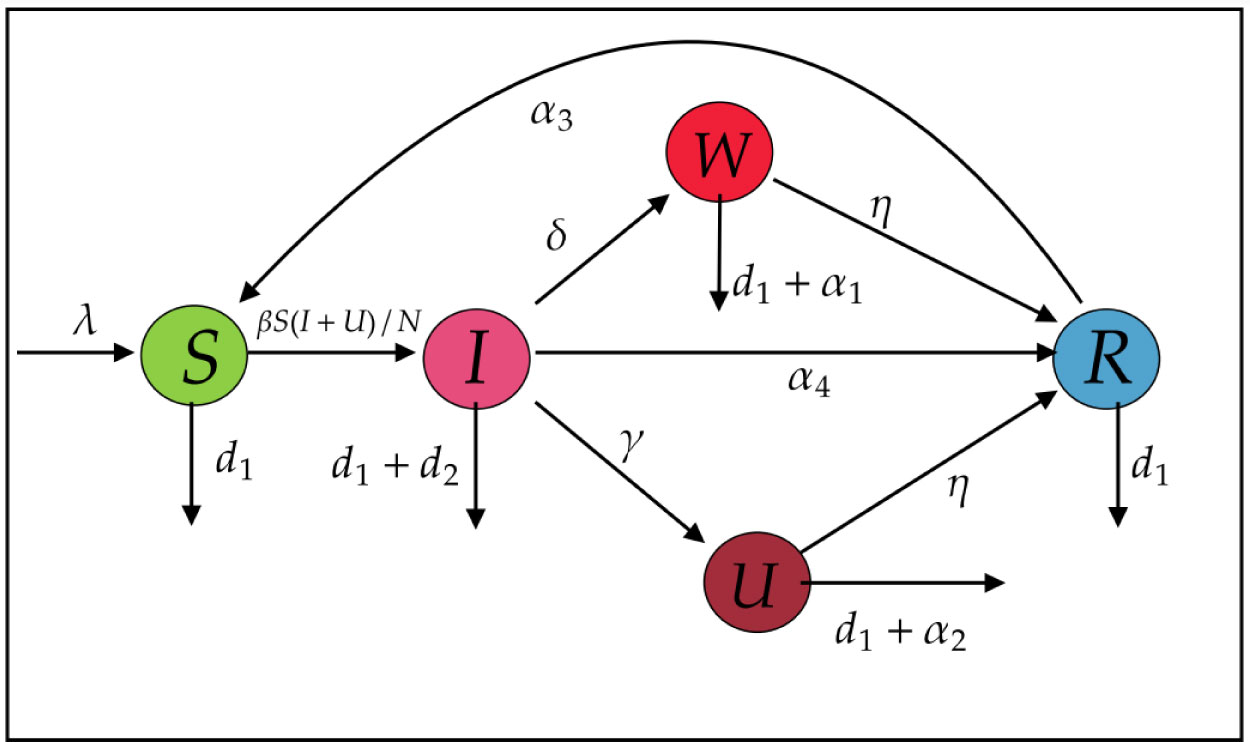
Central bank digital currency (CBDC) signals affect the volatility of stock indices in different sectors differently. This paper aims to examine whether the CBDC signal plays a role on the volatility of different stock indices. First, we employ a text analysis to compile the CBDC signal index, which spans from January 4, 2013 to March 16, 2023. Then, based on the mixing frequency data, we construct generalized autoregressive conditional heteroskedasticity mixed data sampling (GARCH-MIDAS) models to explore the various impacts of CBDC signal on the volatility of stock indices in different sectors. The findings show the heterogeneous effect of CBDC signals on the volatility of stock indices across different sectors. Furthermore, CBDC signals have a heterogeneous effect on the volatility of stock indices in different sectors for different lag periods.
Citation: Wenjie Li, Zimei Huang. Do different stock indices volatility respond differently to Central bank digital currency signals?[J]. Electronic Research Archive, 2023, 31(9): 5573-5588. doi: 10.3934/era.2023283
[1] | Sarbaz H. A. Khoshnaw, Azhi Sabir Mohammed . Computational simulations of the effects of social distancing interventions on the COVID-19 pandemic. AIMS Bioengineering, 2022, 9(3): 239-251. doi: 10.3934/bioeng.2022016 |
[2] | Mehmet Yavuz, Waled Yavız Ahmed Haydar . A new mathematical modelling and parameter estimation of COVID-19: a case study in Iraq. AIMS Bioengineering, 2022, 9(4): 420-446. doi: 10.3934/bioeng.2022030 |
[3] | Sarbaz H. A. Khoshnaw, Kawther Y. Abdulrahman, Arkan N. Mustafa . Identifying key critical model parameters in spreading of COVID-19 pandemic. AIMS Bioengineering, 2022, 9(2): 163-177. doi: 10.3934/bioeng.2022012 |
[4] | Mariem Jelassi, Kayode Oshinubi, Mustapha Rachdi, Jacques Demongeot . Epidemic dynamics on social interaction networks. AIMS Bioengineering, 2022, 9(4): 348-361. doi: 10.3934/bioeng.2022025 |
[5] | Kayode Oshinubi, Augustina Amakor, Olumuyiwa James Peter, Mustapha Rachdi, Jacques Demongeot . Approach to COVID-19 time series data using deep learning and spectral analysis methods. AIMS Bioengineering, 2022, 9(1): 1-21. doi: 10.3934/bioeng.2022001 |
[6] | Fırat Evirgen, Fatma Özköse, Mehmet Yavuz, Necati Özdemir . Real data-based optimal control strategies for assessing the impact of the Omicron variant on heart attacks. AIMS Bioengineering, 2023, 10(3): 218-239. doi: 10.3934/bioeng.2023015 |
[7] | Prasantha Bharathi Dhandapani, Dumitru Baleanu, Jayakumar Thippan, Vinoth Sivakumar . On stiff, fuzzy IRD-14 day average transmission model of COVID-19 pandemic disease. AIMS Bioengineering, 2020, 7(4): 208-223. doi: 10.3934/bioeng.2020018 |
[8] | Kranthi Kumar Lella, Alphonse PJA . A literature review on COVID-19 disease diagnosis from respiratory sound data. AIMS Bioengineering, 2021, 8(2): 140-153. doi: 10.3934/bioeng.2021013 |
[9] | Cong Toai Truong, Kim Hieu Huynh, Van Tu Duong, Huy Hung Nguyen, Le An Pham, Tan Tien Nguyen . Model-free volume and pressure cycled control of automatic bag valve mask ventilator. AIMS Bioengineering, 2021, 8(3): 192-207. doi: 10.3934/bioeng.2021017 |
[10] | Ayub Ahmed, Bashdar Salam, Mahmud Mohammad, Ali Akgül, Sarbaz H. A. Khoshnaw . Analysis coronavirus disease (COVID-19) model using numerical approaches and logistic model. AIMS Bioengineering, 2020, 7(3): 130-146. doi: 10.3934/bioeng.2020013 |
Central bank digital currency (CBDC) signals affect the volatility of stock indices in different sectors differently. This paper aims to examine whether the CBDC signal plays a role on the volatility of different stock indices. First, we employ a text analysis to compile the CBDC signal index, which spans from January 4, 2013 to March 16, 2023. Then, based on the mixing frequency data, we construct generalized autoregressive conditional heteroskedasticity mixed data sampling (GARCH-MIDAS) models to explore the various impacts of CBDC signal on the volatility of stock indices in different sectors. The findings show the heterogeneous effect of CBDC signals on the volatility of stock indices across different sectors. Furthermore, CBDC signals have a heterogeneous effect on the volatility of stock indices in different sectors for different lag periods.
The emergence of the pandemic COVID-19 in China in the late of 2019 became a critical issue these years globally. In 1930, for the first time the history of coronaviruses recorded when some domesticated chickens were infected by bronchitis virus (IBV) infection. After that in 1960 it was reported in humans for the first time. This disease had a great effect on worldwide economy and people's daily life. Due to the quick outbreak of the virus, specialists tried to refer to the drugs they have already used many years ago for treating SARS and common flu [1]. WHO announced COVID-19 epidemic on 11th March 2020. By January 20th, 2022, there were 340,120,476 cases and 5,586,044 of them were deaths and 273,396,004 of them recovered from the disease. It is clear that this disease has not been controlled globally yet and it needs more strategies and preventions to control it.
There were some recently published studies that discussed and analyzed the key critical elements about mathematical models and the spreading of COVID-19 pandemic. There was a study that describes a public data from COVID-19 epidemic in France. It estimates about the model dynamics states of COVID-19 epidemic in France and the regional level SEIR mechanistic model was used. The results showed that a lifting of the lockdown has a great role for further interventions and avoid the epidemic widely [2]. In different studies, a group of researchers developed a mathematical model for this disease, it was defined as a novel Θ-SEIHRD model. This model supports to deal with certain characteristics of this disease that studies the fraction Θ of detected cases for the real total infected cases. It also helps to estimate the required beds in hospitals. The results show that the estimation performed at an early stage of the epidemic could suggest powerful results. These results could be used as a guidance to countries were hugely affected by COVID-19 [3]. A few other researchers conducted some researches on the African continents on COVID-19 epidemic development. The SEIQRDP model was used to establish the realistic estimation of the short-term pandemic condition. The SEIQRDP was a modification of the classical SIR model extensively used in mathematical epidemiology. This model relies on quarantine to a great extent as a productive way of the spread of COVID-19 [4]. Another study sheds light on how social measures such as distance, regional lockdown, quarantine and global public health vigilance, influence the model parameters and the model states. Another critical parameter of COVID-19 is the basic reproduction number called R0. The global sensitivity analysis is another important technique for infectious diseases. This has been used to observe the impact of different parameters on R0 [5]. Recently, there was another study that describes local sensitivity analysis of COVID-19 disease. They identified the model critical parameters and considered the relation between model variables and parameters [6].
An effective key element to discuss this disease further and suggest more preventions is data fitting and confirmed cases. This relation between two sets becomes a great step forward to predict this disease further. Data fitting can be used for all infectious disease models, two mathematical models of epidemics have been used to deal with Ebola epidemic in Guinea, Liberia and Sierra Leone. The simulation studies of the model with variable values of sensitive parameters of the spread of the outbreak are undertaken and incorporated in the paper [7]. Recently, there was a compartmental logistic regression and Gaussian model to study and investigate the spread of COVID-19 in Iraq and Egypt by developing SEIR model. The logistic regression and Gaussian models were applied in both countries for the prediction of confirmed cases, and found a very good fitness for incidence data [8]. In addition, there was another study that focused on situation of Saudi Arabia Kingdom for COVID-19. The least square curve fitting was used for the evaluation of the parameters with R0 ≈ 1.2937 as the number of basic reproduction [9].
There are also many recently published papers on COVID-19 and their impacts in the society. A fractional order of COVID-19 model with Mittag-Leffler kernel was used to analyze this pandemics, the suggested techniques are given in [10]. The stochastic modelling approaches such as transition probabilities and parametric perturbations were applied to calculate some computational dynamics for the SIR model of COVID-19 [11]. The classical SEIR compartment model was used by contact tracing and hospitalization strategies to show the spreading of COVID-19 outbreak [12]. The reader can see more published studies about COVID-19 pandemic in [13]–[20].
Identifying the model critical parameters can be also considered by optimal control analysis that provides more control strategies. This technique has been widely used to COVID-19 pandemics for driving control policies and more interventions. Recently, there was a study that suggested a mathematical model with optimal control to highlight the negative impact of quarantine on diabetic people with Cost-effectiveness presented in [21]. Then, this method was used in analyzing the spread of COVID-19 pandemic with a case study of Brazil and cost-effectiveness, this is given in [22]. More recently, the idea of optimal control analysis was also applied to another suggested model of COVID-19 to show the impact of rapid-tests in controlling this pandemic [23].
Analyzing the suggested SIUWR model based on elasticity of basic reproduction number becomes an important step forward in this study compared to the other existing studies about the confirmed cases of COVID-19 pandemics in Iraq and France. Therefore, we suggest the elasticity coefficient approach to calculate the local sensitivities of basic reproduction number for each model parameters. Another novelty here is the data collection of COVID-19 infected cases for both countries, this provides the future predictions and interventions. Accordingly, we have collected the daily confirmed cases of COVID-19 from January 1st, 2021 to December 25th, 2021. The collected real data here give us a great understanding and suggested more interventions and strategies to control this pandemic. Comparing the model results with the real confirmed cases is another novelty for the suggested SIUWR model.
There are some significant contributions in this study. Firstly, we develop and update our previously published model of the COVID-19 pandemic given in [6]. We add more transmission rates to the suggested SIUWR model in order to understand this disease more widely and accurately. Secondly, we compare the model results with the confirmed cases in Iraq and France from January 1st, 2021 to December 25th, 2021. Another contribution here is identifying the model critical parameters based on sensitivity of basic reproduction number for each model transmission parameters that helps us to identify the key critical model parameters in spreading this disease.
The organization of this paper is as follows. In Section two, we developed our previously published model and construct the suggested SIUWR model based on our assumptions and model transmissions. Using the next generation matrix to drive the formula of basic reproduction number discussed in Section three. Calculating the model sensitivity based elasticity coefficients is given in Section four. The computational model simulations and the daily infected cases is compared in Section five. Finally, some conclusions and suggestions are given in Section six.
The idea of classical kinetic reactions provides an effective tool to represent the biological infectious disease mechanisms in terms of model differential equations with constant rates. There are three important model assumptions in order to define the model equations. They are called model states, parameters, and model equations. We start with n irreversible chemical mechanisms and m chemical species, they are given below:
where αij and βij are chemical constant coefficients, Aj for j = 1,2,...,m are chemical components. The chemical reaction rates for equation (1) can be defined as follows:
The model differential equations for the chemical reactions (1) are given as follows:
where
The functions
All model compartments and parameters are shown in Table 1. Using the mass action law, the model differential equations are defined below:
With initial model populations S(0) >0, I(0) > 0, U(0) > 0, W(0) > 0, R(0) ≥ 0.
Symbols | Biological definitions | Estimated values - Iraq | Estimated values - France | Sources |
S(0) | Initial population of susceptible people | 40584909 | 65063283 | [28] |
I(0) | Initial population of asymptomatic infected people | 789 | 17523 | [28] |
U(0) | Initial population of unreported symptomatic infected people | 395 | 9000 | Assumed |
W(0) | Initial population of reported symptomatic infected people | 394 | 8523 | Assumed |
R(0) | Initial population of recovered people | 581634 | 25000968 | [28] |
N | Total population | 41179351 | 67564251 | Estimated |
λ | Recruitment rate | 1482.5537 | 2241.554 | Assumed |
β | Constant rate between susceptible people and asymptomatic infected people | 0.552 | 0.558 | Assumed |
γ | Constant rate between asymptomatic infected people and reported symptomatic infected people | 0.2 | 0.2 | Assumed |
δ | Constant rate between asymptomatic infected people and unreported symptomatic infected people | 0.1 | 0.1 | Assumed |
η | Constant rate between reported-unreported symptomatic infected people and recovered people | 0.1428 | 0.1428 | Assumed |
d1 | Natural death rate | 0.001 | 0.001 | Assumed |
d2 | The asymptomatic infected death rate | 1.5 × 10−2 | 1.5 × 10−2 | Assumed |
α1 | The unreported symptomatic death rate | 1.5 × 10−4 | 1.5 × 10−4 | Assumed |
α2 | The reported symptomatic death rate | 1.7826 × 10−2 | 1.7826 × 10−2 | Assumed |
α3 | The immunity loss rate | 0.03 | 0.03 | Assumed |
α4 | Transition rate between asymptomatic infected and recovered individuals | 0.488 | 0.538 | Assumed |
The quantity of basic reproduction number, R0 has a great role in spreading of COVID-19. It defines as the number of secondary infections that infected by a single primary infection in the population. According to the recently published works of epidemiological models, R0 can provide a wide range of critical model parameters identification. The next-generation matrix is an effective method to derive the basic reproduction number for infectious disease models. This technique was discussed in more details in [29]–[32]. If the individuals are infected in a compartment, then the compartment is classified as an infected compartment. This compartment includes both asymptomatic and symptomatic individuals. Suppose that an infectious disease model has m compartments (x1, x2,...,xm). The model equations are given as follows:
These compartments can be split into n disease compartments (infected individuals) and k non-disease compartments (non-infected individuals) as follows:
Let Fi(x) be the rate of appearance of new infections in ith compartment and the transfer rate is given below:
where
Again, we denote the subpopulations by
Now, we define the Jacobian matrices F and V as follows:
where x0 is the free equilibrium point for Equation (6), F is the entry wise non-negative and V is a non-singular matrix. The basic reproduction ratio R0 is given by the spectral radius (dominant eigenvalue) of the next generation matrix FV−1 given below:
where ρ denotes the spectral radius. The reader can see more details regarding to the next generation matrix and the basic reproduction ratio with their applications in the infection disease models given in [33]–[38].
Taking the right-hand side of system (5) and equating it to zero, the disease-free equilibrium point is given below:
For system (10) and using the equilibrium point
Therefore, the next generation matrix is as follows:
Thus, FV−1 has one dominant eigenvalue. The equation of the basic reproduction number for system (5) with the free equilibrium point
The concept of elasticity for infectious disease models has an effective role in determining sensitivity between R0 and the model parameters. This technique was discussed in more details in [31],[36],[38]. For calculating such coefficients, the following essential formula is used,
where ω is the set of all parameters, we calculate the elasticity index of R0 for each model parameter. They are given below:
Using the estimated parameters, we can compute the model elasticity; see Table 2.
Elasticity coefficients | Iraq | France |
1 | 1 | |
1 | 1 | |
−0.24875621 | −0.23419203 | |
0.28554195 | 0.29282404 | |
−0.40664526 | −0.40664526 | |
−1.00409143 | −1.00401861 | |
−0.01865671 | −0.01756440 | |
−0.00042714 | −0.00042714 | |
0 | 0 | |
0 | 0 | |
−0.60696517 | −0.62997658 |
Results given in Table 2 show the positive and negative relation for the model elasticity. These give us the direct or indirect relation between R0 and the model parameters. For example, this coefficient is positive for the set of parameters {λ, β, δ}. This means that when the parameters {λ, β, δ} are increased, the value of R0 is increased too and this virus spreads further. On the other hand, the signs of {γ, η, d1, d2, α1, α4} are negative. It means that the value of basic reproduction number can be reduced by increasing such parameters. However, the reproduction number does not have relation with the parameters {α2, α3} that means increasing or decreasing the value of {α2, α3} do not make a significant effect on the value of R0.
Using computational tools are essential for analyzing complex infectious disease models. Here in this study, we have used MATLAB tools to compute the predicated values for the populations of the spreading COVID-19. We collected the real data of this disease in Iraq and France from 1st of January 2021 to 25th of December 2021. All the confirmed cases for both countries are given in the supplementary files (Tables 3 and 4). The estimated variables and parameters given in Table 1 for Iraq and France are used in computational simulations using MATLAB. Figures 3 and 6 show the model results using the initial states and parameters given in Table 1 for Iraq and France, respectively. The total populations of susceptible, symptomatic infected, unreported symptomatic infected, reported symptomatic infected and recovered individuals are computed. In addition, Figure 4(a) shows the total number of infected cases in Iraq using real data given in Table 3. Accordingly, the model results and confirmed infected cases are compared in Figure 4(b), it is clear that there is a good agreement between both model results and the real data. In this vein, Figure 5(a) shows the total infected people in France using the real data given in Table 4. On the other hand, Figure 5(b) shows both model results and real data of the number of infected individuals in France. It is seen that the model results have a good agreement with the real data. Therefore, the suggested SIWUR model can be further used for future predictions and estimations.
Numerical results here give more biological interpretations. As it is clear that computational simulations help international efforts for controlling this pandemic significantly. The dynamics of each compartment can be clearly shown, such model dynamics provide an important environment to predict the future spreading of this virus. For instance, the number of asymptomatic infected, reported symptomatic, unreported symptomatic individuals are simply shown in the computational results here. Accordingly, our model results show that the number of infected people in Iraq has been dramatically changed for the months of February to October. While, there was a big change in the number of confirmed cases in France between March and July. Interestingly, the number of infected people in both countries were significantly declined at the end of 2021.
Analyzing and studying the spreading of COVID-19 pandemic has become a global topic and many studies have been proposed. Comparing the confirmed cases with the model results provide a wide range of environment to predict and give suggestions for further preventions.
In this study, we developed and updated our previously published model of the COVID-19 pandemic and added more transmission rates in order to understand this disease more widely and accurately. In addition, we compared the model results and the confirmed cases in Iraq and France from January 1st, 2021 to December 25th, 2021. Then, the model critical parameters based on sensitivity of basic reproduction number for each model transmission parameters are identified. This helps us to identify the key critical model parameters in spreading this disease.
The suggested model here is more appropriated and fitted to the real confirmed cases in Iraq and France. Accordingly, the computational results show that there are good agreements between the model dynamics and daily cases in both countries. This is an effective forward step to study this model more widely and accurately. Identifying the critical model parameters based on sensitivity and elasticity techniques help us to understand such global issues theoretically and practically. Interestingly, the suggested model can be also used to further predications and give more control strategies.
[1] |
Y. Tan, Z. H. Li, S. M. Liu, M. I. Nazir, M. Haris, Competitions in different banking markets and shadow banking: Evidence from China, Int. J.. Emerg. Mark., 17 (2022), 1465–1483. https://doi.org/10.1108/ijoem-04-2020-0401 doi: 10.1108/ijoem-04-2020-0401
![]() |
[2] |
Z. Li, H. Chen, B. Mo, Can digital finance promote urban innovation? Evidence from China, Borsa Istanbul Rev., 23 (2023), 285–296. https://doi.org/10.1016/j.bir.2022.10.006 doi: 10.1016/j.bir.2022.10.006
![]() |
[3] |
Z. H. Huang, G. K. Liao, Z. H. Li, Loaning scale and government subsidy for promoting green innovation, Technol. Forecasting Soc. Change, 144 (2019), 148–156. https://doi.org/10.1016/j.techfore.2019.04.023 doi: 10.1016/j.techfore.2019.04.023
![]() |
[4] |
Z. H. Li, Z. M. Huang, Y. Y. Su, New media environment, environmental regulation and corporate green technology innovation: Evidence from China, Energ. Econ., 119 (2023), 106545. https://doi.org/10.1016/j.eneco.2023.106545 doi: 10.1016/j.eneco.2023.106545
![]() |
[5] |
T. Li, X. Li; G. Liao, Business cycles and energy intensity. Evidence from emerging economies, Borsa Istanbul Rev., 22 (2021), 560–570. https://doi.org/10.1016/j.bir.2021.07.005 doi: 10.1016/j.bir.2021.07.005
![]() |
[6] |
Z. H. Huang, H. Dong, S. S. Jia, Equilibrium pricing for carbon emission in response to the target of carbon emission peaking, Energ. Econ., 112 (2022), 106160. https://doi.org/10.1016/j.eneco.2022.106160 doi: 10.1016/j.eneco.2022.106160
![]() |
[7] |
J. Barrdear, M. Kumhof, The macroeconomics of central bank digital currencies, J. Econ. Dyn. Control, 142 (2022), 104148. https://doi.org/10.1016/j.jedc.2021.104148 doi: 10.1016/j.jedc.2021.104148
![]() |
[8] |
Z. H. Li, B. Mo, H. Nie, Time and frequency dynamic connectedness between cryptocurrencies and financial assets in China, Int. Rev. Econ. Financ., 86 (2023), 46–57. https://doi.org/10.1016/j.iref.2023.01.015 doi: 10.1016/j.iref.2023.01.015
![]() |
[9] |
Z. H. Li, H. Dong, C. Floros, A. Charemis, P. Failler, Re-examining Bitcoin Volatility: A CAViaR-based Approach, Emerg. Mark. Financ. Trade, 19 (2021), 1320–1338. https://doi.org/10.1080/1540496x.2021.1873127 doi: 10.1080/1540496x.2021.1873127
![]() |
[10] |
E. Y. Oh, S. Zhang, Informal economy and central bank digital currency, Econ. Inq., 60 (2022), 1520–1539. https://doi.org/10.1111/ecin.13105 doi: 10.1111/ecin.13105
![]() |
[11] | Y. S. Kim, O. Kwon, Central bank digital currency, credit supply, and financial stability, 55 (2023), 297–321. https://doi.org/https://doi.org/10.1111/jmcb.12913 |
[12] |
D. Andolfatto, Assessing the impact of central bank digital currency on private banks, Econ. J., 131 (2021), 525–540. https://doi.org/10.1093/ej/ueaa073 doi: 10.1093/ej/ueaa073
![]() |
[13] |
B. Xin, K. Jiang, Central bank digital currency and the effectiveness of negative interest rate policy: A DSGE analysis, Res. Int. Bus. Financ., 634 (2023), 525–540. https://doi.org/10.1016/j.ribaf.2023.101901 doi: 10.1016/j.ribaf.2023.101901
![]() |
[14] |
W. Shen, L. Hou, China's central bank digital currency and its impacts on monetary policy and payment competition: Game changer or regulatory toolkit?, Comput. law Secur. Rev., 41 (2021), 105577. https://doi.org/10.1016/j.clsr.2021.105577 doi: 10.1016/j.clsr.2021.105577
![]() |
[15] |
Y. Wang, B. M. Lucey, S. A. Vigne, L. Yarovaya, The effects of central bank digital currencies news on financial markets, Tech. Forecast. Soc. Change, 180 (2022), 121715. https://doi.org/10.1016/j.techfore.2022.121715 doi: 10.1016/j.techfore.2022.121715
![]() |
[16] |
Z. H. Li, C. Y. Yang, Z. H. Huang, How does the fintech sector react to signals from central bank digital currencies?, Financ. Res. Lett., 50 (2022), 103308. https://doi.org/10.1016/j.frl.2022.103308 doi: 10.1016/j.frl.2022.103308
![]() |
[17] |
Z. H. Li, L. M. Chen, H. Dong, What are bitcoin market reactions to its-related events?, Int. Rev. Econ. Financ., 73 (2021), 1–10. https://doi.org/10.1016/j.iref.2020.12.020 doi: 10.1016/j.iref.2020.12.020
![]() |
[18] |
A. K. Bharti, Asymmetrical herding in cryptocurrency: Impact of COVID 19, Quant. Financ. Econ., 6 (2022), 326–341. https://doi.org/10.3934/qfe.2022014 doi: 10.3934/qfe.2022014
![]() |
[19] |
S. L. Chen, S. M. Liu, R. J. Cai, Y. Y. Zhang, The factors that influence exchange-rate risk: Evidence in China, Emerg. Mark. Financ. Trade, 56 (2020), 1275–1292. https://doi.org/10.1080/1540496x.2019.1636229 doi: 10.1080/1540496x.2019.1636229
![]() |
[20] |
Z. H. Li, Z. H. Huang, H. Dong, The influential factors on outward foreign direct investment: Evidence from the "The Belt and Road", Emerg. Mark. Financ. Trade, 55 (2019), 3211–3226. https://doi.org/10.1080/1540496x.2019.1569512 doi: 10.1080/1540496x.2019.1569512
![]() |
[21] |
S. A. Gyamerah, B. E. Owusu, E. K. Akwaa-Sekyi, Modelling the mean and volatility spillover between green bond market and renewable energy stock market, Green Financ., 4 (2022), 310–328. https://doi.org/10.3934/gf.2022015 doi: 10.3934/gf.2022015
![]() |
[22] |
S. Scharnowski, Central bank speeches and digital currency competition, Financ. Res. Lett., 49 (2022), 103072. https://doi.org/10.1016/j.frl.2022.103072 doi: 10.1016/j.frl.2022.103072
![]() |
[23] |
P. K. Ozili, Central bank digital currency and bank earnings management using loan loss provisions, Digit. Policy Regul. Governance J., 25 (2023), 206–220. https://doi.org/10.1108/DPRG-11-2022-0139 doi: 10.1108/DPRG-11-2022-0139
![]() |
[24] |
S. Rahman, I. H. Moral, M. Hassan, G. S. Hossain, R. Perveen, Review a systematic review of green finance in the banking industry: perspectives from a developing country, Green Financ., 4 (2022), 347–363. https://doi.org/10.3934/gf.2022017 doi: 10.3934/gf.2022017
![]() |
[25] |
C. C. Lee, C. W. Wang, H. Y. Hsieh, W. L. Chen, The impact of central bank digital currency variation on firm's implied volatility, Res. Int. Bus. Financ., 64 (2023), 101878. https://doi.org/10.1016/j.ribaf.2023.101878 doi: 10.1016/j.ribaf.2023.101878
![]() |
[26] |
W. H. You, Y. W. Guo, H. M. Zhu, Y. Tang, Oil price shocks, economic policy uncertainty and industry stock returns in China: Asymmetric effects with quantile regression, Energ. Econ., 68 (2017), 1–18. https://doi.org/10.1016/j.eneco.2017.09.007 doi: 10.1016/j.eneco.2017.09.007
![]() |
[27] |
L. Pastor, P. Veronesi, Uncertainty about government policy and stock prices, J. Financ., 67 (2012), 1219–1264. https://doi.org/10.1111/j.1540-6261.2012.01746.x doi: 10.1111/j.1540-6261.2012.01746.x
![]() |
[28] |
S. Chen, J. Zhong, P. Failler, Does China transmit financial cycle spillover effects to the G7 countries?, Econ. Res.-Ekon. Istraž., 35 (2022), 5184–5201. https://doi.org/10.1080/1331677x.2021.2025123 doi: 10.1080/1331677x.2021.2025123
![]() |
[29] |
T. H. Li, J. H. Zhong, Z. M. Huang, Potential dependence of financial cycles between emerging and developed countries: Based on ARIMA-GARCH copula model, Emerg. Mark. Financ. Trade, 56 (2020), 1237–1250. https://doi.org/10.1080/1540496x.2019.1611559 doi: 10.1080/1540496x.2019.1611559
![]() |
[30] |
S. Deniz, Volatility spillovers among MIST stock markets, Data Sci. Financ. Econ., 2 (2022), 80–95. https://doi.org/10.3934/DSFE.2022004 doi: 10.3934/DSFE.2022004
![]() |
[31] |
P. Maria, M. Annalisa, T. Giacomo, Z. Lea, The informative value of central banks talks: A topic model application to sentiment analysis, Data Sci. Financ. Econ., 2 (2022), 181–204. https://doi.org/10.3934/DSFE.2022009 doi: 10.3934/DSFE.2022009
![]() |
[32] |
J. Park, R. A. Ratti, Oil price shocks and stock markets in the US and 13 European countries, Energy Econ., 30 (2008), 2587–2608. https://doi.org/10.1016/j.eneco.2008.04.003 doi: 10.1016/j.eneco.2008.04.003
![]() |
[33] |
G. K. Liao, P. Hou, X. Y. Shen, K. Albitar, The impact of economic policy uncertainty on stock returns: The role of corporate environmental responsibility engagement, Int. J. Financ. Econ., 26 (2021), 4386–4392. https://doi.org/10.1002/ijfe.2020 doi: 10.1002/ijfe.2020
![]() |
[34] |
Z. H. Li, J. H. Zhong, Impact of economic policy uncertainty shocks on China's financial conditions, Financ. Res. Lett., 35 (2020), 101303. https://doi.org/10.1016/j.frl.2019.101303 doi: 10.1016/j.frl.2019.101303
![]() |
[35] |
Y. Jiang, G. Tian, Y. Wu, B. Mo, Impacts of geopolitical risks and economic policy uncertainty on Chinese tourism‐listed company stock, Int. J. Financ. Econ., 27 (2022), 320–333. https://doi.org/10.1002/ijfe.2155 doi: 10.1002/ijfe.2155
![]() |
[36] |
G. P. Shi, X. X. Liu, Stock price fluctuation and the business cycle in the BRICS countries: A nonparametric quantiles causality approach, Financ. Res. Lett., 33 (2020), 101223. https://doi.org/10.1016/j.frl.2019.06.021 doi: 10.1016/j.frl.2019.06.021
![]() |
[37] |
M. Arouri, C. Estay, C. Rault, D. Roubaud, Economic policy uncertainty and stock markets: Long-run evidence from the US, Financ. Res. Lett., 18 (2016), 136–141. https://doi.org/10.1016/j.frl.2016.04.011 doi: 10.1016/j.frl.2016.04.011
![]() |
[38] |
S. Chen, Y. Wang, K. Albitar, Z. Huang, Does ownership concentration affect corporate environmental responsibility engagement? The mediating role of corporate leverage, Borsa Istanbul Rev., 21 (2021), S13–S24. https://doi.org/10.1016/j.bir.2021.02.001 doi: 10.1016/j.bir.2021.02.001
![]() |
[39] |
Y. Liu, Z. H. Li, M. R. Xu, The influential factors of financial cycle spillover: Evidence from China, Emerg. Mark. Financ. Trade, 56 (2020), 1336–1350. https://doi.org/10.1080/1540496x.2019.1658076 doi: 10.1080/1540496x.2019.1658076
![]() |
[40] |
Z. Li, G. Liao, K. Albitar, Does corporate environmental responsibility engagement affect firm value? The mediating role of corporate innovation, Bus. Strategy Environ., 29 (2019), 1045–1055. https://doi.org/10.1002/bse.2416 doi: 10.1002/bse.2416
![]() |
[41] |
Y. H. Jiang, G. Y. Tian, B. Mo, Spillover and quantile linkage between oil price shocks and stock returns: new evidence from G7 countries, Financ. Innov., 6 (2020). https://doi.org/10.1186/s40854-020-00208-y doi: 10.1186/s40854-020-00208-y
![]() |
[42] |
J. K. Sra, A. L. Booth, R. A. K. Cox, Voluntary carbon information disclosures, corporate-level environmental sustainability efforts, and market value, Green Financ., 4 (2022), 179–206. https://doi.org/10.3934/gf.2022009 doi: 10.3934/gf.2022009
![]() |
[43] |
C. K. M. Lau, E. Demir, M. H. Bilgin, Experience-based corporate corruption and stock market volatility: Evidence from emerging markets, Emerg. Mark. Rev., 17 (2013), 1–13. https://doi.org/10.1016/j.ememar.2013.07.002 doi: 10.1016/j.ememar.2013.07.002
![]() |
[44] |
P. C. Tetlock, Giving content to investor sentiment: The role of media in the stock market, J. Financ., 62 (2007), 1139–1168. https://doi.org/10.1111/j.1540-6261.2007.01232.x doi: 10.1111/j.1540-6261.2007.01232.x
![]() |
[45] |
D. Zhang, J. Engelberg, P. J. Gao, The sum of All FEARS investor sentiment and asset prices, Rev. Financ. Stud., 28 (2015), 1–32. https://doi.org/10.1093/rfs/hhu072 doi: 10.1093/rfs/hhu072
![]() |
[46] |
G. Kaplanski, H. Levy, Sentiment and stock prices: The case of aviation disasters, J. Financ. Econ., 95 (2010), 174–201. https://doi.org/10.1016/j.jfineco.2009.10.002 doi: 10.1016/j.jfineco.2009.10.002
![]() |
[47] |
S. K. Agyei, A. Bossman, Investor sentiment and the interdependence structure of GⅡPS stock market returns: A multiscale approach, Quant. Financ. Econ., 7 (2023), 87–116. https://doi.org/10.3934/qfe.2023005 doi: 10.3934/qfe.2023005
![]() |
[48] |
Y. Chen, Z. Huang, Measuring the effects of investor attention on China's stock returns, Data Sci. Financ. Econ., 1 (2021), 327–344. https://doi.org/10.3934/DSFE.2021018 doi: 10.3934/DSFE.2021018
![]() |
[49] |
Z. Li, Z. Huang, P. Failler, Dynamic correlation between crude oil price and investor sentiment in China: Heterogeneous and asymmetric effect. Energies, 15 (2022), 687. https://doi.org/10.3390/en15030687 doi: 10.3390/en15030687
![]() |
[50] |
S. L. Chung, C. H. Hung, C. Y. Yeh, When does investor sentiment predict stock returns?, J. Empirical Financ., 19 (2012), 217–240. https://doi.org/10.1016/j.jempfin.2012.01.002 doi: 10.1016/j.jempfin.2012.01.002
![]() |
[51] |
R. F. Engle, J. G. Rangel, The Spline-GARCH model for low-frequency volatility and its global macroeconomic causes, Rev. Financ. Stud., 21 (2008), 1187–1222. https://doi.org/10.1093/rfs/hhn004 doi: 10.1093/rfs/hhn004
![]() |
[52] |
R. F. Engle, E. Ghysels, B. Sohn, Stock market volatility and macroeconomic fundamentals, Rev. Econ. Stat., 95 (2013), 776–797. https://doi.org/10.1162/REST_a_00300 doi: 10.1162/REST_a_00300
![]() |
[53] |
Z. Li, F. Zou, B. Mo, Does mandatory CSR disclosure affect enterprise total factor productivity?, Econ. Res.-Ekon. Istraž., 35 (2021), 1–20. https://doi.org/10.1080/1331677x.2021.2019596 doi: 10.1080/1331677x.2021.2019596
![]() |
[54] |
Y. Zheng, Z. Wang, Z. Huang, T. Jiang, Comovement between the Chinese business cycle and financial volatility: Based on a DCC-MIDAS model, Emerg. Mark. Financ. Trade, 56 (2020), 1181–1195. https://doi.org/10.1080/1540496x.2019.1620100 doi: 10.1080/1540496x.2019.1620100
![]() |
[55] |
S. Charfi, F. Mselmi, Modeling exchange rate volatility: application of GARCH models with a Normal Tempered Stable distribution, Quant. Financ. Econ., 6 (2022), 206–222. https://doi.org/10.3934/qfe.2022009 doi: 10.3934/qfe.2022009
![]() |
1. | Alaa Falih Mahdi, Hussein K. Asker, A Sensitivity Analysis of the PSITPS Epidemic Model’s Parameters for COVID-19, 2024, 11, 2518-0010, 79, 10.31642/JoKMC/2018/110210 |
Symbols | Biological definitions | Estimated values - Iraq | Estimated values - France | Sources |
S(0) | Initial population of susceptible people | 40584909 | 65063283 | [28] |
I(0) | Initial population of asymptomatic infected people | 789 | 17523 | [28] |
U(0) | Initial population of unreported symptomatic infected people | 395 | 9000 | Assumed |
W(0) | Initial population of reported symptomatic infected people | 394 | 8523 | Assumed |
R(0) | Initial population of recovered people | 581634 | 25000968 | [28] |
N | Total population | 41179351 | 67564251 | Estimated |
λ | Recruitment rate | 1482.5537 | 2241.554 | Assumed |
β | Constant rate between susceptible people and asymptomatic infected people | 0.552 | 0.558 | Assumed |
γ | Constant rate between asymptomatic infected people and reported symptomatic infected people | 0.2 | 0.2 | Assumed |
δ | Constant rate between asymptomatic infected people and unreported symptomatic infected people | 0.1 | 0.1 | Assumed |
η | Constant rate between reported-unreported symptomatic infected people and recovered people | 0.1428 | 0.1428 | Assumed |
d1 | Natural death rate | 0.001 | 0.001 | Assumed |
d2 | The asymptomatic infected death rate | 1.5 × 10−2 | 1.5 × 10−2 | Assumed |
α1 | The unreported symptomatic death rate | 1.5 × 10−4 | 1.5 × 10−4 | Assumed |
α2 | The reported symptomatic death rate | 1.7826 × 10−2 | 1.7826 × 10−2 | Assumed |
α3 | The immunity loss rate | 0.03 | 0.03 | Assumed |
α4 | Transition rate between asymptomatic infected and recovered individuals | 0.488 | 0.538 | Assumed |
Elasticity coefficients | Iraq | France |
1 | 1 | |
1 | 1 | |
−0.24875621 | −0.23419203 | |
0.28554195 | 0.29282404 | |
−0.40664526 | −0.40664526 | |
−1.00409143 | −1.00401861 | |
−0.01865671 | −0.01756440 | |
−0.00042714 | −0.00042714 | |
0 | 0 | |
0 | 0 | |
−0.60696517 | −0.62997658 |
Symbols | Biological definitions | Estimated values - Iraq | Estimated values - France | Sources |
S(0) | Initial population of susceptible people | 40584909 | 65063283 | [28] |
I(0) | Initial population of asymptomatic infected people | 789 | 17523 | [28] |
U(0) | Initial population of unreported symptomatic infected people | 395 | 9000 | Assumed |
W(0) | Initial population of reported symptomatic infected people | 394 | 8523 | Assumed |
R(0) | Initial population of recovered people | 581634 | 25000968 | [28] |
N | Total population | 41179351 | 67564251 | Estimated |
λ | Recruitment rate | 1482.5537 | 2241.554 | Assumed |
β | Constant rate between susceptible people and asymptomatic infected people | 0.552 | 0.558 | Assumed |
γ | Constant rate between asymptomatic infected people and reported symptomatic infected people | 0.2 | 0.2 | Assumed |
δ | Constant rate between asymptomatic infected people and unreported symptomatic infected people | 0.1 | 0.1 | Assumed |
η | Constant rate between reported-unreported symptomatic infected people and recovered people | 0.1428 | 0.1428 | Assumed |
d1 | Natural death rate | 0.001 | 0.001 | Assumed |
d2 | The asymptomatic infected death rate | 1.5 × 10−2 | 1.5 × 10−2 | Assumed |
α1 | The unreported symptomatic death rate | 1.5 × 10−4 | 1.5 × 10−4 | Assumed |
α2 | The reported symptomatic death rate | 1.7826 × 10−2 | 1.7826 × 10−2 | Assumed |
α3 | The immunity loss rate | 0.03 | 0.03 | Assumed |
α4 | Transition rate between asymptomatic infected and recovered individuals | 0.488 | 0.538 | Assumed |
Elasticity coefficients | Iraq | France |
1 | 1 | |
1 | 1 | |
−0.24875621 | −0.23419203 | |
0.28554195 | 0.29282404 | |
−0.40664526 | −0.40664526 | |
−1.00409143 | −1.00401861 | |
−0.01865671 | −0.01756440 | |
−0.00042714 | −0.00042714 | |
0 | 0 | |
0 | 0 | |
−0.60696517 | −0.62997658 |