x∗1 to x∗2, respectively.
Proof. (ⅰ) and (ⅱ) directly follow from Proposition 4.1, (ⅲ)-a and Theorems 2.5 and 3.4.
For (ⅲ), we follow the same proof as in Theorem 4.2 in [5]. See also [18]. For the sake of completeness, we rewrite it. Let K={x∗1}. Clearly, K is an isolated and unstable compact invariant set in C[0,x∗1] and C[x∗1,ˆG(x∗1)].
By applying Corollary 2.9 in [33] to Φ|R+×C[0,x∗1] and Φ|R+×C[x∗1,ˆG(x∗1)], respectively, there exist two pre-compact full orbits X(1):R→C[0,x∗1]∖{0} and X(2):R→C[x∗1,ˆG(x∗1)]∖{0} such that α(X1)=α(X2)=K. This together with statements (i) and (ii) gives ω(X(1))={0} and ω(X(2))={x∗2}. In other words, there exist two heteroclinic orbits X(1) and X(2), which connect 0 to x∗1 and x∗1 to x∗2, respectively. This completes the proof.
For the case (ⅲ)-b in Proposition 4.1, we first show that
f(A)≥g(M) with g(A)=f(M) and A≥M. | (4.3) |
Lemma 4.4. Suppose that f(s)=μs. Condition (4.3) holds if and only if M≤1μg(1μg(M)).
Proof. It follows that f(A)≥g(M) if and only if A≥f−1(g(M))≥M. Since g is a decreasing function over [M,∞), we have g(A)≤g(f−1(g(M)))=g(1μ(g(M))). Moreover, since g(A)=f(M), we have M≤1μg(1μg(M)). The lemma is proved.
Lemma 4.5. For Eq (4.1), condition (4.3) holds if and only if
0<μ≤k3. | (4.4) |
Proof. By a straightforward computation, we have f−1(x)=x/μ. For G(x)=g(x)/μ, we obtain
(GoG)(x)=x(2px6+px3)8x9+(12+2p)x6+6x3+1, |
where p=k3/μ3. By applying Lemma 4.4, it is readily to see that 1≤(GoG)(1) holds if and only if
1≤3p2p+27, |
and thus, 0<μ≤k/3.
Theorem 4.6. Suppose that 0<μ≤k3. Then, the positive equilibrium x∗2 of Eq (4.1) is globally asymptotically stable for all ϕ∈C[x∗1,ˆG(x∗1)]∖{x∗1}, where ˆG(x∗1)∈[1,∞) satisfies g(ˆG(x∗1))=g(x∗1).
Proof. Observe that, in view of Lemmas 4.4 and 4.5, all hypotheses of Lemma 3.6 are satisfied. Now, in order to apply Theorem 3.7, we only show that (GoG)(x)x is nonincreasing over [M,A]. Indeed, by a simple computation, we have
((GoG)(x)x)′=−48px14−48px11−6p2x8+12px5+3px2[8x9+(12+2p)x6+6x3+1]2, |
where p=k3/μ3. Finally, observe that
((GoG)(x)x)′<0, |
for x≥1. In this case, both of (H3) and (H4) in Theorem 3.7 hold. This completes the proof.
Finally, for the tangential case, we have
Theorem 4.7. Suppose that μ=2133k. Then
(i) The trivial equilibrium of Eq (4.1) is globally asymptotically stable for all ϕ∈C[0,x∗1]∖{x∗1}.
(ii) If ϕ∈C[x∗1,ˆG(x∗1)]∖{x∗1}, then the unique positive equilibrium x∗1 is unstable and attracts every solution of Eq (4.1).
Proof. The proof of this theorem follows immediately from Theorems 2.5 and 3.9.
In this section, we perform numerical simulation that supports our theoretical results. As in Section 4, we assume that
f(x)=μx,g(x)=kx21+2x3, |
and confirm the validity of Theorems 4.2, 4.3, 4.6 and 4.7. In what follows, we fix
τ=1,k=1,h(a)=e−(a−0.5τ)2×102∫τ0e−(σ−0.5τ)2×102dσ | (5.1) |
and change μ and ϕ. Note that 2133k≈0.42 and h is a Gaussian-like distribution as shown in Figure 1.
First, let μ=0.5. In this case, μ>2133k and thus, by Theorem 4.2, the trivial equilibrium is globally asymptotically stable. In fact, Figure 2 shows that x(t) converges to 0 as t increases for different initial data.
Next, let μ=0.37. In this case, k3<μ<2133k and x∗1≈0.43, x∗2≈0.89 and ˆG(x∗1)≈3.09 (Figure 3). Hence, by Theorem 4.3, we see that the trivial equilibrium is globally asymptotically stable for ϕ∈C[0,x∗1]∖{x∗1}, whereas the positive equilibrium x∗2 is so for ϕ∈C[x∗1,ˆG(x∗1)]∖{x∗1}. In fact, Figure 4 shows such a bistable situation. Moreover, heteroclinic orbits X(1) and X(2), which were stated in Theorem 4.3 (ⅲ), are shown in Figure 5.
Thirdly, let μ=0.27<k3≈0.33. In this case, we have x∗1≈0.28, x∗2≈1.2 and ˆG(x∗1)≈6.52 (Figure 6). Thus, by Theorem 4.6, we see that the positive equilibrium x∗2 is globally asymptotically stable for ϕ∈C[x∗1,ˆG(x∗1)]∖{x∗1}. In fact, Figure 7 shows that x(t) converges to x∗2 as t increases for different initial data.
Finally, let μ=2133k≈0.42. In this case, we have x∗1≈0.63 and ˆG(x∗1)≈1.72 (Figure 8). By Theorem 4.7, we see that the trivial equilibrium is globally asymptotically stable for ϕ∈C[0,x∗1]∖{x∗1}, whereas x∗1 is unstable and attracts all solutions for ϕ∈C[x∗1,ˆG(x∗1)]∖{x∗1}. In fact, Figure 9 shows such two situations.
In this paper, we studied the bistable nonlinearity problem for a general class of functional differential equations with distributed delay, which includes many mathematical models in biology and ecology. In contrast to the previous work [5], we considered both cases x∗2<M and x∗2≥M, and obtained sufficient conditions for the global asymptotic stability of each equilibrium. The general results were applied to a model with Allee effect in Section 4, and numerical simulation was performed in Section 5. It should be pointed out that our theoretical results are robust for the variation of the form of the distribution function h. This might suggest us that the distributed delay is not essential for the dynamical system of our model. We conjecture that our results still hold for τ=∞, and we leave it for a future study. Extension of our results to a reaction-diffusion equation could also be an interesting future problem.
We deeply appreciate the editor and the anonymous reviewers for their helpful comments to the earlier version of our manuscript. The first author is partially supported by the JSPS Grant-in-Aid for Early-Career Scientists (No.19K14594). The second author is partially supported by the DGRSDT, ALGERIA.
All authors declare no conflicts of interest in this paper.
[1] | Correll DL (1998) The Role of Phosphorus in the Eutrophication of Receiving Waters: A Review. J Environ Qual 27: 261-266. |
[2] | Dokulil MT, Teubner K (2011) Eutrophication and Climate Change: Present Situation and Future Scenarios. Dordrecht Heidelberg London New York: Springer, 1-16. |
[3] | Fleming-Lehtinen V, Andersen JH, Carstensen J, et al. (2015) Recent developments in assessment methodology reveal that the Baltic Sea eutrophication problem is expanding. Ecol Indic 48: 380-388. |
[4] | Graham LE, Graham JM, Wilcox LW (2009) Algae. 2.ed. San Francisco: Benjamin/Cummings. |
[5] |
Shen PP, Shi Q, Hua ZC, et al. (2003) Analysis of microcystins in cyanobacteria blooms and surface water samples from Meiliang Bay, Taihu Lake, China. Environ Int 29: 641-647. doi: 10.1016/S0160-4120(03)00047-3
![]() |
[6] |
Falconer IR, Humpage AR (2005) Health Risk Assessment of Cyanobacterial (Blue-green Algal) Toxins in Drinking Water. Int J Environ Res Public Health 2: 43-50. doi: 10.3390/ijerph2005010043
![]() |
[7] |
Ruuhijarvi J, Rask M, Vesala S, et al. (2010) Recovery of the fish community and changes in the lower trophic levels in a eutrophic lake after a winter kill of fish. Hydrobiologia 646: 145-158. doi: 10.1007/s10750-010-0186-y
![]() |
[8] | Lampert W, Sommer U (1999) Limnoökologie. Stuttgart: Thieme. |
[9] | Stadler GA, Wehdorn M (2007) Architektur im Verbund. Vienna: Springer. |
[10] | Donabaum K, Donabaum U, Großschartner M, et al. (2011) Sanierung Heustadelwasser—Monitoring 2011–project report. Vienna, 113. |
[11] | Adey WH, Loveland K (1991) Dynamic aquaria—building living ecosystems. San Diego: Academic Press. |
[12] |
Sabater S, Guasch H, Romani A, et al. (2002) The effect of biological factors on the efficiency of river biofilms in improving water quality. Hydrobiologia 469: 149-156. doi: 10.1023/A:1015549404082
![]() |
[13] |
Hoffmann JP (1998) Wastewater treatment with suspended and nonsuspended algae. J Phycol 34: 757-763. doi: 10.1046/j.1529-8817.1998.340757.x
![]() |
[14] |
Powell N, Shilton A, Chisti Y, et al. (2009) Towards a luxury uptake process via microalgae - defining the polyphosphate dynamics. Water res 43: 4207-4213. doi: 10.1016/j.watres.2009.06.011
![]() |
[15] |
Hartley AM, House WA, Callow ME, et al. (1997) Coprecipitation of phosphate with calcite in the presence of photosynthesizing green algae. Water Res 31: 2261-2268. doi: 10.1016/S0043-1354(97)00103-6
![]() |
[16] | Adey W, Luckett C, Jensen K (1993) Phosphorus removal from natural waters using controlled algal production. Restor Ecol 1: 29-39. |
[17] |
D’Aiuto PE, Patt JM, Albano JP, et al. (2015) Algal turf scrubbers: Periphyton production and nutrient recovery on a South Florida citrus farm. Ecol Eng 75: 404-412. doi: 10.1016/j.ecoleng.2014.11.054
![]() |
[18] |
Mayr M, Jerney J, Schagerl M (2015) Combating planktonic algae with benthic algae. Ecol Eng 74: 310-318. doi: 10.1016/j.ecoleng.2014.10.034
![]() |
[19] |
Adey WH, Luckett C, Smith M (1996) Purification of industrially contaminated groundwaters using controlled ecosystems. Ecol Eng 7: 191-212. doi: 10.1016/0925-8574(96)00008-0
![]() |
[20] |
Craggs RJ, Adey WH, Jessup BK, et al. (1996) A controlled stream mesocosm for tertiary treatment of sewage. Ecol Eng 6: 149-169. doi: 10.1016/0925-8574(95)00056-9
![]() |
[21] |
Mulbry W, Kangas P, Kondrad S (2010) Toward scrubbing the bay: Nutrient removal using small algal turf scrubbers on Chesapeake Bay tributaries. Ecol Eng 36: 536-541. doi: 10.1016/j.ecoleng.2009.11.026
![]() |
[22] |
Mulbry W, Westhead EK, Pizarro C, et al. (2005) Recycling of manure nutrients: use of algal biomass from dairy manure treatment as a slow release fertilizer. Bioresour Technol 96: 451-458. doi: 10.1016/j.biortech.2004.05.026
![]() |
[23] |
Chisti Y (2007) Biodiesel from microalgae. Biotechnol Adv 25: 294-306. doi: 10.1016/j.biotechadv.2007.02.001
![]() |
[24] |
Douglas B (1958) The ecology of the attached diatoms and other algae in a small stony stream. J Ecol 46: 295-322. doi: 10.2307/2257397
![]() |
[25] | Wright SW, Jeffrey SW, Mantoura RFC, et al. (1991) Improved HPLC method for the analysis of chlorophylls and carotenoids from marine phytoplankton. Mar Ecol Prog Ser 77: 183-196. |
[26] | Jeffrey SW (1997) Phytoplankton pigments in oceanography: guidelines to modern methods. Paris: Unesco Publishing. |
[27] |
Mackey MD, Mackey DJ, Higgins HW, et al. (1996) CHEMTAX- A program for estimating class abundances from chemical markers: application to HPLC measurements of phytoplankton pigments. Mar Ecol Progr Ser 144: 265-283. doi: 10.3354/meps144265
![]() |
[28] | Pfister P, Pipp E (2010) Leitfaden zur Erhebung der biologischen Qualitätselemente. Teil A3 - Phytobenthos. Vienna: Bundesministerium für Land- und Forstwirtschaft, Umwelt und Wasserwirtschaft, Eigenverlag. |
[29] | Krammer K, Lange-Bertalot H (1986) Naviculaceae. Bacillariophyceae - Band 2/1. Stuttgart: G. Fischer. |
[30] | Krammer K, Lange-Bertalot H (1988) Bacillariaceae, Epithemiaceae, Surirellaceae. Bacillariophyceae - Band 2/2. Stuttgart: G.Fischer. |
[31] | Krammer K, Lange-Bertalot H, Achnanthaceae. Kritische Ergänzungen zu Navicula (Lineolatae) und Gomphonema; Gesamtliteraturverzeichnis. G Fischer. |
[32] | Krammer K, Lange-Bertalot H (2004) Centrales, Fragilariaceae, Eunotiaceae. Bacillariophyceae - Band 2/3. Stuttgart: G Fischer. |
[33] | Ettl H (1983) Phytomonadina, Chlorophyta - Band 9. Stuttgart: G. Fischer. |
[34] | Ettl H, Gärtner G (1988) Tetrasporales, Chlorococcales, Gloeodendrales – Band 10. Chlorophyta. Stuttgart: G. Fischer. |
[35] | Kadłubowska JZ (1984) Zygnemales. Chlorophyta - Band 16. Stuttgart: G. Fischer. |
[36] | Mrozińska T (1985) Oedogoniophyceae: Oedogoniales, Chlorophyta VI - Band 14. Stuttgart: G. Fischer. |
[37] | Pascher A HF (1930) Die Süsswasser-Flora Mitteleuropas. Jena: Verlag von Gustav Fischer. |
[38] | Starmach K (1985) Chrysophyceae und Haptophyceae. Stuttgart: Fischer. |
[39] | Lenzenweger R (1996) Desmidiaceenflora von Österreich, Teil 1. Berlin: Cramer. |
[40] | Lenzenweger R (1997) Desmidiaceenflora von Österreich, Teil 2. Berlin: Cramer. |
[41] | Lenzenweger R (1999) Desmidiaceenflora von Österreich, Teil 3. Berlin: Cramer. |
[42] | Geitler L (1930) Cyanophyceae (von Europa, unter Berücks. d. anderen Kontinente). Leipzig: Akad. Verl. Ges. |
[43] | Komárek J, Anagnostidis K (2005) Oscillatoriales. Cyanoprokaryota - Band 19/2. Stuttgart: G. Fischer. |
[44] | Komárek J, Anagnostidis K (1999) Chroococcales. Cyanoprokaryota - Band 19/1. Stuttgart: Fischer. |
[45] | Popovský J, Pfiester LA (2008) Dinophyceae (Dinoflagellida)—Band 6. Jena: Spektrum. |
[46] | Rieth A (1980) Xanthophyceae, 2. Teil - Band 4. Stuttgart: G. Fischer. |
[47] | Ettl H (1978) Xanthophyceae, 1. Teil - Band 3. Stuttgart: G. Fischer. |
[48] | Smith TM, Smith RL (2009) Ökologie. Czech Republic, Munich, Boston: Pearson Education Deutschland GmbH. |
[49] | Krebs CJ (1986) Ecology : the experimental analysis of distribution and abundance. New York: Harper & Row. |
[50] | McCune B, Mefford MJ (2006) PC-ORD. Multivariate Analysis of Ecological Data. 5 ed. Gleneden Beach, Oregon, U.S.A.: MjM Software. |
[51] | Schönhuber M (2006) Kieselalgengemeinschaften von ausgewählten Kärntner Seen. Klagenfurt. |
[52] |
Adey WH, Laughinghouse HD, Miller JB, et al. (2013) Algal turf scrubber (ATS) floways on the Great Wicomico River, Chesapeake Bay: productivity, algal community structure, substrate and chemistry 1. J Phycol 49: 489-501. doi: 10.1111/jpy.12056
![]() |
[53] |
Tamm M, Freiberg R, Tõnno I, et al. (2015) Pigment-Based Chemotaxonomy—A Quick Alternative to Determine Algal Assemblages in Large Shallow Eutrophic Lake? PLoS ONE 10: e0122526. doi: 10.1371/journal.pone.0122526
![]() |
[54] |
Chen N, Li J, Wu Y, et al. (2015) Nutrient removal at a drinking water reservoir in China with an algal floway. Ecol Eng 84: 506-514. doi: 10.1016/j.ecoleng.2015.09.049
![]() |
[55] |
Sindelar HR, Yap JN, Boyer TH, et al. (2015) Algae scrubbers for phosphorus removal in impaired waters. Ecol Eng 85: 144-158. doi: 10.1016/j.ecoleng.2015.09.002
![]() |
[56] |
Ray NE, Terlizzi DE, Kangas PC (2015) Nitrogen and phosphorus removal by the Algal Turf Scrubber at an oyster aquaculture facility. Ecol Eng 78: 27-32. doi: 10.1016/j.ecoleng.2014.04.028
![]() |
[57] |
Hoagland KD, Roemer SC, Rosowski JR, et al. (1982) Colonization and community structure of two periphyton assemblages , with emphasis on the diatoms (Bacillariophyceae). Am J Bot 69: 188-213. doi: 10.2307/2443006
![]() |
[58] |
Malkin SY, Sorichetti RJ, Wiklund JA, et al. (2009) Seasonal abundance, community composition, and silica content of diatoms epiphytic on Cladophora glomerata. J Great Lakes Res 35: 199-205. doi: 10.1016/j.jglr.2008.12.008
![]() |
[59] |
Peterson CG, Stevenson RJ (1992) Resistance and resilience of lotic algal communities—importance of distrurbance timing and current. Ecology 73: 1445-1461. doi: 10.2307/1940689
![]() |
[60] | Stevenson RJ, Bothwell ML, Lowe RL (1996) Algal ecology: Freshwater benthic ecosystems, 788. |
[61] |
McCormick PV, Stevenson RJ (1991) Mechanisms of benthic algal sucession in lotic environments. Ecology 72: 1835-1848. doi: 10.2307/1940982
![]() |
[62] |
Larson Ca, Passy SI, Laanbroek R (2012) Taxonomic and functional composition of the algal benthos exhibits similar successional trends in response to nutrient supply and current velocity. FEMS microbiol ecol 80: 352-362. doi: 10.1111/j.1574-6941.2012.01302.x
![]() |
[63] |
Cardinale BJ (2011) Biodiversity improves water quality through niche partitioning. Nature 472:86-89. doi: 10.1038/nature09904
![]() |
[64] | Jackson CR (2003) Changes in community properties during microbial succession. Oikos 2: 444-448. |
[65] | Pandit SN, Kolasa J (2011) Opposite effects of environmental variability and species richness on temporal turnover of species in a complex habitat mosaic. Hydrobiologia 685: 145-154. |
[66] |
Blersch DM, Kangas PC, Mulbry WW (2013) Turbulence and nutrient interactions that control benthic algal production in an engineered cultivation raceway. Algal Res 2: 107-112. doi: 10.1016/j.algal.2013.01.001
![]() |
1. | Arda Işıldar, Eric D. van Hullebusch, Markus Lenz, Gijs Du Laing, Alessandra Marra, Alessandra Cesaro, Sandeep Panda, Ata Akcil, Mehmet Ali Kucuker, Kerstin Kuchta, Biotechnological strategies for the recovery of valuable and critical raw materials from waste electrical and electronic equipment (WEEE) – A review, 2019, 362, 03043894, 467, 10.1016/j.jhazmat.2018.08.050 | |
2. | Mária Čížková, Pauline Mezricky, Dana Mezricky, Marian Rucki, Vilém Zachleder, Milada Vítová, Bioaccumulation of Rare Earth Elements from Waste Luminophores in the Red Algae, Galdieria phlegrea, 2020, 1877-2641, 10.1007/s12649-020-01182-3 | |
3. | Xia Kang, Laszlo Csetenyi, Geoffrey Michael Gadd, Monazite transformation into Ce‐ and La‐containing oxalates by Aspergillus niger , 2020, 22, 1462-2912, 1635, 10.1111/1462-2920.14964 | |
4. | Melissa K Corbett, Elizabeth LJ Watkin, Microbial cooperation improves bioleaching recovery rates, 2018, 39, 1324-4272, 50, 10.1071/MA18013 | |
5. | Yang Qu, Hui Li, Xiaoqing Wang, Wenjie Tian, Ben Shi, Minjie Yao, Ying Zhang, Bioleaching of Major, Rare Earth, and Radioactive Elements from Red Mud by using Indigenous Chemoheterotrophic Bacterium Acetobacter sp., 2019, 9, 2075-163X, 67, 10.3390/min9020067 | |
6. | Alexander M. Panichev, Ivan V. Seryodkin, Yuri N. Kalinkin, Raisa A. Makarevich, Tatiana A. Stolyarova, Alexander A. Sergievich, Pavel P. Khoroshikh, Development of the “rare-earth” hypothesis to explain the reasons of geophagy in Teletskoye Lake are kudurs (Gorny Altai, Russia), 2018, 40, 0269-4042, 1299, 10.1007/s10653-017-0056-x | |
7. | Norma Cecilia Martinez-Gomez, Huong N. Vu, Elizabeth Skovran, Lanthanide Chemistry: From Coordination in Chemical Complexes Shaping Our Technology to Coordination in Enzymes Shaping Bacterial Metabolism, 2016, 55, 0020-1669, 10083, 10.1021/acs.inorgchem.6b00919 | |
8. | Marcos Y. Voutsinos, Jillian F. Banfield, John W. Moreau, Secondary lanthanide phosphate mineralisation in weathering profiles of I-, S- and A-type granites, 2021, 85, 0026-461X, 82, 10.1180/mgm.2020.90 | |
9. | Matthias Wehrmann, Patrick Billard, Audrey Martin-Meriadec, Asfaw Zegeye, Janosch Klebensberger, Xinning Zhang, Dianne K. Newman, Functional Role of Lanthanides in Enzymatic Activity and Transcriptional Regulation of Pyrroloquinoline Quinone-Dependent Alcohol Dehydrogenases in Pseudomonas putida KT2440, 2017, 8, 2150-7511, 10.1128/mBio.00570-17 | |
10. | Ata Akcil, Nazym Akhmadiyeva, Rinat Abdulvaliyev, Pratima Meshram, Overview On Extraction and Separation of Rare Earth Elements from Red Mud: Focus on Scandium, 2018, 39, 0882-7508, 145, 10.1080/08827508.2017.1288116 | |
11. | Romy Auerbach, Katrin Bokelmann, Stefan Ratering, Rudolf Stauber, Sylvia Schnell, Jörg Zimmermann, Recycling of Florescent Phosphor Powder Y2O3:Eu by Leaching Experiments, 2017, 262, 1662-9779, 596, 10.4028/www.scientific.net/SSP.262.596 | |
12. | Limin Zhang, Hailiang Dong, Yan Liu, Liang Bian, Xi Wang, Ziqi Zhou, Ying Huang, Bioleaching of rare earth elements from bastnaesite-bearing rock by actinobacteria, 2018, 483, 00092541, 544, 10.1016/j.chemgeo.2018.03.023 | |
13. | Vesna Vukojević, Slađana Đurđić, Violeta Stefanović, Jelena Trifković, Dragan Čakmak, Veljko Perović, Jelena Mutić, Scandium, yttrium, and lanthanide contents in soil from Serbia and their accumulation in the mushroom Macrolepiota procera (Scop.) Singer, 2019, 26, 0944-1344, 5422, 10.1007/s11356-018-3982-y | |
14. | Mariele Canal Bonfante, Jéssica Prats Raspini, Ivan Belo Fernandes, Suélen Fernandes, Lucila M.S. Campos, Orestes Estevam Alarcon, Achieving Sustainable Development Goals in rare earth magnets production: A review on state of the art and SWOT analysis, 2021, 137, 13640321, 110616, 10.1016/j.rser.2020.110616 | |
15. | Yehia Osman, Ahmed Gebreil, Amr M. Mowafy, Tarek I. Anan, Samar M. Hamed, Characterization of Aspergillus niger siderophore that mediates bioleaching of rare earth elements from phosphorites, 2019, 35, 0959-3993, 10.1007/s11274-019-2666-1 | |
16. | Yang Qu, Hui Li, Xiaoqing Wang, Wenjie Tian, Ben Shi, Minjie Yao, Lina Cao, Lingfan Yue, Selective Parameters and Bioleaching Kinetics for Leaching Vanadium from Red Mud Using Aspergillus niger and Penicillium tricolor, 2019, 9, 2075-163X, 697, 10.3390/min9110697 | |
17. | Xia Kang, Laszlo Csetenyi, Geoffrey Michael Gadd, Biotransformation of lanthanum by Aspergillus niger, 2019, 103, 0175-7598, 981, 10.1007/s00253-018-9489-0 | |
18. | Subhabrata Dev, Ankur Sachan, Fahimeh Dehghani, Tathagata Ghosh, Brandon R. Briggs, Srijan Aggarwal, Mechanisms of biological recovery of rare-earth elements from industrial and electronic wastes: A review, 2020, 397, 13858947, 124596, 10.1016/j.cej.2020.124596 | |
19. | Stefanie Hopfe, Katrin Flemming, Falk Lehmann, Robert Möckel, Sabine Kutschke, Katrin Pollmann, Leaching of rare earth elements from fluorescent powder using the tea fungus Kombucha, 2017, 62, 0956053X, 211, 10.1016/j.wasman.2017.02.005 | |
20. | Marja Salo, Oleg Knauf, Jarno Mäkinen, Xiaosheng Yang, Pertti Koukkari, Integrated acid leaching and biological sulfate reduction of phosphogypsum for REE recovery, 2020, 155, 08926875, 106408, 10.1016/j.mineng.2020.106408 | |
21. | Charles S. Cockell, Rosa Santomartino, Kai Finster, Annemiek C. Waajen, Lorna J. Eades, Ralf Moeller, Petra Rettberg, Felix M. Fuchs, Rob Van Houdt, Natalie Leys, Ilse Coninx, Jason Hatton, Luca Parmitano, Jutta Krause, Andrea Koehler, Nicol Caplin, Lobke Zuijderduijn, Alessandro Mariani, Stefano S. Pellari, Fabrizio Carubia, Giacomo Luciani, Michele Balsamo, Valfredo Zolesi, Natasha Nicholson, Claire-Marie Loudon, Jeannine Doswald-Winkler, Magdalena Herová, Bernd Rattenbacher, Jennifer Wadsworth, R. Craig Everroad, René Demets, Space station biomining experiment demonstrates rare earth element extraction in microgravity and Mars gravity, 2020, 11, 2041-1723, 10.1038/s41467-020-19276-w | |
22. | Alessandra Marra, Alessandra Cesaro, Eldon R. Rene, Vincenzo Belgiorno, Piet N.L. Lens, Bioleaching of metals from WEEE shredding dust, 2018, 210, 03014797, 180, 10.1016/j.jenvman.2017.12.066 | |
23. | Leonid Plyatsuk, Magdalena Balintova, Yelizaveta Chernysh, Iryna Ablieieva, Oleksiy Ablieiev, 2020, Chapter 84, 978-3-030-22364-9, 843, 10.1007/978-3-030-22365-6_84 | |
24. | Milada Vítová, Mária Čížková, Vilém Zachleder, 2019, Chapter 6, 978-1-78985-009-3, 10.5772/intechopen.80260 | |
25. | Homayoun Fathollahzadeh, Jacques J. Eksteen, Anna H. Kaksonen, Elizabeth L. J. Watkin, Role of microorganisms in bioleaching of rare earth elements from primary and secondary resources, 2019, 103, 0175-7598, 1043, 10.1007/s00253-018-9526-z | |
26. | Saskia Bindschedler, Thi Quynh Trang Vu Bouquet, Daniel Job, Edith Joseph, Pilar Junier, 2017, 99, 9780128120507, 53, 10.1016/bs.aambs.2017.02.002 | |
27. | Hongyue Jin, David W. Reed, Vicki S. Thompson, Yoshiko Fujita, Yongqin Jiao, Michael Crain-Zamora, Jacob Fisher, Katherine Scalzone, Mike Griffel, Damon Hartley, John W. Sutherland, Sustainable Bioleaching of Rare Earth Elements from Industrial Waste Materials Using Agricultural Wastes, 2019, 7, 2168-0485, 15311, 10.1021/acssuschemeng.9b02584 | |
28. | K.A. Natarajan, 2018, 9780128040225, 305, 10.1016/B978-0-12-804022-5.00011-6 | |
29. | Romy Auerbach, Katrin Bokelmann, Rudolf Stauber, Sylvia Schnell, Stefan Ratering, Arite Werner, Roland Haseneder, Radek Vostal, Martin Bertau, Carsten Gellermann, Bioleaching zum Recycling von Sekundärrohstoffen, 2018, 52, 00092851, 330, 10.1002/ciuz.201800829 | |
30. | Xia Kang, Laszlo Csetenyi, Geoffrey Michael Gadd, Colonization and bioweathering of monazite by Aspergillus niger : solubilization and precipitation of rare earth elements , 2021, 1462-2912, 10.1111/1462-2920.15402 | |
31. | Megan Barnett, Barbara Palumbo-Roe, Simon Gregory, Comparison of Heterotrophic Bioleaching and Ammonium Sulfate Ion Exchange Leaching of Rare Earth Elements from a Madagascan Ion-Adsorption Clay, 2018, 8, 2075-163X, 236, 10.3390/min8060236 | |
32. | Payam Rasoulnia, Robert Barthen, Aino-Maija Lakaniemi, A critical review of bioleaching of rare earth elements: The mechanisms and effect of process parameters, 2021, 51, 1064-3389, 378, 10.1080/10643389.2020.1727718 | |
33. | Romy Auerbach, Stefan Ratering, Katrin Bokelmann, Carsten Gellermann, Thilo Brämer, Renate Baumann, Sylvia Schnell, Bioleaching of valuable and hazardous metals from dry discharged incineration slag. An approach for metal recycling and pollutant elimination, 2019, 232, 03014797, 428, 10.1016/j.jenvman.2018.11.028 | |
34. | Ludmila Chistoserdova, Applications of methylotrophs: can single carbon be harnessed for biotechnology?, 2018, 50, 09581669, 189, 10.1016/j.copbio.2018.01.012 | |
35. | Yilin He, Lingya Ma, Xurui Li, Heng Wang, Xiaoliang Liang, Jianxi Zhu, Hongping He, Mobilization and fractionation of rare earth elements during experimental bio-weathering of granites, 2023, 343, 00167037, 384, 10.1016/j.gca.2022.12.027 | |
36. | Alexander Panichev, Nataly Baranovskaya, Ivan Seryodkin, Igor Chekryzhov, Elena Vakh, Tatyana Lutsenko, Olga Patrusheva, Raisa Makarevich, Alexey Kholodov, Kirill Golokhvast, Kudurs (mineral licks) in the Belukha Mountain area, Altai Mountains, Russia, 2022, 15, 1866-7511, 10.1007/s12517-022-10478-8 | |
37. | Jean-Luc Mukaba, Chuks Paul Eze, Omoniyi Pereao, Leslie Felicia Petrik, Rare Earths’ Recovery from Phosphogypsum: An Overview on Direct and Indirect Leaching Techniques, 2021, 11, 2075-163X, 1051, 10.3390/min11101051 | |
38. | A. M. Panichev, N. V. Baranovskaya, I. V. Seryodkin, I. Yu. Chekryzhov, E. A. Vakh, B. R. Soktoev, A. I. Belyanovskaya, R. A. Makarevich, T. N. Lutsenko, N. Yu. Popov, A. V. Ruslan, D. S. Ostapenko, A. V. Vetoshkina, V. V. Aramilev, A. S. Kholodov, K. S. Golokhvast, Landscape REE anomalies and the cause of geophagy in wild animals at kudurs (mineral salt licks) in the Sikhote-Alin (Primorsky Krai, Russia), 2022, 44, 0269-4042, 1137, 10.1007/s10653-021-01014-w | |
39. | Ayoub Bounaga, Anwar Alsanea, Karim Lyamlouli, Chen Zhou, Youssef Zeroual, Rachid Boulif, Bruce E. Rittmann, Microbial transformations by sulfur bacteria can recover value from phosphogypsum: A global problem and a possible solution, 2022, 57, 07349750, 107949, 10.1016/j.biotechadv.2022.107949 | |
40. | Camino García‐Balboa, Paloma Martínez‐Alesón García, Victoria López‐Rodas, Eduardo Costas, Beatriz Baselga‐Cervera, Microbial biominers: Sequential bioleaching and biouptake of metals from electronic scraps, 2022, 11, 2045-8827, 10.1002/mbo3.1265 | |
41. | Rashmi Upadhyay, Perumalla Janaki Ramayya, 2023, Chapter 20, 978-3-031-25677-6, 319, 10.1007/978-3-031-25678-3_20 | |
42. | Emmanuel Yaw Owusu-Fordjour, Xinbo Yang, Bioleaching of rare earth elements challenges and opportunities: A critical review, 2023, 11, 22133437, 110413, 10.1016/j.jece.2023.110413 | |
43. | Alessandra Cesaro, 2024, Chapter 4, 978-3-031-43624-6, 67, 10.1007/978-3-031-43625-3_4 | |
44. | S Cebekhulu, A. Gómez-Arias, A. Matu, J Alom, A. Valverde, M. Caraballo, O. Ololade, P. Schneider, J Castillo, Role of indigenous microbial communities in the mobilization of potentially toxic elements and rare-earth elements from alkaline mine waste, 2024, 03043894, 133504, 10.1016/j.jhazmat.2024.133504 | |
45. | Sabrina Hedrich, Anja Breuker, Mirko Martin, Axel Schippers, Rare earth elements (bio)leaching from zircon and eudialyte concentrates, 2023, 219, 0304386X, 106068, 10.1016/j.hydromet.2023.106068 | |
46. | Xu Zhang, Ningjie Tan, Seyed Omid Rastegar, Tingyue Gu, 2024, 9781119894339, 321, 10.1002/9781119894360.ch13 | |
47. | Yoshiko Fujita, Dan Park, Margaret Lencka, Andre Anderko, David Reed, Vicki Thompson, Gaurav Das, Ali Eslamimanesh, Yongqin Jiao, 2024, 9781119515036, 251, 10.1002/9781119515005.ch8 | |
48. | Helena Singer, Robin Steudtner, Ignacio Sottorff, Björn Drobot, Arjan Pol, Huub J. M. Op den Camp, Lena J. Daumann, Learning from nature: recovery of rare earth elements by the extremophilic bacterium Methylacidiphilum fumariolicum, 2023, 59, 1359-7345, 9066, 10.1039/D3CC01341C | |
49. | Tingting Yue, Yuankun Yang, Lunzhen Li, Mingyue Su, Maosheng Wang, Yucheng Liao, Liang Jia, Shu Chen, Application Prospect of Anaerobic Reduction Pathways in Acidithiobacillus ferrooxidans for Mine Tailings Disposal: A Review, 2023, 13, 2075-163X, 1192, 10.3390/min13091192 | |
50. | Khyati Joshi, Sara Magdouli, Satinder Kaur Brar, Bioleaching for the recovery of rare earth elements from industrial waste: A sustainable approach, 2025, 215, 09213449, 108129, 10.1016/j.resconrec.2025.108129 | |
51. | Yujian Liang, Zuotan Huang, Qiudong Xiao, Xuan Guan, Xinman Xu, Hongmin Jiang, Yijian Zhong, Zhihong Tu, Dissolution and mechanism of bastnaesite mediated by Acidithiobacillus ferrooxidans, 2025, 10020721, 10.1016/j.jre.2025.03.025 | |
52. | Alexander Paul Fritz, Lena Josefine Daumann, Stefan Schwarzer, Bioleaching of Rare Earth Fluorescent Lamp Phosphors Using Kombucha, 2025, 0021-9584, 10.1021/acs.jchemed.4c01532 |