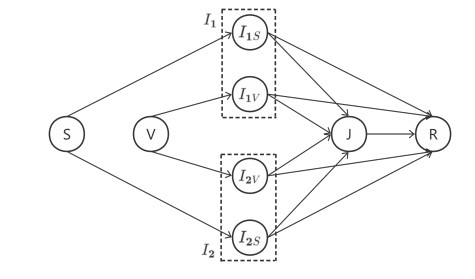
Coinfection is the process of an infection of a single host with two or more pathogen variants or with two or more distinct pathogen species, which often threatens public health and the stability of economies. In this paper, we propose a novel two-strain epidemic model characterizing the co-evolution of coinfection and voluntary vaccination strategies. In the framework of evolutionary vaccination, we design two game rules, the individual-based risk assessment (IB-RA) updated rule, and the strategy-based risk assessment (SB-RA) updated rule, to update the vaccination policy. Through detailed numerical analysis, we find that increasing the vaccine effectiveness and decreasing the transmission rate effectively suppress the disease prevalence, and moreover, the outcome of the SB-RA updated rule is more encouraging than those results of the IB-RA rule for curbing the disease transmission. Coinfection complicates the effects of the transmission rate of each strain on the final epidemic sizes.
Citation: Kelu Li, Junyuan Yang, Xuezhi Li. Effects of co-infection on vaccination behavior and disease propagation[J]. Mathematical Biosciences and Engineering, 2022, 19(10): 10022-10036. doi: 10.3934/mbe.2022468
[1] | Anthony Morciglio, R. K. P. Zia, James M. Hyman, Yi Jiang . Understanding the oscillations of an epidemic due to vaccine hesitancy. Mathematical Biosciences and Engineering, 2024, 21(8): 6829-6846. doi: 10.3934/mbe.2024299 |
[2] | Abba B. Gumel, C. Connell McCluskey, James Watmough . An sveir model for assessing potential impact of an imperfect anti-SARS vaccine. Mathematical Biosciences and Engineering, 2006, 3(3): 485-512. doi: 10.3934/mbe.2006.3.485 |
[3] | Majid Jaberi-Douraki, Seyed M. Moghadas . Optimal control of vaccination dynamics during an influenza epidemic. Mathematical Biosciences and Engineering, 2014, 11(5): 1045-1063. doi: 10.3934/mbe.2014.11.1045 |
[4] | Manoj Kumar, Syed Abbas, Abdessamad Tridane . Optimal control and stability analysis of an age-structured SEIRV model with imperfect vaccination. Mathematical Biosciences and Engineering, 2023, 20(8): 14438-14463. doi: 10.3934/mbe.2023646 |
[5] | Kai Zhang, Xinwei Wang, Hua Liu, Yunpeng Ji, Qiuwei Pan, Yumei Wei, Ming Ma . Mathematical analysis of a human papillomavirus transmission model with vaccination and screening. Mathematical Biosciences and Engineering, 2020, 17(5): 5449-5476. doi: 10.3934/mbe.2020294 |
[6] | Nawei Chen, Shenglong Chen, Xiaoyu Li, Zhiming Li . Modelling and analysis of the HIV/AIDS epidemic with fast and slow asymptomatic infections in China from 2008 to 2021. Mathematical Biosciences and Engineering, 2023, 20(12): 20770-20794. doi: 10.3934/mbe.2023919 |
[7] | Eunha Shim, Beth Kochin, Alison Galvani . Insights from epidemiological game theory into gender-specific vaccination against rubella. Mathematical Biosciences and Engineering, 2009, 6(4): 839-854. doi: 10.3934/mbe.2009.6.839 |
[8] | Linda J. S. Allen, P. van den Driessche . Stochastic epidemic models with a backward bifurcation. Mathematical Biosciences and Engineering, 2006, 3(3): 445-458. doi: 10.3934/mbe.2006.3.445 |
[9] | Olivia Prosper, Omar Saucedo, Doria Thompson, Griselle Torres-Garcia, Xiaohong Wang, Carlos Castillo-Chavez . Modeling control strategies for concurrent epidemics of seasonal and pandemic H1N1 influenza. Mathematical Biosciences and Engineering, 2011, 8(1): 141-170. doi: 10.3934/mbe.2011.8.141 |
[10] | Zhiping Liu, Zhen Jin, Junyuan Yang, Juan Zhang . The backward bifurcation of an age-structured cholera transmission model with saturation incidence. Mathematical Biosciences and Engineering, 2022, 19(12): 12427-12447. doi: 10.3934/mbe.2022580 |
Coinfection is the process of an infection of a single host with two or more pathogen variants or with two or more distinct pathogen species, which often threatens public health and the stability of economies. In this paper, we propose a novel two-strain epidemic model characterizing the co-evolution of coinfection and voluntary vaccination strategies. In the framework of evolutionary vaccination, we design two game rules, the individual-based risk assessment (IB-RA) updated rule, and the strategy-based risk assessment (SB-RA) updated rule, to update the vaccination policy. Through detailed numerical analysis, we find that increasing the vaccine effectiveness and decreasing the transmission rate effectively suppress the disease prevalence, and moreover, the outcome of the SB-RA updated rule is more encouraging than those results of the IB-RA rule for curbing the disease transmission. Coinfection complicates the effects of the transmission rate of each strain on the final epidemic sizes.
Many infectious diseases, such as novel coronavirus pneumonia (COVID-19), Ebola and monkeypox, are caused by pathogen variants that can be spread among people and which endanger human life and threaten national security. With the increase of the convenience of modern logistics, some extinct diseases recur, and some re-surging epidemics emerge. Therefore, how to effectively curtail the disease spread has been a major concern of the whole modern society.
Vaccines can boost the production of competent antibodies by stimulating the immune system and lower the morbidity and mortality rates. Hence, vaccination plays a crucial role in preventing and controlling outbreaks of the diseases. Specific immunity induced by vaccination effectively fights against the invasion of viruses, bacteria and other pathogens. With the advancement of science and technology, more and more vaccines have been developed to effectively resist infectious diseases and radically eradicate the diseases, such as smallpox, diphtheria, varicella, etc.
Facing an emerging disease, it is sometimes possible to take a voluntary vaccination strategy to curb its prevalence. In this case, everyone is inclined to either get vaccinated or not. Therefore, the onset of an epidemic depends not only on the transmission mechanisms of an infectious disease, but also on the choices of potential vaccine recipients. For a bounded rationality, they will evaluate the payoff from vaccination, including the side effects of vaccines and the potential risk if they do not take the vaccine, to decide to get vaccinated or not [1]. A myriad of studies has shown that voluntary vaccination policies cannot radically eliminate the outbreak of an epidemic because of the behavior-disease-vaccination interactions, which may form a feedback loop [2,3,4,5]. When a disease breaks out and causes lots of deaths, most people would like to receive a vaccination for preventing the disease infection. Once the vaccination coverage reaches a certain level and produces herd immunity, the disease will be effectively controlled. As a consequence, the non-vaccinated have no motivation to get vaccinated and lead to the resurgence of the epidemic.
To further study how individuals' behaviors influence infectious diseases, researchers have employed the evolutionary game theory to set up co-evolutionary models of infectious diseases and human behaviors [6,7,8,9,10,11,12,13,14]. Indeed, such models provide useful tools to understand the effects of human behaviors on the development of an epidemic. For instance, Kuge and Tanimoto simultaneously proposed a susceptible-infected-recovered (SIR) epidemic model to consider the effects of innate immunity from the treatment and specific immunity from vaccination [15]. Alam et al. adopted an SIR epidemic model to find that imperfect immunization attenuates the rate of virus transmission and gives a second protection to the vaccinated even though they do not receive perfect vaccine protection [16]. Later, Alam coupled the vaccination evolutionary game and an SEIR/V model to study the effects of two control strategies: isolation and quarantine. It was found that the effects of isolation are better than those of quarantine [17]. Arefin et al, based on evolutionary game theory, proposed a two-strain model to evaluate the trade-off of the evolutionary mutation [18]. Kabir et al. combined the SIR model with unawareness and awareness to study the effect of information dissemination on preventing the transmission of infectious diseases [19]. Huang et al. proposed a mathematical model based on complex networks to investigate the co-evolution of epidemic transmission and individuals' behaviors [20].
Most infectious diseases are caused by a single pathogenic strain, but in reality, the disease may be caused by different variants of the pathogen, such as the variants of COVID-19 represented by more than 5 serotypes: alpha, beta, gamma, delta and omicron [21]. Influenza can be categorized by three serotypes: A, B and C. Such multi-strains interact with each other, and some trade-off mechanisms have been identified that result in the coexistence of pathogens in epidemic models. Coinfection is one such trade-off mechanism, and it is defined as a process of the single host infected by two or more pathogenic strains. Evidences has shown that coinfection is common in HIV (human immunodeficiency virus) infection, with combined infections with TB, hepatitis and malaria. Most importantly, co-infection by variants of a pathogen often leads to more mortality and brings a huge challenge to public health. Therefore, it is necessary to create appropriate approaches to evaluate the effects of coinfection. Many researchers have used infectious disease models to analyze the impacts of coinfection on epidemic spread. Gao et al. showed that coinfection leads to the coexistence of muti-strain mechanisms [22]. In [23], Elaiw et al. proposed a single-host SARS-CoV-2/HIV co-infection model, and they showed that weak CD4+ T cell immunity in co-infected patients can cause severe SARS-CoV-2 infections from a cellular perspective. Hezam et al. proposed the co-infection phenomenon between COVID-19 and cholera to evaluate the combinations of the best policies for curbing such two diseases [24]. Newman and Ferrario studied a co-infection model on a contact network and showed that the results on scale-free networks are in agreement with those results on large wired networks [25]. Osman and Makinde built a listeriosis and anthrax coinfection model, and they studied the effects on each strain and coinfected strain by varying exposure rates [26]. Martcheva and Pilyugin used an age-structured epidemic model to characterize the coinfection phenomena [27]. Through detailed analysis, they showed that coinfection produces rich dynamics, including backward bifurcation and Hopf bifurcation. Li et al. proposed a multiscale system taking into account for interplays of within and between systems [28]. On an intra-host scale, the two strains compete with each other, and the one with a larger basic reproduction number dominates. On a population scale, co-infection results in the coexistence of the two strains. Sanz et al. proposed a two-strain epidemic model with coinfection on complex networks [29].
In most of the reviewed existing epidemic models with coinfection, they have neglected the effects of individual vaccination behaviors on the evolution of the epidemic infection. In this paper, we couple the evolutionary vaccination behaviors and epidemic models to examine the effect of the co-infection mechanism on the development of an epidemic. The aim of this paper is to explore the co-evolution of flu infection and uptaking vaccination strategies of individuals.
This paper is organized as follows: In Section 2, we design a game theoretical-compartmental epidemic model with coinfection. In Section 3, we study the dynamics of the model through a detailed analysis. Finally, in Section 4, we end with a brief discussion.
We assume that an outbreak of an epidemic happens at two distinct time scales. One is the local time scale (tlocal), and the other is the global time scale (tglobal). On the first time scale, the period goes through the onset to the end of the epidemic. On the last time scale, individuals taking vaccines dynamically evolves along with periodical outbreaks of the epidemic.
Similar to [18], we classify the total population into eight categories: S(tlocal) is the proportion of susceptible individuals at time t, V(tlocal) is the proportion of vaccinated individuals at time tlocal, I1S(tlocal) is the proportion of susceptible individuals infected by strain one at time tlocal, I1V(tlocal) is the proportion of vaccinated individuals infected by strain one at time tlocal, I2S(tlocal) is the proportion of susceptible individuals infected by strain two at time tlocal, I2V(tlocal) is the proportion of vaccinated individuals infected by strain two at time tlocal, J(tlocal) is the proportion of individuals infected by strain one and strain two at time tlocal, and R(tlocal) is the proportion of recovered individuals at time tlocal.
We assume that individuals initially infected by strain 1 can be re-infected by strain 2, and they move to the co-infection class (J(tlocal)). Similarly, individuals initially infected by strain 2 can be re-infected by strain 1, and they move to the co-infection class (J(tlocal)). Since the vaccine is not fully effective, we assume that its efficacy values for strain 1 and strain 2 are the coefficients e1 and e2. Susceptibles (S(tlocal)), vaccinated individuals (V(tlocal)), susceptibles infected by strain 2 (I2S(tlocal)) and vaccinated individuals infected by strain 2 (I2V(tlocal)) can become individuals infected by strain 1 at transmission rate β1. Susceptibles (S(tlocal)), vaccinated individuals (V(tlocal)), susceptibles infected by strain 1 (I1S(tlocal)) and vaccinated individuals infected by strain 1 (I1V(tlocal)) can become ones infected by strain 2 at a transmission rate β2. Individuals infected by strain 1 (I1S(tlocal), I1V(tlocal)) recover at rate γ1, and then they move to the recovery class (R(tlocal)). Individuals infected by strain 2 (I2S(tlocal), I2V(tlocal)) recover at a rate of γ2, and then they move to the recovery class (R(tlocal)). It is assumed that co-infected individuals (J(tlocal)) take a longer time to recover than individuals infected by one of the strains alone (γ3<min{γ1,γ2}). A schematic diagram of our model is presented in Figure 1. The framework of a two-strain epidemic model with coinfection takes the following form.
dS(tlocal)dt=−β1S(tlocal)(I1(tlocal)+J(tlocal))−β2S(tlocal)(I2(tlocal)+J(tlocal)),dV(tlocal)dt=−β1(V(tlocal)−e1V(x,0))(I1(tlocal)+J(tlocal))−β2(V(tlocal)−e2V(0))(I2(tlocal)+J(tlocal)),dI1S(tlocal)dt=β1S(tlocal)(I1(tlocal)+J(tlocal))−β2I1S(tlocal)(I2(tlocal)+J(tlocal))−γ1I1S(tlocal),dI1V(tlocal)dt=β1(V(tlocal)−e1V(0))(I1(tlocal)+J(tlocal))−β2I1V(tlocal)(I2(tlocal)+J(tlocal))−γ1I1V(tlocal),dI2S(tlocal)dt=β2S(tlocal)(I2(tlocal)+J(tlocal))−β1I2S(tlocal)(I1(tlocal)+J(tlocal))−γ2I2S(tlocal),dI2V(tlocal)dt=β2(V(tlocal)−e2V(0))(I2(tlocal)+J(tlocal))−β1I2V(tlocal)(I1(tlocal)+J(tlocal))−γ2I2V(tlocal),dJ(tlocal)dt=β2I1S(tlocal)(I2(tlocal)+J(tlocal))+β2I1V(tlocal)(I2(tlocal)+J(tlocal))+β1I2S(tlocal)(I1(tlocal)+J(tlocal))+β1I2V(tlocal)(I1(tlocal)+J(tlocal))−γ3J(tlocal),dR(tlocal)dt=γ1I1S(tlocal)+γ1I1V(tlocal)+γ2I2S(tlocal)+γ2I2V(tlocal)+γ3J(tlocal). | (2.1) |
Define
I1(tlocal)=I1S(tlocal)+I1V(tlocal),I2(tlocal)=I2S(tlocal)+I2V(tlocal). |
To satisfy the biological significance, all parameters are positive, and they are chosen from Table 1. The total population is given by
S(tlocal)+V(tlocal)+I1(tlocal)+I2(tlocal)+J(tlocal)+R(tlocal)=1. |
Parameters | Description | Value | Source |
β1 | The transmission rate for strain 1 | 0.5 day−1 | Assumed |
β2 | The transmission rate for strain 2 | 0.7 day−1 | Assumed |
γ1 | Recovery rate after infection by strain 1 | 1/11 day−1 | [30] |
γ2 | Recovery rate after infection by strain 2 | 1/9 day−1 | [30] |
γ3 | Recovery rate after infection by strain 1 and strain 2 | 1/14 day−1 | Assumed |
e1 | Effectiveness of vaccination at protecting against strain 1 | 0.61 | [31] |
e2 | Effectiveness of vaccination at protecting against strain 2 | 0.33 | [31] |
The initial values satisfy
S(0)=1−x>0,V(0)=x≥0,I1(0)=0,I2(0)=0,J(0)=0,R(0)=0. |
In fact, in model 2.1, strain 1 can be considered as influenza A (H1N1) virus, and strain 2 is associated with influenza A (H3N2) virus. As we know, an influenza quadrivalent vaccine can protect against four different influenza viruses. In addition, all people over 6 months of age need to get an annual flu shot for slowing down influenza transmission.
The final epidemic size is the proportion of the population that is infected at the end of the epidemic. Since all the infected individuals finally become the recovered ones at the end of the disease, the proportion of recovered individuals at the final stage of the epidemic ((tlocal=∞)) is the final size of the epidemic, denoted by R(∞). Similarly, we have the following.
● HV is a collective term referring to individuals who choose to get vaccinated before the outbreak, but they are not infected by any strain during the epidemic, represented by HV(∞).
● I1V is a collective term referring to individuals who choose to get vaccinated before the outbreak, and they are infected by strain 1 during the epidemic, represented by I1V(∞).
● I2V is a collective term referring to individuals who choose to get vaccinated before the outbreak, and they are infected by strain 2 during the epidemic, represented by I2V(∞).
● IV is another collective term referring to individuals who choose to get vaccinated before the outbreak, and they are co-infected by strains 1 and 2 during the epidemic, represented by IV(∞).
● SFR is a collective term referring to individuals who are not vaccinated before the outbreak, and they are not infected by any strain during the epidemic, represented by SFR(∞).
● FFR1 is a collective term referring to individuals who are not vaccinated before the outbreak, but they are infected by strain 1 during the epidemic, represented by FFR1(∞).
● FFR2 is a collective term referring to individuals who are not vaccinated before the outbreak, but they are infected by strain 2 during the epidemic, represented by FFR2(∞).
● FFR is a collective term referring to individuals who are not vaccinated before the outbreak, but they are infected by both strain 1 and strain 2 during the epidemic, represented by FFR(∞).
Based on the next generation matrix [32], the reproduction number associated with each strain is defined by
Ri=βi(1−eix)γi,i=1,2. |
Hence, the basic reproduction number is
R0=max{R1,R2}. |
When R0<1, system (2.1) has only a disease-free equilibrium (DFE), which means that neither strain colonizes the host, and the disease becomes extinct. When R1>1,R2<1, it follows from the competitive exclusion principle that strain 1 is dominant, and strain 2 is eliminated. Similarly, when R2>1,R1<1, strain 2 is dominant, and strain 1 is eradicated. When R1>1,R2>1, the two strains coexist, and the two strains both invade the host.
Although the extermination of smallpox served as a perfect example of completely eliminating a disease by vaccines, there are still many diseases that cannot be radically eradicated in a region. There is a paradox in immuno-epidemiology: While vaccination leads to herd immunity, individuals who have not been vaccinated are indirectly protected against the disease with no incentive to take vaccines, and they don't pay any costs. These are called free riders. From a personal payment perspective, free riders are not required to pay any costs associated with the epidemic.
Next, we analyze the payoffs of different types of people. The cost of the vaccinated from direct costs and side-effects is denoted by CV. The payoff of potential infection from the risk of death and suffering is denoted by CI. Thus, the relative payoff of vaccination is Cr=CV/CI. We assume that the payoff of the vaccinated is less than the that of the non-vaccinated, and 0<Cr≤1. Here, we divide the relative payoffs of the population into eight categories (see Table 2). We can obtain the average social payoff π, the average vaccinated payoff ⟨πV⟩, and the average unvaccinated payoff ⟨πNV⟩.
π=−CrHV(∞)−(Cr+1)(I1V(∞)+I2V(∞))−(Cr+2)IV(∞)−(FFR1(∞)+FFR2(∞)+2FFR(∞)), | (2.2) |
⟨πV⟩=−(CrHV(∞)+(Cr+1)(I1V(∞)+I2V(∞))+(Cr+2)IV(∞))/x, | (2.3) |
⟨πNV⟩=−(FFR1(∞)+FFR2(∞)+2FFR(∞))/(1−x). | (2.4) |
State/Strategy | Healthy | Infected with strain 1 | Infected with strain 2 | Coinfected with strain 1 and strain 2 |
Vaccination (V) | πHV=−Cr | πI1V=−Cr−1 | πI2V=−Cr−1 | πIV=−Cr−2 |
Non-vaccination (NV) | πSFR=0 | πFFR1=−1 | πFFR2=−1 | πFFR=−2 |
Unlike adopting static game-theoretic strategies, we assume that the information about the epidemic is limited, which implies that at the end of each cycle of the epidemic, individuals update their strategies for the next period depending on their neighbors' payoffs. For the sake of convenience, we employ the individual-based risk assessment (IB-RA) strategy proposed by Fu et al. [33] and the strategy-based risk assessment (SB-RA) strategy proposed by Fukuda et al. [34] to derive the evolutionary game-theoretical model.
1) Individual-based risk assessment (IB-RA) model
To explore the role played by individual imitative behavior and group structure in vaccination, Fu et al. [33] proposed an evolutionary game approach. Individual i randomly selects one of his or her neighbours j to imitate, and he or her would like to imitate one who acquired higher benefits in the previous epidemic course. The probability of individual i adopting the strategy of individual j is assumed to be given in the Fermi type [26,27,28,29], which takes the form of
P(Si←Sj)=11+exp[−(πj−πi)/κ], | (2.5) |
where Si denotes the strategy adopted by individual i. πi denotes the payoff by individual i during the previous epidemic. κ denotes selection intensity. The larger the value of κ, the more likely the individual is to switch his behaviors. For example, the probability that a healthy vaccinator (HV) will imitate a successful free-rider (SFR) is formulated by
P(HV←SFR)=11+exp[−(0−(−Cr))/κ]. |
Other cases are shown in the appendix.
2) Strategy-based risk assessment (SB-RA) model
Fukuda et al. modified the uptaken IB-RA rule from the payoff of an individual to an average one. The transfer probability for individual i is taken in the form of
P(Si←Sj)=11+exp[−(⟨πj⟩−πi)/κ], | (2.6) |
where ⟨πj⟩ denotes the average payoff for individuals who adopt strategy Sj. For example, the probability that a healthy vaccinator (HV) imitates non-vaccinators is
P(HV←NV)=11+exp[−(⟨πNV⟩−(−Cr))/κ]. |
Similarly, other cases are shown in the appendix.
The vaccination dynamics corresponding to different imitative behaviors can be derived from the imitation probabilities mentioned above. The global dynamics of an individual behavior in the IB-RA framework at the global time scale (tglobal) is given by
dxdtglobal=HV(∞)⋅SFR(∞)⋅(P(SFR←HV)−P(HV←SFR))+HV(∞)⋅FFR1(∞)⋅(P(FFR1←HV)−P(HV←FFR1))+HV(∞)⋅FFR2(∞)⋅(P(FFR2←HV)−P(HV←FFR2))+HV(∞)⋅FFR(∞)⋅(P(FFR←HV)−P(HV←FFR))+I1V(∞)⋅SFR(∞)⋅(P(SFR←I1V)−P(I1V←SFR))+I1V(∞)⋅FFR1(∞)⋅(P(FFR1←I1V)−P(I1V←FFR1))+I1V(∞)⋅FFR2(∞)⋅(P(FFR2←I1V)−P(I1V←FFR2))+I1V(∞)⋅FFR(∞)⋅(P(FFR←I1V)−P(I1V←FFR))+I2V(∞)⋅SFR(∞)⋅(P(SFR←I2V)−P(I2V←SFR))+I2V(∞)⋅FFR1(∞)⋅(P(FFR1←I2V)−P(I2V←FFR1))+I2V(∞)⋅FFR2(∞)⋅(P(FFR2←I2V)−P(I2V←FFR2))+I2V(∞)⋅FFR(∞)⋅(P(FFR←I2V)−P(I2V←FFR))+IV(∞)⋅SFR(∞)⋅(P(SFR←IV)−P(IV←SFR))+IV(∞)⋅FFR1(∞)⋅(P(FFR1←IV)−P(IV←FFR1))+IV(∞)⋅FFR2(∞)⋅(P(FFR2←IV)−P(IV←FFR2))+IV(∞)⋅FFR(∞)⋅(P(FFR←IV)−P(IV←FFR)). | (2.7) |
The global dynamics of individual behavior in the SB-RA framework at the global time scale (tglobal) is taken in the form of
dxdtglobal=SFR(∞)⋅V(∞)⋅P(SFR←V)+FFR1(∞)⋅V(∞)⋅P(FFR1←V)+FFR2(∞)⋅V(∞)⋅P(FFR2←V)+FFR(∞)⋅V(∞)⋅P(FFR←V)−HV(∞)⋅NV(∞)⋅P(HV←NV)−I1V(∞)⋅NV(∞)⋅P(I1V←NV)−I2V(∞)⋅NV(∞)⋅P(I2V←NV)−IV(∞)⋅NV(∞)⋅P(IV←NV). | (2.8) |
Due to the complexity of the system, we have conducted numerical simulations to show the dynamics of the model. We focus on how the parameters affect the dynamics. Similar to [18], we first investigated the influence of vaccine effectiveness on the game-theoretic epidemic system in the frameworks of the IB-RA and SB-RA rules. Meanwhile, we investigated the effects of the transmission rate of each strain on the evolutionary game system by adopting such two rules.
The top and bottom panels of Figure 2 respectively show the influence of e1 and e2 on the reproduction number of Strain 1 (R1), the reproduction number of Strain 2 (R2), final epidemic size (FES), vaccination coverage (VC) and social average payment (ASP) in the frameworks of the IB-RA and SB-RA rules. In Figure 2, the horizontal coordinate represents the effectiveness of the vaccine against Strain 1 and the vertical coordinate indicates the effectiveness of the vaccine against Strain 2. First, we have noted that there exists a triangular region in the lower left corners of subplots of Figure 2. In such regions, the efficacy of vaccines is not high enough to curtail the two strains, a large number of people will be infected, and fewer people will take vaccines. As a consequence, it will cause a huge socioeconomic burden. With the increase of the vaccine effectiveness against Strain 1, the reproduction number associated with strain 1 is monotonically decreasing. Similarly, the reproduction number with respect to strain 2 monotonically decreases as e2 increases (see Figure 2b-*). This phenomenon suggests that improving the efficacy of immunity from vaccines is in favor of controlling the disease spread. From Figure 2c-*, 2d-* and 2e-*, we have concluded that the efficacies of vaccines associated with the two strains are equal and high, and this leads to the best response for curbing the disease transmission. Comparing the results by adopting the IB-RA and SB-RA strategies, we found that the SB-RA strategy is more effective in preventing the disease.
The top and bottom panels of Figure 3 show the effects of β1 and β2 on the reproduction number of strain 1 (R1), the reproduction number of strain 2 (R2), final size (FES), vaccine coverage (VC), and social average payment (ASP) corresponding to the IB-RA and SB-RA rules, respectively. In Figure 3, the horizontal coordinate represents the transmission rate of strain 1, and the vertical coordinate represents the transmission rate of strain 2.
In Figure 3a-*, the reproduction number associated with strain 1 monotonically increases as the rate of transmission of strain 1 increases. A familiar trend was observed for the reproduction number with respect to strain 2 in Figure 3b-*. Furthermore, the reproduction number associated with strain 2 is not only dependent on β2, but it is influenced by β2. Figure 3c-* displays the regions of disease-free equilibrium (DFE), the dominance of strain 1, the dominance of strain 2 and the coexistence area (see Figure 3c-*), which is substantially different when compared with the results of [18]. Comparing the results of the IB-RA and SB-RA updated rules, we have seen that that the epidemic, by adopting the SB-RA strategy, has a larger final size, a less vaccine coverage, and a greater economic burden on society.
This work aims to study the co-evolution of a two-strain epidemic with coinfection and the evolution of game-theoretical vaccination behaviors. We adopted the IB-RA and the SB-RA updated rules to uptake the individuals' vaccination strategies. Through numerical analysis, we have found that co-infection deeply aggravates the spread of disease, and the basic reproduction number is closely related with both β1 and β2. Similarly, those biomass, such as the epidemic final size, the average social payoff, etc. strongly depend on the efficacy of each strain ϵj(j=1,2). This is substantially different from those outcomes of traditional epidemic models with only a single strain. We have shown that higher vaccine effectiveness and lower transmission rate are encouraged in curbing infectious diseases under the IB-RA and SB-RA updated rules, which are consistent with those results in [18]. Comparing with those results, adopting SB-RA updated rules has a better effect on reducing the epidemic final size and lowering the payoff of society.
Due to the introduction of the coinfection mechanism, there exists four scenarios: the region of the eradication of the disease, the region of strain 1 dominating, the area of strain 2 dominating and the area of the coexistence. The closer the transmission rates of the two strains are, the heavier the social burden will be, even if a huge proportion of people would like to get vaccinated. All these findings are significant guidance for the control of epidemics with coinfection by two strains.
We thank the editor and the referees for their exceptionally helpful comments during the review process. This work is partially supported by the National Natural Science Foundation of China (No.11771017, No.61573016), the Shanxi Province Science foundation (20210302123454), and the Shanxi Province Science Foundation for Youths (No. 201901D211413).
All authors declare no conflicts of interest in this paper.
P(HV←SFR)=11+exp[−(0−(−Cr))/κ],P(HV←FFR1)=11+exp[−(−1−(−Cr))/κ],P(HV←FFR2)=11+exp[−(−1−(−Cr))/κ],P(HV←FFR)=11+exp[−(−2−(−Cr))/κ],P(I1V←SFR)=11+exp[−(0−(−Cr−1))/κ],P(I1V←FFR1)=11+exp[−(−1−(−Cr−1))/κ],P(I1V←FFR2)=11+exp[−(−1−(−Cr−1))/κ],P(I1V←FFR)=11+exp[−(−2−(−Cr−1))/κ],P(I2V←SFR)=11+exp[−(0−(−Cr−1))/κ],P(I2V←FFR1)=11+exp[−(−1−(−Cr−1))/κ],P(I2V←FFR2)=11+exp[−(−1−(−Cr−1))/κ],P(I2V←FFR)=11+exp[−(−2−(−Cr−1))/κ],P(IV←SFR)=11+exp[−(0−(−Cr−2))/κ],P(IV←FFR1)=11+exp[−(−1−(−Cr−2))/κ],P(IV←FFR2)=11+exp[−(−1−(−Cr−2))/κ],P(IV←FFR)=11+exp[−(−2−(−Cr−2))/κ],P(SFR←HV)=11+exp[−(−Cr−0)/κ],P(SFR←I1V)=11+exp[−(−Cr−1−0)/κ],P(SFR←I2V)=11+exp[−(−Cr−1−0)/κ],P(SFR←IV)=11+exp[−(−Cr−2−0)/κ],P(FFR1←HV)=11+exp[−(−Cr−(−1))/κ],P(FFR1←I1V)=11+exp[−(−Cr−1−(−1))/κ],P(FFR1←I2V)=11+exp[−(−Cr−1−(−1))/κ],P(FFR1←IV)=11+exp[−(−Cr−2−(−1))/κ],P(FFR2←HV)=11+exp[−(−Cr−(−1))/κ],P(FFR2←I1V)=11+exp[−(−Cr−1−(−1))/κ],P(FFR2←I2V)=11+exp[−(−Cr−1−(−1))/κ],P(FFR2←IV)=11+exp[−(−Cr−2−(−1))/κ],P(FFR←HV)=11+exp[−(−Cr−(−2))/κ],P(FFR←I1V)=11+exp[−(−Cr−1−(−2))/κ],P(FFR←I2V)=11+exp[−(−Cr−1−(−2))/κ],P(FFR←IV)=11+exp[−(−Cr−2−(−2))/κ]. |
P(HV←NV)=11+exp[−(⟨πNV⟩−(−Cr))/κ],P(I1V←NV)=11+exp[−(⟨πNV⟩−(−Cr−1))/κ],P(I2V←NV)=11+exp[−(⟨πNV⟩−(−Cr−1))/κ],P(IV←NV)=11+exp[−(⟨πNV⟩−(−Cr−2))/κ],P(SFR←V)=11+exp[−(⟨πV⟩−(0))/κ],P(FFR1←V)=11+exp[−(⟨πV⟩−(−1))/κ],P(FFR2←V)=11+exp[−(⟨πV⟩−(−1))/κ],P(FFR←V)=11+exp[−(⟨πV⟩−(−2))/κ]. |
[1] |
S. Basu, G. B. Chapman, A. P. Galvani, Integrating epidemiology, psychology, and economics to achieve hpv vaccination targets, Proc. Natl. Acad. Sci., 105 (2008), 19018–19023. https://doi.org/10.1073/pnas.0808114105 doi: 10.1073/pnas.0808114105
![]() |
[2] |
C. T. Bauch, A. P. Galvani, D. J. D. Earn, Group interest versus self-interest in smallpox vaccination policy, Proc. Natl. Acad. Sci., 100 (2003), 10564–10567. https://doi.org/10.1073/pnas.1731324100 doi: 10.1073/pnas.1731324100
![]() |
[3] |
C. T. Bauch, D. J. D. Earn, Vaccination and the theory of games, Proc. Natl. Acad. Sci., 101 (2004), 13391–13394. https://doi.org/10.1073/pnas.0403823101 doi: 10.1073/pnas.0403823101
![]() |
[4] |
C. T. Bauch, Imitation dynamics predict vaccinating behaviour, Proc. R. Soc. B, 272 (2005), 1669–1675. https://doi.org/10.1098/rspb.2005.3153 doi: 10.1098/rspb.2005.3153
![]() |
[5] |
Z. Wang, Y. Moreno, S. Boccaletti, M. Perc, Vaccination and epidemics in networked populations–an introduction, Chaos Solitons Fractals, 103 (2017), 177–183. https://doi.org/10.1016/j.chaos.2017.06.004 doi: 10.1016/j.chaos.2017.06.004
![]() |
[6] |
K. Kuga, J. Tanimoto, Impact of imperfect vaccination and defense against contagion on vaccination behavior in complex networks, J. Stat. Mech. Theory Exp., 2018 (2018), 113402. https://doi.org/10.1088/1742-5468/aae84f doi: 10.1088/1742-5468/aae84f
![]() |
[7] |
R. Vardavas, R. Breban, S. Blower, Can influenza epidemics be prevented by voluntary vaccination?, PLoS Comput. Biol., 3 (2007), e85. https://doi.org/10.1371/journal.pcbi.0030085 doi: 10.1371/journal.pcbi.0030085
![]() |
[8] |
A. Cardillo, C. Reyes-Suárez, F. Naranjo, J. Gómez-Gardenes, Evolutionary vaccination dilemma in complex networks, Phys. Rev. E, 288 (2013), 032803. https://doi.org/10.1103/PhysRevE.88.032803 doi: 10.1103/PhysRevE.88.032803
![]() |
[9] |
B. Wu, F. Fu, L. Wang, Imperfect vaccine aggravates the long-standing dilemma of voluntary vaccination, PloS One, 6 (2011), e20577. https://doi.org/10.1371/journal.pone.0020577 doi: 10.1371/journal.pone.0020577
![]() |
[10] |
Z. Wang, C. T. Bauch, S. Bhattacharyya, A. d'Onofrio, P. Manfredi, M. Perc, et al., Statistical physics of vaccination, Phys. Rep., 664 (2016), 1–113. https://doi.org/10.1016/j.physrep.2016.10.006 doi: 10.1016/j.physrep.2016.10.006
![]() |
[11] |
A. Deka, S. Bhattacharyya, Game dynamic model of optimal budget allocation under individual vaccination choice, J. Theor. Biol., 470 (2019), 108–118. https://doi.org/10.1016/j.jtbi.2019.03.014 doi: 10.1016/j.jtbi.2019.03.014
![]() |
[12] |
G. B. Chapman, M. Li, J. Vietri, Y. Ibuka, D. Thomas, H. Yoon, et al., Using game theory to examine incentives in influenza vaccination behavior, Psychol. Sci., 23 (2012), 1008–1015. https://doi.org/10.1177/0956797612437606 doi: 10.1177/0956797612437606
![]() |
[13] |
G. Ichinose, T. Kurisaku, Positive and negative effects of social impact on evolutionary vaccination game in networks, Physica A: Statistical Mechanics and its Applications, 468 (2017), 84–90. https://doi.org/10.1016/j.physa.2016.10.017 doi: 10.1016/j.physa.2016.10.017
![]() |
[14] |
K. M. A. Kabir, K. Kuga, J. Tanimoto, Analysis of sir epidemic model with information spreading of awareness, Chaos Solitons Fractals, 119 (2019), 118–125. https://doi.org/10.1016/j.chaos.2018.12.017 doi: 10.1016/j.chaos.2018.12.017
![]() |
[15] |
K. Kuga, J. Tanimoto, imperfect vaccination or defense against contagion?, Journal of Statistical Mechanics: Theory and Experiment, 2018 (2018), 023407. https://doi.org/10.1088/1742-5468/aaac3c doi: 10.1088/1742-5468/aaac3c
![]() |
[16] |
M. Alam, M. Tanaka, J. Tanimoto, A game theoretic approach to discuss the positive secondary effect of vaccination scheme in an infinite and well-mixed population, Chaos Solitons Fractals, 125 (2019), 201–213. https://doi.org/10.1016/j.chaos.2019.05.031 doi: 10.1016/j.chaos.2019.05.031
![]() |
[17] |
M. Alam, K. Kabir, J. Tanimoto, Based on mathematical epidemiology and evolutionary game theory, which is more effective: quarantine or isolation policy?, J. Stat. Mech. Theory Exp., 2020 (2020), 033502. https://doi.org/10.1088/1742-5468/ab75ea doi: 10.1088/1742-5468/ab75ea
![]() |
[18] |
M. Arefin, K. Kabir, J. Tanimoto, A mean-field vaccination game scheme to analyze the effect of a single vaccination strategy on a two-strain epidemic spreading, J. Stat. Mech. Theory Exp., 2020 (2020), 033501. https://doi.org/10.1088/1742-5468/ab74c6 doi: 10.1088/1742-5468/ab74c6
![]() |
[19] |
K. Kabir, M. Jusup, J. Tanimoto, Behavioral incentives in a vaccination-dilemma setting with optional treatment, Phys. Rev. E, 100 (2019), 062402. https://doi.org/10.1103/PhysRevE.100.062402 doi: 10.1103/PhysRevE.100.062402
![]() |
[20] |
J. Huang, J. Wang, C. Xia, Role of vaccine efficacy in the vaccination behavior under myopic update rule on complex networks, Chaos Solitons Fractals, 130 (2020), 109425. https://doi.org/10.1016/j.chaos.2019.109425 doi: 10.1016/j.chaos.2019.109425
![]() |
[21] |
T. Krueger, K. Gogolewski, M. Bodych, A. Gambin, G. Giordano, S. Cuschieri, et al., Risk assessment of covid-19 epidemic resurgence in relation to sars-cov-2 variants and vaccination passes, Commun. Med., 2 (2022), 1–14. https://doi.org/10.1038/s43856-022-00084-w.eCollection2022 doi: 10.1038/s43856-022-00084-w.eCollection2022
![]() |
[22] |
D. Gao, T. Porco, S. Ruan, Coinfection dynamics of two diseases in a single host population, J. Math. Anal. Appl., 442 (2016), 171–188. https://doi.org/10.1016/j.jmaa.2016.04.039 doi: 10.1016/j.jmaa.2016.04.039
![]() |
[23] |
A. Elaiw, A. Agha, S. Azoz, E. Ramadan, Global analysis of within-host sars-cov-2/hiv coinfection model with latency, Eur. Phys. J. Plus, 137 (2022), 1–22. https://doi.org/10.1140/epjp/s13360-022-02387-2 doi: 10.1140/epjp/s13360-022-02387-2
![]() |
[24] |
I, Hezam, A, Foul, A, Alrasheedi, A dynamic optimal control model for covid-19 and cholera co-infection in yemen, Adv. Differ. Equations, 2021 (2021), 1–30. https://doi.org/10.1186/s13662-021-03271-6 doi: 10.1186/s13662-021-03271-6
![]() |
[25] |
M. Newman, C. Ferrario, Interacting epidemics and coinfection on contact networks, PloS One, 8 (2013), e71321. https://doi.org/10.1371/journal.pone.0071321 doi: 10.1371/journal.pone.0071321
![]() |
[26] |
S. Osman, O. Makinde, A mathematical model for coinfection of listeriosis and anthrax diseases, Int. J. Math. Math. Sci., 2018 (2018). https://doi.org/10.1155/2018/1725671 doi: 10.1155/2018/1725671
![]() |
[27] |
M. Martcheva, S. Pilyugin, The role of coinfection in multidisease dynamics. SIAM J. Appl. Math., 66 (2006), 843–872. https://doi.org/10.1137/040619272 doi: 10.1137/040619272
![]() |
[28] |
X. Li, S. Gao, Y. Fu, M. Martcheva, Modeling and research on an immuno-epidemiological coupled system with coinfection, Bull. Math. Biol., 83 (2021), 1–42. https://doi.org/10.1007/s11538-021-00946-9 doi: 10.1007/s11538-021-00946-9
![]() |
[29] |
J. Sanz, C. Xia, S. Meloni, Y. Moreno, Dynamics of interacting diseases, Phys. Rev. X, 4 (2014), 041005. https://doi.org/10.1103/PhysRevX.4.041005 doi: 10.1103/PhysRevX.4.041005
![]() |
[30] | Centers for disease control and prevention, Vaccine effectiveness: How well do flu vaccines work?, 2021. Available from: https://www.cdc.gov/flu/vaccines-work/vaccineeffect.htm. |
[31] | World Health Organization, Influenza (seasonal), 2018. Available from: https://www.who.int/news-room/fact-sheets/detail/influenza-(seasonal). |
[32] |
P. Van den Driessche, J. Watmough, Reproduction numbers and sub-threshold endemic equilibria for compartmental models of disease transmission, Math. Biosci., 180 (2002), 29–48. https://doi.org/10.1016/S0025-5564(02)00108-6 doi: 10.1016/S0025-5564(02)00108-6
![]() |
[33] |
F. Fu, D. Rosenbloom, L. Wang, M. Nowak, Imitation dynamics of vaccination behaviour on social networks, Proc. R. Soc. B, 278 (2011), 42–49. https://doi.org/10.1098/rspb.2010.1107 doi: 10.1098/rspb.2010.1107
![]() |
[34] |
E. Fukuda, S. Kokubo, J. Tanimoto, Z. Wang, A. Hagishima, N. Ikegaya, Risk assessment for infectious disease and its impact on voluntary vaccination behavior in social networks, Chaos Solitons Fractals, 68 (2014), 1–9. https://doi.org/10.1016/j.chaos.2014.07.004 doi: 10.1016/j.chaos.2014.07.004
![]() |
1. | Dun Han, Xiao Wang, Vaccination strategies and virulent mutation spread: A game theory study, 2023, 176, 09600779, 114106, 10.1016/j.chaos.2023.114106 |
Parameters | Description | Value | Source |
β1 | The transmission rate for strain 1 | 0.5 day−1 | Assumed |
β2 | The transmission rate for strain 2 | 0.7 day−1 | Assumed |
γ1 | Recovery rate after infection by strain 1 | 1/11 day−1 | [30] |
γ2 | Recovery rate after infection by strain 2 | 1/9 day−1 | [30] |
γ3 | Recovery rate after infection by strain 1 and strain 2 | 1/14 day−1 | Assumed |
e1 | Effectiveness of vaccination at protecting against strain 1 | 0.61 | [31] |
e2 | Effectiveness of vaccination at protecting against strain 2 | 0.33 | [31] |
State/Strategy | Healthy | Infected with strain 1 | Infected with strain 2 | Coinfected with strain 1 and strain 2 |
Vaccination (V) | πHV=−Cr | πI1V=−Cr−1 | πI2V=−Cr−1 | πIV=−Cr−2 |
Non-vaccination (NV) | πSFR=0 | πFFR1=−1 | πFFR2=−1 | πFFR=−2 |
Parameters | Description | Value | Source |
β1 | The transmission rate for strain 1 | 0.5 day−1 | Assumed |
β2 | The transmission rate for strain 2 | 0.7 day−1 | Assumed |
γ1 | Recovery rate after infection by strain 1 | 1/11 day−1 | [30] |
γ2 | Recovery rate after infection by strain 2 | 1/9 day−1 | [30] |
γ3 | Recovery rate after infection by strain 1 and strain 2 | 1/14 day−1 | Assumed |
e1 | Effectiveness of vaccination at protecting against strain 1 | 0.61 | [31] |
e2 | Effectiveness of vaccination at protecting against strain 2 | 0.33 | [31] |
State/Strategy | Healthy | Infected with strain 1 | Infected with strain 2 | Coinfected with strain 1 and strain 2 |
Vaccination (V) | πHV=−Cr | πI1V=−Cr−1 | πI2V=−Cr−1 | πIV=−Cr−2 |
Non-vaccination (NV) | πSFR=0 | πFFR1=−1 | πFFR2=−1 | πFFR=−2 |