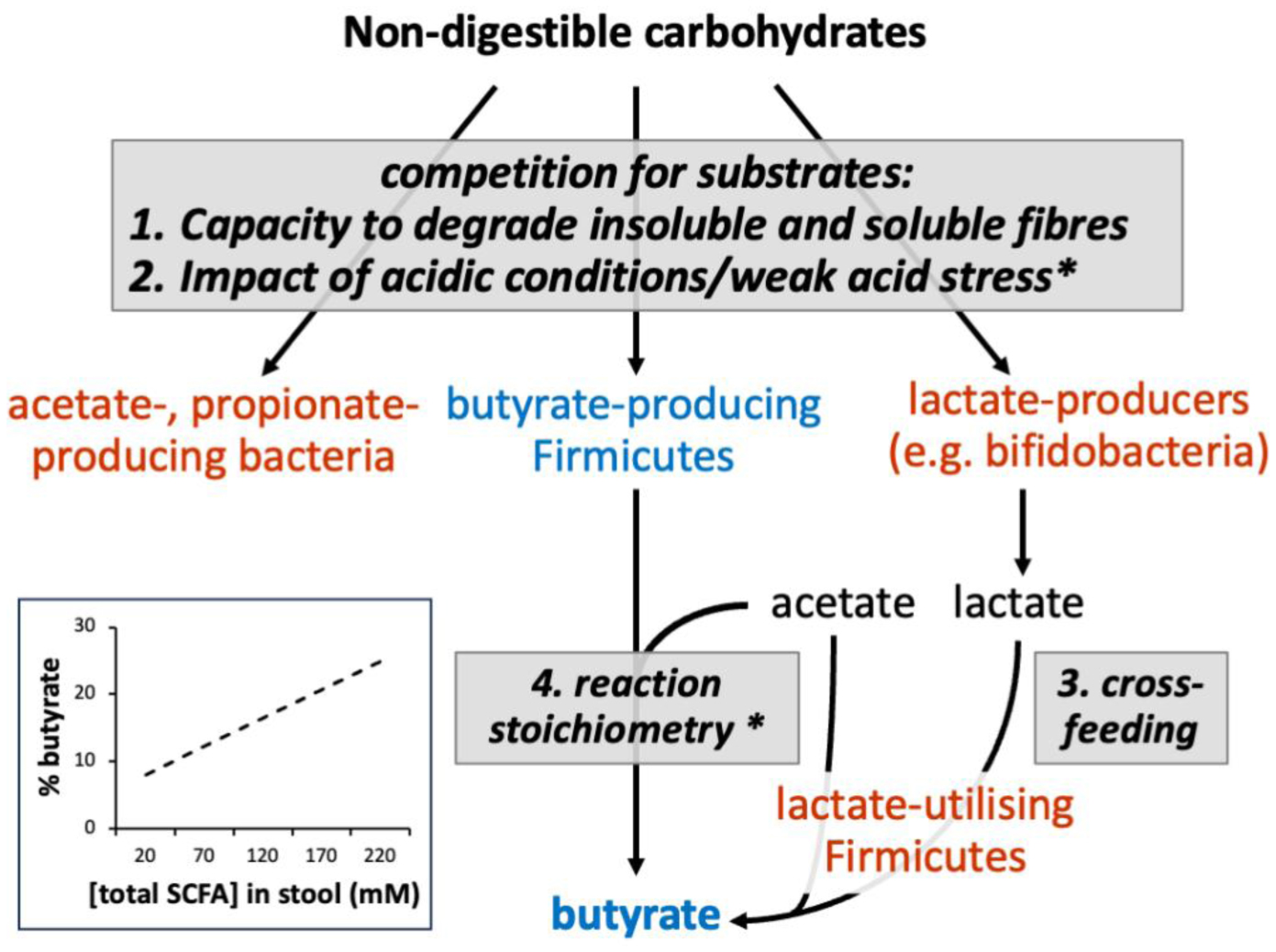
The microbial community of the human large intestine mainly ferments dietary fiber to short chain fatty acids (SCFAs), which are efficiently absorbed by the host. The three major SCFAs (acetate, propionate, and butyrate) have different fates within the body and different effects on health. A recent analysis of 10 human volunteer studies established that the proportions of these SCFA in fecal samples significantly shifted towards butyrate as the overall concentration of SCFA increased. Butyrate plays a key role in gut health and is preferentially utilized as an energy source by the colonic epithelium. Here we discuss possible mechanisms that underlie this ‘butyrate shift’; these include the selection for butyrate-producing bacteria within the microbiota by certain types of fiber, and the possibility of additional butyrate formation from lactate and acetate by metabolite cross-feeding. However, a crucial factor appears to be the pH in the proximal colon, which decreases as the SCFA concentrations increase. A mildly acidic pH has been shown to have an important impact on microbial competition and on the stoichiometry of butyrate production. Understanding these complex interactions has been greatly aided by the refinement of theoretical models of the colonic microbiota that assume a small number (10) of microbial functional groups (MFGs).
Citation: Harry J. Flint, Petra Louis, Sylvia H. Duncan. Why does increased microbial fermentation in the human colon shift toward butyrate?[J]. AIMS Microbiology, 2024, 10(2): 311-319. doi: 10.3934/microbiol.2024016
[1] | Javier Fernández, Sául Redondo-Blanco, Elisa M. Miguélez, Claudio J. Villar, Alfonso Clemente, Felipe Lombó . Healthy effects of prebiotics and their metabolites against intestinal diseases and colorectal cancer. AIMS Microbiology, 2015, 1(1): 48-71. doi: 10.3934/microbiol.2015.1.48 |
[2] | Alaa Emara Rabee, Khalid Z. Kewan, Hassan M. El Shaer, Mebarek Lamara, Ebrahim A. Sabra . Effect of olive and date palm by-products on rumen methanogenic community in Barki sheep. AIMS Microbiology, 2022, 8(1): 26-41. doi: 10.3934/microbiol.2022003 |
[3] | Abdullahi Yusuf Muhammad, Malik Amonov, Chandrika Murugaiah, Atif Amin Baig, Marina Yusoff . Intestinal colonization against Vibrio cholerae: host and microbial resistance mechanisms. AIMS Microbiology, 2023, 9(2): 346-374. doi: 10.3934/microbiol.2023019 |
[4] | Zakaria M. Solaiman, Paul Blackwell, Muhammad Izhar Shafi, Nariman D. Salman, Paul Storer, Emre Babur . Co-application of biochar and phosphorus increases soil microbial biomass, mycorrhizal colonization, growth, and nutrition of subterranean clover. AIMS Microbiology, 2025, 11(1): 59-73. doi: 10.3934/microbiol.2025004 |
[5] | Moh. A'inurrofiqin, Endang Sutriswati Rahayu, Dian Anggraini Suroto, Tyas Utami, Yunika Mayangsari . Safety assessment of the indigenous probiotic strain Lactiplantibacillus plantarum subsp. plantarum Kita-3 using Sprague–Dawley rats as a model. AIMS Microbiology, 2022, 8(4): 403-421. doi: 10.3934/microbiol.2022028 |
[6] | MP Lizardo, FK Tavaria . Probiotic growth in skin-like conditions. AIMS Microbiology, 2022, 8(4): 388-402. doi: 10.3934/microbiol.2022027 |
[7] | Chika C. Anotaenwere, Omoanghe S. Isikhuemhen, Peter A. Dele, Michael Wuaku, Joel O. Alabi, Oludotun O. Adelusi, Deborah O. Okedoyin, Kelechi A. Ike, DeAndrea Gray, Ahmed E. Kholif, Uchenna Y. Anele . Ensiled Pleurotus ostreatus based spent mushroom substrate from corn: In vitro gas production, greenhouse gas emissions, nutrient degradation, and ruminal fermentation characteristics. AIMS Microbiology, 2025, 11(1): 1-21. doi: 10.3934/microbiol.2025001 |
[8] | Smita H. Panda, Jyothsna Khanna Goli, Sushrirekha Das, NakulanandaMohanty . Production, optimization and probiotic characterization of potential lactic acid bacteria producing siderophores. AIMS Microbiology, 2017, 3(1): 88-107. doi: 10.3934/microbiol.2017.1.88 |
[9] | Marina Santiago, Lindsay Eysenbach, Jessica Allegretti, Olga Aroniadis, Lawrence J. Brandt, Monika Fischer, Ari Grinspan, Colleen Kelly, Casey Morrow, Martin Rodriguez, Majdi Osman, Zain Kassam, Mark B. Smith, Sonia Timberlake . Microbiome predictors of dysbiosis and VRE decolonization in patients with recurrent C. difficile infections in a multi-center retrospective study. AIMS Microbiology, 2019, 5(1): 1-18. doi: 10.3934/microbiol.2019.1.1 |
[10] | Ananya Mukherjee, Puja Bhattacharjee, Rituparna Das, Arundhati Pal, Amal K. Paul . Endophytic bacteria with plant growth promoting abilities from Ophioglossum reticulatum L.. AIMS Microbiology, 2017, 3(3): 596-612. doi: 10.3934/microbiol.2017.3.596 |
The microbial community of the human large intestine mainly ferments dietary fiber to short chain fatty acids (SCFAs), which are efficiently absorbed by the host. The three major SCFAs (acetate, propionate, and butyrate) have different fates within the body and different effects on health. A recent analysis of 10 human volunteer studies established that the proportions of these SCFA in fecal samples significantly shifted towards butyrate as the overall concentration of SCFA increased. Butyrate plays a key role in gut health and is preferentially utilized as an energy source by the colonic epithelium. Here we discuss possible mechanisms that underlie this ‘butyrate shift’; these include the selection for butyrate-producing bacteria within the microbiota by certain types of fiber, and the possibility of additional butyrate formation from lactate and acetate by metabolite cross-feeding. However, a crucial factor appears to be the pH in the proximal colon, which decreases as the SCFA concentrations increase. A mildly acidic pH has been shown to have an important impact on microbial competition and on the stoichiometry of butyrate production. Understanding these complex interactions has been greatly aided by the refinement of theoretical models of the colonic microbiota that assume a small number (10) of microbial functional groups (MFGs).
The short chain fatty acids (SCFA) produced by microbial fermentation of digestive residues (fiber) in the human large intestine have multiple effects on gut and systemic health [1]. This makes it important to understand what factors affect the production rates and relative concentrations of these acids in the colon. A recent paper by LaBouyer et al. (2022) [2] analyzed data on fecal SCFA concentrations from 158 volunteers at baseline (i.e., before any dietary intervention) in 10 previous human studies conducted at Aberdeen, UK. This analysis revealed some highly significant relationships between increasing total SCFA concentrations and the proportion of SCFA accounted for by branched chain fatty acids (BCFA, which declined) and by butyrate (which increased). The decline in the BCFA proportion is easily accounted for by assuming that an increased total SCFA mainly results from an increased fermentation of carbohydrate fiber. Since BCFA are only derived from fermentation of branched chain amino acids, this is consistent with the fraction of digestive residues arriving in the colon that is derived from protein decreasing with a higher fermentable fiber intake. However, it is much less obvious why the proportion of butyrate should increase. Given the importance of butyrate for colonic health [1],[3], this question needs to be considered in greater detail.
Why should the proportion of butyrate among acidic fermentation products of microbial fermentation increase as more digestive residue is fermented? Research from human studies, chemostat models, and isolated human colonic bacteria suggest four possible explanations for this phenomenon that are discussed below (Figure 1). In two of these, colonic pH plays the key role. It is well established that the colonic pH (especially in the proximal colon) in vivo decreases as the overall concentration of acids produced by fermentation increases [4],[5]. A typical pH in the proximal colon is often measured around 5.5, rising to 6.5 in the distal colon [4]–[6]. It is important to note that the highly abundant Bacteroidota (synonym Bacteroidetes) mainly produces propionate and acetate, but no butyrate, while butyrate production is a characteristic of certain genera of Bacillota (synonym Firmicutes) [7].
Different strategies for accessing carbohydrate fibers are used by different groups of colonic bacteria. The ‘sequestration’ systems found in Bacteroidetes are equipped to capture soluble carbohydrates, whereas the extracellular enzyme systems and attachment mechanisms found in certain Firmicutes and bifidobacteria may be superior in accessing insoluble substrates such as resistant starch and plant cell wall material [8]. Butyrate-producing Firmicutes can potentially utilize a wide variety of fiber-derived carbohydrates [9]. In a carefully controlled human volunteer study, wheat bran NSP fiber was found to increase the relative abundance of certain Firmicutes, especially Lachnospiraceae, among the fecal microbiota [10]. A follow-up study conducted in vitro at a controlled pH of 6.5 identified butyrate-producing Firmicutes that became enriched by association with insoluble wheat bran [11].
Another human study showed that reducing dietary fiber (and total carbohydrate) intake decreased the absolute numbers and relative abundance of butyrate-producing Firmicutes related to Roseburia and E. rectale [12]. This suggests that some butyrate producers could be directly promoted by certain fiber sources. Meanwhile, there is good evidence that lactate-producing Actinobacteria, especially bifidobacteria, are promoted by carbohydrate fibers, including prebiotics [13].
A slightly acidic pH is known to have differential effects on the bacterial growth rates [14]. Human colonic anaerobes tested in pure cultures showed a range of pH sensitivities in the presence of physiological concentrations of fermentation acids, with Bacteroides strains among those showing the greatest sensitivity to a pH of 5.5, whereas many butyrate-producing Firmicutes showed a greater tolerance [15]. This must reflect differences in physiological responses to pH changes and a sensitivity to weak acids [16],[17]. Studies examining communities of human colonic bacteria maintained in anaerobic, pH-controlled, continuous flow fermenters supplied with soluble fibers have shown that the Gram-negative Bacteroides dominate the community at near neutral pH values around 6.5 [18]–[20]. Bacteroides spp. mainly produce acetate and propionate, which were the major metabolites detected at this pH; however, lower pH values resulted in a shift towards either butyrate or lactate. A suppression of Bacteroides growth at a lower pH appears to help species more tolerant of acidic pH levels, including butyrate-producing Firmicutes and lactate-producing bifidobacteria, to compete for the carbohydrate substrates supplied, thus increasing their relative abundance in the community [18]–[20]. A negative correlation between the fecal pH and the relative abundance of butyrate-producing Roseburia-related bacteria has been reported in vivo [2].
Certain Firmicutes bacteria (Anaerostipes hadrus, A. caccae, Anaerobutyricum hallii, A. soehngenii) have the ability to convert lactate and acetate into butyrate [21],[22]. Provided that the pH is not too low, this results in an efficient conversion within the microbial community of lactate (e.g., produced by bifidobacteria and lactobacilli) into butyrate [20],[23]. It is well established that the relative abundance of bifidobacteria can be promoted by many different dietary fibers, including prebiotics, and by an acidic pH, thus potentially increasing the supply of lactate in the colon.
In the predominant Firmicutes bacteria that are major producers of butyrate, the final step in butyrate formation is achieved via the enzyme butyryl-CoA:acetate CoA-transferase, which utilizes acetate and butyryl-CoA as substrates (Figure 2) [7]. This often involves the net uptake of acetate, such that the carbon in butyrate is partly derived from carbohydrate fermentation and partly from acetate [24]. In isolates of Roseburia spp. and Faecalibacterium spp., it has been shown that a slightly more acidic pH leads to a greater uptake of acetate and a greater production of butyrate for every mol of glucose fermented [7],[25]. In a study that involved batch culture incubations with mixed faecal microbiota, the composition of the microbial community was compared with the metabolites produced [26], taking the phylogenetic distribution of the relevant fermentation pathways into account [7]. At a given % of potential propionate producers within the community, there was no impact of the initial pH (5.5 or 6.5) on the % propionate among the SCFA products. In marked contrast, for any given % of butyrate producers within the community, there was a higher butyrate % among the SCFA products at a pH of 5.5 compared to at a pH of 6.5 [26]. This strongly suggests that more acidic conditions are likely to boost butyrate production in the mixed community in vivo simply because of a shift in the stoichiometry of butyrate formation.
The aforementioned explanations should not be regarded as strict alternatives, and all four mechanisms may play a role. It is not easy to distinguish the impact of a decreasing pH (a consequence of fermentation) on microbial competition (2, above) from the possible selective promotion of butyrate producers by certain fiber sources (1, above). Indeed, the decrease in the colonic pH may be largely responsible for the promotion of butyrate by fiber. It is also likely that these different mechanisms will vary in importance between individuals, which is influenced by diet choices and differences in the microbiota composition (in particular, the representation of butyrate producers, bifidobacteria, and lactate utilizers (Figure 1)).
Individuals also vary in the representation of methanogenic archaea within their gut microbiota. Methanogens, especially Methanobrevibacter smithii, are hydrogenotrophs whose activity can depress the concentration of hydrogen ([H2]) resulting from fermentation. Recent work indicated that [H2] in the colon contents had the potential to influence the metabolism and populations of butyrate-producing bacteria within the microbial community [27]. Responses differed between major butyrate-producing species Faecalibacterium prausnitzii, Roseburia intestinalis, and Eubacterium rectale, depending in part on whether they possess a ferredoxin hydrogenase that enables the production of hydrogen as an electron sink. This suggests that colonic [H2] may have an important influence on growth and the metabolism of some butyrate producers by shifting the metabolism toward a higher relative production of fermentation acids at the expense of hydrogen. It is also suggested that high fiber intakes will tend to increase H2 production by fermentation, with the hydrogenotrophs within the community (acetogens, sulfate-reducing bacteria and methanogenic archaea) failing to utilize all of the H2 produced. Methanogenic individuals have been reported to show lower fecal butyrate concentrations as compared to non-methanogenic individuals [27],[28], although differences in gut transit could also be a factor here. It can also be noted that the supply of vitamins and trace nutrients have the potential to alter populations of individual butyrate producers, many of which lack the ability to synthesize essential vitamins [29]. Moreover, butyrate-producing species widely vary in their oxygen sensitivity and responses to low oxygen concentrations [30]. Oxygen consumption accompanying butyrate oxidation by epithelial cells may play a particularly important role in determining the microenvironment of the intestinal mucosa [31].
With such a complex ecosystem, it is important to critically examine the explanatory hypotheses. For example, are the differences in the growth rate and pH sensitivities observed between isolated strains sufficient to explain the pH-driven shifts in the microbiota composition and SCFA ratios that occur within the complex microbial community? To address this, some years ago, a theoretical model was developed to represent the human colonic microbiota and its major metabolic products. This model postulated 10 microbial functional groups (MFG) that differed, among other things, in the substrate preferences, metabolic end products, and pH sensitivities, and made use of experimental data on growth rates and pH sensitivities obtained from isolated bacteria [25]. The model has remarkably proved successful in predicting the transition seen in the microbial community and metabolite profiles between mildly acidic and near neutral pHs in our chemostat studies [18],[20].
The same theoretical model has recently been greatly refined [32] to make it relevant to the interpretation of data obtained from in vivo studies. Refinement required the introduction of multiple gut compartments, gut transit, mucosal absorption of SCFA and water, and a link between the local SFCA concentration and the gut luminal pH. We can note that, retaining our assumptions on the differential sensitivities of the MFG to the pH, the refined model predicted an increase in the butyrate proportion, with an increasing total concentration of fermentation acids within the feces [32]. Importantly, this modelling helped to establish that the explanations for the observed ‘butyrate shift’ given above are consistent at a rigorous quantitative level, with the experimental data from multiple human studies reported by LaBouyer et al. [2].
Is the ‘butyrate shift’ that accompanies increased fermentation simply an unavoidable consequence of the fundamental biochemistry and microbial ecology of the large intestinal microbiota? Or could this phenomenon be modulated by different host physiological responses? If the latter is true, then this implies that there is a net benefit to the host from the ‘butyrate shift’. The pH within the proximal colon is broadly under the control of the host through the secretion of bicarbonate that tends to neutralize fermentation acids and provides buffering [33]. Therefore, this ability to regulate the colonic pH should allow the host some control over the supply of butyrate relative to that of the other fermentation acids. Since the colonic epithelium uses butyrate as its preferred energy source, an enhanced butyrate supply in healthy individuals can contribute to colonic health by maximizing the trophic effect of microbially-produced butyrate in maintaining a healthy mucosal barrier function [1],[3],[34]. This might explain why the pH is often allowed to drop into the mid-pH 5 range in the proximal colon [5]. AN adequate butyrate supply is considered an important factor in the prevention of colorectal cancer through its multiple influences on the physiology of epithelial cells [35]. Furthermore, Roediger [36] proposed that inflammatory bowel disease could be considered an energy deficiency condition, as the SCFA (especially butyrate) supply is often severely disrupted. We now know that this disruption is accompanied by major changes in the intestinal microbiota, especially a loss of butyrate producing Firmicutes [37]. While the factors involved are complex, and an interplay with the host's immune system is crucial, departure from the normal balance of fermentation and the energy supply remains an important feature of the pathogenesis of these conditions [31]. Indeed, the fecal pH can become too acidic in severe colitis, to the point of acidosis, where production of both propionate and butyrate can become compromised and lactate accumulates, this emphasizing the very fine balance that is required for healthy gut function [20],[38]. We should also note that high rates of fermentation can have a negative health impact for some individuals, especially those suffering from inflammatory bowel diseases and irritable bowel disorders [39]. In conclusion, a better understanding of the impact of pH-dependent shifts in fermentation patterns within the human colon is very important if we are to properly understand the role of diet and the gut microbiota in overall health. There is a need for more comprehensive human volunteer studies that combine fecal microbiota profiling with dietary intake data and reliable measurements of fecal (or colonic) pH, SCFA, and breath hydrogen. These would include new dietary intervention studies that involve fiber sources. For a more complete picture, it would also be important to try to monitor gut transit in such studies. Careful data analyses, together with theoretical modelling, should then reveal the importance of inter-individual microbiota variation and factors such as pH, dietary fiber, and transit in determining SCFA and butyrate outputs. The butyrate producing capacity can be estimated from DNA sequence data and related to the examined butyrate concentration [26]. In addition, studies on isolated butyrate-producing bacteria, including work with a defined consortia, could help us to understand the ecology of these organisms and explain their behavior within the complex community in vivo [27],[29],[30].
No potential conflicts of interest were disclosed.
[1] | Liu P, Wang Y, Yang G, et al. (2020) The role of short chain fatty acids in intestinal barrier function, inflammation, oxidative stress, and colonic carcinogenesis. Pharm Res 165: 1054200. https://doi.org/10.1016/j.phrs.2021.105420 |
[2] |
LaBouyer M, Holtrop G, Horgan G, et al. (2022) Higher total faecal short-chain fatty acid concentrations correlate with increasing proportions of butyrate and decreasing proportions of branched-chain fatty acids across multiple human studies. Gut Microbiome 3: e2. https://doi.org/10.1017/gmb.2022.1 ![]() |
[3] |
Hamer HM, Jonkers D, Venema K, et al. (2008) Research article: the role of butyrate on colonic function. Aliment Pharm Therap 27: 104-119. https://doi.org/10.1111/j.1365-2036.2007.03562.x ![]() |
[4] | Macfarlane GT, Gibson GR, Cummings JH (1992) Comparison of fermentation reactions in different regions of the human colon. J Appl Bacteriol 72: 57-64. https://doi.org/10.1111/j.1365-2672.1992.tb04882.x |
[5] |
Bown RL, Gibson JA, Sladen PJ, et al. (1974) Effects of lactulose and other laxatives on ileal and colonic pH as measured by radiotelemetry device. Gut 15: 999-1004. https://doi.org/10.1136/gut.15.12.999 ![]() |
[6] |
Yamamura R, Inoue KM, Nishino K, et al. (2023) Intestinal and fecal pH in human health. Front Microbiomes 2: 1192316. https://doi.org/10.3389/frmbi.2023.1192316 ![]() |
[7] |
Louis P, Flint HJ (2017) Formation of propionate and butyrate by the human colonic microbiota. Environ Microbiol 19: 29-41. https://doi.org/10.1111/1462-2920.13589 ![]() |
[8] |
Flint HJ (2020) How gut microorganisms make use of available carbohydrates. Why Gut Microbes Matter.Springer Fascinating Life Sciences 81-96. https://doi.org/10.1007/978-3-030-43246-1_7 ![]() |
[9] |
Fu XD, Lin ZM, Zhu CL, et al. (2019) Nondigestible carbohydrates, butyrate and butyrate-producing bacteria. Crit Rev Food Sci Nutr 59: S130-S152. https://doi.org/10.1080/10408398.2018.1542587 ![]() |
[10] |
Salonen A, Lahti L, Salojärvi J, et al. (2014) Impact of diet and individual variation on intestinal microbiota composition and fermentation products in obese men. ISME J 8: 2218-2230. https://doi.org/10.1038/ismej.2014.63 ![]() |
[11] |
Duncan SH, Russell WR, Quartieri A, et al. (2016) Wheat bran promotes enrichment within the human colonic microbiota of butyrate-producing bacteria that release ferulic acid. Environ Microbiol 18: 2214-2225. https://doi.org/10.1111/1462-2920.13158 ![]() |
[12] |
Duncan SH, Belenguer A, Holtrop G, et al. (2007) Reduced dietary intake of carbohydrate by obese subjects results in decreased concentrations of butyrate and butyrate-producing bacteria in feces. Appl Environ Microbiol 73: 1073-1078. https://doi.org/10.1128/AEM.02340-06 ![]() |
[13] |
Kleessen B, Schwarz S, Boehm A, et al. (2007) Jerusalem artichoke and chicory inulin in bakery products affect faecal microbiota in healthy volunteers. Br J Nutr 98: 540-549. https://doi.org/10.1017/S0007114507730751 ![]() |
[14] |
Ramaneda J, Stallard-Olivera E, Hoffert M, et al. (2023) Building a genome-based understanding of bacterial pH preferences. Sci Adv 9: eadf8998. https://doi.org/10.1126/sciadv.adf8998 ![]() |
[15] |
Duncan SH, Louis P, Thomson JM, et al. (2009) The role of pH in determining the species composition of the human colonic microbiota. Environ Microbiol 11: 2112-2122. https://doi.org/10.1111/j.1462-2920.2009.01931.x ![]() |
[16] | Schwartz J, Schumaker K, Bremeyer S, et al. (2022) Bacterial battle against acidity. FEMS Microbiol Revs : 1-27. https://doi.org/10.1093/femsre/fuac037 |
[17] |
Trcek J, Mira NP, Jarboe LR (2015) Adaptation and tolerance of bacteria against acetic acid. Appl Microbiol Biotechnol 99: 6215-6229. https://doi.org/10.1007/s00253-015-6762-3 ![]() |
[18] |
Walker AW, Duncan SH, McWilliam Leitch E, et al. (2005) pH and peptide supply can radically alter bacterial populations and short chain fatty acid ratios within human colonic microbial communities. Appl Environ Microbiol 71: 3692-3700. https://doi.org/10.1128/AEM.71.7.3692-3700.2005 ![]() |
[19] |
Chung WCF, Walker AW, Louis P, et al. (2016) Modulation of the human gut microbiota by dietary fibres occurs at the species level. BMC Biol 14: 3. https://doi.org/10.1186/s12915-015-0224-3 ![]() |
[20] |
Wang SP, Rubio LA, Duncan SH, et al. (2020) Pivotal roles for pH, lactate, and lactate-utilizing bacteria in the stability of a human colonic microbial ecosystem. mSystems 5: e00645-20. https://doi.org/10.1128/mSystems.00645-20 ![]() |
[21] |
Duncan SH, Louis P, Flint HJ (2004) Lactate-utilising bacteria from human feces that produce butyrate as a major fermentation product. Appl Environ Microbiol 70: 5810-5817. https://doi.org/10.1128/AEM.70.10.5810-5817.2004 ![]() |
[22] |
Louis P, Duncan SH, Sheridan PO, et al. (2022) Microbial lactate utilisation and the stability of the gut microbiome. Gut Microbiome 3: e3. https://doi.org/10.1017/gmb.2022.3 ![]() |
[23] |
Belenguer A, Duncan SH, Calder G, et al. (2006) Two routes of metabolic cross-feeding between bifidobacteria and butyrate-producing anaerobes from the human gut. Appl Environ Microbiol 72: 3593-3599. https://doi.org/10.1128/AEM.72.5.3593-3599.2006 ![]() |
[24] |
Duncan SH, Zuur G, Lobley GE, et al. (2004) Contribution of acetate to butyrate formation by human faecal bacteria. Br J Nutr 91: 915-923. https://doi.org/10.1079/BJN20041150 ![]() |
[25] |
Kettle H, Louis P, Holtrop G, et al. (2015) Modelling the emergent dynamics and major metabolites of the human colonic microbiota. Environ Microbiol 17: 1615-1630. https://doi.org/10.1111/1462-2920.12599 ![]() |
[26] |
Reichardt N, Vollmer M, Holtrop G, et al. (2018) Specific substrate-driven changes in human faecal microbiota compostion contrasts with functional redundancy in short chain fatty acid production. ISME J 12: 610-622. https://doi.org/10.1038/ismej.2017.196 ![]() |
[27] |
Campbell A, Gdanetz K, Schmidt AW, et al. (2023) H2 generated by fermentation in the human gut microbiome influences metabolism and competitive fitness of gut butyrate producers. Microbiome 11: 133. https://doi.org/10.1186/s40168-023-01565-3 ![]() |
[28] | Abell GCJ, Conlon MA, McOrist AL (2009) Methanogenic archaea in adult human faecal samples are inversely related to butyrate concentrations. Microb Ecol Health Dis 18: 154-160. https://doi.org/10.1080/08910600601048969 |
[29] |
Soto-Martin EC, Warnke I, Farquharson FM, et al. (2020) Vitamin biosynthesis by human gut butyrate-producing bacteria and cross-feeding in synthetic microbial communities. mBio 11: e00886-20. https://doi.org/10.1128/mBio.00886-20 ![]() |
[30] |
Khan MT, Duncan SH, Stams AJM, et al. (2012) The gut anaerobe Faecalibacterium prausnitzii uses an extracellular electron shuttle to grow at oxic-anoxic interphases. ISME J 6: 1578-1585. https://doi.org/10.1038/ismej.2012.5 ![]() |
[31] |
Colgan SP, Wang RX, Hall CHT, et al. (2023) Revisiting the ‘starved gut’ hypothesis in inflammatory bowel disease. Immunometabolism 5: e0016. https://doi.org/10.1097/IN9.0000000000000016 ![]() |
[32] |
Kettle H, Louis P, Flint HJ (2022) Process-based modelling of microbial community dynamics in the human colon. J Royal Soc Interface 19: 20220489. https://doi.org/10.1098/rsif.2022.0489 ![]() |
[33] |
Gawenis LR, Bradford EM, Prasad V, et al. (2007) Colonic anion secretory defects and metabolic acidosis in mice lacking NBC1 Na+/HCO3− cotransporter. J Biol Chem 283: 9042-52. https://doi.org/10.1074/jbc.M607041200 ![]() |
[34] |
Roediger WEW (1982) Utilization of nutrients by isolated epithelial cells of the rat colon. Gastroenterology 83: 424-429. https://doi.org/10.1016/S0016-5085(82)80339-9 ![]() |
[35] |
Louis P, Hold GL, Flint HJ (2014) The gut microbiota, bacterial metabolites and colorectal cancer. Nat Rev Microbiol 12: 661-672. https://doi.org/10.1038/nrmicro3344 ![]() |
[36] |
Roediger WEW (1980) The colonic epithelium in ulcerative colitis: an energy deficiency disease?. Lancet 116: 712-715. https://doi.org/10.1016/S0140-6736(80)91934-0 ![]() |
[37] |
Manichanh C, Borruel N, Cassillas F, et al. (2012) The gut microbiota in IBD. Nat Rev Gastroenterol Hepatol 9: 599-608. https://doi.org/10.1038/nrgastro.2012.152 ![]() |
[38] |
Hove H, Nordgaard-Andersen I, Mortensen PB (1994) Fecal DL-lactate concentration in 100 gastrointestinal patients. Scand J Gastroenterol 29: 255-259. https://doi.org/10.3109/00365529409090473 ![]() |
[39] |
Dear KLE, Elia M, Hunter JO (2005) Do interventions which reduce colonic fermentation improve symptoms of irritable bowel syndrome?. Dig Dis Sci 50: 758-766. https://doi.org/10.1007/s10620-005-2570-4 ![]() |
1. | Dawit Adisu Tadese, James Mwangi, Lei Luo, Hao Zhang, Xiaoshan Huang, Brenda B. Michira, Shengwen Zhou, Peter Muiruri Kamau, Qiumin Lu, Ren Lai, The microbiome’s influence on obesity: mechanisms and therapeutic potential, 2024, 1674-7305, 10.1007/s11427-024-2759-3 |