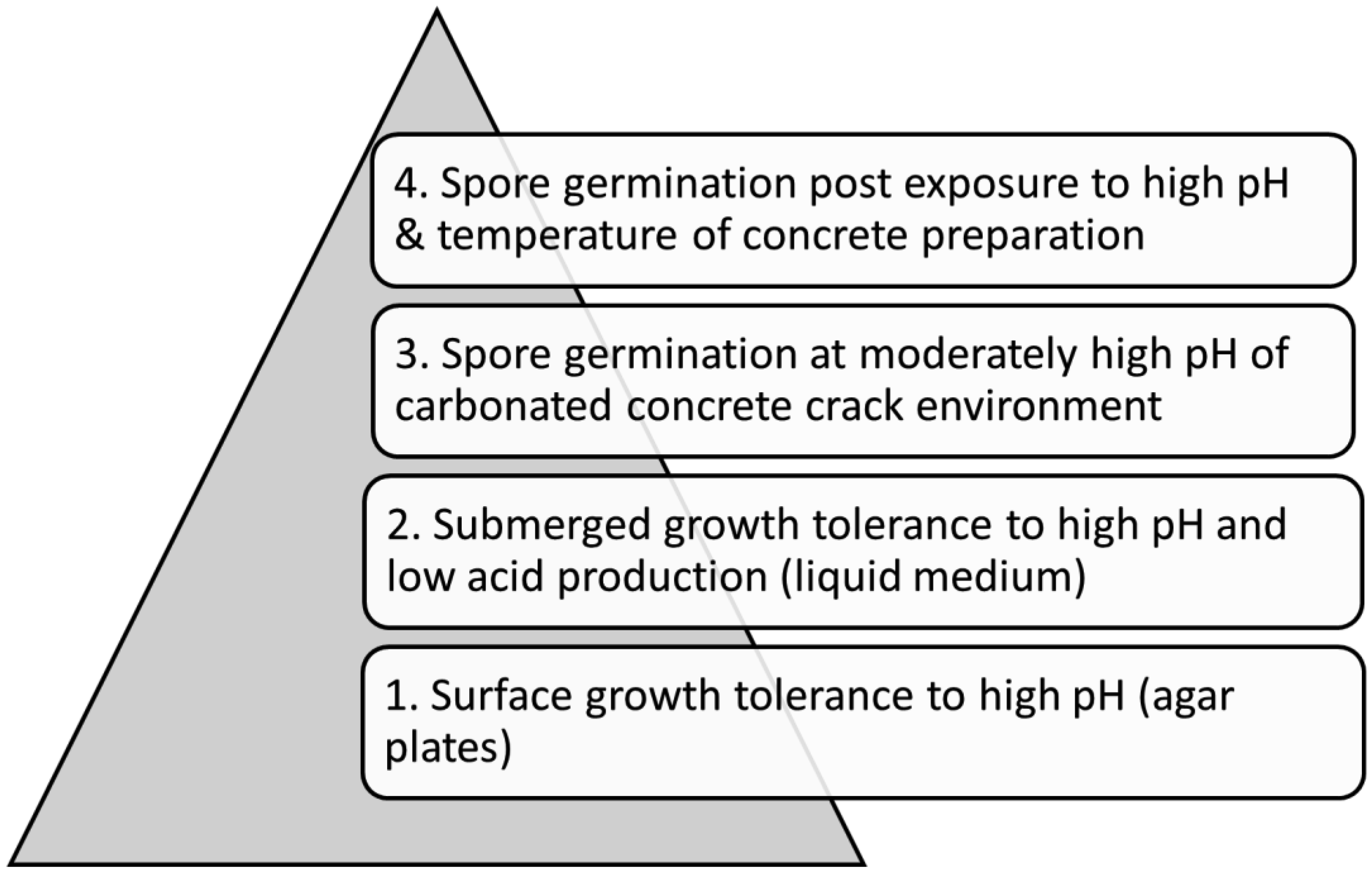
This work addresses the problem of having no existent protocol that closely simulates the harsh concrete environment, namely, high pH (about 13) and temperature (up to above 50 °C during cement hydration), for selecting microorganisms to use in microbe-effected self-healing concrete (MESHC). This problem critically impedes the progress of MESHC development, which has tremendous significance because concrete cracking reduces service life while making new concrete consumes substantial energy and emits a large amount of CO2. The objective of this work was to develop an effective and innovative screening protocol that mimics not only the concrete-relevant pH and temperature but also the essential process/scenario of MESHC, that is, microbial spores remain dormant through the harsh conditions during concrete mixing and curing and germinate later at the less harsh condition (pH 9–11, ambient temperature) in concrete cracks. The protocol includes 4 steps with increasing complexity to reduce strains for time-consuming steps. The first 2 steps screen for strains capable of vegetative growth on agar and liquid media with initial pH ≥ 10. Step 3 evaluates the spore germinability in medium with pH gradually decreasing from 12. In Step 4, spores were subjected to both high pH (12, 12.9) and temperature (45, 55 °C) for 2 h before being stored in the high-pH medium at room temperature for up to 21 days. Spores were finally collected and resuspended in pH 9.5 medium to observe germinability. 18 alkalotolerant strains were screened. Scopulariopsis brevicaulis (S. brevicaulis) is identified as the best candidate. Results also indicate the importance of incorporating designs to locally lower pH near spores, at least slightly (from 12.9 to 12), and to limit temperature to below 53 °C. Survivability of S. brevicaulis spores in contact with mortar undergoing cement hydration was also monitored. The work identifies promising fungi and indicates critical directions for developing spore-protection technologies for MESHC.
Citation: Ahsanul Kabir Sumon, Krutika Invally, Lu-Kwang Ju. Screening fungi for use as biocatalysts in self-healing concrete with protocol simulating concrete environment[J]. AIMS Bioengineering, 2024, 11(3): 323-342. doi: 10.3934/bioeng.2024017
[1] | Arindam Mitra, Suman Mukhopadhyay . Biofilm mediated decontamination of pollutants from the environment. AIMS Bioengineering, 2016, 3(1): 44-59. doi: 10.3934/bioeng.2016.1.44 |
[2] | Flávia da Silva Fernandes, Luan Reis Honorato da Silva, Érica Simplício de Souza, Lívia Melo Carneiro, João Paulo Alves Silva, Steven Zelski, João Vicente Braga de Souza, Jacqueline da Silva Batista . Isolation, genetic identification of Amazonian yeasts and analysis of thermotolerance and alcohol tolerance of Saccharomyces cerevisiae from Theobroma grandiflorum and Eugenia stipitata. AIMS Bioengineering, 2024, 11(1): 24-43. doi: 10.3934/bioeng.2024003 |
[3] | Aline Lima de Aguiar, Ana Luiza Figueira da Silva, Rayane Bonfim Ferreira Xavier, Marcos Diones Ferreira Santana, Dávia Marciana Talgatti, Fernando Abreu Oliveira, Carlos Ivan Aguillar-Vildoso, Érica Simplício de Souza, Márcio Barreto Rodrigues, João Paulo Alves Silva, Lívia Melo Carneiro, Clarice Maia Carvalho, João Vicente Braga de Souza, Eveleise Samira Martins Canto . Exploring the potential of fungal proteases in aquatic environments of the Tapajós Basin, Brazil. AIMS Bioengineering, 2025, 12(1): 162-176. doi: 10.3934/bioeng.2025007 |
[4] | Liwei Chen, Jaslyn Lee, Wei Ning Chen . The use of metabolic engineering to produce fatty acid-derived biofuel and chemicals in Saccharomyces cerevisiae: a review. AIMS Bioengineering, 2016, 3(4): 468-492. doi: 10.3934/bioeng.2016.4.468 |
[5] | José Luis Calvo-Guirado, Marta Belén Cabo-Pastor, Francisco Martínez-Martínez, Miguel Ángel Garcés-Villalá, Félix de Carlos-Villafranca, Nuria García-Carrillo, Manuel Fernández-Domínguez . Histologic and histomorphometric evaluation of minicono abutment on implant surrounding tissue healing and bone resorption on implants placed in healed bone. An experimental study in dogs. AIMS Bioengineering, 2023, 10(3): 183-201. doi: 10.3934/bioeng.2023013 |
[6] | Fabrizio Belleggia . Hard and soft tissue augmentation of vertical ridge defects with the “hard top double membrane technique”: introduction of a new technique and a case report. AIMS Bioengineering, 2022, 9(1): 26-43. doi: 10.3934/bioeng.2022003 |
[7] | Satarupa Dey, Amal Kanti Paul . Assessment of heavy metal tolerance and hexavalent chromium reducing potential of Corynebacterium paurometabolum SKPD 1204 isolated from chromite mine seepage. AIMS Bioengineering, 2016, 3(3): 337-351. doi: 10.3934/bioeng.2016.3.337 |
[8] | Olga M. Tsivileva, Inna M. Uchaeva, Nikolay A. Yurasov . Biotesting of technologically important carboxy containing acridones with solid-state fungal culture. AIMS Bioengineering, 2021, 8(1): 1-13. doi: 10.3934/bioeng.2021001 |
[9] | Kathryn A. Whitehead, Christopher M. Liauw, Joels S. T. Wilson-Nieuwenhuis, Anthony J. Slate, Ted Deisenroth, Andrea Preuss, Joanna Verran . The effect of the surface properties of poly(methyl methacrylate) on the attachment, adhesion and retention of fungal conidia. AIMS Bioengineering, 2020, 7(3): 165-178. doi: 10.3934/bioeng.2020015 |
[10] | Bennet Brockhagen, Fabian Schoden, Jan Lukas Storck, Timo Grothe, Christian Eßelmann, Robin Böttjer, Anke Rattenholl, Frank Gudermann . Investigating minimal requirements for plants on textile substrates in low-cost hydroponic systems. AIMS Bioengineering, 2021, 8(2): 173-191. doi: 10.3934/bioeng.2021016 |
This work addresses the problem of having no existent protocol that closely simulates the harsh concrete environment, namely, high pH (about 13) and temperature (up to above 50 °C during cement hydration), for selecting microorganisms to use in microbe-effected self-healing concrete (MESHC). This problem critically impedes the progress of MESHC development, which has tremendous significance because concrete cracking reduces service life while making new concrete consumes substantial energy and emits a large amount of CO2. The objective of this work was to develop an effective and innovative screening protocol that mimics not only the concrete-relevant pH and temperature but also the essential process/scenario of MESHC, that is, microbial spores remain dormant through the harsh conditions during concrete mixing and curing and germinate later at the less harsh condition (pH 9–11, ambient temperature) in concrete cracks. The protocol includes 4 steps with increasing complexity to reduce strains for time-consuming steps. The first 2 steps screen for strains capable of vegetative growth on agar and liquid media with initial pH ≥ 10. Step 3 evaluates the spore germinability in medium with pH gradually decreasing from 12. In Step 4, spores were subjected to both high pH (12, 12.9) and temperature (45, 55 °C) for 2 h before being stored in the high-pH medium at room temperature for up to 21 days. Spores were finally collected and resuspended in pH 9.5 medium to observe germinability. 18 alkalotolerant strains were screened. Scopulariopsis brevicaulis (S. brevicaulis) is identified as the best candidate. Results also indicate the importance of incorporating designs to locally lower pH near spores, at least slightly (from 12.9 to 12), and to limit temperature to below 53 °C. Survivability of S. brevicaulis spores in contact with mortar undergoing cement hydration was also monitored. The work identifies promising fungi and indicates critical directions for developing spore-protection technologies for MESHC.
Concrete is the second most widely used material after water. It is made of cement, water, sand, aggregates, and other chemicals [1]. Concrete is strong but liable to cracking, which affects concrete structural integrity and shortens its service life [2]. Because detecting and repairing cracks require laborious and costly maintenance logistics [3], the development of self-healing concrete, including one that is based on embedded microbial agents, has attracted much attention [4]. Complex reactions occur in concrete preparation, including the reaction of calcium silicates with water to form calcium silicate hydrate (C–S–H) and Ca(OH)2 as main hydration products [5]. The Ca(OH)2 crystal formed contributes significantly to the high compressive strength of concrete. Ca(OH)2 also makes concrete a highly basic material with a pH of about 13 in the aqueous phase present for mixing and curing (generally for 21–28 days) [6]. Further, the exothermic hydration reaction can raise the adiabatic temperature to above 50 °C [7], although in applications the heat loss from surface water vaporization makes the surface temperature generally lower than 35–40 °C [8] and the higher core temperature a transient early-stage phenomenon lasting < 2 h except for massive structures [9],[10]. After complete curing, concrete can be very dry with only 2–5% moisture content [11]. On concrete surface (and inside the cracks), Ca(OH)2 undergoes carbonation with the CO2 in air to form CaCO3 [12], giving a carbonated pH of about 9 [13].
Accordingly, microbes used for self-healing concrete can encounter seriously damaging factors, particularly the very high pH and certain elevated temperature. For example, Van Wylick et al. showed that the growth and CaCO3 precipitation by fungus Trichoderma reesei was clearly inhibited in liquid cultures incubated in the presence of a cement paste piece [14]. Compared to vegetative cells, fungal spores and bacterial endospores can withstand harsher environments. But even the spores were found to have very low survivability in concrete. While survivability tests for fungal spores in concrete have not been reported, all bacterial spores added in concrete were found to die in 2 months [15] and 80% died in 22 days [16]. Encapsulation or immobilization to protect spores was therefore suggested and studied [16]–[20]. Even with the use of protection materials, it is essential to select microorganisms that are more tolerant to the higher pH and temperature conditions in concrete, to improve their chance of survival and make the protection design simpler, less expensive, and more effective.
Some screening studies of fungi and bacteria for potential use in self-healing concrete have been reported. In a screening study of bacteria, Zhang et al. focused on the calcium precipitation ability, not on the bacteria's suitability for concrete environment [21]. To select fungi for self-healing concrete, Martuscelli et al. collected 19 fungal strains from 6 existing concrete structures and screened them based on their urease enzyme producing ability [22]. In another work, Luo et al. inoculated 6 selected fungal strains on nutrient-added agar laid on wet concrete and found one strain to grow; the strain was then determined to be able to produce calcite [23]. The authors also worked with pH regulatory mutants of Aspergillus nidulans [24]. Zhao et al. isolated fungal strains from colonies on concrete and screened them for the following abilities: urea hydrolysis, formation of CaCO3 precipitate and/or CaCO3 and SrCO3 coprecipitate, and growth at relatively high pH (pH 8.6 and 10.6) [25] although the concrete pH is much higher (pH 13).
In these reported studies, the mycelial growth at high pH was screened only with semisolid agar media, not in liquid media. On agar media, cells are not fully surrounded by the high-pH aqueous media. The situation can be significantly different from the environment encountered by the bioagents for self-healing concrete. Moreover, the screening was all based on the abilities (growth, ureolysis, and/or calcite formation) of vegetative cells, while spores are the more feasible bioagents added to self-healing concrete. It is important to include spores' survivability in concrete environment in the screening strategy. Further, the reported studies did not simulate the challenging concrete environment well. The most serious damage to spores (or mycelia) likely comes from the combined high pH and temperature during the initial concrete mixing and setting stages and the subsequent longer-term (about 1 month) high pH during the curing. It is important to screen the microorganisms for their spores' capability to survive and remain dormant through these most damaging concrete environments and stages, and then to germinate and grow at conditions when cracks appear in the concrete structure.
The objective of this work is to develop an effective screening protocol that addresses the problems mentioned above for previous studies. As described in more detail in the next section (strain screening pyramid), the carefully designed protocol screens microorganisms for the ability of not only vegetative growth but also spore survivability on agar plates and in liquid media at concrete-relevant pH and temperature. More innovatively, the protocol also examines/compares the performance of different microorganisms when going through the essential process/scenario of microbe-effected self-healing of concrete, that is, the ability of microbial spores to remain viable but dormant through the harsh condition during concrete mixing and curing, and the ability to germinate later at the less harsh condition in carbonated concrete cracks. The screening protocol was applied to 18 potentially alkalophilic/alkalotolerant fungi in this study and was successful in identifying the more promising fungal strains for use in further development of self-healing concrete.
A hierarchy has been designed with 4 screening tests, and the 4 tests are increasingly more complex and time-consuming from Test 1 to Test 4. The design helps reduce the number of strains to test by the more effort-demanding procedures and creates a decision-making point for considering additional spore protection designs. The pyramid of criteria is shown in Figure 1. While experimental details are described in the materials and methods section, the rationales are given here. All strains considered (from experience and literature survey) were screened by Test 1 where the cells were inoculated on agar plates containing nutrient solutions that had been adjusted to high initial pH (e.g., 10 and 11), to examine the extent of negative effect of the high pH on cell growth. Those that could grow were then subjected to Test 2, where cells were inoculated into liquid nutrient media of high pH (10). The main purpose of Test 2 was to confirm the strain's ability of submerged growth in high-pH liquid media, and the strains that could not grow would be removed from further testing. The test also allowed monitoring of the pH change during cell growth at high pH. Some microorganisms have evolved to survive high pH environments by producing organic acids that lower the pH locally. However, the microbial acids produced can negatively affect the integrity of cementitious structures and can cause corrosion of the reinforcing steel. By Test 2, we can detect the active acid-producers for more cautious consideration of their use in self-healing concrete.
In Test 3, spores of the candidates that passed Test 2 were evaluated for germination at a moderately high pH which is relevant to the cementitious environments under carbonation of ambient air (e.g., 9.5) like inside the cracks, with comparison to the germination at neutral pH as control. The species passing Test 3 were further evaluated by Test 4 where the spores were first exposed to the high pH and/or temperature conditions relevant to those encountered during mixing, setting, and curing of cementitious materials (e.g., pH 12.9 and 45 or 55 °C) and then observed for spore germination ability at neutral or moderately high pH. If no spores from any screened strains can tolerate these simulated concrete conditions, designs need to be considered for protecting spores from the damaging factors.
A literature review was first conducted to understand the distribution of fungi in high pH environments and establish a list of potential alkalophilic and alkalotolerant fungi that have reported capability to grow at pH ≥ 8.5. Fungi recorded as plant, animal, and human pathogens were avoided. 18 strains were then selected and obtained from the NRRL (Northern Regional Research Laboratory) culture collection (Agricultural Research Service, US Department of Agriculture). The 18 strains were Aspergillus glaucus NRRL 66587, Aspergillus thermomutatus NRRL 180, Aspergillus nidulans NRRL 187, A. nidulans NRRL 194 [24] Paecilomyces variotii NRRL 1115, Scopulariopsis brevicaulis NRRL 1100, Trichoderma reesei NRRL 3652, T. reesei NRRL 6156 [26], Myrothecium verrucaria NRRL 2003, Gliomastix murorum NRRL 62986, Purpureocillium lilacinum NRRL 895 [27], Gliocladium virens NRRL 2308, Gliocladium sp. NRRL 22971 [28], Cladosporium cladosporioides NRRL 3182 [29], Penicillium expansum NRRL 62431, Penicillium citrinum NRRL 756 [30], Chrysosporium sp. NRRL 22978 [31], and Aspergillus niger NRRL 341 [32]. None of these strains required USDA Animal and Plant Health Inspection Service' (APHIS') Plant Protection and Quarantine (PPQ) 526 permit or Veterinary Services (VS) 16-3 permit. The fungal cultures were maintained on potato dextrose agar (PDA, 40 g/L, pH 7) (Sigma Aldrich 70139).
This test was done with potato dextrose agar (PDA, 40 g/L) plates prepared with 3 pH values: pH 7 as control and pH 10 and 11 for evaluating cell growth at high pH. The pH 10 and 11 plates were prepared with 10 (for pH 10) and 20 (for pH 11) mM sodium carbonate-bicarbonate buffers. Each of these plates was inoculated at the center with a loop of cells collected from a pregrown PDA plate (pH 7). The inoculated plates were incubated at room temperature (21 ± 2) °C to observe growth of cells as an expanding colony. The colony diameters were measured with a slide caliper at Days 2, 4, and 6. Four plates were used for each fungal strain. Reported later in results and discussion are the averages and standard deviations of the colony diameters measured from 4 plates.
This test was done by observing cell growth in 100 mL potato dextrose broth (PDB, 40 g/L) (Sigma Aldrich P6685) prepared in 250 mL Erlenmeyer flasks. The initial medium pH was adjusted to 10 using 0.1 M NaOH. No additional buffer was added to PDB because the test was also to investigate the pH drops due to acid production by fungi while growing in the high-pH liquid media. The flasks were covered by cheesecloth-wrapped cotton. After inoculation with 3 loops of cells collected from a pregrown PDA plate (pH 7), the flasks were put on a shaker (Innova 4080 Digital Incubator Shaker New Brunswick Scientific Co., Inc) at 125 RPM (revolutions per minute) and observed for cell growth and pH change for 4 days. Cell growth, indicated by increasing broth turbidity, was observed (but not quantitated) by the naked eye and confirmed by observing samples under an optical microscope. For pH change, samples were taken from the flasks every 8 h and measured for pH. A control with no fungal inoculation was also prepared, and the abiotic pH drop of medium was measured.
Small amounts of spores were produced for each strain to test. Inoculated PDA plates were grown for 14 days at room temperature. By then, the mycelia would have covered the plates and sporulated. 30 mL of 1 g/L sterile aqueous Tween 80 (Fisher Scientific T164) solution were added to a plate, and spores were gently scraped off the culture with a sterile wire loop. 1 mL of the spore suspension collected was inoculated to a 250 mL Erlenmeyer flask containing 100 mL PDB buffered at pH 12 with 0.025 M Na2HPO4 and 0.027 M NaOH. Like in Test 2, the flasks were covered by cheesecloth-wrapped cotton and shaken at 125 RPM. Samples were then taken every 8 h to examine, using a microscope, whether spores had germinated. The time taken for the spores to germinate was recorded. Controls with PDB having an initial pH of 6.5 were also prepared and similarly inoculated with spores, for comparison of spore germination in neutral and high pH media.
PDB was prepared to have an initial pH of 12 or 12.9. The pH 12 medium was buffered with 0.05 M Na2HPO4 and 0.054 M NaOH, the pH 12.9 medium with 0.064 M KCl and 0.136 M NaOH. Spore suspensions were produced as described for Test 3. 1 mL spore suspension was added to 9 mL PDB in a 20-mL glass scintillation vial (Kimble 74504-20). A strip of Teflon tape (Anti-Seize 26135, low density polytetrafluoroethylene thread seal tape) was wrapped around the vial neck and the cap was tightly closed. The tightly capped vials were used to minimize pH decrease due to carbonation. An assessment test showed that pH would drop to 9.5–9.9 in 4 days for the shaken flasks used in Test 3, covered with cheesecloth-covered cotton, but only to 11.2 ± 0.6 for the closed-cap vials after 28 days.
To simulate the high pH-temperature conditions during concrete preparation, the vials with spores were placed for 2 h in water baths of 45 and 55 °C, respectively, or at room temperature (control). Afterward, the vials were kept at room temperature and observed for up to 21 days (simulating the concrete curing period) for spore survivability at these high pH conditions. Hereafter, these vials are referred to as the storage vials. The storage vials were sacrificed weekly, and the spores were collected and washed once with deionized water by centrifugation (Thermo Scientific Sorvall Legend X1R) for 10 min at 12000 RPM (17672 g). The washed spores were reinoculated in PDB of pH 6.5 (control) and 9.5 (simulating the carbonated pH condition in concrete cracks), respectively, and observed for spore germination. These latter sets of vials are referred to as the germination vials. Two storage vials and two germination vials were prepared for each germination test time in PDB of each pH (6.5 or 9.5), for each strain stored in PDB of each pH (12 or 12.9) and subjected to each heat-treatment condition (room temperature, 45 or 55 °C).
To help separate the damaging roles of high pH and temperature, and to narrow the threshold range of temperature tolerance by S. brevicaulis NRRL 1100, experiments were done at near neutral pH. The spores were added to multiple vials of PDB and deionized water, respectively. Then, the spore suspensions were exposed to thermal treatment for 2 h at 45, 47, 49, 51, 53, and 55 °C, respectively. An additional system was included to test the effect of treatment at 53 °C for 4 h, to assess whether the spores were very sensitive to a longer period of high-temperature exposure. For each temperature, 4 vials of spore suspensions in PDB and 4 vials in water were tested. After the heat treatment, the suspensions in PDB were kept at room temperature and observed for spore germination. The suspensions in water were periodically sacrificed to collect spores by centrifugation (10 min at 12000 RPM) and then reinoculate the collected spores in fresh PDB at pH 6.5 to observe for spore germination. Including both PDB and water suspensions in the experiments was to investigate whether the presence of nutrients in PDB would affect the spores' temperature tolerance significantly.
Ordinary Portland cement and sand were dry-heat sterilized at 150 °C in an oven for 4 h and then mixed with autoclave-sterilized deionized water at the ratio of cement: sand: water = 1: 2.75: 0.5. Two sterile petri dishes were each added with a thin layer (about 45 mL) of wet mortar mixture thus prepared. Then, 5 mL of a S. brevicaulis spore suspension were carefully added on top of the mortar layer using a pipette. These procedures created systems having a layer of aqueous spore suspension on top of a layer of wet mortar that underwent cement hydration. Samples were taken on 3, 6, 9, 14, 21 and 28 days, from the spore suspension with a sterile wire loop, which were then inoculated on PDA to observe spore germination. pH was also measured/estimated for the sampled spore suspension using pH test papers (Hydrion, Micro Essential Lab).
On pH 7 PDA (control), all 18 tested strains grew well with the colony diameter exceeding 3 cm in 3 to 4 days. On pH 10 and 11 PDA, 7 of the strains showed no growth by 6 days. These strains were P. citrinum NRRL 756, Gliocladium sp. NRRL 22971, A. thermomutatus NRRL 180, P. variotii NRRL 1115, T. reesei NRRL 6156, Chrysosporium sp. NRRL 22978, and A. glaucus NRRL 66587. Test 1 results excluded them from further screening tests.
For the other 11 strains, the profiles of expanding colony diameter on PDA at initial pH 10 and 11 are shown in Figure 2. Many of these strains showed growth delays at high pH, presumably because the pH of PDA plates (although buffered) dropped with time due to carbonation by the ambient CO2 diffusing in. A. nidulans NRRL 187 and P. expansum NRRL 62431 managed to grow at pH 10 but could not grow at pH 11. G. virens NRRL 2308 grew after 4 days at both pH 10 and 11. The growth of A. nidulans NRRL 194 was delayed by 2 days at pH 10 and 4 days at pH 11. T. reesei NRRL 3652, P. lilacinum NRRL 895, and M. verrucaria NRRL 2003 showed 2-day growth delays at both pH 10 and 11. Only C. cladosporioides NRRL 3182, G. murorum NRRL 62986, S. brevicaulis NRRL 1100, and A. niger NRRL 341 did not show growth delays at these high pH's; their colony growth was visible and measurable by 2 days. It should be noted that the colonies of different strains could differ in biomass packing densities; some tended to spread rapidly with thinner colonies, while others formed densely packed colonies that spread more slowly. Therefore, the strains with faster colony spreading rates did not necessarily have higher biomass production rates (not measured here).
This step was done on the 11 strains that passed Test 1. The initial pH of the liquid growth medium was adjusted to pH 10 and the lowest pH values observed during 4 days of growth are given in Table 1. 4 stains: A. nidulans NRRL 194, A. niger NRRL 341, T. reesei NRRL 3652, and P. expansum NRRL 62431, could not grow in this high-pH liquid medium, although they could grow on PDA at high pH in Test 1. These strains were eliminated from further testing. The pH on PDA surface might have dropped faster, due to carbonation, than the pH in liquid medium because PDA plates had a larger surface area-to-volume ratio, and the cells grew right on the agar surface. This observation also indicated the strain-dependent sensitivity to high pH for surface growth (with only partial exposure to the high pH) versus submerged growth.
G. virens NRRL 2308, M. verrucaria NRRL 2003, and P. lilacinum NRRL 895 managed to grow in the high pH liquid medium, but they dropped the pH to 5.08–5.50; they were considered as high acid-producing strains. C. cladosporioides NRRL 3182 produced less acids and dropped the pH to 6.76. S. brevicaulis NRRL 1100, G. murorum NRRL 62986, and A. nidulans NRRL 187 were low acid producers, dropping pH only to 8.25–8.49.
Strain | Lowest pH | Acid production |
Abiotic control | 9.25 | Carbonation |
A. niger NRRL 341 | 9.25 | No growth |
T. reesei NRRL 3652 | 9.11 | No growth |
P. expansum NRRL 62431 | 8.94 | No growth |
A. nidulans NRRL 194 | 8.88 | No growth |
A. nidulans NRRL 187 | 8.49 | Very low |
G. murorum NRRL 62986 | 8.46 | Very low |
S. brevicaulis NRRL 1100 | 8.25 | Very low |
C. cladosporioides NRRL 3182 | 6.76 | Low |
P. lilacinum NRRL 895 | 5.50 | High |
M. verrucaria NRRL 2003 | 5.40 | High |
G. virens NRRL 2308 | 5.08 | High |
This step was done on the 7 strains that passed Test 2. Spores of all 7 strains germinated in PDB of initial pH 6 by 36 h, but high pH delayed or prevented their germination. The determination was made by taking periodic samples and observing them under a microscope. As shown in Figure 3, spore germination and stages of subsequent mycelial extension and branching are clearly differentiable, enabling determination of the time required for spore germination and the increasing percentage of spore germination with time. For example, the profiles of increasing germination percentage for S. brevicaulis at initial pH 6 and 12, respectively, are shown in Figure 4. At pH 6, the spores started to germinate after 6–7 h and practically all germinated after about 30 h. In the high pH medium, buffered with 25 mM Na2HPO4 and 27 mM NaOH, spores germinated much later, starting after 30–45 h and completing after about 96 h. The test was done in flasks covered with cotton placed between two layers of cheesecloth. In cell-free controls, carbonation was found to drop the medium pH from 12 to about 10.6 in 1 day and to 9.5–9.9 in 4 days, then the pH remained at the 9.5–9.9 range without clear further decrease (figure not shown). The results indicated that S. brevicaulis spores could survive through high initial pH 12 and started to germinate in the pH range of 10.3–10.5 (estimated using the pH profile of cell-free control).
Germination of the spores of the 7 strains differed significantly in PDB of initial pH 12. For comparison, the times when spores of the 7 strains were first found to germinate (e.g., 45 h for S. brevicaulis in Figure 4) in the high pH medium are given in Table 2. Also given in Table 2 are the corresponding pH ranges when germination occurred, estimated using the pH profiles from the cell-free control flasks. Out of the 7 strains, 4 of them, i.e., S. brevicaulis, A. nidulans, P. lilacinum, and M. verrucaria showed positive spore germination after 48 to 72 h in the pH ranges of 10.3–10.5 to 9.9–10.1. These pH ranges are lower than the pH of cement slurry (about 13) but higher than the pH in carbonated cracks (about 9.5). The finding suggested that spores of these strains would remain dormant (but viable if properly protected against other non-pH-related damages) during the initial mixing, setting, and curing stages but could germinate when exposed to cracks developing in cementitious structures. Among these 4 strains, as shown in Table 1, P. lilacinum and M. verrucaria are high acid-producing strains and potentially less suitable for use in concrete than S. brevicaulis and A. nidulans. Spores of the other 3 strains, i.e., G. murorum, G. virens, and C. cladosporioides, could not germinate after 84 h, either because the spores were severely damaged by exposure to high pH or because they needed lower pH for germination. Accordingly, these strains are not suitable for use in self-healing concrete.
Strain | Initial spore germination observed |
|
Time | pH | |
S. brevicaulis (NRRL 1100) | 48 h | 10.3–10.5 |
A. nidulans (NRRL 187) | 48 h | 10.3–10.5 |
P. lilacinum (NRRL 895) | 64 h | 10.1–10.3 |
M. verrucaria (NRRL 2003) | 72 h | 9.9–10.1 |
G. murorum (NRRL 62986) | Not germinated (84 h) | |
G. virens (NRRL 2308) | Not germinated (84 h) | |
C. cladosporioides (NRRL 3182) | Not germinated (84 h) |
This test was performed on the 4 promising strains identified from the above Tests 1–3. Unlike in Test 3, here the spores were kept in tightly closed vials to minimize the pH drop due to carbonation. Tests showed the pH remained at 11.2 ± 0.6 even after 28 days (figure not shown). The vials of high-pH spore suspensions were also subjected to heat treatment for 2 h at 45 and 55 °C, respectively, in addition to the control at room temperature, before being stored at room temperature for up to 21 days with weekly sampling and reinoculation into 6.5 or 9.5 PDB for spore germination observation.
This screening test was designed to answer two important questions for the intended use of spores in self-healing concrete:
Question 1: Would spores remain dormant or germinate at the high pH of intact concrete, after exposure to high pH and elevated temperature during the initial mixing and setting stages?
Including all systems for all tested strains, spores in 280 out of 288 high-pH storage vials remained dormant without germination throughout the 21-day testing period. This finding is good for self-healing concrete because we would like the spores to remain dormant in intact concrete and germinate only when cracks have developed. The few exceptions with spore germination in the storage vials occurred with S. brevicaulis and P. lilacinum after 21 days of storage (Table 3). As shown in Table 2 of Test 3, spores of these more high-pH tolerant strains could germinate at pH 10.3–10.5 and 10.1–10.3, respectively. Therefore, the exceptions probably occurred because these vials were not sealed as tightly as normal/expected and the carbonation dropped the pH slightly more, to levels allowing these strains to germinate after 21 days.
Question 2: Could the dormant spores at high concrete pH germinate later when exposed to lower pH in cracks?
The test results answering this question are presented in Table 3 (in a heatmap format). The table is arranged in the descending order of observed tolerance to harsh concrete conditions: S. brevicaulis > P. lilacinum > M. verrucaria > A. nidulans (NRRL 187). Results were first considered for all 4 strains together to delineate the pH and temperature effects. For the effect of treatment and storage pH, considering results from all treatment temperatures and storage durations, pH 12.9 was substantially more damaging than pH 12 to spores of these alkalotolerant strains; spores in only about 22% (32 out of 144) of the pH 12.9 systems could survive and germinate after being reinoculated whereas 62% (89/144) from the pH 12 systems could survive and germinate. For the effect of 2-h treatment temperature, considering results from all storage pH and durations, 55 °C was substantially more damaging than 45 °C. The percentage of storage vials showing germination of reinoculated spores was about 67% (64/96) for those without high temperature (45 or 55 °C) treatment; the percentage decreased moderately to 49% (47/96) for vials treated at 45 °C but drastically to only 10% (10/96) for vials treated at 55 °C. pH 12 alone, without high temperature treatment, was not seriously damaging to spores of these selected alkalotolerant strains because spores from 96% (46/48) of the storage vials could germinate after reinoculation in pH 6.5 and 9.5 media; however, pH 12.9 alone was already very damaging, allowing spores reinoculated from only 38% (18/48) of vials to germinate. The most damaging condition was the combined high treatment/storage temperature and pH; no spores survived (for 7 or more storage days) in the pH 12.9 system with 2-h heat treatment at 55 °C.
As for the tolerance of different strains, S. brevicaulis was the toughest against the high pH and/or temperature. After reinoculation, S. brevicaulis spores germinated from 64% of all storage vials, i.e., 46 out of 72 vials testing the effects of two pH (12 and 12.9) and three temperatures (room temperature (RT), 45 and 55 °C), followed by P. lilacinum at 53% (38/72), M. verrucaria at 32% (23/72), and A. nidulans (NRRL 187) at 19% (14/72). Because pH 12.9 is the likely pH that unprotected spores would encounter during concrete mixing and curing stages, the tolerance of spores to this higher pH is focused more here for answering the above-posted Question 2. A. nidulans (NRRL 187) and M. verrucaria struggled to germinate after being exposed to pH 12.9 even without the high temperature (45 or 55 °C) treatment. Therefore, if no special spore-protection designs are used, the answer to Question 2 for these 2 strains is negative, and they are less suitable for use in self-healing concrete. For S. brevicaulis and P. lilacinum, the answer is a conditional yes, depending on the temperature reached during concrete mixing and curing. For S. brevicaulis, the germination rates were comparable for vials without and with heat treatment at 45 °C, averaging at 71% (17/24). For P. lilacinum, the germination rate was 58% (7/12) from vials without high-temperature heat treatment; for vials treated for 2 h at 45 °C, the rate dropped to 33% (4/12) and germination occurred only from the 7-day storage vials. For both S. brevicaulis and P. lilacinum strains, no spores from the vials treated at 55 °C could germinate after reinoculation. Because in concrete applications 55 °C may only be reached under strictly adiabatic conditions [7] and the surface temperature was generally lower than 35–40 °C [8], the results suggested that some spores of these two strains, particularly S. brevicaulis, can survive through the high pH and slightly elevated temperature in the concrete mixing-curing stages (if no other damaging factors) and germinate later when exposed to lower pH in cracks.
Importantly, the study results also indicated the significant benefits obtainable with properly designed spore protection mechanisms. By having a mechanism to just slightly lower the local pH around spores from 12.9–12, the spore germination rate would improve drastically. For S. brevicaulis, the germination rate would increase from 71% at pH 12.9 to 100% at pH 12 for vials without and with heat treatment at 45 °C, and from 0% to 42% for vials treated at 55 °C. For P. lilacinum, the germination rates would increase from 58%, 33%, and 0% at pH 12.9 to 100%, 83%, and 42% at pH 12, respectively for the room temperature, 45°C, and 55°C treatment systems. Even A. nidulans (NRRL 187) and M. verrucaria could be feasible for applications without appreciable temperature rise, germinating at about 92% (11/12).
![]() |
The strain screening results observed in this study are summarized in Table 4, in the final order of suitability for use in self-healing concrete.
![]() |
Notes: Strains passing the tests are colored in green, those failing the tests are in red. For T2, strains that grew but produced acid to lower the pH greatly are colored yellow. For T4, no strains passed all the conditions included in the test; the two more tolerant strains are colored in yellow to indicate their passing the slightly milder conditions of up to pH 12 and 45 °C.
Summarized in Tables 5 are the results of the more detailed study on the temperature tolerance, in absence of high pH, of S. brevicaulis spores, which was found to be the toughest among the 18 strains tested. After 2-h treatment at temperatures up to 51 °C, spores in all 8 vials could germinate regardless of whether the spores were suspended in PDB or deionized water during the heat treatment. However, after 2-h treatment at 53 and 55 °C, spores germinated from only 50% of the vials (2 out of 4) containing either PDB- or water-suspended spores. For spores subjected to 4-h treatment at 53 °C, germination occurred in all 4 PDB vials but none from the deionized water vials (after reinoculation to PDB). These results concluded that, in absence of the concurring damaging effect from high pH, S. brevicaulis spores can tolerate temperatures up to 51 °C without substantial damage. At higher, more damaging temperatures of 53 and 55 °C, the results were inconclusive on the two side effects tentatively investigated in this study: (1) effect of nutrient presence, by comparing the nutrient-containing PDB vs. deionized water as the suspending medium during heat treatment, and (2) effect of longer heat treatment duration, by comparing 4-h vs. 2-h treatment at 53 °C. A more extensive study is needed to conclude on these effects. Nevertheless, the observations did not suggest that the S. brevicaulis spores were very sensitive to longer periods (> 2 h) of exposure to 53 °C.
For separating the roles of high pH and temperature in the damage to S. brevicaulis spores, the results in Tables 3 and 5 for treatment at 55 °C can be compared. The 50% germination seen in media at near-neutral pH (Table 5) is same/similar to the 50% germination seen in media at pH 12, even after further storage for 7 or 14 days at room temperature in the high pH media (Table 3), presuming the S. brevicaulis spores do not germinate significantly differently in PDB at pH 6.5 and 9.5. On the other hand, the spores did not germinate from any vials treated at 55 °C and pH 12.9 (Table 3). Accordingly, the results suggested that pH up to 12 did not significantly add further damage to the S. brevicaulis spores exposed to 55 °C, but pH 12.9 did. This again indicated the important benefit of incorporating spore protection mechanisms to slightly lower the local pH around spores (say, from 12.9 to 12).
![]() |
Note: Heatmap color codes: green-germinated, and red-did not germinate; germination observed either directly in PDB or, for spores heat-treated in deionized water, after being reinoculated in PDB. * This system was treated at high temperature for 4 h, instead of 2 h as for all other systems.
In this experiment, an aqueous suspension of S. brevicaulis spores were added on top of a freshly laid layer of wet mortar mixture undergoing cement hydration (details given in materials and methods). Due to higher density, the spores gradually settled onto the surface of mortar slurry, forming close spore-mortar contact. The aqueous samples taken from such a setup consistently changed the pH test strip color to one that corresponded most closely to pH 13, showing no significant pH decrease due to carbonation. The spores in samples collected at up to 14 days consistently germinated from all inoculation points on the PDA plates. However, only one out of 4 inoculation points germinated for the samples collected at 21 days, and none germinated for samples collected at 28 days.
Because of the small amount of cement used in this experimental setup, there was no appreciable temperature increase in the aqueous spore suspension added on top of the mortar mixture. Therefore, the condition resembled most closely the vials of S. brevicaulis spores at pH 12.9 and room temperature (i.e., without heat treatment at 45 or 55 °C) in Table 3 from the screening Test 4. The germination rate from those vials (Table 3) was 100% at 7 days, 50% at 14 days, and 25% at 21 days, similar to the germination rates observed in this experiment: 100% at up to 14 days, 25% at 21 days, and 0% at 28 days. Note that in Test 4, the high pH was created by addition of a NaOH-containing buffer. Here, the high pH was from complex cement hydration products, mainly Ca(OH)2 but including also various other soluble inorganic ions. This experiment confirmed that, in absence of elevated temperature, the selected S. brevicaulis spores could survive the extreme pH associated with cement hydration for about 14–21 days, and the various soluble inorganic ions from cement/mortar did not cause apparent toxicity to the spores.
This work was the first of its kind in developing a screening protocol that closely mimicked the concrete-relevant pH and temperature in not only the concrete mixing and curing stages but also the carbonated concrete cracks. Further, the final step in the protocol was innovatively designed to screen microorganisms for performance through the essential process/scenario of MESHC, that is, the ability of microbial spores to remain viable but dormant through the harsh condition of concrete mixing and curing, and the ability to germinate later at the less harsh condition in carbonated concrete cracks. The screening test results indicated that the high pH and elevated temperature present in concrete, particularly during mixing and curing stages, could negatively affect fungal growth and damage fungal spores, and the effects varied significantly with specific fungi. Results confirmed that the high pH would prevent spores from germination during mixing and curing, and that the dormant spores, if from suitable strains and adequately protected, could germinate at the carbonation-lowered pH inside concrete cracks. The screening protocol selected S. brevicaulis as the top candidate from 18 alkalotolerant/alkalophilic fungal strains. But even the spores of the selected S. brevicaulis strain could not survive the harshest condition used in the screening: 2-h exposure of spore suspension at pH 12.9 to 55 °C. The study results indicated the significant benefits obtainable from incorporating proper designs to lower the local pH near spores, even if just to slightly lower the pH from 12.9 to 12. Temperature should also be limited to below 53 °C, as higher temperatures would significantly impact the survivability of S. brevicaulis spores, even in absence of high pH. The screening test results were consistent with the germination results of S. brevicaulis spores in contact with wet mortar mixture undergoing cement hydration, supporting the adequacy of the screening design, and indicating no apparent toxic effect of mortar ingredients on the spores. Overall, the developed screening protocol and the screening results from the 18 tested fungi are valuable for facilitating the development of self-healing concrete, which in turn resolves the concrete service-life shortening due to cracking and decreases the amount of new concrete production and its associated massive energy consumption and CO2 emission.
The authors declare they have not used Artificial Intelligence (AI) tools in the creation of this article.
[1] | Mamlouk MS, Zaniewski JP (2011) Materials for Civil and Construction Engineers. New York: Prentice Hall. |
[2] |
Pacheco J, Polder R (2012) Corrosion initiation and propagation in cracked concrete–a literature review. Advances in Modeling Concrete Service Life. Dordrecht: Springer 85-93. ![]() |
[3] |
Silva FB, Boon N, De Belie N, et al. (2015) Industrial application of biological self-healing concrete: Challenges and economical feasibility. J Commer Biotechnol 21: 31-38. https://doi.org/10.5912/jcb662 ![]() |
[4] |
Amran M, Onaizi AM, Fediuk R, et al. (2022) Self-healing concrete as a prospective construction material: A review. Materials 15: 1-46. https://doi.org/10.3390/ma15093214 ![]() |
[5] |
Williamson RB (1968) Constitutional supersaturation in Portland cement solidified by hydration. J Cryst Growth 3: 787-794. https://doi.org/10.1016/0022-0248(68)90267-4 ![]() |
[6] |
Behnood A, Van Tittelboom K, De Belie N (2016) Methods for measuring pH in concrete: A review. Constr Build Mater 105: 176-188. https://doi.org/10.1016/j.conbuildmat.2015.12.032 ![]() |
[7] |
Zou X, Chao A, Tian Y, et al. (2012) An experimental study on the concrete hydration process using Fabry-Perot fiber optic temperature sensors. Measurement 45: 1077-1082. https://doi.org/10.1016/j.measurement.2012.01.034 ![]() |
[8] |
Siang GC (2017) Determination of temperature rise and temperature differentials of CEMII/B-V cement for 20 MPa mass concrete using adiabatic temperature rise data. IOP Conf Ser Mater Sci Eng 217: 012008. https://doi.org/10.1088/1757-899X/217/1/012008 ![]() |
[9] |
Matthieu B, Farid B, Jean-Michel T, et al. (2012) Analysis of semi-adiabatic tests for the prediction of early-age behavior of massive concrete structures. Cem Concr Compos 34: 634-641. https://doi.org/https://doi.org/10.1016/j.cemconcomp.2011.09.001 ![]() |
[10] |
Kuriakose B, Rao BN, Dodagoudar GR (2016) Early-age temperature distribution in a massive concrete foundation. Procedia Technol 25: 107-114. https://doi.org/10.1016/j.protcy.2016.08.087 ![]() |
[11] | Straube J (2000) Moisture properties of plaster and stucco for strawbale buildings. CMHC . https://www.ecobuildnetwork.org/ |
[12] | Possan E, Thomaz WA, Aleandri GA, et al. (2017) CO2 uptake potential due to concrete carbonation: A case study. Case Stud Constr Mat 6: 147-161. https://doi.org/10.1016/j.cscm.2017.01.007 |
[13] |
Ji Y, Yuan Y, Shen J, et al. (2010) Comparison of concrete carbonation process under natural condition and high CO2 concentration environments. J Wuhan Univ Technol 25: 515-522. https://doi.org/10.1007/s11595-030-0034-y ![]() |
[14] |
Van Wylick A, Rahier H, De Laet L, et al. (2024) Conditions for CaCO3 biomineralization by Trichoderma reesei with the perspective of developing fungi-mediated self-healing concrete. Glob Chall 8: 2300160. https://doi.org/10.1002/gch2.202300160 ![]() |
[15] | Jonkers HM (2011) Bacteria-based self-healing concrete. Heron 56: 1-12. |
[16] |
Jonkers HM, Thijssen A, Muyzer G, et al. (2010) Application of bacteria as self-healing agent for the development of sustainable concrete. Ecol Eng 36: 230-235. https://doi.org/10.1016/j.ecoleng.2008.12.036 ![]() |
[17] |
Gao M, Guo J, Cao H, et al. (2020) Immobilized bacteria with pH-response hydrogel for self-healing of concrete. J Environ Manage 261: 110225. https://doi.org/10.1016/j.jenvman.2020.110225 ![]() |
[18] |
Khushnood RA, Ali AM, Faraz Bhatti M, et al. (2022) Self-healing fungi concrete using potential strains Rhizopus oryzae and Trichoderma longibrachiatum. J Build Eng 50: 104155. https://doi.org/10.1016/j.jobe.2022.104155 ![]() |
[19] |
Houshmand Khaneghahi M, Rahmaninezhad SA, Kamireddi D, et al. (2024) Carbonate biomineralization potential of endospore-laden polymeric fibers (BioFibers) for bio-self-healing applications. Develop Built Environment 17: 100351. https://doi.org/10.1016/j.dibe.2024.100351 ![]() |
[20] |
Van Wylick A, Brouwers E, Rahier H, et al. (2024) Encapsulation of Trichoderma reesei and Neurospora crassa in calcium alginate capsules for fungi-mediated self-healing concrete: An explorative study on the protocol, survival of the organisms and CaCO3 biomineralization. Develop Built Environment 18: 100445. https://doi.org/10.1016/j.dibe.2024.100445 ![]() |
[21] |
Zhang JL, Wu RS, Li YM, et al. (2016) Screening of bacteria for self-healing of concrete cracks and optimization of the microbial calcium precipitation process. Appl Microbiol Biotechnol 100: 6661-6670. https://doi.org/10.1007/s00253-016-7382-2 ![]() |
[22] |
Martuscelli C, Soares C, Camões A, et al. (2020) Potential of fungi for concrete repair. Procedia Manuf 46: 180-185. https://doi.org/10.1016/j.promfg.2020.03.027 ![]() |
[23] |
Luo J, Chen X, Crump J, et al. (2018) Interactions of fungi with concrete: Significant importance for bio-based self-healing concrete. Constr Build Mater 164: 275-285. https://doi.org/10.1016/j.conbuildmat.2017.12.233 ![]() |
[24] |
Menon RR, Luo J, Chen X, et al. (2019) Screening of fungi for potential application of self-healing concrete. Sci Rep 9: 2075. https://doi.org/10.1038/s41598-019-39156-8 ![]() |
[25] |
Zhao J, Csetenyi L, Gadd GM (2022) Fungal-induced CaCO3 and SrCO3 precipitation: A potential strategy for bioprotection of concrete. Sci Total Environ 816: 151501. https://doi.org/10.1016/j.scitotenv.2021.151501 ![]() |
[26] | Kladwang W, Hywel-Jones N, Bhumirattana A (2003) Alkaline-tolerant fungi from Thailand. Fungal Divers 13: 69-84. |
[27] |
Grum-Grzhimaylo AA, Georgieva ML, Debets AJM, et al. (2013) Are alkalitolerant fungi of the Emericellopsis lineage (Bionectriaceae) of marine origin?. IMA Fungus 4: 213-228. https://doi.org/10.5598/imafungus.2013.04.02.07 ![]() |
[28] |
Nagai K, Sakai T, Rantiatmodjo RM, et al. (1995) Studies on the distribution of alkalophilic and alkali-tolerant soil fungi I. Mycoscience 36: 247-256. https://doi.org/10.1007/BF02268598 ![]() |
[29] |
Grum-Grzhimaylo AA, Georgieva ML, Bondarenko SA, et al. (2016) On the diversity of fungi from soda soils. Fungal Divers 76: 27-74. https://doi.org/10.1007/s13225-015-0320-2 ![]() |
[30] | Anke T, Schüffler A (2018) Physiology and Genetics: Selected Basic and Applied Aspects. Berlin: Springer. |
[31] |
Nagai K (1998) Studies on the distribution of alkalophilic and alkali-tolerant soil fungi II: Fungal flora in two limestone caves in Japan. Mycoscience 39: 293-298. https://doi.org/10.1007/bf02464011 ![]() |
[32] |
Kaur G, Siddique R, Rajor A (2013) Micro-structural and metal leachate analysis of concrete made with fungal treated waste foundry sand. Constr Build Mater 38: 94-100. https://doi.org/10.1016/j.conbuildmat.2012.07.112 ![]() |
Strain | Lowest pH | Acid production |
Abiotic control | 9.25 | Carbonation |
A. niger NRRL 341 | 9.25 | No growth |
T. reesei NRRL 3652 | 9.11 | No growth |
P. expansum NRRL 62431 | 8.94 | No growth |
A. nidulans NRRL 194 | 8.88 | No growth |
A. nidulans NRRL 187 | 8.49 | Very low |
G. murorum NRRL 62986 | 8.46 | Very low |
S. brevicaulis NRRL 1100 | 8.25 | Very low |
C. cladosporioides NRRL 3182 | 6.76 | Low |
P. lilacinum NRRL 895 | 5.50 | High |
M. verrucaria NRRL 2003 | 5.40 | High |
G. virens NRRL 2308 | 5.08 | High |
Strain | Initial spore germination observed |
|
Time | pH | |
S. brevicaulis (NRRL 1100) | 48 h | 10.3–10.5 |
A. nidulans (NRRL 187) | 48 h | 10.3–10.5 |
P. lilacinum (NRRL 895) | 64 h | 10.1–10.3 |
M. verrucaria (NRRL 2003) | 72 h | 9.9–10.1 |
G. murorum (NRRL 62986) | Not germinated (84 h) | |
G. virens (NRRL 2308) | Not germinated (84 h) | |
C. cladosporioides (NRRL 3182) | Not germinated (84 h) |
![]() |
![]() |
Notes: Strains passing the tests are colored in green, those failing the tests are in red. For T2, strains that grew but produced acid to lower the pH greatly are colored yellow. For T4, no strains passed all the conditions included in the test; the two more tolerant strains are colored in yellow to indicate their passing the slightly milder conditions of up to pH 12 and 45 °C.
![]() |
Note: Heatmap color codes: green-germinated, and red-did not germinate; germination observed either directly in PDB or, for spores heat-treated in deionized water, after being reinoculated in PDB. * This system was treated at high temperature for 4 h, instead of 2 h as for all other systems.
Strain | Lowest pH | Acid production |
Abiotic control | 9.25 | Carbonation |
A. niger NRRL 341 | 9.25 | No growth |
T. reesei NRRL 3652 | 9.11 | No growth |
P. expansum NRRL 62431 | 8.94 | No growth |
A. nidulans NRRL 194 | 8.88 | No growth |
A. nidulans NRRL 187 | 8.49 | Very low |
G. murorum NRRL 62986 | 8.46 | Very low |
S. brevicaulis NRRL 1100 | 8.25 | Very low |
C. cladosporioides NRRL 3182 | 6.76 | Low |
P. lilacinum NRRL 895 | 5.50 | High |
M. verrucaria NRRL 2003 | 5.40 | High |
G. virens NRRL 2308 | 5.08 | High |
Strain | Initial spore germination observed |
|
Time | pH | |
S. brevicaulis (NRRL 1100) | 48 h | 10.3–10.5 |
A. nidulans (NRRL 187) | 48 h | 10.3–10.5 |
P. lilacinum (NRRL 895) | 64 h | 10.1–10.3 |
M. verrucaria (NRRL 2003) | 72 h | 9.9–10.1 |
G. murorum (NRRL 62986) | Not germinated (84 h) | |
G. virens (NRRL 2308) | Not germinated (84 h) | |
C. cladosporioides (NRRL 3182) | Not germinated (84 h) |
![]() |
![]() |
![]() |