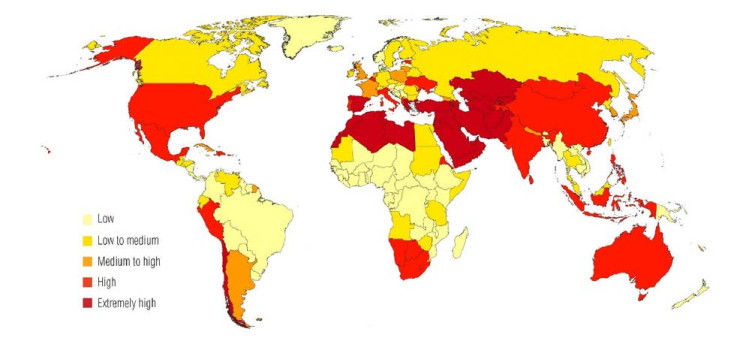
This review is inspired by the increasing shortage of fresh water in areas of the world, and is written in response to the expanding demand for sustainable technologies due to the prevailing crisis of depleting natural water resources. It focuses on comprehending different solar energy-based technologies. Since the increasing population has resulted in the rising demand for freshwater, desalination installation volume is rapidly increasing globally. Conventional ways of desalination technologies involve the use of fossil fuels to extract thermal energy which imparts adverse impacts on the environment. To lessen the carbon footprint left by energy-intensive desalination processes, the emphasis has shifted to using renewable energy sources to drive desalination systems. The growing interest in combining solar energy with desalination with an emphasis on increasing energy efficiency has been sparked by the rapid advancements in solar energy technology, particularly solar thermal. This review paper aims to reflect various developments in solar thermal desalination technologies and presents prospects of solar energy-based desalination techniques. This paper reviews direct and indirect desalination techniques coupled with solar energy, and goes on to explain recent trends in technologies. This review also summarizes the emerging trends in the field of solar thermal desalination technologies. The use of nanoparticles and photo-thermal materials for localized heating in solar desalination systems has decreased energy consumption and enhanced the efficiency of the system. Solar power combined with emerging processes like membrane distillation (MD) has also a recent resurgence.
Citation: Laveet Kumar, Jahanzaib Soomro, Hafeez Khoharo, Mamdouh El Haj Assad. A comprehensive review of solar thermal desalination technologies for freshwater production[J]. AIMS Energy, 2023, 11(2): 293-318. doi: 10.3934/energy.2023016
[1] | Mark P. McHenry, P. V. Brady, M. M. Hightower . PV-Li-ion-micropump membrane systems for portable personal desalination. AIMS Energy, 2016, 4(3): 444-460. doi: 10.3934/energy.2016.3.444 |
[2] | Seif Bayoumi, Mohamed E.A. Ali, Islam Amin, Raneem El Torky, Selda Oterkus, Hosam Shawky, Erkan Oterkus . Environmentally-driven design of a floating desalination platform (Case study: reverse osmosis floating desalination platform of ras gharib, Egypt). AIMS Energy, 2021, 9(3): 623-650. doi: 10.3934/energy.2021030 |
[3] | Wei An, Yifan Zhang, Bo Pang, Jun Wu . Synergistic design of an integrated pv/distillation solar system based on nanofluid spectral splitting technique. AIMS Energy, 2021, 9(3): 534-557. doi: 10.3934/energy.2021026 |
[4] | Emmanuel Arthur . Energy development: A global perspective and advances in Ghana. AIMS Energy, 2022, 10(2): 306-339. doi: 10.3934/energy.2022017 |
[5] | Kaovinath Appalasamy, R Mamat, Sudhakar Kumarasamy . Smart thermal management of photovoltaic systems: Innovative strategies. AIMS Energy, 2025, 13(2): 309-353. doi: 10.3934/energy.2025013 |
[6] | Gemma Graugés Graell, George Xydis . Solar Thermal in the Nordics. A Belated Boom for All or Not?. AIMS Energy, 2022, 10(1): 69-86. doi: 10.3934/energy.2022005 |
[7] | Ladislas Mutunda Kangaji, Atanda Raji, Efe Orumwense . Cutting-edge progress in offshore wind and tidal stream power technology—State-of-the-Art. AIMS Energy, 2025, 13(1): 188-230. doi: 10.3934/energy.2025007 |
[8] | Nassir D. Mokhlif, Muhammad Asmail Eleiwi, Tadahmun A. Yassen . Experimental evaluation of a solar water heater integrated with a corrugated absorber plate and insulated flat reflectors. AIMS Energy, 2023, 11(3): 522-539. doi: 10.3934/energy.2023027 |
[9] | Chao-Jen Li, Peiwen Li, Kai Wang, Edgar Emir Molina . Survey of Properties of Key Single and Mixture Halide Salts for Potential Application as High Temperature Heat Transfer Fluids for Concentrated Solar Thermal Power Systems. AIMS Energy, 2014, 2(2): 133-157. doi: 10.3934/energy.2014.2.133 |
[10] | Abdulrahman Almutlaq . Indoor experiments of a horizontal multiple effects diffusion solar still: Influence of heat input and the number of stages. AIMS Energy, 2024, 12(2): 532-547. doi: 10.3934/energy.2024025 |
This review is inspired by the increasing shortage of fresh water in areas of the world, and is written in response to the expanding demand for sustainable technologies due to the prevailing crisis of depleting natural water resources. It focuses on comprehending different solar energy-based technologies. Since the increasing population has resulted in the rising demand for freshwater, desalination installation volume is rapidly increasing globally. Conventional ways of desalination technologies involve the use of fossil fuels to extract thermal energy which imparts adverse impacts on the environment. To lessen the carbon footprint left by energy-intensive desalination processes, the emphasis has shifted to using renewable energy sources to drive desalination systems. The growing interest in combining solar energy with desalination with an emphasis on increasing energy efficiency has been sparked by the rapid advancements in solar energy technology, particularly solar thermal. This review paper aims to reflect various developments in solar thermal desalination technologies and presents prospects of solar energy-based desalination techniques. This paper reviews direct and indirect desalination techniques coupled with solar energy, and goes on to explain recent trends in technologies. This review also summarizes the emerging trends in the field of solar thermal desalination technologies. The use of nanoparticles and photo-thermal materials for localized heating in solar desalination systems has decreased energy consumption and enhanced the efficiency of the system. Solar power combined with emerging processes like membrane distillation (MD) has also a recent resurgence.
With the advent of climate change, more and more areas of the world are observing rising water stress at an accelerated pace. According to the UN World Water Development Survey, 3.7 billion individuals are affected by the scarcity of water. This population of affected people may rise to as many as 5.7 billion by 2050 [1]. Being a victim of the crisis, the Middle East has been transformed into the world's most water-stressed region. The unavailability of natural resources enforces people to migrate in search of a better life; according to the UN refugee agency (UNHCR), "roughly 90pc of the refugees come from countries that are most vulnerable and least ready to adapt to the impacts of climate change". In 2021, natural disasters alone forced nearly three million people to leave their homes in Africa and the middle east- an international organization for migration. The fact that 3.5 million people lose their lives each year due to the unavailability of clean water and sanitation highlights the importance of water as a resource for the entire world. Figure 1 represents the forecasting of the world by 2040 and depicts the regions that would be most affected Nations in the tropical zones already experience decreasing freshwater resources and such forecasting drags concerns of humanity [2]. It is important to note that 71 percent of the earth is water covered. However, only 3 percent is freshwater; of that, only 1.2 percent is available as drinkable; the rest of it is frozen as glaciers. To meet the demand for freshwater, seawater can be used after removing salts, known as desalination. Desalination is a process used to remove salt from saltwater, making it useful for producing drinking water and other things. To make that happen, a lot of energy is required. Due to the cheap price of fossil fuels, less than $3 for one barrel of oil, the earliest technologies were thermally propelled, which still prevails. The first desalination facilities were set up in the late 1950s to address the steadily rising freshwater demand brought on by population growth and welfare concerns [3, 4].
With the development of science, it was discovered that desalination using renewable energy may produce water at rates comparable to those of plants using fossil fuels while also reducing greenhouse gas emissions that cause global warming [5].
Solar energy and its availability have attracted various researchers and scientists to use solar energy to desalinate water. Various techniques are being used to increase freshwater generation by using solar energy. Methods to increase the evaporation of water to separate it from salts are porous media, phase change materials, nanotechnology, and photovoltaic and localized thermal heating. According to some estimations, currently, more than 21, 000 desalination systems are operating in the world having the potential to produce 86.5 million cubic meters per day of freshwater. Of all desalination methods, Reverse Osmosis (RO) plants are more dominant. 66 percent of all installed plants use RO based [5]. However, RO plants use electricity as a driving force to operate high-pressure pumps and fossil fuels are used to produce electricity which as result exhausts greenhouse gases to the environment. To drive RO plants using Photovoltaic panels is not suitable, since the conversion of solar energy into electrical energy to operate high-pressure pumps for the large-scale production of freshwater is not economical. On the other hand, solar thermal energy is widely available. Solar thermal energy can be used to heat saline water to generate water vapors, and when these vapors are condensed fresh water can be produced.
This paper has focused on solar thermal energy desalination systems. The paper critically reviews the available solar thermal energy-driven systems and reflects on how solar energy could be coupled with desalination technologies.
Then the paper reviews the existing and emerging solar thermal desalination technologies and also those that could be used with solar energy to produce a hybrid system. Finally, the paper provides recommendations for the existing technologies to become the most appropriate solar-powered desalination technologies.
In short, the key contributions of this review paper to the research field are:
● Various ways in which solar thermal energy is used to produce freshwater with nearly zero harmful effects on the environment.
● Reflecting the recent trends in the field of solar thermal desalination technologies which aim to increase the efficiency and output of the system and it also provides the prospects of the desalination system along with future recommendations
The paper is organized as follows:
Section 2 defines the need for solar thermal technologies and explains how this approach is used to produce freshwater with efficiency and sustainability. Section 3 is focused on various types of renewable energies concerning solar energy. Section 4 defines some existing trends and 5 elaborates on the prospects of desalination. Sections 6 and 7 provides the conclusion and recommendation.
Above all the renewable energy resources, this paper focuses more on solar thermal renewable energy resources. Despite recent advancements in desalination technology, these processes are still primarily regarded as energy intensive. Solutions to reduce unit costs and so boost the financial viability of such initiatives are being carried out [6]. One direction is the employment of renewable energy sources (RESs), which play an important part, given key trends in combating climate change [7].
Desalination and renewable energy are two distinct concepts that can be mixed in numerous ways. Researchers from the desalination and renewable energy sectors must collaborate to create successful integrated designs. The energy produced on-site from readily available renewable energy sources in the area can help the desalination process. The potential of employing renewable energy in desalination techniques is shown in Figure 2. Hydro, wind, and geothermal energy can be used to produce electricity to operate mechanical vapor compression (MCV), reverse osmosis (RO), Electrodialysis (ED), Capacitive deionization (CDI), and Electrode-ionization (EDI). Since thermal energy is difficult to store, the thermal energy generated using stored electricity can operate various other thermal-driven desalination systems as shown in Figure 2. The aforementioned figure also depicts various ways in which a hybrid system can be produced to run a desalination system. Heat, electricity, or mechanical energy are just a few of the different ways that this energy can be produced. Any RES technology, especially those that use energy for desalination, theoretically might coexist with a water desalination plant. The energy consumed for desalination is countered by connecting these facilities to the power grid, if electricity generation is not steady or constant throughout a particular day or season, as is the case with solar or wind power systems. Consequently, there is a balance between the energy generated and spent (compensation concept).
Figure 3 represents the commercialization of various techniques to desalinate water. On a vertical scale, it states the status of the respective technology. For instance, solar stills are in the application of producing freshwater with a capacity of less than 0.1-meter cube per day. On the other hand, RO plants are also in the application phase with a production capacity of more than 5000-meter cubes per day. In addition, solar MD, geothermal, and solar MEH are in the phase of advanced research and development. While the technologies in the basic research are solar EDR, Wind ED, etc.
The thermal energy of the sun, being received in the form of radiation is captured by a variety of methods, including 1) concentrated solar power (CSP), technologies that extract energy from the sun in terms of thermal energy; and 2) photovoltaic (PV)/concentrator photovoltaic (CPV)—solar modules that capture the solar energy carried by photons as electricity.
Solar thermal is the most widely used method which is under research consideration technologies. It usually employs thermal collectors and concentrated thermal collectors to extract the required energy. The thermal energy is responsible for driving: Membrane Desalination Multi-Stage Flash, Multi-Effect Desalination, and vapor compression distillation. These mechanisms consume a significant amount of energy, particularly in regions with more salinity in the water, such as African countries (having the potential to approach 45 mg/mL) [8]. Whereas, the following areas demonstrate favorable solar irradiation conditions, with seasonal ranges of 2200 to 2400 kWh/m2 [9]. Innovative designs are on their way to desalination, such as in Southern Xinjiang (China), Fang et al. [10] developed a new solar photothermal desalination technique that involved the use of an evaporator and membrane processing equipment to produce freshwater from brackish water.
Chao et al. [11] evaluated the credibility of solar power amalgamated with desalination processes to mitigate the upcoming crisis with the need for drinkable water in China. A unique solar-driven desalination method for efficient water output was proposed by Lu et al. [12] Comprises of a cascaded latent heat recovery structure integrated with a thermal concentration design. The system has a lot of promise for use in both commercial and small-scale desalination. Traditional sun-powered thermal desalination requires a large amount of land surface area and, as a result, a lot of solar radiation-concentrating equipment with a large surface area. This has a detrimental economic effect as compared to the use of conventional fuels. Among others, Zheng and Hatzell [13] have created a technical and financial design for the optimization of the Reverse Osmosis process running on solar thermal energy. They used theoretical data to assess the conditions in 7 maritime cities in the United States, and they concluded that Miami is the ideal location (Florida).
Studies that have been done on this technique generally focus on the viability of employing PV technology for water desalination in the Reverse Osmosis method, or PV-RO, provided that there is sufficient availability or solar irradiation for the system to operate on its own.
The various studies referring to the investigation of desalination methods employing Reverse Numerous scholars and researchers have studied Osmosis and Photovoltaic, such as; Findings from a work of Calise et al. [14] that talked about using solar collectors to desalinate saltwater and synchronized cooling, heating, and electricity process based on photovoltaics. Several studies on the use of PVs in the RO process for the desalination of seawater without the need for a battery system were conducted by Thomson et al. [15]. Karavas et al. [16] examined the potential for combining a DC microgrid and hybrid transitory energy saving. Antonio et al. [17] and Yong et al. [18] both made intriguing recommendations for limited (500 Wp) systems situated in rural regions. Yong came to the same conclusions when they asserted that limited PV systems are an especially attractive answer in places that are off the grid. Researchers from Spain presented the early findings of a cutting-edge hybrid solar (PV) and brackish water RO desalination system [19]. Although connecting solar systems with desalination systems can be advantageous in the next generation, there is still an obligation of assessing the sustainability, lucrative, and environmental implications of such systems. Nanotechnology was identified as a possible development direction, by Bait [20], from the perspective of lowering the costs involved with the elimination of, for example, microbes in the water treatment process and he also Reflected the Nano fluids-based solar desalination processes including direct and indirect systems Another intriguing idea is concentrated photovoltaic technology (CPV). Their working concept is similar to that of conventional PV systems, but they require substantially fewer cells due to the use of extra mirrors that concentrate solar radiation on the PV cells [21]. To automatically follow the sun throughout the day, these systems typically need a solar tracking device. Due to their better efficiency and thus higher energy yields per megawatt of installed capacity, particularly in the temperate zone, CPV systems are an alternative to conventional PV installations [22].
Aside from solar energy, wind energy is the most frequently used and researched renewable energy source concerning pairing it with water desalination devices. In this instance, electricity produced by renewable energy sources is used to operate an independent desalination plant [23]. For example, Qingfen & Hui [24], Mohammad Ali et al. [25], Soraida Aguilar et al. [26], Jamie et al. [27], and Diaz and Soares [28] have collated the most recent evaluations of wind power utilization concerning offshore wind farms.
Biomass energy is the sole form of energy that has yet to be used in the desalination of water. However, recent years have seen the publication of the results of numerous studies that looked at the possibility of using bioenergy in composite systems in combination with other sustainable and non-sustainable energy sources.
Power and heat generation are considered when employing geothermal energy for water desalination, even though high-enthalpy geothermal resources are rather uncommon in comparison to low-enthalpy ones, which are far more frequently available [29].
Aguilar-Jim'enez et al. [30] suggested a novel strategy for combining Membrane Desalination technology and Organic Rankine Cycle. It was suggested to install the ORC because it enables merged PV and desalination utilizing low-grade energy resources in the initial phase of MD performance. According to the results, the desalination method combined having Organic Rankine Cycle shows 22% more effectiveness at desalinating water in comparison to a traditional MED system while only requiring a 6.9% increase in the heat exchange rate. It ought to be emphasized that geothermal water could also be treated if it has suitable chemical properties, in addition to geothermal energy, in desalination procedures [31]. Although several desalination/treatment plants using geothermal energy are in operation globally, these projects are frequently suitable for small-scale purposes [32]. For the island of Nisyros, Jang et al. offered a different model; the system was able of providing freshwater at a projected 225 m3/day [33].
Based on how technology obtains energy from the sun, this technology is split to direct and indirect, two different approaches [34]. Systems that use direct solar desalination, like solar stills, directly harvest solar thermal energy. As opposed to this, indirect solar desalination systems use solar energy, either as thermal or electrical energy, to power the desalination process. Even though various cutting-edge and optimization studies for solar still technologies have been conducted, these technologies are not frequently deployed for large-scale freshwater production [35]. Additionally, research is ongoing to find more sophisticated solar stills that can be used for desalination. Reverse osmosis, electrodialysis, humidification and dehumidification, membrane desalination, multi-effect desalination, multi-stage flash, and multi-effect desalination are examples of indirect desalination systems. Reverse osmosis is seen as practical for industrial production but not for small-scale desalination [36].
Despite being among the earliest and most basic desalination systems, solar energy is still being investigated for new materials [37]. Its operation is simple and easy to comprehend. In this technology, feed water is directly vaporized using incoming solar radiation and then the evaporated vapors are condensed as distilled water [38]. Studies are undergoing for solar stills to work in the absence of solar energy by latent heat storage systems [39]. Large-scale systems are not deemed ideal for solar still designs [40]. Due to the few pieces of equipment and simple design, it is affordable and maintenance costs are also low. However, it is less efficient and includes heat losses. However, recent advancements and modifications have again paved the way for solar still desalination to be effective and capable of producing freshwater.
Thakur et al. [40] used limited graphene oxide layered absorber plate consisting of an activated carbon pellet which resulted in a water yield of 58.15% and an efficiency throughout the day of 64.44 respectively. It proved to be more efficient in comparison to Conventional Solar Still. Shoeibi et al. [41] used activated carbon as porous materials which resulted in an energy efficiency enhancement of 94.14. He conducted an experiment on three different solar stills and elaborated difference in the yield. Additionally, by using porous materials such as black steel wool fiber and aluminum fins, the production from a solar still desalination was enhanced by roughly 42.3% and 20.9%, respectively. The comparison of the daily accumulated yield is shown in Figure 4. The figure represents the yield comparison of three different solar stills, named as the conventional solar still (CSS), solar still with reduced graphene oxide coated absorber (SS-RGO), and the solar still with combined RGO and activated carbon pallet (SS-RGO-ACP). In each of the cases, the maximum yield is achieved at 2:00 p.m.; the conventional solar still (CSS) resulted 0.56 L/m2 at the intensity of 971 W/m2. In the other case, a solar still with (RGO) coated absorber plate, the heat transfer rate increased and peak hour freshly yield increased by 21.4% as 0.68 L/m2. The combined effects of the RGO coating and the porous activated carbon's ability to retain thermal energy significantly increased the yield in the case of the SS-RGO-ACP. The peak hourly and daily water yields of SS-RGO-ACP were observed to be 0.79 L/m2 and 4.46 L/m2 respectively.
In an additional attempt, Pani and PEGDA double-network hydrogel were applied on an insulating layer of polyethylene (EPE) coated in cellulose, which was developed by Yin et al. [42], for a steam formation method. And the device resulted in an efficiency of 91.5% as a solar steam generator under 1 kw/m2. However, the review suggested that the parameters of nanoparticles following their conduct in solar still in addition to the way the heat transfer process takes place are to be investigated. Additionally, in the direct solar stills, nanofluids are added to the saline water basin, and environmental concerns must be treated seriously [43]. Parasa et al. [44] evaluated some innovative designs such as adding an external thermoelectric condenser and using an Ag-based Nanofluid. The system yielded around 8 L/m2 and has an efficiency of 30.6 and 50.8% for Nano fluid-based solar still and condenser-based solar still. Yu et al. [45] employed targeted evaporation to help with desalination and manufactured gold nanoparticle (AuNP) films on a nonporous anodized aluminum oxide (AA) supporting layer.
MSF is a thermally driven technique. Conventionally, brine solution waste heat was used to heat water and then subjected to flashing through numerous chambers by changing the saturation pressure. Moustafa et al. [46] Used a three-way valve hot water thermal storage system which resulted in a GOR of 6.5 to 8. Kapil et al. [47] provided a conceptual model of a brine loop system for MSF desalination represented in Figure 5. The system includes a Direct absorption solar collector (DASC) which is a counter-flow heat exchanger, it transfers heat from nanofluid to preheat recycled brine. The DASC is made up of a confined area through which the nanofluid moves and absorbs solar energy. The incident radiation can pass through the transparent cover on the collector's top surface and reach the nanofluid. After reaching the desired temperature, the nanofluid exits the collector and enters the counter-flow heat exchanger, where it transfers heat to recycled brine that has already been heated and is leaving the condenser tubes of the BR-MSF system's first flashing stage (at temperature T1). The nanofluid is then pumped back to the DASC to complete its cycle after exiting the heat exchanger.
The system resulted in a GOR of 11 to 14 and this model led to another concept of using a parabolic trough collector system coupled with MSF
Alsehli [48] Experimentally Validated the Solar Powered MFD system withstand in the Storage method. The output reflected that the process is well equipped to produce freshwater water economically as 1.92 m2 of solar thermal collector area wash enough for a daily yield of 19.7 kg of fresh water with as low as $0.015 per liter. In another study, Babaeebazaz et al. [49] practically evaluated a simple, twin-model, multi-stage flash desalination system featuring a vacuum pump, a solar parabolic collector, and a conical cavity receiver was tested in practice. The freshwater yield was 3.22 L per 5 hours in a day while the flow rate of feed water was heated to 94.25 ℃.
Direct steam generation is one of those technologies capable of higher cycle temperature and higher system efficiency and it is also a current trend of many researchers Vahland et al. [50]. In continuation of the system, Khoshrou et al. [51] came up with a modification of Direct steam generation as an alternative renewable energy source which resulted in 17.8 MW of fossil fuel energy replacement with solar energy. And about 53 percent of feed water rejected to the sea is also conserved.
The several effects (single evaporators) of the multi-effect desalination system are linked in series with a condenser, several feed water preheaters, and flashing chambers. Multi-effect desalination systems typically come in three different shapes and sizes: parallel cross-feed forward feeds, and reverse feed [52]. With the advent of technology, various innovative designs have been embedded with the existing desalination techniques. For instance, d'Accadia et al. [53] and Calise et al. [54] studied MED technology integrated with a polygeneration system in addition to the concentrating Photovoltaic thermal collector, a boiler running on biofuel, and an absorption chiller, which was used for distant and social services. In another study [55], multi-effect desalination with 3 to 14 evaporators resulted in steam at 72 ℃ with a simple payback time of 12.5 to 15 years for polygeneration systems. The studies on environmental analysis reflect that the freshwater produced by solar-driven-MED prevents 10 kg of CO2 as compared to one produces with fossil fuels. In addition, the Linear Fresnel collector proved to be more efficient than the parabolic trough collector for the MED system. The evacuated thermal collector was investigated by Xiaohua et al. [56] That ranges between 84 ℃ to 94 ℃. The results indicated that the system produces 35 m3/day at a minimum cost of 3.64 $/m3 when the collector area is 6000 m2.
The vapor partial pressure differential of the two streams on the membrane sides, which is a function of the temperature difference across the membrane, is what drives permeation over the membrane during membrane desalination, which is a thermally driven separation process. Although many desalination methods have been developed, they are quite energy-intensive. On the other hand, membrane distillation (MD), which depends on heat energy to function, is a fortunate technology. Geothermal energy, thermal collectors, and waste heat from various thermal systems can all be used to harvest thermal energy from solar energy [57]. Even in industries, a huge amount of waste heat is unloaded into the atmosphere which, if utilized, could greatly benefit both community and industry. Various desalination techniques have been invented, but they require an enormous amount of energy. But, membrane distillation (MD) offers to be a promising technology since its dependence on thermal energy to work. as required energy could be extracted from solar radiations using collectors, geothermal energy, and waste heat of different thermal systems [57]. Membrane desalination technology has vast applications ranging from pharmaceuticals to juices, industrial waste treatment to textile and chemical treatments [58]. In distant locations where solar energy is available, membrane desalination proved to be competitive and economical [59]. Thomas et al. [60] Studied the phases of membrane desalination and came across three distinct phases. First, the initiation phase (1970–1990), then the emergence phase (1991–2010), and finally the growth phase (2010–2016). The recent development in photothermal material has also paved the way for the MD desalination system; photothermal efficiency has become an important variable for the MD system's operation. The materials can absorb the entire solar radiation, with a wavelength of 250–2500 nm. The materials used are semiconductors combined with organic-inorganic and metallic semiconductors. Until now, numerous MD units have been designed and investigated using solar thermal energy devices such as flat plate thermal collectors (FPC) [61], evacuated tube collectors [62], compound parabolic collectors (CPC) [62], solar ponds [63] and solar still [64]. There are four common MD configurations: (1) direct contact membrane distillation (DCMD), (2) air gap membrane distillation (AGMD), (3) sweeping gas MD, and (4) vacuum MD, of all this paper discusses only DCMD system.
Direct-contact membrane-based desalination systems involve fluids that are in direct junctions with the membrane on both the hot and cold sides. The heated saline water flows through the feed side of the module with adequate energy for evaporation. When the evaporated water travels the membrane sides, it condenses on the permeate side where cold water continually circulates [65]. The thermal energy required for the evaporation of water molecules is provided by the use of flat plate thermal collectors, evacuated tube collectors, parabolic concentrators, and salinity gradient pools [66]. Whereas, the electrical energy required for the operation of electrical devices, such as pumps, is provided by the use of PV panels, also known as the stand-alone way [67, 68]. Figure 6 shows the single-loop and double-loop solar powered desalination systems. Between them, the dual loop is mostly preferred to avoid corrosion due to saline water. The first method is to continue use the heated saline water in the MD module, while the second one uses heat storage tank to increase the output with smooth flow [69, 70].
This evaporation and condensation are responsible for the temperature gradient which results in vapor pressure difference [71]. Moreover, the DCMD technique needs lower pre-treatment [69]. It has lower losses of heat, is suitable for low pressures, is easy to comprehend the operation and greater efficiencies, and requires the least equipment [72]. For better performance of the DCMD process, it is suggested to use a low-cost heating source [73]. In addition, even moderate temperature could be the driving force across the membrane empowering the amalgamation of the DCMD system with solar thermal energy. The solution to water scarcity is also a sustainable solution by eliminating salts and other unwanted minerals from salty water available to engender fresh drinkable water [74]. Several researchers, up to date, attempted to put forward cutting-edge designs to conciliate solar energy with the DCMD system. Nakoa et al. [75] presented a sustainable method of desalination with negligible liquid leaving out. The system is amalgamated into a salinity gradient solar pool and uses DCMD technology. The technology includes the solar heating of saline water and various research works are continued on it. Shafeian et al. [76] proposed amalgamated membrane-based vacuum glass tubes added to the desalination system to improve its capacity to absorb sun energy units. Experimental investigations on the system's performance—without the cooling unit in Case Ⅰ, with it in Case Ⅱ, and without it in Case Ⅲ—in summer and winter (Case Ⅲ). The system's overall efficiency increased from 46.6% in Case Ⅰ to 61.8% in Case Ⅱ, demonstrating the cooling unit's technical performance when installed in the system's permeate flow loop. The production of the system is shown in Figure 7 which states that in case Ⅰ the maximum fresh water production rate, at 14:30, is 3.8 L/m2. However, in case Ⅱ the production rate is 4.7 L/m2 which is greater than the case Ⅰ. At last, the case Ⅲ yields the lowest of all cases, around 2.2 L/m2.
Another proposed model of DCMD system is shown in the schematic diagram, Figure 8. The DC pump will pump the saline water from storage tank 1 into the thermal collector; the flow rate of the DC pump is regulated by the control valve. The control valve regulates the flow by varying the opening of the valve. The saline water after absorbing heat is stored in tank 2; where another dc pump throws the feed into the cascade of the feed loop. On the other hand, a cold permeate is stored in tank 3, where permeate pump pumps the cold stream into the DCMD unit's cold side and vapors from a hot stream are condensed. The permeate flux is stored in the tank from where it can be extracted.
Bouguecha et al. [77] proposed a thermal collector of flat plate design to run a solar DCMD system and provided valuable results of the system. Huang et al. [78] presented work on solar membrane desalination enabled by PVDF membrane and localized heating. Ma et al. [79] using concurrently equations of mass and heat transfer studied the relationship between a thermal collector of flat plate shape to a DCMD unit of weensy size for distant areas. Kim et al. [80] present direct contact membrane distillation (DCMD) system obliged with solar energy for the energy recovery concepts using a temperature-modulating scheme for the working of the whole day. Tlili et al. [81] presented a single-dimensional unique design to evaluate the effects of different functioning and geometric variables on the energy utilization of DCMD processes which helps in building the basic concept of the system. Krnac et al. [82] used a concentric photovoltaic (CPV) and DCMD hybrid system to produce fresh water. The membrane-based desalination system could be coupled with waste heat to desalinate water. The use of low-grade energy has attracted the concern of various researchers. Laqbaqbi et al. [83] presented work on the desalination process that was utilized for dye solution of textile wastewater treatment operating polyvinylidene fluoride (PVDF) membrane's thin sheet. Kumar et al. [84] presented a review of the innovation of solar thermal energy technologies. These technologies mainly deal with industrial process heat and its prospects. It is an extensive compilation of solar thermal energy and its uses in various processes. The work presents different types of thermal collectors and their subdivisions and sun-tracking technologies. Morave et al. [85] presented a performance evaluation of an innovative design in thermal collectors. It is a flat-panel collector with a circular shape and spiral pipes and their efficiency comparison shows that the change in the shape of the collector to circular has resulted in higher efficiency than that of the rectangular-shaped collector. Kumar et al. [86] carried out the modeling and outdoor experimental performance analysis of a solar-assisted process heating (SAPH) system for industrial process heat. Nanophotonics-enabled solar membrane distillation system was proposed by a group at Rice University. The concept utilizes nanoparticle-assisted solar vaporization. The comparison of conventional and nanoparticle embedded is shown in Figure 9 [87].
The use of nanoparticles embedded in electro-spun polyvinyl alcohol layered in conventional PVDF MD membrane [87]. The localized heating resulted in 99.5% salt rejection and 5.38 kg/m2h of flux.
In solar-driven MD systems, vapors transfer matter the most. MD membranes are usually porous and hydrophobic to avoid being wet. The full-scale application of MD systems has been hampered by the present membranes' low wetting resilience [88]. For DCMD utilizing 0.5 M NaCl as feed, Li et al. [89] modified quartz fiber membranes with alkylsilane molecules and discovered that the least wetting-resistant membrane had twice the vapor transfer of the most wetting-resistant membrane. To increase hydrophobicity, Xie et al. [90] applied spray-coated CNTs to three distinct substrates: polytetrafluoroethylene (PTFE), polypropylene (PP), and polyvinylidene fluoride (PVDF). By fluorinating the ZnO blended electrospun PVDF membrane, Li et al. [91] created a super hydrophobic PDTS-ZnO-PVDF membrane. The type of ions in the feed affected the wetting resistance, with CaSO4 and SDS increasing membrane wetting. Cross-linked PVDF-based hollow-fiber membranes with dual wettability were created by Zou et al. [92]. The thick hydrophilic layer was created using a PVDF/PEG blend, and both layers were created by spinning PVDF. A mixture of PVDF, ammonium, and water with varying ammonium and water contents was used to make the dope solution for spinning. For seawater desalination, they reported outstanding performance with flux as high as 97.6 kg/m2h and 99.9% rejection. Yin et al. [93] compared the effects of gypsum and silica scaling in MD on a hydrophobic membrane, a hydrophilic-hydrophobic membrane, and a superhydrophobic membrane.
In membrane desalination, the temperature gradient between the sides of the membrane is usually lower than the temperature gradient between feed and permeate [94]. The recent trend of photothermal materials is overcoming this problem through localized heating, which in fact, increases the temperature gradient at the sides of the membrane than bulk fluid and hence enhances MD performance [95], the schematic depicts the technique in Figure 10.
Some of the studies reflect that solar vapor generation has already reached the limits of its efficiency. The following tactics are still the most effective for maximizing STD performance: (Ⅰ) light-to-heat energy conversion; (ⅱ) thermal vapor creation; and (ⅲ) vapor-to-water condensation [97]. Traditional solar-powered thermal desalination requires a lot of land surface area and, consequently, a lot of solar radiation-concentrating equipment with a big surface area. Compared to the usage of traditional fuels, this has an adverse economic impact. Among many others, Zheng and Hatzell have established a technical and economic model for the optimization of RO thermal water desalination systems utilizing solar energy [13]. Based on theoretical data, they analyzed the conditions prevailing in seven U.S. coastal cities and concluded that the optimal location in Miami (Florida). The results obtained in their technological and economic model indicated that the discounted cost of freshwater production at the rate of 1000 m3/day given a solar collector unit price of USD 100/m2 and collectors operating at 40% efficiency would be USD $0.97 per 1 m3 of pure water [13], which is indeed an impressive achievement in comparison with the observed global trends in the unit cost of obtaining water fit for human consumption both in systems with and without desalination techniques.
The most widely used and researched renewable desalination methods today are the solar photovoltaic integrated RO desalination process, the hybrid solar photovoltaic-wind integrated RO desalination technique, the hybrid solar photovoltaic-thermal (PVT) incorporated RO desalination technique, and the hybrid solar photovoltaic-thermal effect distillation (PVT-MED) desalination process. However, significant research is still needed underway to lower costs, reduce heat loss, improve performance, and boost output [96, 97].
Forward osmosis is a form of desalination procedure that is osmotically or salinity gradient-driven and involves the preferential movement of water over a semi-permeable membrane. Unlike other membrane-based desalination methods, FO requires minimal energy, lower fouling, and lower operational and capital costs. The power consumption of FO energy can be as low as 0.25 kWh/m3 for pumping a fluid. Solar thermal energy, in various ways, can provide the energy required to operate the system. According to recent statistical research by Ang et al. [98], the amount of literature on FO has steadily climbed since 2009, reaching over 250 annual publications between 2016 and 2018. The research is underway in the following areas:
● Real-time observation in FO
● Modulation of the support layer
● Alteration of the active layer employing nanomaterials
Solar-powered desalination techniques have the potential in the future in terms of efficiency, cost savings, and/or performance. Since solar energy is intermittent, the fluctuations could be greatly reduced when a hybrid system is coupled. For instance, a hybrid PV-thermal MD system would prove to be much more efficient than standalone MD [99]. Figure 11 shows a hybrid solar powered MD system with a PV panels, wind energy and biomass. The output of standalone solar driven MD system decreases in the absence of sunlight, but when a hybrid renewable energy source is attached which supplies electricity with the help of batteries in the absence of light, the efficiency of the system will greatly improve. For instance, an electric heater is connected to the system that stores solar energy and provides heat in the absence of sunlight, the efficiency of the system would greatly increases. The scientific community has paid particular attention to the use of hybrid RE systems to power desalination.
Shifting to different, less-discovered desalination methods is one of the future trends in solar desalination. Dewvaporation is one such approach that has the potential for solar-powered desalination. In the dewvaporation method, saturated steam is used as a carrier gas to convert water into steam from saline input into the distillate [100]. It offers the benefit of energy reuse, depending on the use of heat exchangers and improved process design. It has been suggested that a solar-powered dewvaporation device be created for the desalination of salt water. By scientific publication, they adhered gold nanoparticles to the PMMA surface to achieve a water evaporation conversion efficiency of more than 50%.
The localized heating improves MD performance by increasing the temperature difference at the sidewalls of the membrane over bulk fluid. It would make the system compact and more efficient. The special attention to wettability is also going to make significant results.
Particular membrane wettability, to avoid becoming wet, MD membranes are typically permeable and hydrophobic. The poor wetting resistance of the current membranes has prevented MD systems from being used on a large scale. Hydrophobicity is related to a decreased vapor flux at the same time. Another modified quartz fiber membranes with alkylsilane molecules and discovered that, for DCMD utilizing 0.5 M NaCl as feed, the least wetting-resistant membrane had twice the vapor flux of the most wetting-resistant membrane. Scientists are investigating membranes with particular wettability as a result of this trade-off between vapor flux and wetting resistance [101].
One intriguing direction for research on new desalination technology is the hybridization of two or more processes. The goal of hybridization is to raise the overall efficiency of desalination plants by overcoming the constraints of different systems and attaining comparative advantage [102]. The phrase "hybrid desalination systems" used to only refer to thermal RO systems like MSF-RO and MED-RO; however, more recent literature reveals a wide range of hybrid system choices.
Desalination processes still require a lot of energy and contribute significantly to greenhouse gas (GHG) emissions, despite significant progress in the direction of energy-efficient desalination. Although less than thermal processes, the carbon footprint of Sea Water Reverse Osmosis can reach 6.7 kg CO2 eq/m3 [103]. Due to this, locals and environmental organizations occasionally oppose plans to construct desalination plants. Low-carbon technologies are probably going to be crucial in reducing the environmental impact of desalination and increasing public support for it.
The paper has reviewed solar thermal desalination with more focus on emerging and future trends. Reviewing the available literature, it can be concluded that the recent trends have greatly increased the efficiency of the systems. The emergence of nanoparticles and localized heating in solar desalination systems have greatly benefited in reducing energy consumption and enhancing the performance of the system. The development of photo-thermal materials enabled localized heating at the membrane surface and improve MD performance; solar power combined with emerging processes like membrane distillation (MD) has also had a recent resurgence. The future holds unprecedented improvements in desalination technologies in terms of energy savings. Solar energy's potential to power more contemporary processes like forward osmosis and dewvaporation has also been considered, as well as prospects for hybrid solar PV-wind energy systems. With the availability of special wettability membranes, the rate of vapor transfer has significantly increased. Desalination powered by solar energy is a field of study that is expanding quickly and has made major strides in recent years. Desalination is being driven by renewable energy sources due to rising capacity of technologies, an unavoidable need for mitigating carbon emissions, and the prevention of the harmful consequences of global warming. Particularly, since freshwater shortage and sun irradiation coincide in many locations, an ideal energy source for desalination is solar energy.
There have been fewer experimental studies on the Hybrid MD technology than theoretical ones. The practical results would help in comprehending the feasibility of the system. The concentrated thermal collectors propose a very promising technology to be integrated with the MSF desalination system. The CSP is capable of coordinating Rankine Cycle and when integrated with MSF or MD, it can yield beneficial results. The use of nanoparticle-assisted solar vaporization by using black carbon nanoparticles embedded in electrospun polyvinyl alcohol layered in conventional PVDF MD membrane is limited to the tedious area of the membrane and costly for a greater area. The work on the feasibility of the mechanism for the large area would enable the commercialization of the system.
The authors declare no conflict of interest.
[1] | UNESCO World Water Assessment Programme: UN World Water Development Report 2021: 'Valuing Water', 2021. Available from: https://unesdoc.unesco.org/ark:/48223/pf0000375724. |
[2] | World Resource Institute: Aqueduct projected water stress country rankings, 2015. Tech Note. Available from: https://www.wri.org/research/aqueduct-projected-water-stress-country-rankings. |
[3] |
Al-Othman A, Darwish NN, Qasim M, et al. (2019) Nuclear desalination: A state-of-the-art review. Desalination 457: 39–61. https://doi.org/10.1016/j.desal.2019.01.002 doi: 10.1016/j.desal.2019.01.002
![]() |
[4] |
Ghazi ZM, Rizvi SWF, Shahid WM, et al. (2022). An overview of water desalination systems integrated with renewable energy sources. Desalination 542: 116063. https://doi.org/10.1016/j.desal.2022.116063 doi: 10.1016/j.desal.2022.116063
![]() |
[5] |
Chen C, Jiang Y, Ye Z, et al. (2019) Sustainably integrating desalination with solar power to overcome future freshwater scarcity in China. Global Energy Interconnect 2: 98–113. https://doi.org/10.1016/j.gloei.2019.07.009 doi: 10.1016/j.gloei.2019.07.009
![]() |
[6] |
Brendel LP, Shah VM, Groll EA, et al. (2020) A methodology for analyzing renewable energy opportunities for desalination and its application to Aruba. Desalination 493: 114613. https://doi.org/10.1016/j.desal.2020.114613 doi: 10.1016/j.desal.2020.114613
![]() |
[7] |
Gude VG (2016) Geothermal source potential for water desalination: Current status and future perspective. Renewable Sustainable Energy Rev 57: 1038–1065. https://doi.org/10.1016/j.rser.2015.12.186 doi: 10.1016/j.rser.2015.12.186
![]() |
[8] |
l-Nory M, El-Beltagy M (2014) An energy management approach for renewable energy integration with power generation and water desalination. Renewable Energy 72: 377–385. https://doi.org/10.1016/j.renene.2014.07.032 doi: 10.1016/j.renene.2014.07.032
![]() |
[9] |
Ghaffour N, Lattemann S, Missimer T, et al. (2014). Renewable energy-driven innovative energy-efficient desalination technologies. Appl Energy 136: 1155–1165. https://doi.org/10.1016/j.apenergy.2014.03.033 doi: 10.1016/j.apenergy.2014.03.033
![]() |
[10] |
Fang S, Tu W, Mu L, et al. (2019) Saline alkali water desalination project in Southern Xinjiang of China: A review of desalination planning, desalination schemes and economic analysis. Renewable Sustainable Energy Rev 113: 109268. https://doi.org/10.1016/j.rser.2019.109268 doi: 10.1016/j.rser.2019.109268
![]() |
[11] |
Chen C, Jiang Y, Ye Z, et al. (2019) Sustainably integrating desalination with solar power to overcome future freshwater scarcity in China. Global Energy Interconnect 2: 98–113. https://doi.org/10.1016/j.gloei.2019.07.009 doi: 10.1016/j.gloei.2019.07.009
![]() |
[12] |
Huang L, Jiang H, Wang Y, et al. (2020) Enhanced water yield of solar desalination by thermal concentrated multistage distiller. Desalination 477: 114260. https://doi.org/10.1016/j.desal.2019.114260 doi: 10.1016/j.desal.2019.114260
![]() |
[13] |
Zheng Y, Hatzell KB (2020) Technoeconomic analysis of solar thermal desalination. Desalination 474: 114168. https://doi.org/10.1016/j.desal.2019.114168 doi: 10.1016/j.desal.2019.114168
![]() |
[14] |
Calise F, d'Accadia MD, Piacentino A (2014) A novel solar trigeneration system integrating PVT (photovoltaic/thermal collectors) and SW (seawater) desalination: Dynamic simulation and economic assessment. Energy 67: 129–148. https://doi.org/10.1016/j.energy.2013.12.060 doi: 10.1016/j.energy.2013.12.060
![]() |
[15] |
Thomson M, Miranda MS, Infield D (2003) A small-scale seawater reverse-osmosis system with excellent energy efficiency over a wide operating range. Desalination 153: 229–236. https://doi.org/10.1016/S0011-9164(02)01141-4 doi: 10.1016/S0011-9164(02)01141-4
![]() |
[16] |
Karavas CS, Arvanitis G, Kyriakarakos G, et al. (2018) A novel autonomous PV powered desalination system based on a DC microgrid concept incorporating short-term energy storage. Sol Energy 159: 947–961. https://doi.org/10.1016/j.solener.2017.11.057 doi: 10.1016/j.solener.2017.11.057
![]() |
[17] |
Joyce A, Loureiro D, Rodrigues C, et al. (2001). Small reverse osmosis units using PV systems for water purification in rural places. Desalination 137: 39–44. https://doi.org/10.1016/S0011-9164(01)00202-8 doi: 10.1016/S0011-9164(01)00202-8
![]() |
[18] |
Li Y, Samad S, Ahmed FW, et al. (2020) Analysis and enhancement of PV efficiency with hybrid MSFLA–FLC MPPT method under different environmental conditions. J Cleaner Prod 271: 122195. https://doi.org/10.1016/j.jclepro.2020.122195 doi: 10.1016/j.jclepro.2020.122195
![]() |
[19] |
Delgado-Torres AM, García-Rodríguez L, del Moral MJ (2020) Preliminary assessment of innovative seawater reverse osmosis (SWRO) desalination powered by a hybrid solar photovoltaic (PV)-Tidal range energy system. Desalination 477: 114247. https://doi.org/10.1016/j.desal.2019.114247 doi: 10.1016/j.desal.2019.114247
![]() |
[20] |
Bait O (2020) Direct and indirect solar-powered desalination processes loaded with nanoparticles: A review. Sustainable Energy Technol Assess 37: 100597. https://doi.org/10.1016/j.seta.2019.100597 doi: 10.1016/j.seta.2019.100597
![]() |
[21] |
Maka AO, O'Donovan TS (2020) A review of thermal load and performance characterisation of a high concentrating photovoltaic (HCPV) solar receiver assembly. Sol Energy 206: 35–51. https://doi.org/10.1016/j.solener.2020.05.022 doi: 10.1016/j.solener.2020.05.022
![]() |
[22] |
lminshawy NA, Gadalla MA, Bassyouni M, et al. (2020). A novel concentrated photovoltaic-driven membrane distillation hybrid system for the simultaneous production of electricity and potable water. Renewable Energy 162: 802–817. https://doi.org/10.1016/j.renene.2020.08.041 doi: 10.1016/j.renene.2020.08.041
![]() |
[23] |
Mahmoudi H, Spahis N, Goosen MF, et al. (2009) Assessment of wind energy to power solar brackish water greenhouse desalination units: a case study from Algeria. Renewable Sustainable Energy Rev 13: 2149–2155. https://doi.org/10.1016/j.rser.2009.03.001 doi: 10.1016/j.rser.2009.03.001
![]() |
[24] |
Ma Q, Lu H (2011) Wind energy technologies integrated with desalination systems: Review and state-of-the-art. Desalination 277: 274–280. https://doi.org/10.1016/j.desal.2011.04.041 doi: 10.1016/j.desal.2011.04.041
![]() |
[25] |
Abdelkareem MA, Assad ME, Sayed ET, et al. (2018) Corrigendum to "Recent progress in the use of renewable energy sources to power water desalination plants" (vol 435, pg 97, 2018). Desalination 444: 178–178. https://doi.org/10.1016/j.desal.2018.05.003 doi: 10.1016/j.desal.2018.05.003
![]() |
[26] |
Vargas SA, Esteves GRT, Maçaira PM, et al. (2019) Wind power generation: A review and a research agenda. J Cleaner Prod 218: 850–870. https://doi.org/10.1016/j.jclepro.2019.02.015 doi: 10.1016/j.jclepro.2019.02.015
![]() |
[27] |
Baxter J, Walker C, Ellis G, et al. (2020) Scale, history and justice in community wind energy: An empirical review. Energy Res Soc Sci 68: 101532. https://doi.org/10.1016/j.erss.2020.101532 doi: 10.1016/j.erss.2020.101532
![]() |
[28] |
Díaz H, Soares CG (2020) Review of the current status, technology and future trends of offshore wind farms. Ocean Eng 209: 107381. https://doi.org/10.1016/j.oceaneng.2020.107381 doi: 10.1016/j.oceaneng.2020.107381
![]() |
[29] |
Bundschuh J, Ghaffour N, Mahmoudi H, et al. (2015) Low-cost low-enthalpy geothermal heat for freshwater production: Innovative applications using thermal desalination processes. Renewable Sustainable Energy Rev 43: 196–206. https://doi.org/10.1016/j.rser.2014.10.102 doi: 10.1016/j.rser.2014.10.102
![]() |
[30] |
Aguilar-Jiménez JA, Velázquez N, López-Zavala R, et al. (2020). Low-temperature multiple-effect desalination/organic Rankine cycle system with a novel integration for fresh water and electrical energy production. Desalination 477: 114269. https://doi.org/10.1016/j.desal.2019.114269 doi: 10.1016/j.desal.2019.114269
![]() |
[31] |
Kabay N, Köseoğlu P, Yapıcı D, et al. (2013) Coupling ion exchange with ultrafiltration for boron removal from geothermal water-investigation of process parameters and recycle tests. Desalination 316: 17–22. https://doi.org/10.1016/j.desal.2013.01.027 doi: 10.1016/j.desal.2013.01.027
![]() |
[32] |
Çermikli E, Şen F, Altıok E, et al. (2020) Performances of novel chelating ion exchange resins for boron and arsenic removal from saline geothermal water using adsorption-membrane filtration hybrid process. Desalination 491: 114504. https://doi.org/10.1016/j.desal.2020.114504 doi: 10.1016/j.desal.2020.114504
![]() |
[33] |
Jang J, Kang Y, Han JH, et al. (2020) Developments and future prospects of reverse electrodialysis for salinity gradient power generation: Influence of ion exchange membranes and electrodes. Desalination 491: 114540. https://doi.org/10.1016/j.desal.2020.114540 doi: 10.1016/j.desal.2020.114540
![]() |
[34] |
Ahmed FE, Hashaikeh R, Hilal N (2019) Solar powered desalination—Technology, energy and future outlook. Desalination 453: 54–76. https://doi.org/10.1016/j.desal.2018.12.002 doi: 10.1016/j.desal.2018.12.002
![]() |
[35] |
Reif JH, Alhalabi W (2015) Solar-thermal powered desalination: Its significant challenges and potential. Renewable Sustainable Energy Rev 48: 152–165. https://doi.org/10.1016/j.rser.2015.03.065 doi: 10.1016/j.rser.2015.03.065
![]() |
[36] |
Tarazona-Romero BE, Campos-Celador A, Maldonado-Muñoz YA (2022) Can solar desalination be small and beautiful? A critical review of existing technology under the appropriate technology paradigm. Energy Res Soc Sci 88: 102510. https://doi.org/10.1016/j.erss.2022.102510 doi: 10.1016/j.erss.2022.102510
![]() |
[37] |
Sohani A, Hoseinzadeh S, Berenjkar K (2021) Experimental analysis of innovative designs for solar still desalination technologies; an in-depth technical and economic assessment. J Energy Storage 33: 101862. https://doi.org/10.1016/j.est.2020.101862 doi: 10.1016/j.est.2020.101862
![]() |
[38] |
Rufuss DDW, Iniyan S, Suganthi L, et al. (2016) Solar stills: A comprehensive review of designs, performance and material advances. Renewable Sustainable Energy Rev 63: 464–496. https://doi.org/10.1016/j.rser.2016.05.068 doi: 10.1016/j.rser.2016.05.068
![]() |
[39] |
Shukla A, Kant K, Sharma A (2017) Solar still with latent heat energy storage: A review. Innovative Food Sci Emerging Technol 41: 34–46. https://doi.org/10.1016/j.ifset.2017.01.004 doi: 10.1016/j.ifset.2017.01.004
![]() |
[40] |
Thakur AK, Sathyamurthy R, Sharshir SW, et al. (2021) Performance analysis of a modified solar still using reduced graphene oxide coated absorber plate with activated carbon pellet. Sustainable Energy Technol Assess 45: 101046. https://doi.org/10.1016/j.seta.2021.101046 doi: 10.1016/j.seta.2021.101046
![]() |
[41] |
Shoeibi S, Saemian M, Kargarsharifabad H, et al. (2022) A review on evaporation improvement of solar still desalination using porous material. Int Commun Heat Mass Transfer 138: 106387. https://doi.org/10.1016/j.icheatmasstransfer.2022.106387 doi: 10.1016/j.icheatmasstransfer.2022.106387
![]() |
[42] |
Yin X, Zhang Y, Guo Q, et al. (2018) Macroporous double-network hydrogel for high-efficiency solar steam generation under 1 sun illumination. ACS Appl Mater Interfaces 10: 10998–11007. https://doi.org/10.1021/acsami.8b01629 doi: 10.1021/acsami.8b01629
![]() |
[43] |
Iqbal A, Mahmoud MS, Sayed ET, et al. (2021) Evaluation of the nanofluid-assisted desalination through solar stills in the last decade. J Environ Manage 277: 111415. https://doi.org/10.1016/j.jenvman.2020.111415 doi: 10.1016/j.jenvman.2020.111415
![]() |
[44] |
Parsa SM, Rahbar A, Koleini MH, et al. (2020) A renewable energy-driven thermoelectric-utilized solar still with external condenser loaded by silver/nanofluid for simultaneously water disinfection and desalination. Desalination 480: 114354. https://doi.org/10.1016/j.desal.2020.114354 doi: 10.1016/j.desal.2020.114354
![]() |
[45] |
Yu S, Zhang Y, Duan H, et al. (2015) The impact of surface chemistry on the performance of localized solar-driven evaporation system. Sci Rep 5: 13600. https://doi.org/10.1038/srep13600 doi: 10.1038/srep13600
![]() |
[46] |
Moustafa SMA, Jarrar DI, El-Mansy HI (1985) Performance of a self-regulating solar multistage flash desalination system. Sol Energy 35: 333–340. https://doi.org/10.1016/0038-092X(85)90141-0 doi: 10.1016/0038-092X(85)90141-0
![]() |
[47] |
Garg K, Khullar V, Das SK, et al. (2018) Performance evaluation of a brine-recirculation multistage flash desalination system coupled with nanofluid-based direct absorption solar collector. Renewable Energy 122: 140–151. https://doi.org/10.1016/j.renene.2018.01.050 doi: 10.1016/j.renene.2018.01.050
![]() |
[48] |
Alsehli M (2021) Experimental validation of a solar powered multistage flash desalination unit with alternate storage tanks. Water 13: 2143. https://doi.org/10.3390/w13162143 doi: 10.3390/w13162143
![]() |
[49] |
Babaeebazaz A, Gorjian S, Amidpour M (2021) Integration of a solar parabolic dish collector with a small-scale multi-stage flash desalination unit: Experimental evaluation, exergy and economic analyses. Sustainability 13: 11295. https://doi.org/10.3390/su132011295 doi: 10.3390/su132011295
![]() |
[50] | Vahland S (2013) Analysis of parabolic trough solar energy integration into different geothermal power generation concepts. Available from: http://urn.kb.se/resolve?urn=urn%3Anbn%3Ase%3Akth%3Adiva-129093. |
[51] |
Khoshrou I, Nasr MJ, Bakhtari K (2017) New opportunities in mass and energy consumption of the Multi-Stage Flash Distillation type of brackish water desalination process. Sol Energy 153: 115–125. https://doi.org/10.1016/j.solener.2017.05.021 doi: 10.1016/j.solener.2017.05.021
![]() |
[52] |
Al-Mutaz IS, Wazeer I (2014) Comparative performance evaluation of conventional multi-effect evaporation desalination processes. Appl Therm Eng 73: 1194–1203. https://doi.org/10.1016/j.applthermaleng.2014.09.025 doi: 10.1016/j.applthermaleng.2014.09.025
![]() |
[53] |
Calise F, d'Accadia MD, Piacentino A (2015) Exergetic and exergoeconomic analysis of a renewable polygeneration system and viability study for small isolated communities. Energy 92: 290–307. https://doi.org/10.1016/j.energy.2015.03.056 doi: 10.1016/j.energy.2015.03.056
![]() |
[54] |
Calise F, Cipollina A, d'Accadia MD, et al. (2014) A novel renewable polygeneration system for a small Mediterranean volcanic island for the combined production of energy and water: Dynamic simulation and economic assessment. Appl Energy 135: 675–693. https://doi.org/10.1016/j.apenergy.2014.03.064 doi: 10.1016/j.apenergy.2014.03.064
![]() |
[55] |
Alhaj M, Tahir F, Al-Ghamdi SG (2022) Life-cycle environmental assessment of solar-driven Multi-Effect Desalination (MED) plant. Desalination 524: 115451. https://doi.org/10.1016/j.desal.2021.115451 doi: 10.1016/j.desal.2021.115451
![]() |
[56] |
Liu X, Chen W, Gu M, et al. (2013) Thermal and economic analyses of solar desalination system with evacuated tube collectors. Sol Energy 93: 144–150. https://doi.org/10.1016/j.solener.2013.03.009 doi: 10.1016/j.solener.2013.03.009
![]() |
[57] |
Qasem NA, Lawal DU, Aljundi IH, et al. (2022). Novel integration of a parallel-multistage direct contact membrane distillation plant with a double-effect absorption refrigeration system. Appl Energy 323: 119572. https://doi.org/10.1016/j.apenergy.2022.119572 doi: 10.1016/j.apenergy.2022.119572
![]() |
[58] |
Drioli E, Ali A, Macedonio F (2015) Membrane distillation: Recent developments and perspectives. Desalination 356: 56–84. https://doi.org/10.1016/j.desal.2014.10.028 doi: 10.1016/j.desal.2014.10.028
![]() |
[59] |
Banat F, Jumah R, Garaibeh M (2002) Exploitation of solar energy collected by solar stills for desalination by membrane distillation. Renewable Energy 25: 293–305. https://doi.org/10.1016/S0960-1481(01)00058-1 doi: 10.1016/S0960-1481(01)00058-1
![]() |
[60] |
Thomas N, Mavukkandy MO, Loutatidou S, et al. (2017). Membrane distillation research & implementation: Lessons from the past five decades. Sep Purif Technol 189: 108–127. https://doi.org/10.1016/j.seppur.2017.07.069 doi: 10.1016/j.seppur.2017.07.069
![]() |
[61] |
Banat F, Jwaied N, Rommel M, et al. (2007) Desalination by a "compact SMADES" autonomous solarpowered membrane distillation unit. Desalination 217: 29–37. https://doi.org/10.1016/j.desal.2006.11.028 doi: 10.1016/j.desal.2006.11.028
![]() |
[62] |
Chafidz A, Al-Zahrani S, Al-Otaibi MN, et al. (2014) Portable and integrated solar-driven desalination system using membrane distillation for arid remote areas in Saudi Arabia. Desalination 345: 36–49. https://doi.org/10.1016/j.desal.2014.04.017 doi: 10.1016/j.desal.2014.04.017
![]() |
[63] |
Guillén-Burrieza E, Zaragoza G, Miralles-Cuevas S, et al. (2012) Experimental evaluation of two pilot-scale membrane distillation modules used for solar desalination. J Membr Sci 409: 264–275. https://doi.org/10.1016/j.memsci.2012.03.063 doi: 10.1016/j.memsci.2012.03.063
![]() |
[64] |
Shafieian A, Azhar MR, Khiadani M, et al. (2020) Performance improvement of thermal-driven membrane-based solar desalination systems using nanofluid in the feed stream. Sustainable Energy Technol Assess 39: 100715. https://doi.org/10.1016/j.seta.2020.100715 doi: 10.1016/j.seta.2020.100715
![]() |
[65] | Shafieian Dastjerdi A (2020) A solar‐driven membrane‐based water desalination/purification system. Available from: https://ro.ecu.edu.au/theses/2323. |
[66] |
Ullah R, Khraisheh M, Esteves RJ, et al. (2018) Energy efficiency of direct contact membrane distillation. Desalination 433: 56–67. https://doi.org/10.1016/j.desal.2018.01.025 doi: 10.1016/j.desal.2018.01.025
![]() |
[67] |
González D, Amigo J, Suárez F (2017) Membrane distillation: Perspectives for sustainable and improved desalination. Renewable Sustainable Energy Rev 80: 238–259. https://doi.org/10.1016/j.rser.2017.05.078 doi: 10.1016/j.rser.2017.05.078
![]() |
[68] |
Myyas REN, Al-Dabbasa M, Tostado-Véliz M, et al. (2022) A novel solar panel cleaning mechanism to improve performance and harvesting rainwater. Sol Energy 237: 19–28. https://doi.org/10.1016/j.rser.2017.05.078 doi: 10.1016/j.rser.2017.05.078
![]() |
[69] |
Porrazzo R, Cipollina A, Galluzzo M, et al. (2013) A neural network-based optimizing control system for a seawater-desalination solar-powered membrane distillation unit. Comput Chem Eng 54: 79–96. https://doi.org/10.1016/j.compchemeng.2013.03.015 doi: 10.1016/j.compchemeng.2013.03.015
![]() |
[70] |
Lee JG, Kim WS, Choi JS, et al. (2018) Dynamic solar-powered multi-stage direct contact membrane distillation system: Concept design, modeling and simulation. Desalination 435: 278–292. https://doi.org/10.1016/j.desal.2017.04.008 doi: 10.1016/j.desal.2017.04.008
![]() |
[71] |
Khalifa A, Ahmad H, Antar M, et al. (2017) Experimental and theoretical investigations on water desalination using direct contact membrane distillation. Desalination 404: 22–34. https://doi.org/10.1016/j.desal.2016.10.009 doi: 10.1016/j.desal.2016.10.009
![]() |
[72] |
Elzahaby AM, Kabeel AE, Bassuoni MM, et al. (2016) Direct contact membrane water distillation assisted with solar energy. Energy Convers Manage 110: 397–406. https://doi.org/10.1016/j.enconman.2015.12.046 doi: 10.1016/j.enconman.2015.12.046
![]() |
[73] |
Zuo G, Wang R, Field R, et al. (2011) Energy efficiency evaluation and economic analyses of direct contact membrane distillation system using Aspen Plus. Desalination 283: 237–244. https://doi.org/10.1016/j.desal.2011.04.048 doi: 10.1016/j.desal.2011.04.048
![]() |
[74] |
Ahmed FE, Lalia BS, Hashaikeh R, et al. (2020) Alternative heating techniques in membrane distillation: A review. Desalination 496: 114713. https://doi.org/10.1016/j.desal.2020.114713 doi: 10.1016/j.desal.2020.114713
![]() |
[75] |
Nakoa K, Rahaoui K, Date A, et al. (2016) Sustainable zero liquid discharge desalination (SZLDD). Sol Energy 135: 337–347. https://doi.org/10.1016/j.solener.2016.05.047 doi: 10.1016/j.solener.2016.05.047
![]() |
[76] |
Shafieian A, Khiadani M (2019) A novel solar-driven direct contact membrane-based water desalination system. Energy Convers Manage 199: 112055. https://doi.org/10.1016/j.enconman.2019.112055 doi: 10.1016/j.enconman.2019.112055
![]() |
[77] |
Bouguecha ST, Aly SE, Al-Beirutty MH, et al. (2015) Solar driven DCMD: Performance evaluation and thermal energy efficiency. Chem Eng Res Des 100: 331–340. https://doi.org/10.1016/j.cherd.2015.05.044 doi: 10.1016/j.cherd.2015.05.044
![]() |
[78] |
Huang J, Hu Y, Bai Y, et al. (2020) Novel solar membrane distillation enabled by a PDMS/CNT/PVDF membrane with localized heating. Desalination 489: 114529. https://doi.org/10.1016/j.desal.2020.114529 doi: 10.1016/j.desal.2020.114529
![]() |
[79] |
Ma Q, Ahmadi A, Cabassud C (2018) Direct integration of a vacuum membrane distillation module within a solar collector for small-scale units adapted to seawater desalination in remote places: Design, modeling & evaluation of a flat-plate equipment. J Membr Sci 564: 617–633. https://doi.org/10.1016/j.memsci.2018.07.067 doi: 10.1016/j.memsci.2018.07.067
![]() |
[80] |
Kim YD, Thu K, Ghaffour N, et al. (2013) Performance investigation of a solar-assisted direct contact membrane distillation system. J Membr Sci 427: 345–364. https://doi.org/10.1016/j.memsci.2012.10.008 doi: 10.1016/j.memsci.2012.10.008
![]() |
[81] |
Tlili I, Sajadi SM, Baleanu D, et al. (2022) Flat sheet direct contact membrane distillation study to decrease the energy demand for solar desalination purposes. Sustainable Energy Technol Assess 52: 102100. https://doi.org/10.1016/j.seta.2022.102100 doi: 10.1016/j.seta.2022.102100
![]() |
[82] |
Krnac A, Araiz M, Rana S, et al. (2019) Investigation of direct contact membrane distillation coupling with a concentrated photovoltaic solar system. Energy Procedia 160: 246–252. https://doi.org/10.1016/j.egypro.2019.02.143 doi: 10.1016/j.egypro.2019.02.143
![]() |
[83] |
Laqbaqbi M, García-Payo MC, Khayet M, et al. (2019) Application of direct contact membrane distillation for textile wastewater treatment and fouling study. Sep Purif Technol 209: 815–825. https://doi.org/10.1016/j.seppur.2018.09.031 doi: 10.1016/j.seppur.2018.09.031
![]() |
[84] |
Kumar L, Hasanuzzaman M, Rahim NA (2019) Global advancement of solar thermal energy technologies for industrial process heat and its future prospects: A review. Energy Convers Manage 195: 885–908. https://doi.org/10.1016/j.enconman.2019.05.081 doi: 10.1016/j.enconman.2019.05.081
![]() |
[85] |
Moravej M, Saffarian MR, Li LK, et al. (2020) Experimental investigation of circular flat-panel collector performance with spiral pipes. J Therm Anal Calorim 140: 1229–1236. https://doi.org/10.1007/s10973-019-08879-1 doi: 10.1007/s10973-019-08879-1
![]() |
[86] |
Kumar L, Hasanuzzaman M, Rahim NA, et al. (2021) Modeling, simulation and outdoor experimental performance analysis of a solar-assisted process heating system for industrial process heat. Renewable Energy 164: 656–673. https://doi.org/10.1016/j.renene.2020.09.062 doi: 10.1016/j.renene.2020.09.062
![]() |
[87] |
Dongare PD, Alabastri A, Pedersen S, et al. (2017) Nanophotonics-enabled solar membrane distillation for off-grid water purification. Proc Natl Acad Sci 114: 6936–6941. https://doi.org/10.1073/pnas.1701835114 doi: 10.1073/pnas.1701835114
![]() |
[88] |
Eykens L, De Sitter K, Dotremont C, et al. (2017) Wetting resistance of commercial membrane distillation membranes in waste streams containing surfactants and oil. Appl Sci 7: 118. https://doi.org/10.3390/app7020118 doi: 10.3390/app7020118
![]() |
[89] |
Li C, Li X, Du X, et al. (2020) Elucidating the trade-off between membrane wetting resistance and water vapor flux in membrane distillation. Environ Sci Technol 54: 10333–10341. https://doi.org/10.1021/acs.est.0c02547 doi: 10.1021/acs.est.0c02547
![]() |
[90] |
Xie B, Xu G, Jia Y, et al. (2021) Engineering carbon nanotubes enhanced hydrophobic membranes with high performance in membrane distillation by spray coating. J Membr Sci 625: 118978. https://doi.org/10.1016/j.memsci.2020.118978 doi: 10.1016/j.memsci.2020.118978
![]() |
[91] |
Li J, Ren LF, Zhou HS, et al. (2021) Fabrication of superhydrophobic PDTS-ZnO-PVDF membrane and its anti-wetting analysis in direct contact membrane distillation (DCMD) applications. J Membr Sci 620: 118924. https://doi.org/10.1016/j.memsci.2020.118924 doi: 10.1016/j.memsci.2020.118924
![]() |
[92] |
Zou L, Zhang X, Gusnawan P, et al. (2021) Crosslinked PVDF based hydrophilic-hydrophobic dual-layer hollow fiber membranes for direct contact membrane distillation desalination: from the seawater to oilfield produced water. J Membr Sci 619: 118802. https://doi.org/10.1016/j.memsci.2020.118802 doi: 10.1016/j.memsci.2020.118802
![]() |
[93] |
Yin Y, Jeong N, Tong T (2020) The effects of membrane surface wettability on pore wetting and scaling reversibility associated with mineral scaling in membrane distillation. J Membr Sci 614: 118503. https://doi.org/10.1016/j.memsci.2020.118503 doi: 10.1016/j.memsci.2020.118503
![]() |
[94] |
Martínez‐Díez L, Vázquez‐Gonzàlez MI (1996) Temperature polarization in mass transport through hydrophobic porous membranes. AIChE J 42: 1844–1852. https://doi.org/10.1002/aic.690420706 doi: 10.1002/aic.690420706
![]() |
[95] |
Wang P (2018) Emerging investigator series: the rise of nano-enabled photothermal materials for water evaporation and clean water production by sunlight. Environ Sci: Nano 5: 1078–1089. https://doi.org/10.1039/C8EN00156A doi: 10.1039/C8EN00156A
![]() |
[96] |
Wang Z, Horseman T, Straub AP, et al. (2019). Pathways and challenges for efficient solar-thermal desalination. Sci Adv 5: eaax0763. https://doi.org/10.1126/sciadv.aax0763 doi: 10.1126/sciadv.aax0763
![]() |
[97] |
Lotfy HR, Staš J, Roubík H (2022) Renewable energy powered membrane desalination—review of recent development. Environ Sci Pollut Res 29: 46552–46568. https://doi.org/10.1007/s11356-022-20480-y doi: 10.1007/s11356-022-20480-y
![]() |
[98] |
Ang WL, Mohammad AW, Johnson D, et al. (2019) Forward osmosis research trends in desalination and wastewater treatment: A review of research trends over the past decade. J Water Process Eng 31: 100886. https://doi.org/10.1016/j.jwpe.2019.100886 doi: 10.1016/j.jwpe.2019.100886
![]() |
[99] |
Khan MA, Rehman S, Al-Sulaiman FA (2018) A hybrid renewable energy system as a potential energy source for water desalination using reverse osmosis: A review. Renewable Sustainable Energy Rev 97: 456–477. https://doi.org/10.1016/j.rser.2018.08.049 doi: 10.1016/j.rser.2018.08.049
![]() |
[100] | Ranganathan S (2017) Final Scientific/Technical Report for Program Title: Solar Powered Dewvaporation Desalination System (No. DOE-PTI-15837). Polestar Technologies Inc., Needham Heights, MA (United States). Available from: https://www.osti.gov/biblio/1347924-final-scientific-technical-report-program-title-solar-powered-dewvaporation-desalination-system. |
[101] |
Hamieh BM, Beckman JR (2006) Seawater desalination using dewvaporation technique: Experimental and enhancement work with economic analysis. Desalination 195: 14–25. https://doi.org/10.1016/j.desal.2005.09.035 doi: 10.1016/j.desal.2005.09.035
![]() |
[102] |
Yao M, Tijing LD, Naidu G, et al. (2020) A review of membrane wettability for the treatment of saline water deploying membrane distillation. Desalination 479: 114312. https://doi.org/10.1016/j.desal.2020.114312 doi: 10.1016/j.desal.2020.114312
![]() |
[103] |
Cornejo PK, Santana MV, Hokanson DR, et al. (2014) Carbon footprint of water reuse and desalination: A review of greenhouse gas emissions and estimation tools. J Water Reuse Desalination 4: 238–252. https://doi.org/10.2166/wrd.2014.058 doi: 10.2166/wrd.2014.058
![]() |
1. | Faisal Maqbool, Mujeeb Iqbal Soomro, Laveet Kumar, Khanji Harijan, Modeling and simulation of direct contact membrane distillation system integrated with a photovoltaic thermal for electricity and freshwater production, 2024, 12, 2296-598X, 10.3389/fenrg.2024.1344214 | |
2. | Abdul Wahab, Waqas Javid, Hamza Ahmed, Abdullah Sheikh, Muhammad Shahbaz, Shahid Iqbal, 2024, Enhancing Fresh Water Production in Solar Parabolic Dish Desalination System, 22, 10.3390/materproc2024017022 | |
3. | Dana Alshamaileh, Omar Quran, Mohammad R. Almajali, Study the effect of solar power on the efficiency of desalinating saline water: Case studies Al-Khafji and Gulf of Aqaba areas, 2024, 16, 1687-8132, 10.1177/16878132241290119 | |
4. | Hiba Chebli, Francesco Fornarelli, Nicola Bellantuono, Comparison of Desalination Technologies and Assessment of Their Sustainability, 2023, 2648, 1742-6588, 012021, 10.1088/1742-6596/2648/1/012021 | |
5. | Habib Ben Bacha, Mohammad Alhuyi Nazari, Naeem Ullah, Nehad Ali Shah, Applications of different types of heat pipes in solar desalinations: A comprehensive review, 2024, 89, 0273-1223, 2044, 10.2166/wst.2024.094 | |
6. | Mohamed El-Sayed M. Essa, Hemdan S. El-sayed, Elwy E. El-kholy, Mohammed Amer, Mahmoud Elsisi, Uzair Sajjad, Khalid Hamid, Hilmy El-sayed Awad, Developments in solar-driven desalination: Technologies, photovoltaic integration, and processes, 2024, 25901745, 100861, 10.1016/j.ecmx.2024.100861 | |
7. | Ratan Kumar Das, Abhijit Date, Sustainable water desalination using eductor and waste heat: A review and suggestion for future research, 2025, 603, 00119164, 118687, 10.1016/j.desal.2025.118687 | |
8. | Ernest C. Nwosu, Chibuike Ononogbo, Godswill N. Nwaji, Nnamdi V. Ogueke, Emmanuel E. Anyanwu, Solar-thermal desalination in multi-stage units: a detailed review, 2025, 1573-2975, 10.1007/s10668-025-06044-3 | |
9. | Faisal Maqbool, Laveet Kumar, Mujeeb Iqbal Soomro, Khanji Harijan, Global advancement of solar photovoltaic thermal technologies integrated with membrane distillation systems: a comprehensive review, 2025, 1614-7499, 10.1007/s11356-025-36279-6 |