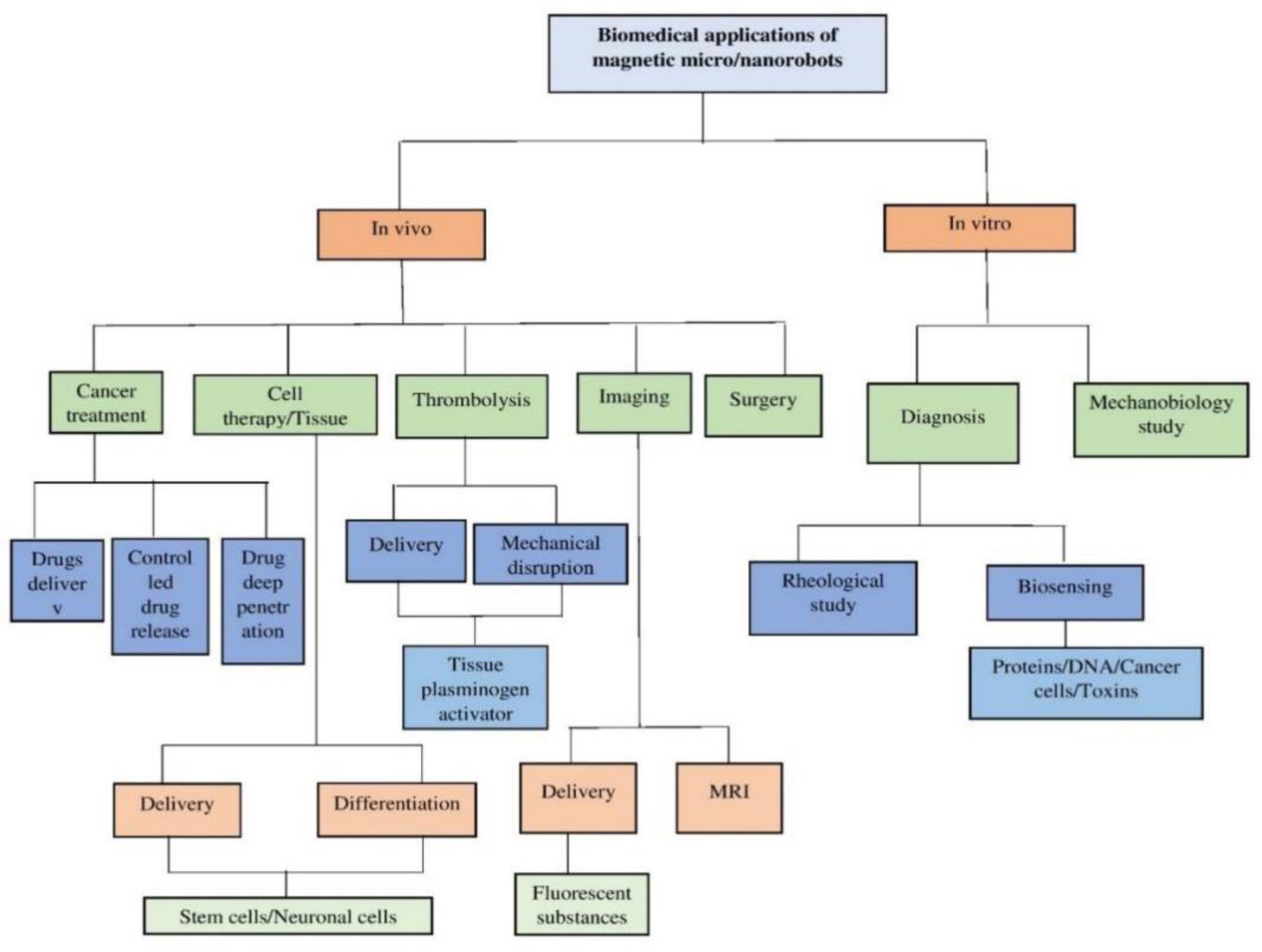
The convergence of biomedical science and robotics has ushered in an era of innovation and transformation within healthcare. This article, delves into the profound impact of this synergy, showcasing their symbiotic relationship and the potential they hold in revolutionizing patient care, medical research and healthcare delivery. From the evolution of surgical robots to advancements in medical imaging, we explore how this collaboration is redefining the boundaries of what's achievable. The exploration extends to social robots that alleviate caregiver workloads and therapeutic robots that aid patient recovery. While the benefits are evident, challenges and ethical considerations are also scrutinized. As we explore the dynamic intersection of technology and medicine, a promising transformation emerges, offering the potential for improved clinical outcomes and fresh opportunities in healthcare. This article aims to offer readers insights into the importance, challenges and possible future advancements in the fusion of biomedical science and robotics. It serves as a call to action for researchers, scientists and healthcare professionals to continually push the boundaries, explore uncharted territories and leverage this synergy to bring us closer to a world of healthier and happier lives.
Citation: Induni N. Weerarathna, Anurag Luharia. Exploring the nexus of biomedical science and robots for enhanced clinical outcomes—a literature review[J]. AIMS Bioengineering, 2024, 11(1): 1-17. doi: 10.3934/bioeng.2024001
[1] | Leelakrishna Reddy, Segun Akinola . Transforming healthcare with the synergy of biotechnology and information technology. AIMS Bioengineering, 2023, 10(4): 421-439. doi: 10.3934/bioeng.2023025 |
[2] | Daniel Calle, Duygu Yilmaz, Sebastian Cerdan, Armagan Kocer . Drug delivery from engineered organisms and nanocarriers as monitored by multimodal imaging technologies. AIMS Bioengineering, 2017, 4(2): 198-222. doi: 10.3934/bioeng.2017.2.198 |
[3] | Meher Langote, Saniya Saratkar, Praveen Kumar, Prateek Verma, Chetan Puri, Swapnil Gundewar, Palash Gourshettiwar . Human–computer interaction in healthcare: Comprehensive review. AIMS Bioengineering, 2024, 11(3): 343-390. doi: 10.3934/bioeng.2024018 |
[4] | David Raymond, Induni Nayodhara Weerarathna, John Kessellie Jallah, Praveen Kumar . Nanoparticles in biomedical implants: Pioneering progress in healthcare. AIMS Bioengineering, 2024, 11(3): 391-438. doi: 10.3934/bioeng.2024019 |
[5] | Valeria Miranda-Arizmendi, Diana Fimbres-Olivarria, Anselmo Miranda-Baeza, Agustín Rascón-Chu, Jorge Marquez-Escalante, Jaime Lizardi-Mendoza, Mayra A. Méndez-Encinas, Elizabeth Carvajal-Millan . Sulfated polysaccharides from marine diatoms: Insight into molecular characteristics and biological activity. AIMS Bioengineering, 2024, 11(1): 110-129. doi: 10.3934/bioeng.2024007 |
[6] | Houssem Ben Khalfallah, Mariem Jelassi, Jacques Demongeot, Narjès Bellamine Ben Saoud . Decision support systems in healthcare: systematic review, meta-analysis and prediction, with example of COVID-19. AIMS Bioengineering, 2023, 10(1): 27-52. doi: 10.3934/bioeng.2023004 |
[7] | Meiling Sun, Changlei Cui . Transforming lung cancer care: Synergizing artificial intelligence and clinical expertise for precision diagnosis and treatment. AIMS Bioengineering, 2023, 10(3): 331-361. doi: 10.3934/bioeng.2023020 |
[8] | P. Mora-Raimundo, M. Manzano, M. Vallet-Regí . Nanoparticles for the treatment of osteoporosis. AIMS Bioengineering, 2017, 4(2): 259-274. doi: 10.3934/bioeng.2017.2.259 |
[9] | Shital Hajare, Rajendra Rewatkar, K.T.V. Reddy . Design of an iterative method for enhanced early prediction of acute coronary syndrome using XAI analysis. AIMS Bioengineering, 2024, 11(3): 301-322. doi: 10.3934/bioeng.2024016 |
[10] | Segun Akinola, Leelakrishna Reddy . Nanoscale antenna systems: Transforming wireless communications and biomedical applications. AIMS Bioengineering, 2023, 10(3): 300-330. doi: 10.3934/bioeng.2023019 |
The convergence of biomedical science and robotics has ushered in an era of innovation and transformation within healthcare. This article, delves into the profound impact of this synergy, showcasing their symbiotic relationship and the potential they hold in revolutionizing patient care, medical research and healthcare delivery. From the evolution of surgical robots to advancements in medical imaging, we explore how this collaboration is redefining the boundaries of what's achievable. The exploration extends to social robots that alleviate caregiver workloads and therapeutic robots that aid patient recovery. While the benefits are evident, challenges and ethical considerations are also scrutinized. As we explore the dynamic intersection of technology and medicine, a promising transformation emerges, offering the potential for improved clinical outcomes and fresh opportunities in healthcare. This article aims to offer readers insights into the importance, challenges and possible future advancements in the fusion of biomedical science and robotics. It serves as a call to action for researchers, scientists and healthcare professionals to continually push the boundaries, explore uncharted territories and leverage this synergy to bring us closer to a world of healthier and happier lives.
Imagine a surgical suite where a highly skilled surgeon is accompanied by an extraordinary assistant. However, this assistant is not a fellow human but a precision-guided robot. Imagine this robot delicately suturing tissues with unparalleled accuracy, potentially reducing recovery times and even saving lives. These scenarios are not confined to the realms of science fiction, they are swiftly becoming a reality in today's operating rooms and medical facilities. In the domain where biomedical science converges with robot-assisted surgery, innovation meets precision, setting the stage for a transformative journey that holds the promise of revolutionizing clinical outcomes. This article embarks on an exploration of this cutting-edge intersection, uncovering its remarkable potential and delving into the challenges that lie on the horizon [1].
Biomedical robots also referred to as medical robots or healthcare robots, are specialized robotic systems made for a range of uses in the medical and healthcare industries. These robots can carry out specific activities in clinical settings, research labs, and other healthcare locations since they are outfitted with cutting-edge technology, sensors and precise control mechanisms. Numerous uses for biomedical robots exist, such as robotic surgery, patient care and assistance, medical imaging and diagnostics, rehabilitation and more [2]. In the realm of healthcare, the dynamic interplay between biomedical science and robotics has ushered in a new era of innovation and transformation [3]. The convergence of these two fields has not only redefined the boundaries of medical practice but has also opened up exciting possibilities for enhanced clinical outcomes. This review article, titled “Exploring the nexus of biomedical science and robots for enhanced clinical outcomes,” delves into the profound impact of this synergy on healthcare delivery, patient care and medical research. Here, we illuminate the significance of biomedical science and robotics in healthcare, showcasing their symbiotic relationship and how they collectively stand as a beacon of hope in the pursuit of better health and improved quality of life [4].
The concept of utilizing robotics in surgical procedures was initially conceived in 1967, but it remained a distant aspiration for approximately three decades. It wasn't until the United States Department of Defense established research entities that we began to witness the gradual emergence of the first surgical robots, designed to perform a variety of tasks [5]. These early robotic systems were primarily employed in military contexts, particularly on battlefields. Today, one of the most rapidly advancing domains in the integration of robotics within healthcare is the field of surgery. Its primary objective is to augment human capabilities and address inherent limitations in surgical practices. Surgical robots have found extensive utilization in performing over a million surgical procedures across various branches of the healthcare industry [6]. Artificial intelligence plays a crucial role by providing real-time alerts to surgeons and offering pertinent recommendations during surgical procedures. Deep learning data proves invaluable in tailoring surgical approaches that are most suitable for individual patients. Additionally, robotics contributes to bridging the gap for patients residing in small towns and rural areas by enabling access to expertise that is often concentrated in larger cities and less accessible to remote populations [7]. Robotics extend their impact beyond the operating room and find valuable roles in clinics and outpatient settings, ultimately elevating patient care. Notably, during the COVID-19 pandemic, robots played a pivotal role in screening individuals with suspected infections at healthcare facility entrances. This intersection of biomedical science and robotics is also evident in research laboratories, where these advanced machines adeptly tackle repetitive and manual tasks, liberating scientists to focus on more intricate aspects of their work, and thereby accelerating the pace of discoveries [8].
Furthermore, within the context of this exploration, therapeutic robots emerge as key players in post-stroke, paralysis and traumatic brain injury rehabilitation. These robots diligently monitor patients as they engage in prescribed exercises, delivering precise assessments of motion in various positions, surpassing the capabilities of the human eye. Additionally, social robots become instrumental in patient interactions, serving not only as companions but also as motivators and sources of support, exemplifying the synergy between biomedical science and robotics in advancing clinical outcomes [9]. Medical robots play a crucial role in optimizing processes and mitigating risks, rendering them highly adaptable for a wide range of applications. For instance, robots possess the capability to independently clean and arrange patients' rooms, thereby reducing the potential for interpersonal contact in wards dedicated to infectious diseases. In this context, human support robots (HSR) are employed specifically for cleaning tasks. Additionally, robots equipped with medicine identification software assist in the efficient dispensing of medications to patients within hospital settings. This level of support allows healthcare personnel to allocate more of their time to directly providing care to patients [10].
The potential for medical robotics to enhance the precision and capabilities of physicians in surgical procedures is substantial. The utilization of robots in medicine can be categorized in various ways. While diverse clinical applications are under exploration, it's important to recognize that the field of medical robotics is still in its early stages, and we are just embarking on this journey. Currently, only a few commercial companies are operating in this space, and the annual sales of medical robots remain quite limited [11]. This is partly due to the complexity of the medical environment, which makes the adoption of new technology challenging. Furthermore, the successful execution of a medical robotics project demands a collaborative partnership between engineers and clinicians, which can be challenging to establish. In terms of technological challenges and research areas within medical robotics, there's a need for advancements in system components such as system architecture, software design, mechanical design, imaging-compatible designs, user interfaces and safety measures [12].
Many different industries, including the automotive, aerospace, medicinal, culinary, construction and electronics sectors, heavily rely on 3D printing (3DP) technology [13]. Even in this day and age, traditional large robots are still made using classical manufacturing techniques for a variety of industrial purposes [14]. Among these techniques' most notable drawbacks are their high energy consumption rates and material waste. As a result, researchers are always looking for cutting-edge technology to advance the creation of soft robotics and other intelligent devices [15]. Robots at the micro- and nanoscale can likewise be produced using 3DP/4DP technology [16]. There are certain limits to traditional micro-fabrication procedures concerning material selection, design and geometries [17]. Small-scale 4DP technology has demonstrated great promise for the development of soft robotics and actuators in the domains of mechanical, material science and biomedical engineering [18]. These robots employ cutting-edge integrated technology that includes micro- and nanoscale chemistry, control systems, smart/intelligent materials and acoustics [19]. This technology combines the key characteristics of 3DP, such as its repeatability, reproducibility and controllability, with auxiliary technological advancements like the modeling of innovative 3D-structured soft robots. With the use of particular stimuli, dedicated researchers have built shape memory polymer (SMP) based soft robots. As an illustration, Keneth et al. [20] developed various 3D printable SMP inks with controlled transition and melting temperatures.
As the realms of biomedical science and robotics intertwine, they bring with them the promise of revolutionary advancements in healthcare. However, this collaboration is not without its share of formidable challenges and complexities. Understanding and addressing these issues are paramount for harnessing the full potential of biomedical science and robots in improving clinical outcomes [21]. This review article embarks on a journey to dissect and navigate these challenges. From ethical dilemmas surrounding autonomous surgical robots to the intricacies of integrating cutting-edge technology into the established healthcare infrastructure, we will scrutinize the hurdles that must be overcome. In doing so, we aim to provide a comprehensive understanding of the multifaceted landscape that lies ahead, where biomedical science and robotics are poised to redefine the future of healthcare. The purpose of this review article is to illuminate the transformative potential of the collaboration between biomedical science and robotics in the field of healthcare. In doing so, we seek to provide a comprehensive understanding of how this convergence addresses the limitations of current health practices and offers solutions for improved clinical outcomes. Our review will encompass an exploration of the significance of this interdisciplinary synergy, an examination of the pressing challenges and ethical considerations it presents and an in-depth analysis of its practical applications. Throughout this article, we will preview key points, ranging from the positive impact on patient care to the ethical concerns associated with robotic integration, providing readers with a roadmap to navigate the intricate landscape where science and technology converge to redefine the future of healthcare.
A comprehensive search strategy was employed to identify relevant sources for this review article, which explores the intersection of biomedical science and robotics for enhanced clinical outcomes. Academic databases, including PubMed, Web of Science and Scopus, were systematically queried to retrieve pertinent studies, reviews and reports published between 2010 and the present. The search strategy incorporated precise keywords such as “Biomedical Science”, “Robotics”, “Healthcare Innovation”, “Clinical Outcomes” and “Technology Integration” to ensure the identification of studies at the nexus of these fields.
In line with predefined inclusion and exclusion criteria, articles, reviews and reports that directly addressed the research question were selected. The inclusion criteria stipulated that chosen sources be written in the English language, discuss recent advances in biomedical science applied in the context of clinical outcomes, explore the integration of robotics in healthcare innovation and encompass studies conducted between 2010 and 2023. This rigorous search methodology was designed to capture a comprehensive and up-to-date body of literature, facilitating a robust analysis of the subject matter.
In the not-so-distant future, your surgeon might not have a heartbeat, yet their hands will be as steady as a rock. Imagine a world where a patient's recovery doesn't just depend on human expertise but is significantly enhanced by the precise movements of a robotic scalpel. This is the captivating reality that unfolds at the nexus of biomedical science and robotics. As we venture into this remarkable intersection of technology and medicine, we witness a transformation that promises to revolutionize clinical outcomes, offering new hope and opportunities in the realm of healthcare. From diagnostics to surgery and patient care, the collaboration of science and machines is forging a path toward unprecedented advancements in healthcare [22].
Today, the exploration of the synergy between biomedical science and robotics is of paramount significance. Recent advancements in biomedical science have opened new frontiers in healthcare, while robotic technology has become increasingly sophisticated. This nexus promises to revolutionize clinical outcomes, offering more precise diagnostics, personalized treatments and enhanced patient care. Moreover, in the face of the ongoing COVID-19 pandemic, the deployment of robots in healthcare settings to reduce pathogen exposure underscores the urgency of this topic. The convergence of these fields has the potential to address pressing healthcare challenges, making it a timely and pivotal subject of inquiry [23].
Micro robots and nanorobots are small-scale manipulable devices at the micrometer and nanometer scale that have applications in many fields and have attracted a lot of interest in the healthcare field [24]. The small size of these devices makes it feasible to execute far less invasive operations instead of surgery and non-targeted chemical and radiation therapy, which is particularly useful in the healthcare sector [25]. This greatly facilitates sickness diagnosis and detection and reduces the risk of infection, complications, side effects and patient recovery time. Furthermore, they are widely used in other laboratory-based biomedical applications such as biological fluid property studies, tissue and genetic engineering, and imaging [26]. Robots operate at micro and nanoscales by magnetic field actuation, including their actuation techniques and biomedical applications. Magnetic fields are useful in biomedicine because of the body's permeability to magnetic fields and their minimal interaction with tissues below magnetic field magnitudes of 3 Tesla (T) [27]. The main use of magnetic nanotechnology in biomedicine in the early phases was the use of magnetic nanoparticles (MNPs), whose manipulation mostly hinged on how magnetic materials respond to magnetic field gradients [28]. Magnetic separation for laboratory synthesis and analysis, magnetic drug targeting, imaging, hyperthermia and diagnostics are a few of these applications [29]. Figure 1 summarizes the present uses of micro/nanorobots in biomedical applications. In vivo and in vitro applications are the two main groups into which they can be divided. We have presented the common uses for medical micro-robots, with typical illustrations provided in Figure 2.
Robotics, a rapidly evolving field driven by artificial intelligence (AI), has seen substantial integration into the realm of surgical medicine over the past few decades. These surgical robotic systems, initially introduced in 1985, have become a common sight in operating rooms. Their primary role is to assist in surgical procedures, offering a level of precision and stability in surgical actions. These systems are typically operated or supervised by human experts. A prime example is the da Vinci Surgical System, approved by the Food & Drug Administration (FDA), which stands as one of the most prevalent robotic systems used in minimally invasive surgeries. It utilizes multiple robotic arms remotely controlled by a console, effectively replicating a surgeon's hand movements and delivering precise actions while filtering out tremors [32]. Furthermore, substantial strides are being taken in the advancement of autonomous robots capable of independently executing specific tasks, offering valuable time-saving benefits in surgical practice. Notably, robots equipped with the ability to autonomously perform tasks like suturing and cochleostomy have already been created. It is hoped that ongoing advancements in deep learning and computer vision will pave the way for the automation or semi-automation of various medical procedures, promising increased efficiency and precision in healthcare settings [33].
The comparison table below provides an overview of various robots used in biomedical applications (Table 1). They are currently being implemented in hospitals and treatment centers to improve the quality of care and patient outcomes.
Robot name | Application(s) | Key features | Benefits |
da Vinci Surgical Robot [34]. | Surgical procedures | 3D high-definition vision, precise incisions | Enhanced control, faster patient healing |
Xenex Germ-Zapping Robot [35] | Disinfection of hospital rooms | Pulsed UV rays, kill infectious bacteria | Reduces hospital-acquired infections |
PARO Therapeutic Robot [36] | Improving the quality of life during recovery | Interactive, resembles an animal | Reduces stress, provides comfort |
CyberKnife [37] | Radiation therapy for tumors | Sub-millimeter precision, non-invasive | Precise tumor targeting, minimal radiation |
TUG [38] | Hospital material transportation | Autonomous, sensor-equipped, navigates hospital | Efficient material transport frees up staff |
Medical robotics has ushered in a paradigm shift in the healthcare landscape. It's revolutionized surgical procedures, streamlined the delivery of essential supplies and liberated healthcare professionals to focus on patient care. Intel boasts a wide-ranging tech portfolio catering to medical robot development, encompassing surgical aids, modular systems and autonomous mobile robots. The inception of medical robots dates back to the 1980s, with the early versions primarily assisting surgeons through robotic arms [39]. However, as time has progressed, the integration of AI, computer vision and data analytics has broadened the scope of medical robots, extending their impact across various facets of the healthcare sector. Today, robots have expanded their role from the confines of the operating room to various clinical settings, where they play a crucial role in assisting healthcare professionals and elevating the quality of patient care. Beyond surgical applications, hospitals and clinics have embraced the use of robots in a broader spectrum of tasks, especially in response to the pressing need to minimize exposure to pathogens during the ongoing COVID-19 pandemic [40].
The field of medical robotics is in its early stages and has emerged as a result of technological advancements in recent decades. The systems currently in use have not been around long enough to support long-term studies, and the full scope of the potential benefits offered by medical robots remains not completely understood. Medical robotics has undergone only a few technological shifts, and the technology is rapidly evolving and expanding into new domains. Nevertheless, by examining the present market and representative research systems, informed predictions can be formulated regarding the influence of robots on the near future of healthcare [41].
In the realm of surgical robotics, there's been a shift away from autonomous or semi-autonomous actions, moving instead towards collaborative manipulation and the concept of virtual fixtures. In this context, robots serve as guidance tools, offering information and, occasionally, gentle physical guidance to assist surgeons in maintaining precision. This approach necessitates the accurate tracking of tissues within the surgical area, even as they undergo manipulation during the procedure [40]. As the development of medical robots continues into new medical domains, alternative tools may also start fulfilling roles currently occupied by robots. To remain relevant, medical robots must demonstrate a clear and substantial contribution to improved medical outcomes. Otherwise, they run the risk of being overshadowed by advancements in pharmaceuticals, tissue engineering, gene therapy and the rapid innovation of manual surgical tools like the SPIDER Surgical System by TransEnterix and the EndoStitch by Covidien. Consequently, the evolution of medical robotics should prioritize addressing real healthcare challenges, ultimately offering a tangible enhancement in the quality of life compared to other available options [42].
Robotics and automation find applications in research laboratories, streamlining the automation of laborious, repetitive and high-capacity tasks. This shift enables technicians and scientists to redirect their efforts towards more strategic responsibilities, ultimately accelerating the pace of scientific discoveries [43]. Efficient processes and risk mitigation through medical robotics offer benefits across various domains. For instance, robots can independently undertake the cleaning and preparation of patient rooms, contributing to a reduction in person-to-person interactions within infectious disease units. Furthermore, robots equipped with AI-driven medication identification software expedite the process of identifying, matching and dispensing medicines to hospital patients. With advancing technology, robots will progressively operate with greater autonomy, eventually completing specific tasks entirely independently. This evolution will free up doctors, nurses and other healthcare professionals to allocate more of their time to direct patient care [44].
Technological developments, enhancements in medical imaging and growing acceptance of laparoscopic operations and robotic aid are driving forces behind the expansion of medical robotics. According to the Robot Institute of America's definition from 1979, a robot is a multipurpose, reprogrammable manipulator with the ability to carry out a variety of preprogrammed motions [45]. Robots are usually good at activities that need them to move quickly, forcefully, precisely and continuously often with intricate articulations. Although their precise tool manipulation has proven essential in surgeries, their high cost, space restrictions and demand for considerable training remain limits. Medical robots have mostly improved surgical outcomes, decreased patient trauma and shortened hospital stays; nevertheless, it is still unclear how these devices will ultimately affect patient outcomes [40]. Tasks requiring programmed, quick, accurate, and continuous motions often with intricate articulations are best suited for robots. Despite their effectiveness in improving tool motions' accuracy and precision during surgeries, robots have drawbacks, including expensive prices, space constraints, and the need for intensive user training. The most noticeable impact of medical robots has been observed in surgeries, where they have enhanced surgical results, reduced patient trauma, and shortened hospital stays. However, ongoing research is assessing the long-term impacts of robot assistance on patient outcomes [46].
The use of robots in orthopedics offers a crucial advantage in the precise resection of bone, leading to improved alignment of implants with bone and an enhanced contact area between the implant and bone. These factors hold the potential to elevate functional outcomes and extend the longevity of implants. Orthopedic robots have primarily been employed in hip and knee procedures, with some exceptions, such as the Renaissance system used in spinal surgeries. In the initial stages, these systems required bone fixation and relied on bone screws or pins for accurate localization of the surgical site [47].
Intracerebral hemorrhage (ICH) is a subtype of stroke characterized by a high death rate and degree of disability. There are currently no proven treatments available to improve the functional recovery of ICH patients. One significant development in the advancement of less invasive surgical techniques for the treatment of ICH is robot-assisted neurosurgery [48]. The intricate and constantly developing method of robot-assisted surgery has recently been used to treat certain intracerebral hemorrhage cases. It has several clear benefits, such as accurate placement, faster surgical times and resistance to disruption, which lowers the possibility of human error and improves safety. Robot-assisted minimally invasive surgery for ICH is superior to traditional procedures, according to a meta-analysis by Xiong et al. This is because it has lower rates of rebleeding, improves neurological function, and lowers the risk of intracranial infections. Robot-assisted surgery is a crucial frontier in the development of minimally invasive techniques for this ailment because it offers the promise to provide more effective and personalized ICH treatments in the era of precision medicine [49]. Based on their primary functions and capabilities in various applications, we can categorize as in Table 2.
Robot name | Role of the health care |
Autonomous mobile robots (AMR) | AMRs are essential to healthcare because they enable telepresence, effective drug delivery and disinfection [50]. |
Service robots | By performing repetitive jobs like setting up patient rooms, keeping an eye on supplies, creating purchase orders, restocking medical supplies and overseeing the delivery of bed linens, service robots free up healthcare workers. After a task is finished, they operate autonomously and offer status updates [51]. |
Social robots | In long-term care facilities, social robots engage, monitor and encourage patients, improving their wellbeing and adherence to therapy [52]. |
Modular robots | Healthcare-related modular robots, such as robotic limbs and therapeutic exoskeletons, help people recovering from strokes and provide support to those suffering from paralysis, traumatic brain injuries or illnesses like multiple sclerosis [53]. |
Surgical assistant robots | Fast and accurate surgeries are made possible by AI-powered surgical robots, some of which may work independently and provide surgeons with console oversight [54]. |
Currently, on the market, a brand-new kind of robot called a logistics robot is intended to handle hospital logistics duties. With the help of their navigation systems, the logistics robots can transport supplies such as food and drink, medical samples, bedding and prescription drugs across the hospital. Additionally in demand are telepresence, physical therapy, nursing, ambulance and rehabilitation robots.
Emerging technology known as “soft robotics” holds promise for offering creative solutions that stiff robots are unable to satisfy. Rigid joints and the need for a fully mechanical system for actuation are absent from soft robots [55]. They can adapt to any geometry's surface without breaking [56]. They are highly sought after because of their versatility, ability to handle delicate objects, ability to mimic the human body and capacity to operate in a range of environments that rigid robots cannot [57]. Potential uses for a soft robot that can replicate biological structures in terms of compliance include prosthetic limbs, surgical instruments, catheters, stents and artificial muscles. Soft robots may also completely transform the fields of rehabilitation and physical therapy [58]. At present, the restoration of bodily functioning involves inducing motions in a muscle or joint [59]. High potential exists in this field for gel, elastomer, electroactive polymers and conductive polymer actuators [60]. During the healing phase, severe injuries from incidents at work or in cars can require a full muscle implant. Novel soft actuators are being developed to function as prosthetic muscles for individuals with facial paralysis, and ptosis, and to enhance grasping ability in neurological rehabilitation [61]. To improve cardiac inadequacies, ventriculoacous assistive devices (VADs) have already been designed to replace malfunctioning ventricles in the heart [62].
Ventricular support is provided by elastomer-based soft robotics that encircles the heart. Elastomer actuators provide quick response times, and low voltage operation, and are lightweight, thin and flexible. Polymer actuators have a lot of potential in medical applications because of these qualities. Catheter driving is currently done with polymer actuators. A little voltage is applied to the membranes on a catheter to activate this device [63]. To achieve precise drug delivery, drug-loaded nanocapsules are used to construct localized drug delivery systems. Anesthetic, anti-inflammatory, anti-thrombotic and anti-neoplastic drugs can all be delivered using this kind of device. A vital part of the drug delivery apparatus is an electroactive polymer-actuated stent, which contracts and expands in response to a voltage applied to a bodily lumen [64]. The balloons used in percutaneous transluminal coronary angioplasty (PTCA) procedures are made of conductive polymers [65]. To stop a thoracic or abdominal aortic aneurysm from rupturing, conductive polymers are also helpful for aortic aneurysm coils. Expanding and contracting the filter that captures the dislodged blood clot is one use for conductive polymers. Polymer actuators are utilized in several medical pumps, including blood pressure-controlling pumps and drainage pumps [66]. Table 3 shows the advantages and disadvantages of soft robots.
Advantages | Disadvantages |
Able to fit any shaped surface without causing harm [64]. | Usually unable to support big loads and survive a lot of actuation [67]. |
Able to disperse stresses uniformly across a wider contact surface [68]. | Provide difficulties with exact controls because of the almost infinite degree of freedom [69]. |
The materials' inherent flexibility [70]. | Soft robots require different manufacturing techniques due to their varied working conditions and environments [71]. |
Recent strides in biomedical science have forged a path for the seamless integration of robots into healthcare. Innovations in medical imaging have equipped healthcare professionals with sharper diagnostic tools, enhancing precision and personalization in patient care. Genomics has unlocked a treasure trove of insights into individual health, enabling tailored treatments and therapies. Meanwhile, pharmaceutical breakthroughs have yielded more effective treatments and drugs. These advancements, among others, have set the stage for a fruitful synergy between biomedical science and robotics, promising unprecedented advancements in healthcare delivery and outcomes [72].
Through developments in mechanical design, modeling and control, sensors, instrumentation, computing and image processing, biomedical robotics research focuses on the design, development and assessment of medical robotics systems and smart assistive robotic platforms that enhance the physical capabilities of patients and clinicians. Important study areas in this subject include tissue modeling, medical robotics, haptic interfaces, machine learning, soft robotics, robot-assisted surgery and rehabilitation, nanomaterials for biomedical implants, human augmentation, biomechanics and human-robot interaction [73].
Robots are used in a variety of settings in healthcare facilities. They have an indirect or direct impact on the lives of the patients. They even take care of elderly and disabled patients, engaging them in conversation to keep them from being bored or in pain. The primary benefits of medical robots are that robot-assisted surgeries are more efficient and error-free. The surgery's success rate is also higher [74].
The application of robots in the medical field enables efficient clinical procedures, safe surroundings for patients and medical personnel and high-quality patient care. Medical robots can help with minimally invasive surgeries, intelligent medicines, regular and individualized monitoring for patients with chronic conditions and social contact for senior persons. Furthermore, when robots relieve some of the workload, nurses and other staff members may be able to give patients more human interaction and empathy, which will enhance their long-term welfare [75]. AMRs are used to carry supplies and linens in hospitals where there is a chance of disease exposure to assist in safeguarding medical staff. Robots for cleaning and disinfection are already used in hundreds of healthcare facilities to assist in reducing pathogen exposure and hospital-acquired infections (HAIs). AMR social robots assist medical personnel in moving heavy items, such as beds or patients, by performing strenuous lifting for them [76].
Combining biomedical science with robotics offers a multitude of benefits. This synergy promises to revolutionize healthcare, leading to improved patient care, quicker and more accurate diagnostics, and highly precise treatments. Robots can undertake tasks with exceptional precision, reducing the margin for error in surgeries and medical procedures [77]. They can also streamline logistical operations in healthcare facilities, ensuring a more efficient and cost-effective system. Additionally, the integration of artificial intelligence and machine learning into robotic systems holds the potential to enhance medical data analysis and provide real-time insights, aiding medical professionals in making informed decisions [78].
However, this promising integration is not without its challenges and ethical considerations. Patient privacy is a paramount concern, particularly as robots may handle sensitive medical data. Job displacement is another issue, as the increased automation of certain healthcare tasks could potentially affect the workforce. The costs associated with implementing and maintaining robotic systems can be substantial, raising questions about affordability and resource allocation within healthcare organizations [79]. Moreover, ethical dilemmas may emerge in cases where robots are entrusted with medical decision-making. Addressing these challenges and ethical concerns is essential to harnessing the full potential of biomedical science and robotics in healthcare while ensuring the well-being and security of patients and healthcare professionals [80].
In real-world healthcare settings, the integration of biomedical science and robotics has ushered in a new era of innovation. Robotic surgery is a striking example, with systems like the da Vinci Surgical System proving their worth in minimally invasive procedures. These robots offer surgeons greater precision, dexterity and control, leading to reduced patient trauma, shorter recovery times and improved surgical outcomes [41]. Telemedicine, another notable application, has become a lifeline, especially during the COVID-19 pandemic. Robotic telepresence, when equipped with AI-driven diagnostics, enables remote patient monitoring and consultations. This not only ensures the continuity of care but also increases accessibility for patients in remote or underserved areas [81].
Case studies further illustrate the impact of this integration. For instance, the success story of a rural healthcare facility in deploying telemedicine robots highlights how they've expanded their reach and provided specialized care to patients who would otherwise face significant barriers [82]. AI-driven diagnostics have made significant strides in medical imaging, with algorithms detecting diseases like diabetic retinopathy from retinal scans, streamlining the diagnostic process. These real-world applications and case studies underscore the tangible benefits of the biomedical science and robotics nexus in healthcare, from improved patient outcomes to extended reach and accessibility [83].
In the foreseeable future, the synergy of biomedical science and robotics promises a host of exciting developments. Artificial intelligence and machine learning are poised to take center stage, offering even more precise and personalized healthcare solutions [84]. We can anticipate further integration of AI-driven diagnostics, not only in medical imaging but also in data analysis, enabling early disease detection and tailored treatment plans. The advent of more advanced surgical robots, equipped with AI-enhanced capabilities, will refine surgical procedures and expand their application to increasingly complex and delicate surgeries [85]. Additionally, the evolution of wearable medical robots for remote patient monitoring and therapy administration will enhance the delivery of healthcare services. As we move forward, the nexus of biomedical science and robotics is set to bring about a future where healthcare is more accessible, efficient and finely tuned to individual needs, improving clinical outcomes and the overall patient experience [86].
In conclusion, the purpose of this paper was to clarify the mutually beneficial relationship between robotics and biomedical science, with an emphasis on the revolutionary possibilities this relationship has for the medical field. Our main objectives were to demonstrate the synergy's inventive effects and highlight how it has the potential to completely transform patient care, medical research and healthcare delivery. Additionally, we addressed the difficulties and moral dilemmas that come up when integrating robotics into healthcare, realizing how crucial it is to resolve these problems to maximize the benefits of this partnership. The authors hoped to encourage scientists, researchers and healthcare professionals to keep pushing the envelope of what is possible in the quest for improved health and well-being by providing insights into the future of healthcare, with a focus on the role of artificial intelligence and machine learning.
The authors declare that they have not used Artificial Intelligence (AI) tools in the creation of this article.
[1] |
Saeidi H, Le HND, Opfermann JD, et al. (2019) Autonomous laparoscopic robotic suturing with a novel actuated suturing tool and 3D endoscope. 2019 International Conference on Robotics and Automation (ICRA) 2019: 1541-1547. https://doi.org/10.1109/ICRA.2019.8794306 ![]() |
[2] |
Morgan AA, Abdi J, Syed MAQ, et al. (2022) Robots in healthcare: a scoping review. Curr Robot Rep 3: 271-280. https://doi.org/10.1007/s43154-022-00095-4 ![]() |
[3] |
Alexovič M, Urban PL, Tabani H, et al. (2020) Recent advances in robotic protein sample preparation for clinical analysis and other biomedical applications. Clin Chim Acta 507: 104-116. https://doi.org/10.1016/j.cca.2020.04.015 ![]() |
[4] |
Bajwa J, Munir U, Nori A, et al. (2021) Artificial intelligence in healthcare: transforming the practice of medicine. Future Healthc J 8: e188-e194. https://doi.org/10.7861/fhj.2021-0095 ![]() |
[5] |
George EI, Brand TC, LaPorta A, et al. (2018) Origins of robotic surgery: From skepticism to standard of care. JSLS 22: e2018.00039. https://doi.org/10.4293/JSLS.2018.00039 ![]() |
[6] | Martinic G (2014) Glimpses of future battlefield medicine-the proliferation of robotic surgeons and unmanned vehicles and technologies. J Mil Veterans Health 22: 4-12. https://doi/10.3316/informit.935366340330586 |
[7] |
Hashimoto DA, Rosman G, Rus D, et al. (2018) Artificial intelligence in surgery: promises and perils. Ann Surg 268: 70-76. https://doi.org/10.1097/SLA.0000000000002693 ![]() |
[8] |
Raje S, Reddy N, Jerbi H, et al. (2021) Applications of healthcare robots in combating the COVID-19 pandemic. Appl Bionics Biomech 2021: 7099510. https://doi.org/10.1155/2021/7099510 ![]() |
[9] |
Ranzani R, Lambercy O, Metzger JC, et al. (2020) Neurocognitive robot-assisted rehabilitation of hand function: a randomized control trial on motor recovery in subacute stroke. J NeuroEngineering Rehabil 17: 1-13. https://doi.org/10.1186/s12984-020-00746-7 ![]() |
[10] | Deo N, Anjankar A (2023) Artificial intelligence with robotics in healthcare: a narrative review of its viability in India. Cureus 15: e39416. https://doi.org/10.7759/cureus.39416 |
[11] | DelveInsight Blog, How Robots Are Introducing A New Dimension To Healthcare Service Delivery (2021). Available from: https://www.delveinsight.com/blog/robotics-in-healthcare |
[12] |
Cleary K, Nguyen C (2001) State of the art in surgical robotics: clinical applications and technology challenges. Comput Aided Surg 6: 312-328. https://doi.org/10.3109/10929080109146301 ![]() |
[13] |
Khalid MY, Arif ZU, Ahmed W, et al. (2022) 4D printing: Technological developments in robotics applications. Sens Actuators Phys 343: 113670. https://doi.org/10.1016/j.sna.2022.113670 ![]() |
[14] |
Spiegel CA, Hippler M, Münchinger A, et al. (2020) 4D printing at the microscale. Adv Funct Mater 30: 1907615. https://doi.org/10.1002/adfm.201907615 ![]() |
[15] | Hann SY, Cui H, Nowicki M, et al. (2020) 4D printing soft robotics for biomedical applications. Addit Manuf 36: 101567. https://doi.org/10.1016/j.addma.2020.101567 |
[16] |
Niu D, Li D, Chen J, et al. (2022) SMA-based soft actuators with electrically responsive and photoresponsive deformations applied in soft robots. Sens Actuators Phys 341: 113516. https://doi.org/10.1016/j.sna.2022.113516 ![]() |
[17] |
Ma S, Zhang Y, Wang M, et al. (2020) Recent progress in 4D printing of stimuli-responsive polymeric materials. Sci China Technol Sci 63: 532-544. https://doi.org/10.1007/s11431-019-1443-1 ![]() |
[18] |
Zolfagharian A, Kaynak A, Kouzani A (2020) Closed-loop 4D-printed soft robots. Mater Des 188: 108411. https://doi.org/10.1016/j.matdes.2019.108411 ![]() |
[19] |
Bastola AK, Hossain M (2021) The shape – morphing performance of magnetoactive soft materials. Mater Des 211: 110172. https://doi.org/10.1016/j.matdes.2021.110172 ![]() |
[20] |
Sachyani Keneth E, Lieberman R, Rednor M, et al. (2020) Multi-material 3D printed shape memory polymer with tunable melting and glass transition temperature activated by heat or light. Polymers 12: 710. https://doi.org/10.3390/polym12030710 ![]() |
[21] | (2023) National Research Council (US) and Institute of Medicine (US) Committee on the Organizational Structure of the National Institutes of HealthEnhancing the Vitality of the National Institutes of Health: Organizational Change to Meet New Challenges. Washington (DC): National Academies Press (US), New Opportunities, New Challenges: The Changing Nature of Biomedical Science. Available from: https://www.ncbi.nlm.nih.gov/books/NBK43496/ |
[22] | Bramhe S, Pathak SS (2022) Robotic surgery: a narrative review. Cureus 14: e29179. https://doi.org/10.7759/cureus.29179 |
[23] | ETHealthworld.com, Emerging Robotic Technologies in Healthcare: Pioneering Path to Enhanced Precision-ET Health World (2023). Available from: https://health.economictimes.indiatimes.com/news/industry/emerging-robotic-technologies-in-healthcare-pioneering-path-to-enhanced-precision/102626931 |
[24] |
Yang Y, Wang H (2013) Perspectives of nanotechnology in minimally invasive therapy of breast cancer. J Healthc Eng 4: 67-86. https://doi.org/10.1260/2040-2295.4.1.67 ![]() |
[25] |
Linte CA, Yaniv Z (2014) When change happens: computer assistance and image guidance for minimally invasive therapy. Healthc Technol Lett 1: 2-5. https://doi.org/10.1049/htl.2014.0058 ![]() |
[26] |
Li J, Wang H, Cui J, et al. (2019) Magnetic micromachine using nickel nanoparticles for propelling and releasing in indirect assembly of cell-laden micromodules. Micromachines 10: 370. https://doi.org/10.3390/mi10060370 ![]() |
[27] |
Pankhurst QA, Connolly J, Jones SK, et al. (2003) Applications of magnetic nanoparticles in biomedicine. J Phys Appl Phys 36: R167. https://doi.org/10.1088/0022-3727/36/13/201 ![]() |
[28] | Alexiou C, Arnold W, Klein RJ, et al. (2000) Locoregional cancer treatment with magnetic drug targeting. Cancer Res 60: 6641-6648. |
[29] |
Molday RS, MacKenzie D (1982) Immunospecific ferromagnetic iron-dextran reagents for the labeling and magnetic separation of cells. J Immunol Methods 52: 353-367. https://doi.org/10.1016/0022-1759(82)90007-2 ![]() |
[30] |
Koleoso M, Feng X, Xue Y, et al. (2020) Micro/nanoscale magnetic robots for biomedical applications. Mater Today Bio 8: 100085. https://doi.org/10.1016/j.mtbio.2020.100085 ![]() |
[31] |
Zhang D, Gorochowski TE, Marucci L, et al. (2023) Advanced medical micro-robotics for early diagnosis and therapeutic interventions. Front Robot AI 9: 1086043. https://doi.org/10.3389/frobt.2022.1086043 ![]() |
[32] |
Athanasopoulou K, Daneva GN, Adamopoulos PG, et al. (2022) Artificial intelligence: the milestone in modern biomedical research. BioMedInformatics 2: 727-744. https://doi.org/10.3390/biomedinformatics2040049 ![]() |
[33] |
Fiorini P, Goldberg KY, Liu Y, et al. (2022) Concepts and trends in autonomy for robot-assisted surgery. Proc IEEE 110: 993-1011. https://doi.org/10.1109/JPROC.2022.3176828 ![]() |
[34] | Da Vinci Surgical System - an overview, ScienceDirect Topics. Available from: https://www.sciencedirect.com/topics/medicine-and-dentistry/da-vinci-surgical-system |
[35] | Mustafa, Lakeland Surgical & Diagnostic Center, The Xenex LightStrike Germ-Zapping Robot (2017). Available from: https://lsdc.net/lsdc-news/xenex-lightstrike-germ-zapping-robot/ |
[36] | PARO Therapeutic Robot. Available from: http://www.parorobots.com/ |
[37] | Cyberknife-an overview, ScienceDirect Topics. Available from: https://www.sciencedirect.com/topics/medicine-and-dentistry/cyberknife |
[38] | TUG Robot Mercy. Available from: https://www.mercy.net/about/mercy-technology-services/tug-robot |
[39] | Performed R in the medical field are transforming how surgeries are, Delivery SS, disinfection, et al. Intel, Robotics in Healthcare: The Future of Robots in Medicine. Available from: https://www.intel.com/content/www/us/en/healthcare-it/robotics-in-healthcare.html |
[40] | Beasley RA (2012) Medical robots: current systems and research directions. J Robot 2012: 401613. https://doi.org/10.1155/2012/401613 |
[41] | Morris B (2005) Robotic surgery: applications, limitations, and impact on surgical education. MedGenMed 7: 72. |
[42] | Gyles C (2019) Robots in medicine. Can Vet J 60: 819-820. |
[43] | Intelligent Automation Technology for Healthcare | LinkedIn. Available from: https://www.linkedin.com/pulse/intelligent-automation-technology-healthcare-derek-friend/?trk=pulse-article_more-articles_related-content-card |
[44] |
Kitsios F, Kamariotou M, Syngelakis AI, et al. (2023) Recent advances of artificial intelligence in healthcare: A systematic literature review. Appl Sci 13: 7479. https://doi.org/10.3390/app13137479 ![]() |
[45] | Robotics: Technological Advancement of India by 2020 and Beyond - Page 2 of 2 - Electronics For You (2014). Available from: https://www.electronicsforu.com/technology-trends/robotics-technological-advancement-india-2020-beyond/2 |
[46] | Research in medical robotics issuu. Available from: https://issuu.com/clinicalresearchinsider/docs/clir_2_english/s/11146942 |
[47] |
St Mart JP, Goh EL (2021) The current state of robotics in total knee arthroplasty. EFORT Open Rev 6: 270-279. https://doi.org/10.1302/2058-5241.6.200052 ![]() |
[48] |
Chen Y, Godage IS, Sengupta S, et al. (2019) MR-conditional steerable needle robot for intracerebral hemorrhage removal. Int J Comput Assist Radiol Surg 14: 105-115. https://doi.org/10.1007/s11548-018-1854-z ![]() |
[49] |
Wu Z, Chen D, Pan C, et al. (2023) Surgical robotics for intracerebral hemorrhage treatment: state of the art and future directions. Ann Biomed Eng 51: 1933-1941. https://doi.org/10.1007/s10439-023-03295-x ![]() |
[50] |
Holland J, Kingston L, McCarthy C, et al. (2021) Service robots in the healthcare sector. Robotics 10: 47. https://doi.org/10.3390/robotics10010047 ![]() |
[51] | Parul Saini, Webmedy Team, Ways Medical Robots can Enhance Healthcare (2023). Available from: https://webmedy.com/blog/ways-medical-robots-can-enhance-healthcare |
[52] |
González-González CS, Violant-Holz V, Gil-Iranzo RM (2021) Social robots in hospitals: a systematic review. Appl Sci 11: 5976. https://doi.org/10.3390/app11135976 ![]() |
[53] |
Chang WH, Kim YH (2013) Robot-assisted therapy in stroke rehabilitation. J Stroke 15: 174-181. https://doi.org/10.5853/jos.2013.15.3.174 ![]() |
[54] | The Observer, Robot surgeons provide many benefits, but how autonomous should they be?. Available from: https://www.theguardian.com/science/2023/jun/18/robot-surgeons-provide-many-benefits-but-how-autonomous-should-they-be. |
[55] |
Lee C, Kim M, Kim YJ, et al. (2017) Soft robot review. Int J Control Autom Syst 15: 3-15. https://doi.org/10.1007/s12555-016-0462-3 ![]() |
[56] |
Deimel R, Brock O (2016) A novel type of compliant and underactuated robotic hand for dexterous grasping. Int J Robot Res 35: 161-185. https://doi.org/10.1177/0278364915592961 ![]() |
[57] |
Shepherd RF, Ilievski F, Choi W, et al. (2011) Multigait soft robot. Proc Natl Acad Sci 108: 20400-20403. https://doi.org/10.1073/pnas.1116564108 ![]() |
[58] |
Carpi F, Mannini A, De Rossi D (2009) Dynamic splint-like hand orthosis for finger rehabilitation. Biomedical applications of electroactive polymer actuators. UK: John Wiley & Sons 443-461. ![]() |
[59] |
Sahrmann SA (1988) Diagnosis by the physical therapist-a prerequisite for treatment: a special communication. Phys Ther 68: 1703-1706. https://doi.org/10.1093/ptj/68.11.1703 ![]() |
[60] |
Anderson IA, Gisby TA, McKay TG, et al. (2012) Multi-functional dielectric elastomer artificial muscles for soft and smart machines. J Appl Phys 112: 041101. https://doi.org/10.1063/1.4740023 ![]() |
[61] |
Nilsson M, Ingvast J, Wikander J, et al. (2012) The Soft Extra Muscle system for improving the grasping capability in neurological rehabilitation. 2012 IEEE-EMBS Conference on Biomedical Engineering and Sciences 2012: 412-417. ![]() |
[62] |
Tanaka Y, Sato K, Shimizu T, et al. (2007) A micro-spherical heart pump powered by cultured cardiomyocytes. Lab Chip 7: 207-212. https://doi.org/10.1039/B612082B ![]() |
[63] |
Fang BK, Ju MS, Lin CCK (2007) A new approach to develop ionic polymer–metal composites (IPMC) actuator: Fabrication and control for active catheter systems. Sens Actuators Phys 137: 321-329. https://doi.org/10.1016/j.sna.2007.03.024 ![]() |
[64] |
Ashuri T, Armani A, Jalilzadeh Hamidi R, et al. (2020) Biomedical soft robots: current status and perspective. Biomed Eng Lett 10: 369-385. https://doi.org/10.1007/s13534-020-00157-6 ![]() |
[65] |
Kanaan AF, Pinho AC, Piedade AP (2021) Electroactive polymers obtained by conventional and non-conventional technologies. Polymers 13: 2713. https://doi.org/10.3390/polym13162713 ![]() |
[66] | Weber J Medical device with electroactive polymer powered by photovoltaic cell (2014). U.S. Patent: US8744568B2 |
[67] |
Lipson H (2014) Challenges and opportunities for design, simulation, and fabrication of soft robots. Soft Robot 1: 21-27. https://doi.org/10.1089/soro.2013.0007 ![]() |
[68] |
Majidi C (2014) Soft robotics: a perspective—current trends and prospects for the future. Soft Robot 1: 5-11. http://doi.org/10.1089/soro.2013.0001 ![]() |
[69] |
Wang H, Totaro M, Beccai L (2018) Toward perceptive soft robots: progress and challenges. Adv Sci 5: 1800541. https://doi.org/10.1002/advs.201800541 ![]() |
[70] |
Rus D, Tolley MT (2015) Design, fabrication and control of soft robots. Nature 521: 467-475. https://doi.org/10.1038/nature14543 ![]() |
[71] |
Yang D, Mosadegh B, Ainla A, et al. (2015) Buckling of elastomeric beams enables actuation of soft machines. Adv Mater 27: 6323-6327. https://doi.org/10.1002/adma.201503188 ![]() |
[72] |
Armitage C (2019) Small advances amount to big changes in biomedical sciences. Nature 569: S5-S5. https://doi.org/10.1038/d41586-019-01437-7 ![]() |
[73] | Biomedical Robotics, Wallace H. Coulter Department of Biomedical Engineering. Available from: https://bme.gatech.edu/bme/areas/biomedical-robotics |
[74] | DelveInsight, DelveInsight Business Research, Robots In Healthcare, Benefit, Disadvantages and Future of Medical Robot (2021). Available from: https://www.delveinsight.com/blog/robotics-in-healthcare |
[75] |
Denecke K, Baudoin CR (2022) A review of artificial intelligence and robotics in transformed health ecosystems. Front Med 9: 795957. https://doi.org/10.3389/fmed.2022.795957 ![]() |
[76] | Huo Y (2022) Benefits of robotics technology in health care. Int J Swarm Intell Evol Comput 11: 1-2. https://doi.org/10.35248/2090-4908.22.11.252 |
[77] |
Wee IJY, Kuo LJ, Ngu JCY (2020) A systematic review of the true benefit of robotic surgery: ergonomics. Int J Med Robot Comput Assist Surg 16: e2113. https://doi.org/10.1002/rcs.2113 ![]() |
[78] |
Davenport T, Kalakota R (2019) The potential for artificial intelligence in healthcare. Future Healthc J 6: 94-98. https://doi.org/10.7861/futurehosp.6-2-94 ![]() |
[79] | Farhud DD, Zokaei S (2021) Ethical issues of artificial intelligence in medicine and healthcare. Iran J Public Health 50: i-v. https://doi.org/10.18502/ijph.v50i11.7600 |
[80] |
Soares A, Piçarra N, Giger JC, et al. (2023) Ethics 4.0: Ethical dilemmas in healthcare mediated by social robots. Int J Soc Robot 15: 807-823. https://doi.org/10.1007/s12369-023-00983-5 ![]() |
[81] | Tukur M, Saad G, AlShagathrh FM, et al. (2023) Telehealth interventions during COVID-19 pandemic: a scoping review of applications, challenges, privacy and security issues. BMJ Health Care Inform 30: e100676. https://doi.org/10.1136/bmjhci-2022-100676 |
[82] |
Haleem A, Javaid M, Singh RP, et al. (2021) Telemedicine for healthcare: capabilities, features, barriers, and applications. Sens Int 2: 100117. https://doi.org/10.1016/j.sintl.2021.100117 ![]() |
[83] |
Padhy SK, Takkar B, Chawla R, et al. (2019) Artificial intelligence in diabetic retinopathy: A natural step to the future. Indian J Ophthalmol 67: 1004-1009. https://doi.org/10.4103/ijo.IJO_1989_18 ![]() |
[84] |
Ahuja AS (2019) The impact of artificial intelligence in medicine on the future role of the physician. PeerJ 7: e7702. https://doi.org/10.7717/peerj.7702 ![]() |
[85] |
Cepolina F, Razzoli RP (2022) An introductory review of robotically assisted surgical systems. Int J Med Robot 18: e2409. https://doi.org/10.1002/rcs.2409 ![]() |
[86] | Liao Y, Thompson C, Peterson S, et al. (2019) The future of wearable technologies and remote monitoring in health care. ASCO Educational Book 39: 115-121. https://doi.org/10.1200/EDBK_238919 |
1. | Induni N. Weerarathna, Praveen Kumar, Anurag Luharia, Gaurav Mishra, Engineering with Biomedical Sciences Changing the Horizon of Healthcare-A Review, 2024, 15, 2165-5979, 10.1080/21655979.2024.2401269 |
Robot name | Application(s) | Key features | Benefits |
da Vinci Surgical Robot [34]. | Surgical procedures | 3D high-definition vision, precise incisions | Enhanced control, faster patient healing |
Xenex Germ-Zapping Robot [35] | Disinfection of hospital rooms | Pulsed UV rays, kill infectious bacteria | Reduces hospital-acquired infections |
PARO Therapeutic Robot [36] | Improving the quality of life during recovery | Interactive, resembles an animal | Reduces stress, provides comfort |
CyberKnife [37] | Radiation therapy for tumors | Sub-millimeter precision, non-invasive | Precise tumor targeting, minimal radiation |
TUG [38] | Hospital material transportation | Autonomous, sensor-equipped, navigates hospital | Efficient material transport frees up staff |
Robot name | Role of the health care |
Autonomous mobile robots (AMR) | AMRs are essential to healthcare because they enable telepresence, effective drug delivery and disinfection [50]. |
Service robots | By performing repetitive jobs like setting up patient rooms, keeping an eye on supplies, creating purchase orders, restocking medical supplies and overseeing the delivery of bed linens, service robots free up healthcare workers. After a task is finished, they operate autonomously and offer status updates [51]. |
Social robots | In long-term care facilities, social robots engage, monitor and encourage patients, improving their wellbeing and adherence to therapy [52]. |
Modular robots | Healthcare-related modular robots, such as robotic limbs and therapeutic exoskeletons, help people recovering from strokes and provide support to those suffering from paralysis, traumatic brain injuries or illnesses like multiple sclerosis [53]. |
Surgical assistant robots | Fast and accurate surgeries are made possible by AI-powered surgical robots, some of which may work independently and provide surgeons with console oversight [54]. |
Advantages | Disadvantages |
Able to fit any shaped surface without causing harm [64]. | Usually unable to support big loads and survive a lot of actuation [67]. |
Able to disperse stresses uniformly across a wider contact surface [68]. | Provide difficulties with exact controls because of the almost infinite degree of freedom [69]. |
The materials' inherent flexibility [70]. | Soft robots require different manufacturing techniques due to their varied working conditions and environments [71]. |
Robot name | Application(s) | Key features | Benefits |
da Vinci Surgical Robot [34]. | Surgical procedures | 3D high-definition vision, precise incisions | Enhanced control, faster patient healing |
Xenex Germ-Zapping Robot [35] | Disinfection of hospital rooms | Pulsed UV rays, kill infectious bacteria | Reduces hospital-acquired infections |
PARO Therapeutic Robot [36] | Improving the quality of life during recovery | Interactive, resembles an animal | Reduces stress, provides comfort |
CyberKnife [37] | Radiation therapy for tumors | Sub-millimeter precision, non-invasive | Precise tumor targeting, minimal radiation |
TUG [38] | Hospital material transportation | Autonomous, sensor-equipped, navigates hospital | Efficient material transport frees up staff |
Robot name | Role of the health care |
Autonomous mobile robots (AMR) | AMRs are essential to healthcare because they enable telepresence, effective drug delivery and disinfection [50]. |
Service robots | By performing repetitive jobs like setting up patient rooms, keeping an eye on supplies, creating purchase orders, restocking medical supplies and overseeing the delivery of bed linens, service robots free up healthcare workers. After a task is finished, they operate autonomously and offer status updates [51]. |
Social robots | In long-term care facilities, social robots engage, monitor and encourage patients, improving their wellbeing and adherence to therapy [52]. |
Modular robots | Healthcare-related modular robots, such as robotic limbs and therapeutic exoskeletons, help people recovering from strokes and provide support to those suffering from paralysis, traumatic brain injuries or illnesses like multiple sclerosis [53]. |
Surgical assistant robots | Fast and accurate surgeries are made possible by AI-powered surgical robots, some of which may work independently and provide surgeons with console oversight [54]. |
Advantages | Disadvantages |
Able to fit any shaped surface without causing harm [64]. | Usually unable to support big loads and survive a lot of actuation [67]. |
Able to disperse stresses uniformly across a wider contact surface [68]. | Provide difficulties with exact controls because of the almost infinite degree of freedom [69]. |
The materials' inherent flexibility [70]. | Soft robots require different manufacturing techniques due to their varied working conditions and environments [71]. |