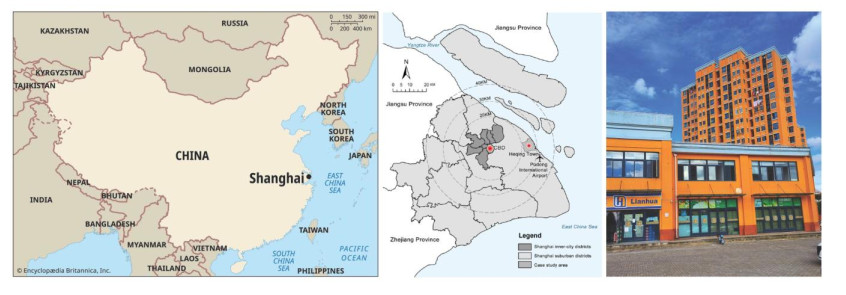
Architectural concrete provides diverse patterns, colors, and forms, offering extensive structural and aesthetic possibilities. In China, advanced techniques such as prefabricated and precast concrete structures are increasingly utilized, delivering benefits like faster construction, reduced resource use, and improved quality control. Recent studies in China have highlighted the environmental benefits and practical considerations of incorporating recycled materials and moderate-heat Portland cement into concrete, which offer promising sustainability advantages. This study, through a case analysis in China, explored the usability, durability, manufacturing costs, and economic implications of architectural concrete. It emphasizes the critical role of architectural concrete in modern structural engineering, financial planning, and design, aiming to reduce variability in strength and uniformity between concrete batches, ensure consistent material quality, and lower maintenance costs while accelerating production. Focusing on quality control in concrete construction in Heqing, Pudong, Shanghai, this research identified unique challenges and provided insights. In Shanghai's architectural context, continuous monitoring of concrete quality is essential for structural stability and durability. This study also addressed the resilience of concrete structures in saltwater and freeze-thaw conditions, underscoring the need to consider environmental factors in quality assurance. Laboratory experiments demonstrated that composite members and deep beams of steel and concrete exhibit notable deformation and shear resistance, highlighting the importance of meticulous material selection and structural design for effective quality control.
Citation: Francis Deng, Armin Mehdipour, Ali Soltani. Construction quality control of concrete structures in architectural engineering—A case in Shanghai, China[J]. Urban Resilience and Sustainability, 2024, 2(3): 256-271. doi: 10.3934/urs.2024013
[1] | Aibin Yan, Dinghan Zheng . Restoration and integration of the Huang Family Garden within the contemporary urban fabric of Shanghai. Urban Resilience and Sustainability, 2024, 2(1): 27-44. doi: 10.3934/urs.2024003 |
[2] | Wei Di Zhang, Jia Chen Liu . Rural public space design in China's western regions: Territorial landscape aesthetics and sustainable development from a tourism perspective. Urban Resilience and Sustainability, 2023, 1(3): 188-213. doi: 10.3934/urs.2023013 |
[3] | Fairuz Amanina, Zul Ilham . Placemaking strategies in greening Universiti Malaya Main Library. Urban Resilience and Sustainability, 2024, 2(1): 76-92. doi: 10.3934/urs.2024005 |
[4] | Irina Di Ruocco . A political concept for the Gragnano Valley of Mills (Valle dei Mulini). Urban redevelopment of cultural-industrial heritage. Urban Resilience and Sustainability, 2023, 1(4): 278-308. doi: 10.3934/urs.2023018 |
[5] | João C. G. Lanzinha . Rehabilitation of existing building parks and its relationship with urban agglomerates–An urgent and demanding task for our common future. Urban Resilience and Sustainability, 2023, 1(2): 86-90. doi: 10.3934/urs.2023006 |
[6] | Sunny Han Han, Yujing Li, Peiheng Yu . What makes a successful industrial heritage park?—China's experience based on the ecosystem cultural services perspective. Urban Resilience and Sustainability, 2024, 2(2): 93-109. doi: 10.3934/urs.2024006 |
[7] | YiFu Hsu, ChunLiang Chen . Service innovation models in cultural districts: A case of Taiwan Yingge Historical Street. Urban Resilience and Sustainability, 2024, 2(4): 371-389. doi: 10.3934/urs.2024020 |
[8] | Shuchang Yi, Qing Liu, Yanze Zhang . High-speed rail construction and market access of cities: Empirical evidence from China. Urban Resilience and Sustainability, 2023, 1(3): 146-162. doi: 10.3934/urs.2023011 |
[9] | Erik Velasco . Circular economy in Singapore: waste management, food and agriculture, energy, and transportation. Urban Resilience and Sustainability, 2024, 2(2): 110-150. doi: 10.3934/urs.2024007 |
[10] | Belhaj Naoufel . Determinants of cities' financial governance for sustainable urban development in Morocco—Case of the regions of Casablanca-Settat and Rabat-Sale-Kenitra. Urban Resilience and Sustainability, 2023, 1(1): 1-19. doi: 10.3934/urs.2023001 |
Architectural concrete provides diverse patterns, colors, and forms, offering extensive structural and aesthetic possibilities. In China, advanced techniques such as prefabricated and precast concrete structures are increasingly utilized, delivering benefits like faster construction, reduced resource use, and improved quality control. Recent studies in China have highlighted the environmental benefits and practical considerations of incorporating recycled materials and moderate-heat Portland cement into concrete, which offer promising sustainability advantages. This study, through a case analysis in China, explored the usability, durability, manufacturing costs, and economic implications of architectural concrete. It emphasizes the critical role of architectural concrete in modern structural engineering, financial planning, and design, aiming to reduce variability in strength and uniformity between concrete batches, ensure consistent material quality, and lower maintenance costs while accelerating production. Focusing on quality control in concrete construction in Heqing, Pudong, Shanghai, this research identified unique challenges and provided insights. In Shanghai's architectural context, continuous monitoring of concrete quality is essential for structural stability and durability. This study also addressed the resilience of concrete structures in saltwater and freeze-thaw conditions, underscoring the need to consider environmental factors in quality assurance. Laboratory experiments demonstrated that composite members and deep beams of steel and concrete exhibit notable deformation and shear resistance, highlighting the importance of meticulous material selection and structural design for effective quality control.
In the realm of civil engineering, the quality of construction materials and methods plays a pivotal role in the durability, safety, and overall performance of structures. As urbanization and infrastructure development continue to advance, the demand for high-quality construction materials and innovative engineering practices has never been greater. Concrete, as a fundamental component in modern construction, is subject to rigorous standards and practices to ensure its effectiveness and longevity. Ensuring the quality of concrete construction necessitates strict adherence to relevant codes and standards, which encompass evaluating strength, durability, and the application of advanced technologies [1,2,3]. Promoting sustainability within the sector is equally vital, with an increasing emphasis on environmental impacts and societal expectations. This shift toward sustainable practices has intensified competition for resources between new and ongoing projects, underscoring the need for comprehensive standards that address both [4]. Future design standards must prioritize performance, serviceability, durability, and safety to effectively address critical aspects of structural integrity and reliability [5]. A design methodology centered on performance is essential for tackling crucial elements of structural integrity, dependability, and intervention methodologies. Moreover, advances in technology and computational models have significantly enhanced our understanding of concrete temperature distributions and related stress challenges. This progress has enabled the development of more effective strategies for managing temperature-related issues in concrete structures.
Concrete structures inherently possess seismic resilience and offer significant benefits in terms of spatial capacity, which can greatly improve the quality of building projects [6]. The process of preparing concrete structures is relatively straightforward and enhances construction efficiency, making them a preferred choice across various engineering applications. Additionally, the development of formwork is a critical aspect of in situ cast-reinforced concrete construction [7]. Achieving precise temperature control during concrete construction is essential and requires a comprehensive approach [8]. This includes optimizing concrete mix ratios, using admixtures to manage adiabatic temperature rise, implementing temperature control techniques during pouring, and closely monitoring temperature variations throughout the curing phase, hence the durability of massive concrete structures can be compromised by delayed ettringite formation and thermal cracking. To address these issues, designers have explored alternative mix designs. The effectiveness of these designs is influenced by the adiabatic temperature rise of the mixtures [9]. In civil construction engineering, there is a growing focus on both the quality of buildings and their aesthetic appeal, alongside cost-effectiveness. However, historical projects often reveal issues such as uneven surfaces and impractical designs that diminish the visual quality of buildings. Therefore, advancing concrete construction technologies is crucial. Such technologies can improve engineering outcomes and surface evenness, thereby addressing the aesthetic needs of civil engineering.
This study investigates the attributes, quality, and durability of exposed concrete, also known as architectural or naked concrete. It focuses on the distinctive characteristics of architectural concrete and the standards for evaluating project excellence. We examine key factors including the color balance of the target area and the proportion of materials that influence cavity characteristics, such as size, material properties, and surface consistency. Achieving high-quality architectural concrete demands advanced technology beyond that required for standard concrete, a necessity reflected in its cost. The study highlights the challenges associated with the surface layers of architectural concrete. Environmental conditions can accelerate degradation, thereby shortening the structure's lifespan. This underscores a significant challenge in construction—the adherence to technology standards. Effective quality control is essential to maintain the durability and quality of concrete structures in architectural engineering while overcoming these technological challenges. This study tries to examine these issues in the context of a case study in Shanghai, China.
In construction projects, leveraging a diverse array of technologies, mechanical equipment, and materials is critical for achieving operational efficiency and project success. China's rapid social and economic advancement has significantly intensified the demands placed on the construction industry, especially in terms of construction quality [10]. This evolving landscape underscores the necessity for professionals in the field to fully grasp the importance of stringent quality and safety controls while navigating the complexities inherent in modern construction tasks [11]. Mastery of advanced technologies and their seamless integration into construction practices are pivotal for enhancing the structural integrity and overall performance of buildings. Construction companies can enhance the safety and longevity of concrete structures by integrating cutting-edge technological advancements and implementing rigorous quality-control procedures [12]. Concrete, being a fundamental component in civil engineering, requires meticulous management to ensure its optimal performance [13]. Proper techniques in pouring and vibration are essential, as they play a crucial role in achieving the desired strength and durability of concrete by ensuring effective compaction and eliminating air voids [14,15]. This approach is essential for avoiding defects that could compromise the structural integrity of the final product.
In the realm of architectural engineering, maintaining the quality of concrete structures involves a comprehensive assessment of various factors. These factors include the precise spacing and clearance of reinforcing bars (rebars), the real-time strength of concrete, the overall structural durability, and the impact of carbonation on the lifespan of reinforced concrete [16]. The deployment of advanced sensing technologies, accurate force monitoring systems, and the application of permanent mold construction techniques are critical for maintaining high-quality standards throughout the construction process. These advanced tools and methods enable real-time monitoring and adjustments, thereby preventing potential issues before they affect the final structure [17]. Moreover, integrating sophisticated structural methods and creating accurate knowledge charts for formal specifications are vital for effective construction management. These practices ensure that all requirements are clearly understood and met, facilitating a smoother construction process. Additionally, efficient strategies implemented in various contexts, such as the successful COVID-19 control measures in Shanghai, provide valuable insights into effective management and safety practices. These strategies highlight the importance of adapting to evolving challenges and incorporating best practices from diverse areas of expertise.
A thorough analysis of rebar arrangement and spacing before the placement of cement is indispensable for ensuring the quality of reinforced concrete. Real-time assessments of cement strength are a fundamental aspect of quality management and provide essential data for effective structural planning and adjustment [18]. Ensuring the durability of concrete structures involves a performance-oriented approach to quality management, adherence to specified durability criteria, and ongoing evaluation of concrete's longevity [19,20]. This holistic approach includes examining factors such as rebar consistency, finishing quality, and the potential effects of carbonation over time [21]. Meanwhile, accurate strength regulation is a key component of ensuring structural stability during construction and throughout the life of the structure [22]. Effective implementation of these methodologies ensures that all construction practices align with current standards and best practices. The ability to adapt and implement effective COVID-19 control measures, as demonstrated by Shanghai, further emphasizes the importance of flexibility and innovation in maintaining safety and efficiency in construction projects [23].
This study highlights the critical role of quality control in architectural engineering construction, addressing key areas such as interlaboratory quality assessments, the impact of creep damage, performance-focused techniques, innovative materials, and durability design. The study emphasizes the importance of precision in quality control protocols and the development of robust steel-concrete composites influenced by nacre. It highlights the need to consider both long-term quality and performance, incorporating probability-based durability and performance-driven concrete quality management. A nonlinear model for creep damage in concrete is introduced, utilizing fractional calculus theory to better understand concrete's response to prolonged strain. Additionally, the research explores the use of inter-laboratory comparative testing as an auxiliary method for evaluating the quality of ready-mixed concrete, extending beyond site-specific assessments.
The study centers on the Hexing residential district located in the suburban area of Shanghai City (Figure 1). The construction project includes a residential building with a total underground area of 86, 563.59 m2 and an above-ground area of 132, 987.16 m2, comprising a ground service area of 2, 908.20 m2, a ground commercial area of 5, 935.69 m², and a ground residential area of 124, 143.27 m2. The building's seismic intensity rating is 8°. The importance of quality control in concrete construction is paramount as it ensures the durability, safety, and performance of buildings. This involves assessing the strength and placement of reinforcing bars, inspecting for corrosion, and using advanced technologies. Controlling thermal cracking and the quality of precast concrete components is vital for reliability. In architectural engineering, achieving concrete durability requires performance-based quality control throughout construction. This includes documenting structural design and construction quality, assessing strength, conducting non-destructive testing, and implementing advanced sensor systems. The use of precast concrete components enhances quality, safety, environmental performance, and construction efficiency. Furthermore, data mining and artificial intelligence have improved inspection procedures, while 3D laser scanning has enhanced the quality assessment process. In summary, construction quality control for concrete structures in architectural engineering involves various measures to ensure the durability, safety, and performance of buildings. These measures include evaluating concrete strength, aligning rebars, conducting corrosion inspections, preventing thermal cracking, and utilizing advanced technologies.
In this case study, the raw materials included cementitious components, coarse aggregates, fine particles, fly ash, and chemical additives. The construction site employed conventional silicate cement with a strength grade of C42.5 [24]. Coarse aggregates, required to be between 5 and 31.5 mm in size, included pebbles and crushed stone [25]. It was crucial to ensure that the maximum aggregate particle size did not exceed three-quarters of the minimum clear distance between steel bars, with mud content limited to 1% and aggregates free from organic impurities [26]. Fine aggregates, such as sand with a fineness modulus ranging from 2.3 to 2.6, had a maximum mud content of 3% and stone content below 8%. Black River medium sand met these specifications and was suitable for the concrete mix. Class II fly ash was incorporated to reduce temperature-induced cracking and enhance concrete strength. The fly ash adhered to specifications with a loss on ignition below 8%, a minimum SiO2 content of 40%, and a maximum SO2 content of 3%, replacing 30% of the cement. The admixture process involved STYUI and HS-II micro-expansion agents during the initial setting, with setting times ranging from 10 to 12 hours for the initial setting and 14 to 16 hours for the final setting. The concrete mix proportions were designed to meet strength requirements after 60 days [27]. Slump measurements were conducted in accordance with mix proportion specifications, with strict controls on water content and optimization of the sand ratio [28]. Water addition was regulated to prevent mix compromise, maintaining a water-to-cement ratio below 0.6 and a sand ratio of approximately 0.4-0.45. Details of the concrete mix proportions used in the project are provided in Table 1.
Cement | Sand | Gravel | Fly ash | Admixture | Water | Water/binder ratio |
320 kg/m3 | 1140 kg/m3 | 620 kg/m3 | 110 kg/m3 | 35 kg/m3 | 165 kg/m3 | 0.35 |
Table 1 outlines the concrete mix composition for the construction project. The cement content is specified at 320 kg/m3, while gravel has a density of 1, 140 kg/m3, and sand is recorded at 620 kg/m3. Fly ash is incorporated at 110 kg/m3, contributing to an improved environmental footprint by reducing the amount of cement used. The water-to-binder ratio is set at 0.35, reflecting a design aimed at achieving higher compressive strength and lower permeability, which is essential for maintaining structural integrity. Additionally, the densities for various components include 35 kg/m3 for an unspecified material and 165 kg/ m3 for hydrogen, though these figures seem unusual for concrete mix specifications. The overall mix design emphasizes a high sand content and a moderate cement concentration to enhance both durability and strength. The use of admixtures likely improves the workability and setting time of the concrete, facilitating a more efficient construction process and better performance of the final structure.
Concrete mixing and transportation: To ensure optimal operation of the equipment, pumps should be strategically deployed around the construction pit. Concrete batching plants must produce concrete materials that meet the specific parameters of the construction site to guarantee both sufficient supply and adherence to quality standards [29]. During the collection phase, accurate measurement of the weights of various raw materials is crucial to ensure their appropriate and rational usage. Concrete slump testing is essential to verify compliance with project construction regulations and control measures [30]. Upon arriving at the construction site, the concrete slump must be retested to confirm that it falls within the range of 160 ± 20 mm to prevent segregation. To facilitate material distribution, six transportation vehicles should be arranged to supply all pumps adequately, with five additional backup vehicles on standby for unforeseen emergencies. It is also necessary to attach a signboard to the front of each concrete transporter upon arrival at the construction site [31]. The precise strength grade of the concrete is critical to avoid confusion and ensure its timely delivery, which is essential for maintaining the project's integrity [32].
Strategic planning of transportation routes for construction materials is crucial to optimize vehicle scheduling and management, ensuring efficient transport even under challenging traffic conditions. Monitoring traffic both inside and outside the construction site is essential for managing logistics effectively, including material deliveries, equipment movements, and worker transportation, to ensure smooth project execution. Optimizing the delivery schedule for ready-mixed concrete (RMC) plays a significant role in reducing CO2 emissions from delivery vehicles, impacting the overall environmental footprint of the project. Ensuring that multiple mixer trucks are available for unloading is necessary to maintain a steady supply of concrete and meet the demands for uninterrupted pouring. Concrete producers must manage their workforce effectively to maintain stringent control over construction sites, ensuring that high-quality concrete is supplied as per project requirements [33]. The concrete should be utilized immediately after mixing and transported to the site within one hour to prevent premature curing. Prior to loading and transportation, it is crucial to clean all pollutants from the hopper to avoid slurry and water accumulation [34]. Organizing shipments efficiently helps minimize waste and maintain a sufficient stock of concrete materials, contributing to the smooth progress of the construction project.
Pre-construction temperature calculation: Before pouring concrete, a comprehensive inspection of both the exterior and interior of the concrete structure is essential. To prevent cracking due to temperature fluctuations, it is crucial to implement precise control techniques. Before beginning the formal pouring process, the building temperature Ti should be accurately determined using Eq (1).
Tj=Ti+(Tq−Ti)(A1+A2+A3) | (1) |
The temperature of the concrete material as it is released from the tank, represented by Ti, is 20.8 ℃, according to Eq (1). The values for Tq are detailed in Table 2. With a mean ambient temperature of 30 ℃, A1 represents the temperature loss associated with the transportation and transfer of concrete materials. The temperature loss coefficient for each loading and unloading cycle is 0.032, applied across three cycles. The value of A2, which quantifies the temperature loss during transportation, is 0.096. This is calculated using the formula A2 = θτ1, where θ represents the amount of heat lost during transportation, and τ1 denotes the transportation duration, which is consistently 30 min per instance. Additionally, A3 accounts for the temperature decrease occurring during pouring and compacting, calculated as A3 = 0.003τ, where τ2 represents the duration of pouring and tamping, which was determined to be 0.06.
The mathematical expression relevant to the research topic is detailed in Eq (1). This equation involves several components: Tj represents the final temperature after a process or reaction, Ti denotes the initial temperature before the process begins, and Tq may indicate a target or critical temperature to be achieved or maintained. The variables A1, A2, and A3 are used to adjust the effect of temperature differences on the outcome. The key findings from this equation suggest a direct relationship between temperature variations and the combined effects of temperature discrepancies and specific coefficients. This approach can be applied for temperature prediction or control within a system, ensuring optimal conditions based on the values of A1, A2, and A3. Understanding how each coefficient impacts the final temperature is essential for precise control in both experimental and industrial settings. After clarifying A1, A2, and A3, we calculated the total of these three, as shown in Eq (2).
∑A=A1+A2+A3=0.096+0.126+0.06=0.282 | (2) |
Equation (2) employs the values 𝐴1 = 0.096, 𝐴2 = 0.126, and 𝐴3 = 0.06. The symbol Σ denotes the sum of these variables, which totals 0.282. This summation might be part of a broader analysis, potentially related to statistical, financial, or scientific data, where accurate summation is essential. The specific values and their aggregate could be pivotal in understanding the context or implications of these variables within the study presented in this paper.
The calculation of Tj value is shown in Eq (3):
Tj=20.8+(30−20.8)×0.282=30.1℃ | (3) |
In this calculation, the base temperature is set at 20.8 ℃, and the target temperature is 30 ℃, with a factor of 0.282 used to adjust the base temperature toward the target. The resulting temperature of 30.1 ℃ demonstrates how the factor of 0.282 effectively moves the base temperature closer to the target. This suggests that precise adjustments are required for accurate temperature control, reflecting the sensitivity of the model or system to changes. Thus, the factor 0.282 is integral to fine-tuning the temperature within the controlled setting, highlighting the importance of exact adjustments in the process being studied.
Concrete placement: A substantial amount of concrete is required for this project, necessitating a layered and segmented pouring approach. Each layer will have a thickness ranging from 600 to 1000 mm and a width of 1.0 m. There will be a one-hour gap between each pouring session, with intensified scrutiny and quality assurance for each step to ensure adherence to specified standards. Given the specific characteristics of the concrete structure used in this study, the pouring process is divided into two stages. Pouring will start from a distance and gradually move closer, beginning at higher points and progressing downward [35]. The transition between these two stages will be marked by the back pouring zone. The pouring will begin in the southern sector and advance toward the northern sector. In constructing the underground garage concrete structure, it is essential to divide the work into two distinct areas: the eastern and western pouring zones. To maintain symmetry, the pouring operation should be carefully coordinated in both the north and south directions, adhering to the established guidelines in a structured manner.
Concrete vibration: To enhance concrete compaction, it is essential to implement vibrations during the pouring process. This involves organizing the insertion points in a staggered or side-by-side configuration with a spacing of 300-400 mm and ensuring uniform distribution. Vibration should be performed at various stages, following the principles of rapid insertion and gradual extraction [36]. To maintain the stability and integrity of adjacent layers, vibration facilities must be carefully inserted into the bottom layer of concrete without causing premature consolidation [37]. The initial time period for continuous vibration at multiple points is set to 30 seconds, but this may be adjusted based on the surface layer's condition during concrete construction. Vibration may cease when there are no air pockets, sinking, or excessive slurry on the surface of the structure. For timely delivery of the pump truck feed information, it should be promptly transmitted to the dispatching room [38]. Considering the construction site's surrounding environment, it is crucial for personnel to implement appropriate control measures. Maintaining a consistent pace throughout the pouring process and ensuring continuous progression of all operations are essential. Each concrete pump must be equipped with three vibrating bars—one positioned in the middle of the inclined plane, one at the discharge point, and one at the foot of the slope, as shown in Figure 1. After completing the multi-area vibration process, the compactness of the entire concrete construction must be evaluated. Construction personnel should adjust the distance, vibration length, and insertion depth for each area to ensure complete control and testing. When discharging materials, a layering technique based on steep slope conditions should be used, with each layer approximately 500 mm thick. Pouring and vibration should be performed sequentially for each layer. Once the quality of a layer is verified to meet the necessary standards, the next layer can be subjected to vibration, and subsequent tasks can follow the established procedures.
Surface treatment of concrete structures: Due to the significant thickness of mud on the concrete surface, water infiltration is a likely issue. To mitigate this problem, several measures can be implemented. Initially, a clapper board or long scraper can be used to pat or scrape the surface. Prior to the initial setting of the concrete, an iron roller should be employed to repeatedly roll and crush the surface, while a wooden wedge can be used to grind the concrete, ensuring compactness and smoothness. Sanding the concrete with wooden wedges helps achieve a dense and even surface. If water infiltration occurs, it is crucial to channel the water to a lower area and use a small pump to remove it. Within 4-8 hours after construction is completed, the surface slurry should be thoroughly cleaned using a long scraper and wooden trowel for scraping and rubbing treatments. This treatment process, ideal for industrial flooring, is valued for its resistance, durability, and anti-dust properties. It involves concrete sanding, applying a concrete hardener to strengthen the surface, using a sealer to minimize water absorption, and final sanding to achieve the desired finish, whether matte, glossy, or a combination. Complete treatment ensures a practical and durable surface. In addition to these concrete maintenance techniques, reducing urban heat islands requires the integration of green infrastructure and efficient building methods. Incorporating elements such as green roofs, urban forests, and reflective surfaces into building designs can decrease heat absorption and enhance energy efficiency.
Concrete structure temperature monitoring: To accurately measure the temperature of both the interior and exterior of concrete structures, it is essential to establish at least four monitoring sites along each testing axis. These measuring points should be positioned within 50 mm of the poured structure's surface to ensure precise readings. To cover the full depth, measuring points should be placed at various depths along the outside, bottom, and middle of the structure, with a spacing of less than 600 mm between points [39]. Temperature measurement points were strategically placed approximately 50 mm above the base of the cast structure to guarantee accurate readings. To ensure precise data, the bottom of the structure was examined by immersing the detection device to a depth of 1 m for 24 h. If no irregularities were detected, the device was considered usable. The device's joints were meticulously regulated, and effective stabilization techniques were applied to prevent damage during vibrations. Central placement of the lead wires on the detection device helped avoid damage to both the wires and the temperature measurement components [40]. Throughout the construction process, the temperatures of both the structure and its surroundings were monitored closely. Measurements were taken at least four times daily, and all data were meticulously recorded. A temperature change graph was then created from this data, visually representing temperature fluctuations in the monitored area [41]. If any anomalies in temperature control data were detected, such as a temperature difference between the interior and exterior exceeding 25 ℃ or an internal temperature surpassing 65 ℃, an alarm was triggered. In such cases, immediate cooling measures were implemented to increase water circulation and address the abnormal temperature readings.
Concrete structures play a vital role in infrastructure, and their proper maintenance is imperative to ensure durability and safety. Diverse methodologies and materials are utilized for the maintenance and restoration of concrete structures, including corrosion surveillance, concrete encasement, carbon nanotube-enhanced cement composites, steel fiber-reinforced concrete, patch repairs, and self-regeneration principles. Implementing preventative maintenance techniques such as fuzzy logic models and reliability-based optimization significantly extends the operational lifespan of concrete structures. Additionally, the use of satellite remote sensing and life cycle cost assessment methods helps identify environmental factors that affect concrete structures and determine the most efficient preventive actions. Textile-reinforced concrete, fissure reduction, and temperature regulation can also be utilized to maintain the integrity of concrete structures.
The maintenance and preservation of concrete structures necessitate a multidisciplinary approach encompassing material research, structural engineering, and environmental impact assessment. Arid conditions at construction sites can lead to moisture accumulation within concrete structures, which subsequently results in cracks and structural deterioration. To preserve moisture, plastic films, vibrant fabric strips, and double geotextiles are used to ensure the structure remains damp [42]. Earth cloth, cotton, and quilt cover are applied to the steel formwork to enhance thermal insulation. The structure should be kept at a minimum temperature of 14 ℃, and dynamic preservation techniques should be employed. A stratified method for insulation layers and enhanced temperature gauging throughout the curing process are recommended. Minimizing water flow and implementing efficient thermal insulation methods can achieve faster cooling rates. To mitigate temperature disparities between the structure and its surroundings, augmenting the thickness of the curing layer is advisable [43].
Table 2 summarizes the maximum variation values for each monitoring point. The highest displacement value was observed at ZY5 (−14.22 mm) on July 27, 2022. The greatest displacement rate was recorded at ZY1 (5.00 mm) on December 15, 2022, with the maximum displacement rate at ZY2 (−3.54 mm/d) on September 20, 2022, and the maximum cumulative displacement at ZY5 (22.655 mm) on December 16, 2022. Displacement measurements ranged between −13.43 and 22.65 mm. The largest cumulative displacement was observed at ZY3 (51.71 mm) on August 27, 2022, with cumulative displacements ranging from −48.08 mm to 51.71 mm. The maximum single displacement was recorded at ZY1 (5.00 mm) on December 15, 2022. The displacement measurements ranged between −13.43 and 22.65 mm.
Maximum single change | Maximum rate of change | Maximum cumulative change | ||||
Point number | Displacement (mm) |
Monitor time | Displacement velocity rate/mm/d |
Monitor time | Displacement (mm) |
Monitor time |
ZY1 | +5.00 | 2022.12.15 | −2.47 | 2022.7.27 | +7.54 | 2022.12.15 |
ZY2 | −3.89 | 2022.10.13 | −3.54 | 2022.9.20 | −3.76 | 2022.10.13 |
ZY3 | −2.76 | 2022.10.17 | −2.45 | 2022.7.27 | −13.43 | 2022.11.19 |
ZY4 | −4.08 | 2022.10.20 | +2.02 | 2022.11.3 | +17.73 | 2022.2.14 |
ZY5 | +3.90 | 2022.12.5 | +1.95 | 2022.12.5 | +22.65 | 2022.12.16 |
ZY6 | +5.00 | 2022.12.15 | −2.47 | 2022.7.27 | +7.54 | 2022.12.15 |
Note: "+" indicates upward movement, "−" indicates downward movement. |
Monitoring of vertical displacement at the top of the support piles: Monitoring the vertical movement at the top of support piles involves evaluating factors such as construction activities, seismic events, and soil conditions. Field monitoring results reveal that construction activities around tunnels create zones of influence affecting both the ground and the piles. Additionally, this study has investigated how inclined piles impact seismic response and lateral displacement of bridge abutments, with numerical simulations offering precise measurements of vertical displacement at the top of the piles. In geotechnical engineering, precise leveling is recognized as the most accurate method for measuring vertical displacements during load tests. Studies have also assessed how the size and distance of vertical pile loads influence lateral deformation in foundation pit-retaining structures. Automated hydrostatic leveling systems provide high-accuracy monitoring of vertical displacements [44]. Consequently, monitoring of vertical movement at the top of support piles usually starts after the completion of the pile crown beam, with an initial set of 36 observations. Design requirements specify that the displacement rate should not exceed 5 mm/d and cumulative displacement should be limited to 25 mm.
Monitoring the settlement of nearby buildings: Settlement monitoring is vital for ensuring the safety and stability of buildings throughout construction activities, offering critical insights into the effects of construction activities on nearby structures, such as shield tunneling, high-rise foundation design, and subway tunnel excavation. Given the complexity of these influences, a thorough and comprehensive analysis is required. This study has highlighted the conservative nature of foundation design for high-rise buildings and the importance of precise settlement estimation methods. Continuous monitoring is essential for detecting subtle changes in settlement patterns, especially in urban areas with dense construction and infrastructure development. During the monitoring process, 26 adjacent structures were observed continuously for signs of subsidence, resulting in a total of 44 monitoring instances. Design requirements specify that the maximum settlement rate should not exceed 3.0 mm per day, and the total cumulative settlement should not surpass 12.0 mm. Table 3 details the highest recorded volume changes at each monitoring point, with the greatest individual settlement of −5.69 mm occurring on June 25, 2022. The highest settlement rate of 2.58 mm per day was observed at JZ2 on October 9, 2022, while the highest cumulative settlement of 11.85 mm was recorded at JZ5 on October 6, 2022. Cumulative settlement values ranged from −5.54 mm to 11.85 mm.
Maximum single change | Maximum rate of change | Maximum cumulative change | ||||
Point number | Displacement (mm) |
Monitor time | Displacement velocity rate/mm/d |
Monitor time | Displacement (mm) |
Monitor time |
JZ1 | +2.44 | 2022.8.25 | +1.22 | 2022.8.25 | −1.35 | 2022.8.23 |
JZ2 | +2.92 | 2022.11.4 | +2.58 | 2022.10.9 | +9.58 | 2022.11.4 |
JZ3 | −1.87 | 2022.12.13 | +1.67 | 2022.8.6 | +2.63 | 2022.8.6 |
JZ4 | −5.69 | 2022.6.25 | −1.90 | 2022.7.27 | −5.54 | 2022.6.25 |
JZ5 | +2.70 | 2022.10.5 | +1.24 | 2022.12.16 | +11.85 | 2022.10.6 |
As depicted in Table 2, the study indicates the highest displacement and velocity fluctuations at various monitoring stations over time, including the monitoring location, maximum single cumulative displacement in millimeters, monitoring time, and the date of maximum displacement. The maximum daily displacement rate is represented in millimeters per day. Data reveal that monitoring points ZY1 and ZY6 exhibited the largest positive displacement (+5.00 mm), indicating significant upward movement. Point ZY4 recorded the highest positive displacement velocity (+2.02 mm/d), suggesting rapid upward change during monitoring. Displacement values varied from −3.89 mm at ZY2 to +5.00 mm at ZY1 and ZY6, demonstrating variability across locations. Displacement velocities ranged from −3.54 mm/d at ZY2 to +2.02 mm/d at ZY4, showing differences in movement rates. The identical maximum displacement and velocity at ZY1 and ZY6 suggest these locations may share similar environmental or structural characteristics. Except for ZY4 and ZY5, all other sites showed negative displacement velocities, indicating a decrease in movement, likely due to localized factors.
Table 3 details the maximum variation values at different monitoring points with respect to displacement changes over time. It includes five monitoring points (JZ1-JZ5) and records the maximum single change, maximum rate of change, and maximum cumulative change, along with monitoring times. JZ2 exhibited the largest single displacement change (+2.92 mm) and cumulative change (+9.58 mm), while JZ4 had the most negative displacement change (−5.69 mm), indicating contraction or similar movement. JZ2 experienced the highest displacement velocity (+2.58 mm/d), whereas JZ4 recorded the lowest (−1.90 mm/d). Significant cumulative displacement increments were observed at JZ2 and JZ5, indicating susceptibility to changes over time. The unfavorable data at JZ4, in both individual and cumulative changes, might indicate different structural reactions or measurement errors. This information is vital for predicting future changes, planning maintenance efforts, and conducting further investigations at these critical sites.
Quality control in concrete engineering faces several challenges, including variability in prefabricated components, incorrect rebar positioning, uneven compressive strength of commercial concrete, distinctive properties of high-performance concrete, and a heavy reliance on visual inspection and labor-intensive measurements. Addressing these issues requires effective assessment techniques and enhanced quality control procedures. The inconsistency of prefabricated concrete components poses a substantial obstacle to constructing concrete structures. The precise rate of reinforcement bar placement and measurement must be meticulously checked before pouring freshly mixed concrete, essential for the construction process. The uneven compressive strength of commercially available concrete highlights the need for improvements in quality control procedures. High-performance concrete structures require special attention to quality. Heavy reliance on visual inspection and labor-intensive measures impedes quality assurance for precast concrete components. Implementing non-destructive testing techniques and varying concrete structures are also essential for enhancing calibration. Conformity control has been shown to improve the quality of approved concrete, emphasizing the importance of conformance standards in influencing service life estimations. Additionally, statistical analysis and artificial intelligence have become indispensable tools for assessing and forecasting concrete's strength, producing high-quality concrete at a lower cost.
Performance-based concrete quality control is implemented during construction by documenting quality and durability. Building Information Modeling (BIM) and 3D laser scanning are used to improve the quality of prefabricated modular construction projects. Sensors and connected project models can enhance construction quality assurance, with real-time quality monitoring and flaw detection preventing construction delays and cost overruns. Total quality management (TQM), focusing on continuous improvement, requires a commitment to learning often misunderstood in the construction sector. TQM extends beyond ISO 9001 and 9002 QA requirements. BIM can also optimize design and increase the efficiency of architectural engineering processes. Automated construction specification processing to support inspection and quality control is required to increase quality control and project quality, as well as a comprehensive, efficient, real-time, and automated quality control system. Finally, the use of optimized BIM-based virtual reality (VR] in architectural engineering design is discussed. In conclusion, performance-based quality control, integration of advanced technologies such as BIM and 3D laser scanning, understanding TQM principles, and collaboration between architectural and engineering professions are essential to improve the quality control of concrete structures in architectural engineering.
The monitoring data for both the horizontal and vertical displacements at the top of the support pile indicated that the measured variations and rates consistently fell within the specified control limits. During excavation, initial displacements exhibited a repeated pattern with relatively high rates. Upon reaching the designated depth, the displacement rate at each monitoring point progressively decelerated and diminished. Subsequent observations showed a tendency toward stabilization in the displacement of each monitoring point. Data on the settlement of adjacent structures indicated that during foundation pit excavation, the settlement rate of monitoring stations for these buildings was relatively low, though sinking and uplift occurrences were noted. The monitoring data suggested that the excavation of the foundation pit had no excessive impact on nearby structures, indicating that the project effectively managed the spatial and temporal impacts of deep foundation pit excavation. The support design was both rational and achievable, ensuring the stability and security of the foundation pit and surrounding structures during construction. This study assessed the structural reliability of engineering designs based on the quality of structural components. It suggests that even modest quality differences can degrade the service life of a structure, highlighting the sensitivity of structural and quality assurance elements to life-cycle reliability. The reliability-based methodology applies to all new concrete structures with on-site or off-site construction parts, especially those with high life cycle performance criteria. Therefore, it is crucial to implement temperature control measures during the construction and maintenance of concrete structures to ensure longevity and reliability. Advanced temperature-control systems, such as insulation and cooling techniques, should be incorporated to closely monitor and manage temperature fluctuations [45,46,47].
For future research, this study recommends focusing on essential technologies for constructing super high-rise buildings, assessing the environmental and health effects of construction activities, integrating sustainable measures, and understanding the impact of state restructuring and innovation on urban development in Shanghai, China. These references offer valuable insights into urban growth, ecological impacts, public health implications, and economic expansion, providing a foundation for future research. By integrating these elements, the quality control of concrete structures in Shanghai's architectural engineering industry can be significantly enhanced.
The authors declare that they have not used Artificial Intelligence (AI) tools in the creation of this article.
The authors are grateful to the informants for their time in discussing the contents of this paper.
The authors declare they have no conflict of interest.
The Authors equally contributed.
[1] |
Mishra V, Sadhu A (2023) Towards the effect of climate change in structural loads of urban infrastructure: A review. Sustain Cities Soc 89: 104352. https://doi.org/10.1016/j.scs.2022.104352 doi: 10.1016/j.scs.2022.104352
![]() |
[2] | Ghosn M, Frangopol DM, McAllister TP, et al. (2016) Reliability-based performance indicators for structural members. J Struct Eng 142: F4016002. https://doi.org/10.1061/(ASCE)ST.1943-541X.0001546 |
[3] |
Alexander M, Beushausen H (2019) Durability, service life prediction, and modelling for reinforced concrete structures–review and critique. Cem Concr Res 122: 17–29. https://doi.org/10.1016/j.cemconres.2019.04.018 doi: 10.1016/j.cemconres.2019.04.018
![]() |
[4] | Li Z, Zhou X, Ma H, et al. (2022) Advanced Concrete Technology, Hoboken: John Wiley & Sons. |
[5] |
Deng F, Hong Z (2013) Focus on sustainable bearing structures. Appl Mech Mater 405: 1182–1186. https://doi.org/10.4028/www.scientific.net/AMM.405-408.1182 doi: 10.4028/www.scientific.net/AMM.405-408.1182
![]() |
[6] |
Zhang S, Teizer J, Lee JK, et al. (2013) Building information modeling (BIM) and safety: Automatic safety checking of construction models and schedules. Autom Constr 29: 183–195. https://doi.org/10.1016/j.autcon.2012.05.006 doi: 10.1016/j.autcon.2012.05.006
![]() |
[7] |
Chang R, Zhang N, Gu Q (2023) A review on mechanical and structural performances of precast concrete buildings. Buildings 13: 1575. https://doi.org/10.3390/buildings13071575 doi: 10.3390/buildings13071575
![]() |
[8] |
Spasiano D, Marotta R, Malato S, et al. (2015) Solar photocatalysis: Materials, reactors, some commercial, and pre-industrialized applications. A comprehensive approach. Appl Catal B-environ 170: 90–123. https://doi.org/10.1016/j.apcatb.2014.12.050 doi: 10.1016/j.apcatb.2014.12.050
![]() |
[9] |
Al-Hasani LE, Park J, Perez G, et al. (2022) Quantifying concrete adiabatic temperature rise based on temperature-dependent isothermal calorimetry; modeling and validation. Mater Struct 55: 191. https://doi.org/10.1617/s11527-022-02023-6 doi: 10.1617/s11527-022-02023-6
![]() |
[10] | Li C (2023) Economic Development of Communist China: An Appraisal of the First Five Years of Industrialization, London: Univ of California Press. |
[11] |
Genc O (2023) Identifying principal risk factors in Turkish construction sector according to their probability of occurrences: a relative importance index (RⅡ) and exploratory factor analysis (EFA) approach. Int J Proj Manag 23: 979–987. https://doi.org/10.1080/15623599.2021.1946901 doi: 10.1080/15623599.2021.1946901
![]() |
[12] | Kibert CJ (2016) Sustainable Construction: Green Building Design and Delivery, Hoboken: John Wiley & Sons. |
[13] |
Mostafaei H, Badarloo B, Chamasemani NF, et al. (2023) Investigating the effects of concrete mix design on the environmental impacts of reinforced concrete structures. Buildings 13: 1313. https://doi.org/10.3390/buildings13051313 doi: 10.3390/buildings13051313
![]() |
[14] | Han B, Zhang L, Ou J (2017) Smart and Multifunctional Concrete toward Sustainable Infrastructures, Singapore: Springer. |
[15] |
Yuan X, Moreu F, Hojati M (2021) Cost-effective inspection of rebar spacing and clearance using RGB-D sensors. Sustainability 13: 12509. https://doi.org/10.3390/su132212509 doi: 10.3390/su132212509
![]() |
[16] |
Yang Z, Tang M, Ji X, et al. (2018) Real-time strength prediction of different types of concrete based on BP neural network. NeuroQuantology 16: 650–656. https://doi.org/10.14704/nq.2018.16.6.1590 doi: 10.14704/nq.2018.16.6.1590
![]() |
[17] |
Gjørv OE (2011) Durability of concrete structures. Arab J Sci Eng 36: 151–172. https://doi.org/10.1007/s13369-010-0033-5 doi: 10.1007/s13369-010-0033-5
![]() |
[18] |
Lee HM, Lee HS, Min S, et al. (2018) Carbonation-induced corrosion initiation probability of rebars in concrete with/without finishing materials. Sustainability 10: 3814. https://doi.org/10.3390/su10103814 doi: 10.3390/su10103814
![]() |
[19] |
Chen J, Chen Y (2019) Research on corrosion detection and assessment method for hydraulic concrete structures. IOP Conf Ser: Mater Sci Eng 300: 052045. https://doi.org/10.1088/1755-1315/300/5/052045 doi: 10.1088/1755-1315/300/5/052045
![]() |
[20] |
Evstigneeva Y, Ibragimov R (2020) Construction technology of fixed formwork and quality control. IOP Conf Ser: Mater Sci Eng 890: 012131. https://doi.org/10.1088/1757-899X/890/1/012131 doi: 10.1088/1757-899X/890/1/012131
![]() |
[21] |
Belentsov YA, Egorov VV, Abu-Khasan MS, et al. (2022) Influence of the accuracy of strength control on the quality of the structures being erected. IOP Conf Ser: Mater Sci Eng 988: 052048. https://doi.org/10.1088/1755-1315/988/5/052048 doi: 10.1088/1755-1315/988/5/052048
![]() |
[22] |
Li J, Liu S, Liu A, et al. (2022) Knowledge graph construction for SOFL formal specifications. Int J Softw Eng Know 32: 605–644. https://doi.org/10.1142/S0218194022500279 doi: 10.1142/S0218194022500279
![]() |
[23] |
Dang Q, Miao R, Liang Y (2020) COVID-19 in Shang Hai: It is worth learning from the successful experience in preventing and controlling the overseas epidemic situation. medRxiv 2020: 20100164. https://doi.org/10.1101/2020.05.13.20100164 doi: 10.1101/2020.05.13.20100164
![]() |
[24] | Wang SR, Wu XG, Yang JH, et al. (2020) Mechanical behavior of lightweight concrete structures subjected to 3D coupled static–dynamic loads. Acta Mech 231: 4497–4511. |
[25] |
Eller B, Movahedi Rad M, Fekete I, et al. (2023) Examination of concrete canvas under quasi-realistic loading by computed tomography. Infrastructures 8: 23. https://doi.org/10.3390/infrastructures8020023 doi: 10.3390/infrastructures8020023
![]() |
[26] |
Fayed S, Madenci E, Özkiliç YO, et al. (2023) Improving bond performance of ribbed steel bars embedded in recycled aggregate concrete using steel mesh fabric confinement. Constr Build Mater 369: 130452. https://doi.org/10.1016/j.conbuildmat.2023.130452 doi: 10.1016/j.conbuildmat.2023.130452
![]() |
[27] |
Le TT, Austin SA, Lim S, et al. (2012) Mix design and fresh properties for high-performance printing concrete. Mater Struct 45: 1221–1232. https://doi.org/10.1617/s11527-012-9828-z doi: 10.1617/s11527-012-9828-z
![]() |
[28] |
Su N, Miao B (2003) A new method for the mix design of medium strength flowing concrete with low cement content. Cem Concr Compos 25: 215–222. https://doi.org/10.1016/S0958-9465(02)00013-6 doi: 10.1016/S0958-9465(02)00013-6
![]() |
[29] | Harris F, McCaffer R, Baldwin A, et al. (2021) Modern Construction Management, Hoboken: John Wiley & Sons. |
[30] |
Tay YWD, Qian Y, Tan MJ (2019) Printability region for 3D concrete printing using slump and slump flow test. Compos B Eng 174: 106968. https://doi.org/10.1016/j.compositesb.2019.106968 doi: 10.1016/j.compositesb.2019.106968
![]() |
[31] | Voss G (2024) Systems Ultra: Making Sense of Technology in a Complex World, London: Verso Books. |
[32] |
Kinable J, Wauters T, Berghe GV (2014) The concrete delivery problem. Comput Oper Res 48: 53–68. https://doi.org/10.1016/j.cor.2014.02.008 doi: 10.1016/j.cor.2014.02.008
![]() |
[33] | Kyriakides S, Corona E (2023) Mechanics of Offshore Pipelines: Volume I: Buckling and collapse, Houston: Gulf Professional Publishing. |
[34] | Jahandari S, Tao Z, Chen Z, et al. (2023) Coal wastes: Handling, pollution, impacts, and utilization, In: The Coal Handbook, Elsevier, 97–163. https://doi.org/10.1016/B978-0-12-824327-5.00001-6 |
[35] |
Sun W, Gao T, Zhao J, et al. (2023) Research on fracture behavior and reinforcement mechanism of fiber-reinforced locally layered backfill: Experiments and models. Constr Build Mater 366: 130186. https://doi.org/10.1016/j.conbuildmat.2022.130186 doi: 10.1016/j.conbuildmat.2022.130186
![]() |
[36] | De Silva CW (2006) Vibration: Fundamentals and Practice, CRC press. |
[37] | Scheffer C, Girdhar P (2004) Practical Machinery Vibration Analysis and Predictive Maintenance, Elsevier. |
[38] |
Weerapura V, Sugathadasa R, De Silva MM, et al. (2023) Feasibility of digital twins to manage the operational risks in the production of a ready-mix concrete plant. Buildings 13: 447. https://doi.org/10.3390/buildings13020447 doi: 10.3390/buildings13020447
![]() |
[39] |
Reyes W, Guzmán A (2011) Evaluation of the slenderness ratio in built-up cold-formed box sections. J Constr Steel Res 67: 929–935. https://doi.org/10.1016/j.jcsr.2011.02.003 doi: 10.1016/j.jcsr.2011.02.003
![]() |
[40] |
Li S, Li J (2017) Condition monitoring and diagnosis of power equipment: Review and prospective. High Volt 2: 82–91. https://doi.org/10.1049/hve.2017.0026 doi: 10.1049/hve.2017.0026
![]() |
[41] | Aguilar E, Auer I, Brunet M, et al. (2003) Guidance on metadata and homogenization. Wmo Td 1186: 1–53. |
[42] |
Al-Barqawi M, Aqel R, Wayne M, et al. (2021) Polymer geogrids: A review of material, design and structure relationships. Materials 14: 4745. https://doi.org/10.3390/ma14164745 doi: 10.3390/ma14164745
![]() |
[43] |
Ellis EA, Springman SM (2001) Full-height piled bridge abutments constructed on soft clay. Geotechnique 51: 3–14. https://doi.org/10.1680/geot.2001.51.1.3 doi: 10.1680/geot.2001.51.1.3
![]() |
[44] |
Liu X, Wang J, Zhou X, et al. (2024) Response of long–short supporting piles due to deep excavation in soil–rock combined strata. Int J Geomech 24: 04023276. https://doi.org/10.1061/IJGNAI.GMENG-8587 doi: 10.1061/IJGNAI.GMENG-8587
![]() |
[45] |
Omrany H, Soebarto V, Sharifi E, et al. (2020) Application of life cycle energy assessment in residential buildings: A critical review of recent trends. Sustainability 12: 351. https://doi.org/10.3390/su12010351 doi: 10.3390/su12010351
![]() |
[46] |
Soltani A, Sharifi E (2017) Daily variation of urban heat island effect and its correlations to urban greenery: A case study of Adelaide. Front Archit Res 6: 529–538. https://doi.org/10.1016/j.foar.2017.08.001 doi: 10.1016/j.foar.2017.08.001
![]() |
[47] |
Soltani A, Sharifi E (2019) Understanding and analysing the urban heat island (UHI) effect in micro-scale. Int J Soc Ecol Sustain Dev (IJSESD) 10: 14–28. https://doi.org/10.4018/IJSESD.2019040102 doi: 10.4018/IJSESD.2019040102
![]() |
Cement | Sand | Gravel | Fly ash | Admixture | Water | Water/binder ratio |
320 kg/m3 | 1140 kg/m3 | 620 kg/m3 | 110 kg/m3 | 35 kg/m3 | 165 kg/m3 | 0.35 |
Maximum single change | Maximum rate of change | Maximum cumulative change | ||||
Point number | Displacement (mm) |
Monitor time | Displacement velocity rate/mm/d |
Monitor time | Displacement (mm) |
Monitor time |
ZY1 | +5.00 | 2022.12.15 | −2.47 | 2022.7.27 | +7.54 | 2022.12.15 |
ZY2 | −3.89 | 2022.10.13 | −3.54 | 2022.9.20 | −3.76 | 2022.10.13 |
ZY3 | −2.76 | 2022.10.17 | −2.45 | 2022.7.27 | −13.43 | 2022.11.19 |
ZY4 | −4.08 | 2022.10.20 | +2.02 | 2022.11.3 | +17.73 | 2022.2.14 |
ZY5 | +3.90 | 2022.12.5 | +1.95 | 2022.12.5 | +22.65 | 2022.12.16 |
ZY6 | +5.00 | 2022.12.15 | −2.47 | 2022.7.27 | +7.54 | 2022.12.15 |
Note: "+" indicates upward movement, "−" indicates downward movement. |
Maximum single change | Maximum rate of change | Maximum cumulative change | ||||
Point number | Displacement (mm) |
Monitor time | Displacement velocity rate/mm/d |
Monitor time | Displacement (mm) |
Monitor time |
JZ1 | +2.44 | 2022.8.25 | +1.22 | 2022.8.25 | −1.35 | 2022.8.23 |
JZ2 | +2.92 | 2022.11.4 | +2.58 | 2022.10.9 | +9.58 | 2022.11.4 |
JZ3 | −1.87 | 2022.12.13 | +1.67 | 2022.8.6 | +2.63 | 2022.8.6 |
JZ4 | −5.69 | 2022.6.25 | −1.90 | 2022.7.27 | −5.54 | 2022.6.25 |
JZ5 | +2.70 | 2022.10.5 | +1.24 | 2022.12.16 | +11.85 | 2022.10.6 |
Cement | Sand | Gravel | Fly ash | Admixture | Water | Water/binder ratio |
320 kg/m3 | 1140 kg/m3 | 620 kg/m3 | 110 kg/m3 | 35 kg/m3 | 165 kg/m3 | 0.35 |
Maximum single change | Maximum rate of change | Maximum cumulative change | ||||
Point number | Displacement (mm) |
Monitor time | Displacement velocity rate/mm/d |
Monitor time | Displacement (mm) |
Monitor time |
ZY1 | +5.00 | 2022.12.15 | −2.47 | 2022.7.27 | +7.54 | 2022.12.15 |
ZY2 | −3.89 | 2022.10.13 | −3.54 | 2022.9.20 | −3.76 | 2022.10.13 |
ZY3 | −2.76 | 2022.10.17 | −2.45 | 2022.7.27 | −13.43 | 2022.11.19 |
ZY4 | −4.08 | 2022.10.20 | +2.02 | 2022.11.3 | +17.73 | 2022.2.14 |
ZY5 | +3.90 | 2022.12.5 | +1.95 | 2022.12.5 | +22.65 | 2022.12.16 |
ZY6 | +5.00 | 2022.12.15 | −2.47 | 2022.7.27 | +7.54 | 2022.12.15 |
Note: "+" indicates upward movement, "−" indicates downward movement. |
Maximum single change | Maximum rate of change | Maximum cumulative change | ||||
Point number | Displacement (mm) |
Monitor time | Displacement velocity rate/mm/d |
Monitor time | Displacement (mm) |
Monitor time |
JZ1 | +2.44 | 2022.8.25 | +1.22 | 2022.8.25 | −1.35 | 2022.8.23 |
JZ2 | +2.92 | 2022.11.4 | +2.58 | 2022.10.9 | +9.58 | 2022.11.4 |
JZ3 | −1.87 | 2022.12.13 | +1.67 | 2022.8.6 | +2.63 | 2022.8.6 |
JZ4 | −5.69 | 2022.6.25 | −1.90 | 2022.7.27 | −5.54 | 2022.6.25 |
JZ5 | +2.70 | 2022.10.5 | +1.24 | 2022.12.16 | +11.85 | 2022.10.6 |