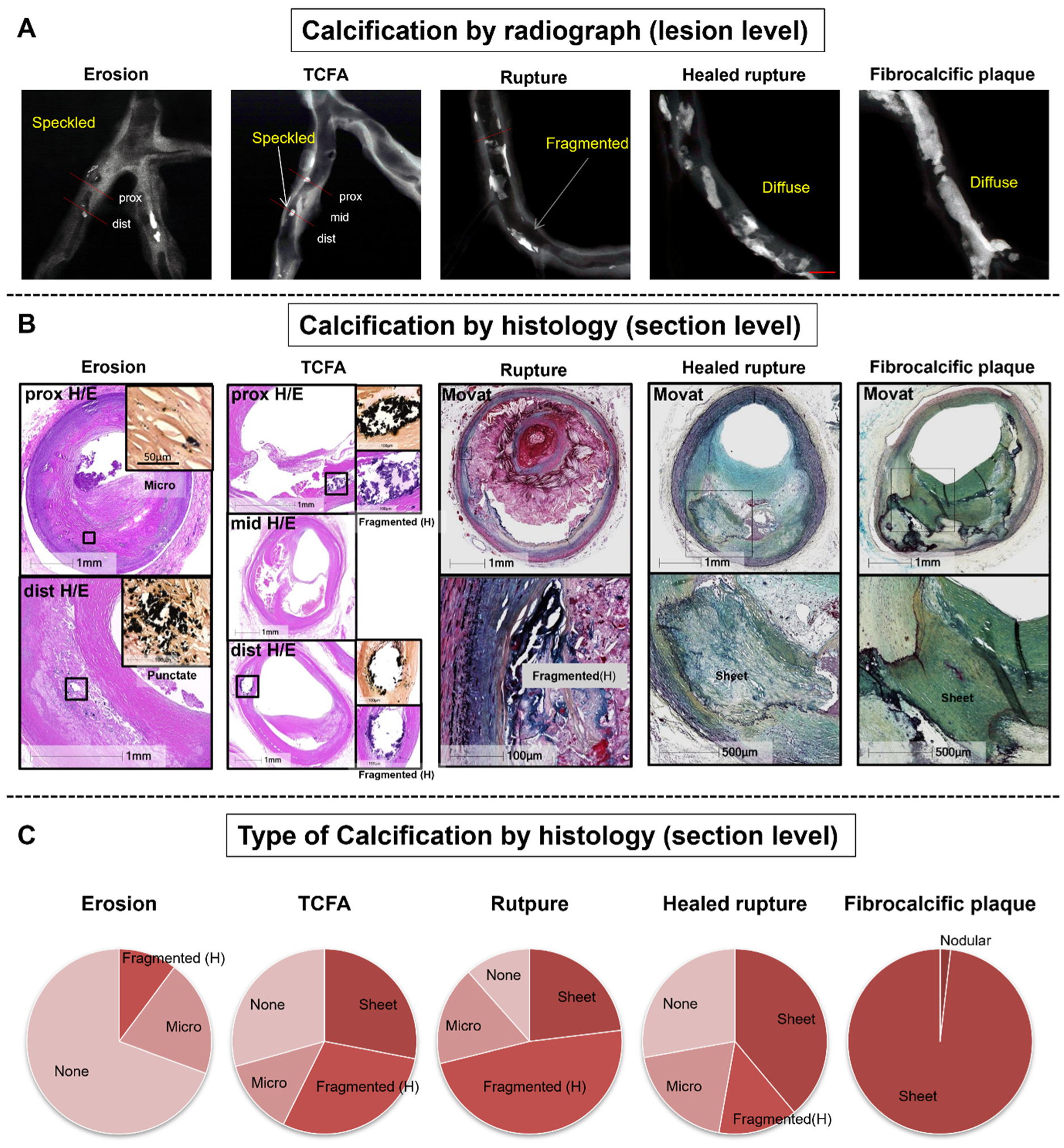
Vascular calcification involves the crystallization of calcium/phosphate in the form of hydroxyapatoite in the extracellular matrix of the arterial wall. Vascular calcification is categorized into 3 main etiologies: (1) inflammatory/atherosclerotic (mostly intimal), (2) metabolic (mostly medial), and (3) genetic background (mostly medial). Several overlapping mechanisms trigger all three types of calcifications. Intimal coronary artery calcification simultaneously develops with the progression of atherosclerosis and has been recognized as a surrogate marker of atherosclerotic inflammatory vascular disease. Pathologically, atherosclerotic calcification initially occurs as microcalcifications (0.5 to 15 µm) and results in larger dense calcification, eventually forming sheet calcifications (>3 mm). Among the plaque types, the degree of calcification is the highest in fibrocalcific plaques, followed by healed plaque ruptures, and is the lowest in pathologic intimal thickening. Recent pathologic and imaging-based studies suggest that massive dense calcifications are usually associated with stable plaques, whereas microcalcifications are indicative of vulnerable plaques which may cause acute thrombotic events. Although the mechanisms of calcification are not fully elucidated, apoptotic inflammatory cells and smooth muscle cells, along with the induction of bone formation, play crucial roles in its initiation and progression. A deeper understanding of vascular calcification will improve the risk stratification and patient outcomes through the development of new therapies.
Citation: Teruo Sekimoto, Takamasa Tanaka, Tatsuya Shiraki, Renu Virmani, Aloke V. Finn. How does atherosclerotic plaque become calcified, and why?[J]. AIMS Medical Science, 2024, 11(4): 421-438. doi: 10.3934/medsci.2024029
[1] | Ilana S. Golub, Angela Misic, Lucia P. Schroeder, Jairo Aldana-Bitar, Srikanth Krishnan, Sina Kianoush, Travis Benzing, Keishi Ichikawa, Matthew J. Budoff . Calcific coronary lesions: management, challenges, and a comprehensive review. AIMS Medical Science, 2024, 11(3): 292-317. doi: 10.3934/medsci.2024021 |
[2] | Nitin Chandra Mohan, Thomas W. Johnson . Role of intracoronary imaging in severely calcific disease. AIMS Medical Science, 2024, 11(4): 388-402. doi: 10.3934/medsci.2024027 |
[3] | Giulia Alagna, Alessia Cascone, Antonino Micari, Giancarlo Trimarchi, Francesca Campanella, Giovanni Taverna, Saro Pistorio, Giuseppe Andò . Type and duration of antithrombotic therapy after treatment of severely calcified lesions. AIMS Medical Science, 2025, 12(1): 38-62. doi: 10.3934/medsci.2025004 |
[4] | Todd T. Nowlen, Luis F. Goncalves, Susan S. Park, Arash A. Sabati . Adolescent Kawasaki Disease patient with coronary calcifications, severe 4-vessel stenosis, and a normal stress MRI. AIMS Medical Science, 2025, 12(1): 28-37. doi: 10.3934/medsci.2025003 |
[5] | Marco Ferrone, Anna Franzone, Bruno Trimarco, Giovanni Esposito, Plinio Cirillo . Percutaneous Coronary Intervention in a Patient with Acute Thrombosis of Saphenous Vein Graft and Patent Native Coronary Artery: Which is the Vessel to Approach?. AIMS Medical Science, 2015, 2(4): 310-315. doi: 10.3934/medsci.2015.4.310 |
[6] | Sophia Khattak, Farhan Shahid, Sohail Q. Khan . Challenges and risks associated with coronary calcified lesions in cardiovascular interventions: What makes calcified lesions more challenging and dangerous?. AIMS Medical Science, 2025, 12(1): 69-89. doi: 10.3934/medsci.2025006 |
[7] | Margarida Pujol-López, Luis Ortega-Paz, Manel Garabito, Salvatore Brugaletta, Manel Sabaté, Ana Paula Dantas . miRNA Update: A Review Focus on Clinical Implications of miRNA in Vascular Remodeling. AIMS Medical Science, 2017, 4(1): 99-112. doi: 10.3934/medsci.2017.1.99 |
[8] | Ryan T. Borne, Arash Aghel, Amit C. Patel, Robert K. Rogers . Innominate Steal Syndrome: A Two Patient Case Report and Review. AIMS Medical Science, 2015, 2(4): 360-370. doi: 10.3934/medsci.2015.4.360 |
[9] | Valeria Paradies, Filippo Masi, Francesco Bartolomucci, Alfredo Marchese, Armando Liso, Fabrizio Resta, Stefano Favale, Martino Pepe . No Reflow-phenomenon: from Current State of the Art to Future Perspectives. AIMS Medical Science, 2015, 2(4): 374-395. doi: 10.3934/medsci.2015.4.374 |
[10] | Lorenzo Azzalini, Philippe L. L’Allier, Jean-François Tanguay . Bioresorbable Scaffolds: The Revolution in Coronary Stenting?. AIMS Medical Science, 2016, 3(1): 126-146. doi: 10.3934/medsci.2016.1.126 |
Vascular calcification involves the crystallization of calcium/phosphate in the form of hydroxyapatoite in the extracellular matrix of the arterial wall. Vascular calcification is categorized into 3 main etiologies: (1) inflammatory/atherosclerotic (mostly intimal), (2) metabolic (mostly medial), and (3) genetic background (mostly medial). Several overlapping mechanisms trigger all three types of calcifications. Intimal coronary artery calcification simultaneously develops with the progression of atherosclerosis and has been recognized as a surrogate marker of atherosclerotic inflammatory vascular disease. Pathologically, atherosclerotic calcification initially occurs as microcalcifications (0.5 to 15 µm) and results in larger dense calcification, eventually forming sheet calcifications (>3 mm). Among the plaque types, the degree of calcification is the highest in fibrocalcific plaques, followed by healed plaque ruptures, and is the lowest in pathologic intimal thickening. Recent pathologic and imaging-based studies suggest that massive dense calcifications are usually associated with stable plaques, whereas microcalcifications are indicative of vulnerable plaques which may cause acute thrombotic events. Although the mechanisms of calcification are not fully elucidated, apoptotic inflammatory cells and smooth muscle cells, along with the induction of bone formation, play crucial roles in its initiation and progression. A deeper understanding of vascular calcification will improve the risk stratification and patient outcomes through the development of new therapies.
Coronary calcification is a key indicator of advanced atherosclerosis. The earliest lesions to show calcification are pathologic intimal thickenings, which contain extracellular lipid pools and proteoglycan matrices with microcalcifications ranging from 15 µm to 1 mm, fragmented calcifications over 1 mm, and sheet-like calcifications over 3 mm. Maximum calcifications are found in fibrocalcific plaques and healed plaque ruptures, which are detectable by either computed tomography or intravascular imaging. Lesions with acute thrombi, such as plaque ruptures and erosions, have less calcification, which often cause acute coronary syndromes. Calcified nodules, which are the least common cause of acute thrombosis, develop in highly calcified lesions and may form around fibrocalcific plaques. This review offers a comprehensive understanding of coronary artery calcifications from a pathological perspective.
Arterial wall calcifications are categorized into three main types: (1) inflammatory (atherosclerotic, mostly intimal), (2) metabolic (chronic kidney disease [CKD] and diabetes mellitus [DM], affecting both intimal and medial, also known as Monckeberg's calcification), and (3) genetic causes [1]. Atherosclerotic intimal calcifications are distinct from medial calcifications, though overlapping factors can contribute to both. While medial calcifications are generally unaffected by lipid deposition or inflammation, intimal calcification typically are. Both types may coexist in the same vessel due to risk factors such as DM, hypercholesterolemia, and CKD. Medial calcifications often result from the transformation of smooth muscle cells into osteochondrogenic cells, which is driven by ossification biomarkers and osteoblastic differentiation factors [2]. Genetic disorders can also influence metabolic mediators, thereby causing both medial and intimal calcifications. Despite these classifications, there is considerable overlap between the mechanisms.
Calcifications are classified by the plaque size and type [3],[4] (Figures 1 and 2). A microcalcification is the earliest form, and it consists of calcium particles that range from ≥0.5 µm to <15 µm in diameter. A punctate calcification ranges from >15 µm to <1 mm and is associated with macrophage apoptosis at the necrotic core's outer rim. The mechanisms of fragmented calcification, which is ≥1 mm in size, are poorly understood. A sheet calcification involves greater than one quadrant of the vessel, thereby affecting smooth muscle cells and the collagen matrix, independent of the necrotic core. A nodular calcification results from the fragmentation of a sheet calcification and is surrounded by fibrin, which does not protrude into the lumen. When such a calcification protrudes into the lumen and forms a calcified nodule, which is often accompanied by a luminal thrombus. It is rare that a bone formation with a trabeculae and a marrow space appears in severely calcified arterial segments, indicating a link between osteogenesis and severe arterial calcifications.
Atherosclerosis involves a dynamic process in which early lesions progress to advanced stages complicated by acute luminal thrombosis (Figure 3). Non-atherosclerotic intimal lesions, such as adaptive intimal thickening (AIT) and diffuse intimal thickening (AHA Type I), exist from birth and are common in atherosclerosis-prone regions. AIT, which is a physiological response to blood flow, can transition into pathologic intimal thickening (PIT) in high-risk areas [5]. Fatty streaks (FS) or intimal xanthomas (AHA Type II) are early lesions mainly composed of macrophage foam cells and lipid-laden smooth muscle cells (SMCs). These early lesions normally lack calcifications. PIT (AHA Type III) is recognized as the earliest lesion of progressive atherosclerosis. PIT contains remnants of SMC within an ECM that consist of proteoglycans and collagen (Type III) with a co-existing lipid pool [6]. This lipid pool is distinctly different from the necrotic core (NC) seen in fibroatheroma (FA), which is characterized by acellular debris and a lack of an ECM. The lipid pool formation marks the initial stage of NC development, driven by macrophage recruitment, increased metalloproteinase activity, and macrophage apoptosis. Calcifications in PIT first appear as microcalcifications within lipid pools [4], primarily due to SMC apoptosis [7]–[9], with contributions from macrophage-derived matrix vesicles [10]. SMC apoptosis produces fine microcalcifications, while apoptotic macrophages yield larger, punctate calcifications. Typically, the calcification is located in the intima near the internal elastic lamina. Microcalcifications are observed in 57% of PIT [11], and these calcifications coexist with bone-related proteins such as osteoprotegerin (OPG), osteopontin (OPN), and matrix Gla protein (MGP) (Figure 2A–B). FA, which is a progressive stage of atherosclerosis (AHA Type IV), features an acellular NC formed through macrophage infiltration into lipid pools [12]. Early FAs show macrophage infiltration, localized ECM loss, and free cholesterol concentration, with proteoglycans and Type III collagen remaining present. Late-stage FAs have increased free cholesterol, cellular debris, and complete ECM depletion due to degradation by matrix metalloproteinases from macrophages and SMCs. Numerous apoptotic macrophages contribute to a high presence of apoptotic bodies within the NC. Early FAs exhibit either microcalcifications or punctate calcifications [11], which coalesce into larger aggregates over time, thus progressing outward from the NC into the collagenous matrix that surrounds it. An intraplaque hemorrhage from compromised vasa vasorum near the NC can cause significant luminal narrowing. As the plaques progress, a thick fibrous cap composed of Type I and III collagen, proteoglycans, and interspersed SMCs with an endothelial layer forms over the NC. If the cap is thin (under 65 µm) [13], it is termed a thin fibrous cap fibroatheroma (TCFA), also called a “vulnerable plaque,” which is prone to rupture and potential luminal thrombus formation. The vulnerability of a plaque is determined by the fibrous cap thickness, primarily composed of Type I collagen and infiltrated by macrophages and T lymphocytes [14]–[16]. The majority of TCFA and ruptured plaques are localized in the proximal left anterior descending coronary artery and left circumflex artery, and are more uniformly distributed in the right coronary artery. In 92% of cases, these lesions clustered within 2 or fewer nonoverlapping 20 mm segments [17]. Vessel-specific hemodynamic conditions likely contribute to plaque progression. However, an understanding of the exact factors that determine whether a lesion progresses to stenosis remains incomplete.
A plaque rupture (PR) is the leading cause of acute coronary syndrome (ACS), which accounts for 65% of luminal thrombosis [6]. Compared to TCFA, ruptured plaques have a larger NC and more inflammatory cells in the fibrous cap. Rupture typically occurs at the thinnest, weakest region of the fibrous cap. Factors such as a high macrophage density, proteases, and a high shear and tensile stress weaken the cap at the rupture site [18],[19]. Additionally, microcalcifications from macrophages or SMCs within a thin fibrous cap may trigger rupture [20]. When the cap ruptures, circulating blood contacts highly thrombogenic components within the NC, thus resulting in luminal thrombus formation.
The second most prevalent cause of ACS (25 to 30%) is plaque erosion, which occurs in the absence of a rupture, where luminal thrombi are in direct contact with a denuded intimal surface composed of SMCs and a proteoglycan matrix [21]. The underlying lesion of eroion is typically less advanced than in ruptured plaques, and usually exhibit the characteristics of early lesions (mostly PIT or early FA) without an extensive NC, hemorrhage, or calcification. Plenty of SMCs and proteoglycans such as versican, hyaluronan, and Type III collagen are observed near the thrombus attachment site of erosion, which are different from ruptured or stable plaques, with the latter being rich in biglycan, decorin, and Type I collagen [22]. Erosion is frequently observed at arterial bifurcations and has been linked to shear stress, endothelial cell dysfunction, and factors such as myeloperoxidaze, neutrophil extracellular traps, and hyaluronan. However, the detailed mechanisms by which erosion occurs remain poorly understood.
Calcified nodules are the least frequent (<5%) cause of ACS [6], primarily occurring in highly calcified, tortuous arteries. Pathologically, breaks in calcified fibroatheromas may form protruding fragmented nodules, thus disrupting the cap and endothelium and leading to a luminal, platelet-rich thrombus [23]. These eccentric nodules often contain fibrin intermingled with calcium spicules, which are occasionally accompanied by osteoclasts and inflammatory cells. More common in older adults and patients with tortuous arteries, diabetes mellitus, or chronic kidney disease, these lesions typically appear at points of maximum artery tortuosity such as the mid-right coronary artery or near bifurcations of the left main While similar and more prevalent, a nodular calcification involves an intact fibrous cap, with the calcified nodules staying within the arterial intima, sometimes causing medial disruption and adventitial protrusion.
Further progression of calcification leads to calcified plaques with sheets or plates (>1 quadrant), and often involve SMCs and a collagenous matrix, regardless of the necrotic core. Healed plaque ruptures are detected in severely narrowed arteries, thereby showing breaks in the Type I collagen-rich fibrous cap with an overlying plaque rich in SMCs and surrounded by proteoglycans or Type III/Type I collagen, depending on the healing phase [24]. Early-healed lesions are dominated by proteoglycans and Type III collagen, and are eventually replaced by Type I collagen. Cross-sectional luminal narrowing increases with more healed ruptures, thus contributing to stenosis progression [24]. Defined by thick fibrous caps and extensive calcification, fibrocalcific plaques are common in patients with a stable angina and severe luminal narrowing. Coronary calcification correlates with plaque burden, though not linearly with plaque instability—greater calcification often means more stable plaques. Fibrocalcific plaques usually have minimal or no NC and likely represent end-stage ruptured plaques, healed plaque ruptures, or fibroatheromas, which are all characterized by dominant calcification. However, there are no comprehensive, longitudinal studies that pathologically evaluate the progression or regression of these plaque characteristics.
Atherosclerotic lesions in the carotid arteries share many characteristics with advanced coronary artery disease, thus allowing for the use of similar classifications. Based on our experience, most lesions from asymptomatic patients with carotid artery disease consist of fibrocalcific plaques. Interestingly, the frequency of calcification in both coronary and carotid arteries is comparable, with maximum calcification in the carotid arteries occurring in lesions where the lumen is narrowed by more than 70% in the cross-sectional area. The incidence of non-thrombotic fibrocalcific plaques in the carotid arteries is 11% in stroke patients, 31.9% in those with TIAs, and 39.1% in asymptomatic patients [25]. A calcified nodule is more common in the carotid than in the coronary bed, and similarly appears to remain the least frequent cause of thrombosis, thereby accounting for 7.7% of all carotid thrombi [25].
Coronary artery calcium (sheet calcification) is often found in significantly stenotic lesions (>75% cross-sectional area) rich in fibrous tissue, and is a significant independent predictor of future cardiac events [26]. However, the relationship between carotid calcification and cerebrovascular disease remains less clear. Some studies suggest that carotid plaque calcification may provide mechanical stability to the plaque, thus potentially serving as a protective feature. Conversely, other research indicates that the calcium within carotid plaques may be an independent marker for luminal stenosis and ischemic symptoms [27].
Intravascular imaging devices such as optical coherence tomography (OCT) and intravascular ultrasound (IVUS), which are used during PCI, can detect coronary artery calcifications. OCT or optical frequency domain imaging (OFDI) can identify sheet calcifications as a signal-poor or heterogeneous region with a sharply delineated border [14]. The appearance of a nodular calcification is different from a sheet calcification. A nodular calcification, which follows a sheet calcification, presents a heterogeneous signal with high attenuation, presumably due to fibrin interposed between nodules (Figure 4). OCT/OFDI offers a higher spatial resolution compared to an IVUS; however, the penetration depth of the near-infrared light used in OCT/OFDI is approximately 2 mm, which is less than half of that of an IVUS. Therefore, while OCT/OFDI excels in observing the fine tissue characteristics on the surface of the vessel, it has limitations in visualizing the entire vessel and assessing remodeling. In particular, lipid components (necrotic core) and acute thrombus often cause significant attenuation of the OCT/OFDI signal due to light scattering, making it difficult to observe the areas behind them (Figure 4).
We have previously reported the utility of micro-computed tomography (micro-CT), which has a resolution at the micron-level range, to detect the morphologic features of atherosclerosis obtained during an autopsy [28],[29]. Figure 5 shows that micro-CT allows for a more detailed observation of the plaque characteristics compared to CT, which is used in clinical practice, thus suggesting the possibility of obtaining images that are closer to the pathological findings [30]. With the improvements in CT imaging technologies, one day, identifying microcalcifications might become feasible during routine clinical practice, which are indicative of unstable plaques, as well as distinguishing between sheet calcifications and nodular calcifications in severe calcified lesions. Techniques such as spectral CT and advanced morphometric analysis may play a key role in achieving these capabilities [31],[32]. Furthermore, it has been reported that a higher calcium density is inversely correlated with the risk of coronary artery disease [33],[34]. The low calcium density is more likely associated with microcalcifications seen in unstable plaques, whereas a high calcium density may represent fibrocalcific plaques, which are indicative of a more stable plaque morphology. They could become instrumental in stratifying high-risk patients, thereby enhancing the precision of cardiovascular risk management.
We previously reported pathological differences in the extent of coronary calcifications between the genders [36],[37]. When stratified by decades, men exhibited greater calcifications than women up to their sixties, whereas the prevalence was similar in the seventies, thus suggesting a rapid calcification development during the postmenopausal period. Additionally, postmenopausal women have three times higher calcifications than premenopausal women. Regarding the etiology of ACS, rupture occurred in 64.7% (n = 178) and erosion in 34.5% (n = 95) of cases in men under 50 years old (n = 275), while rupture was seen in 76.6% (n = 118) and erosion in 16.9% of cases (n = 26) in men over 50 years old (n = 154) [38]. On the other hand, rupture occurred in 22.9% (n = 11) and erosion in 77.1% of cases (n = 37) in women under 50 years old (n = 48), whereas rupture was seen in 50% (n = 23) and erosion in 21.7% of cases (n = 10) in women over 50 years old (n = 46) [38]. Unlike men, young women predominantly experienced erosion; however, the incidence of rupture increased in women over 50 years old. The development of atherosclerosis differed between men and women, not only in the prevalence and impact of coronary risk factors, but also due to the presence of female-specific risk factors, which are gaining attention [39]. The coronary artery calcification score (CACs) evaluated by CT predicts the risk of cardiovascular events over a 10-year period in women in a graded fashion [40]. A study of 63215 asymptomatic individuals evaluated by CT showed that while women were less likely to have prevalent CACs than men at a given age, detectable CACs indicated a higher relative risk of cardiovascular disease in women [41]. An emerging risk marker for women is breast arterial calcification, which is a medial artery calcification incidentally found on mammograms, and are associated with CACs and future cardiovascular risk [42]–[44]. Detecting breast arterial calcifications might be a useful additional cardiovascular risk screening.
DM and CKD are significant risk factors for coronary artery disease. Our autopsy registry showed that the mean percent plaque area composed of NC was greater in DM subjects compared to non-DM subjects. DM subjects had a higher mean percent calcified area than non-DM subjects (12.1% vs. 9.4%; P = 0.05) [45]. Higher HbA1c levels in sudden death cases were associated with fewer non-calcified lesions and more sheet calcifications [46]. Consistent with these morphometric findings, the number of healed plaque ruptures was the highest in the DM subjects, thus indicating more advanced atherosclerotic plaques. Individuals with CKD have a higher incidence and prevalence of CAC and rapid CAC progression compared to the general population [47],[48]. A recent analysis of the Multi-Ethnic Study of Atherosclerosis (MESA) study categorized 6780 subjects by the DM and CKD status, and showed that CACs predict cardiovascular disease onset in CKD patients, regardless of the DM presence [49]. Dysregulated mineral metabolism, including increased phosphate, fibroblast growth factor 23, parathyroid hormone, and 1,25-dihydroxy vitamin D, along with various local and systemic factors, contribute to cardiovascular calcification in CKD [50].
The effect of a high-intensity statin therapy on plaque has been well established using imaging devices. Statins slow the progression of the overall coronary atherosclerosis volume, increase the plaque calcification, and reduce the high-risk plaque features. The PARADIGM clinical trial compared coronary plaque volume changes in statin-naive (n = 474) and statin-taking patients (n = 781) using CT [51]. Statin users showed a slower atheroma volume progression (1.76% vs. 2.04% per year, P = 0.002) but a faster CAC burden progression (1.27% vs. 0.98% per year, P < 0.001). New high-risk plaque features were lower in statin users (0.9% vs. 1.6% per year, P < 0.001). A recent cohort study of 857 patients (2458 lesions) assessed the association between statin use and atherosclerotic plaque progression using CT over more than two years [52]. The plaque compositions were categorized by the CT attenuation values: low attenuation, fibro-fatty, fibrous, low-density calcium, high-density calcium, and 1K plaques. Statin use reduced the low attenuation and fibro-fatty plaques but increased the high-density calcium and 1K plaques. In lesions without either a baseline low-attenuation or fibro-fatty plaque, statin therapy did not change the overall calcified plaque volume but promoted more dense calcium formation. These findings suggest that statins reduce the necrotic core and promote sheet calcifications.
Calcification is a critical finding in the progression of coronary atherosclerosis. It serves not only as a predictor of future cardiovascular events, but also as an indicator that can differentiate between unstable and stable plaques. With advancements in imaging technologies, it has become possible to identify coronary calcifications, thus leading to new insights into its characteristics and implications. However, many aspects of the mechanisms underlying calcification remain unclear. A deeper understanding of the detailed nature and mechanisms of vascular calcification will not only improve the risk stratification, but also lead to the development of new therapies aimed at suppressing cardiovascular events and improving the patient outcomes. Continued research in this area is essential to advance our knowledge and treatment of coronary artery disease.
Teruo Sekimoto, Takamasa Tanaka, Tatsuya Shiraki and Renu Virmani: Conceptualization; Aloke V. Finn: Supervision; Teruo Sekimoto: Writing—original draft.
The authors declare they have not used Artificial Intelligence (AI) tools in the creation of this article.
[1] |
Demer LL, Tintut Y (2014) Inflammatory, metabolic, and genetic mechanisms of vascular calcification. Arterioscler Thromb Vasc Biol 34: 715-723. https://doi.org/10.1161/ATVBAHA.113.302070 ![]() |
[2] |
Qiao JH, Mertens RB, Fishbein MC, et al. (2003) Cartilaginous metaplasia in calcified diabetic peripheral vascular disease: morphologic evidence of enchondral ossification. Hum Pathol 34: 402-407. https://doi.org/10.1053/hupa.2003.72 ![]() |
[3] |
Mori H, Torii S, Kutyna M, et al. (2018) Coronary artery calcification and its progression: what does it really mean?. JACC Cardiovasc Imaging 11: 127-142. https://doi.org/10.1016/j.jcmg.2017.10.012 ![]() |
[4] |
Otsuka F, Sakakura K, Yahagi K, et al. (2014) Has our understanding of calcification in human coronary atherosclerosis progressed?. Arterioscler Thromb Vasc Biol 34: 724-736. https://doi.org/10.1161/ATVBAHA.113.302642 ![]() |
[5] |
Stary HC, Chandler AB, Glagov S, et al. (1994) A definition of initial, fatty streak, and intermediate lesions of atherosclerosis. A report from the committee on vascular lesions of the council on Arteriosclerosis, American Heart Association. Circulation 89: 2462-2478. https://doi.org/10.1161/01.cir.89.5.2462 ![]() |
[6] |
Virmani R, Kolodgie FD, Burke AP, et al. (2000) Lessons from sudden coronary death: a comprehensive morphological classification scheme for atherosclerotic lesions. Arterioscler Thromb Vasc Biol 20: 1262-1275. https://doi.org/10.1161/01.atv.20.5.1262 ![]() |
[7] |
Kockx MM, De Meyer GR, Muhring J, et al. (1998) Apoptosis and related proteins in different stages of human atherosclerotic plaques. Circulation 97: 2307-2315. https://doi.org/10.1161/01.cir.97.23.2307 ![]() |
[8] |
Kelly-Arnold A, Maldonado N, Laudier D, et al. (2013) Revised microcalcification hypothesis for fibrous cap rupture in human coronary arteries. Proc Natl Acad Sci U S A 110: 10741-10746. https://doi.org/10.1073/pnas.1308814110 ![]() |
[9] |
Kapustin AN, Shanahan CM (2012) Calcium regulation of vascular smooth muscle cell-derived matrix vesicles. Trends Cardiovasc Med 22: 133-137. https://doi.org/10.1016/j.tcm.2012.07.009 ![]() |
[10] |
New SE, Goettsch C, Aikawa M, et al. (2013) Macrophage-derived matrix vesicles: an alternative novel mechanism for microcalcification in atherosclerotic plaques. Circ Res 113: 72-77. https://doi.org/10.1161/CIRCRESAHA.113.301036 ![]() |
[11] |
Otsuka F, Kramer MC, Woudstra P, et al. (2015) Natural progression of atherosclerosis from pathologic intimal thickening to late fibroatheroma in human coronary arteries: a pathology study. Atherosclerosis 241: 772-782. https://doi.org/10.1016/j.atherosclerosis.2015.05.011 ![]() |
[12] |
Yahagi K, Kolodgie FD, Otsuka F, et al. (2016) Pathophysiology of native coronary, vein graft, and in-stent atherosclerosis. Nat Rev Cardiol 13: 79-98. https://doi.org/10.1038/nrcardio.2015.164 ![]() |
[13] |
Burke AP, Farb A, Malcom GT, et al. (1997) Coronary risk factors and plaque morphology in men with coronary disease who died suddenly. N Engl J Med 336: 1276-1282. https://doi.org/10.1056/NEJM199705013361802 ![]() |
[14] |
Tearney GJ, Regar E, Akasaka T, et al. (2012) Consensus standards for acquisition, measurement, and reporting of intravascular optical coherence tomography studies: a report from the International Working Group for Intravascular Optical Coherence Tomography Standardization and Validation. J Am Coll Cardiol 59: 1058-1072. https://doi.org/10.1016/j.jacc.2011.09.079 ![]() |
[15] |
Kolodgie FD, Burke AP, Farb A, et al. (2001) The thin-cap fibroatheroma: a type of vulnerable plaque: the major precursor lesion to acute coronary syndromes. Curr Opin Cardiol 16: 285-292. https://doi.org/10.1097/00001573-200109000-00006 ![]() |
[16] |
Falk E, Nakano M, Bentzon JF, et al. (2013) Update on acute coronary syndromes: the pathologists' view. Eur Heart J 34: 719-728. https://doi.org/10.1093/eurheartj/ehs411 ![]() |
[17] |
Cheruvu PK, Finn AV, Gardner C, et al. (2007) Frequency and distribution of thin-cap fibroatheroma and ruptured plaques in human coronary arteries: a pathologic study. J Am Coll Cardiol 50: 940-949. https://doi.org/10.1016/j.jacc.2007.04.086 ![]() |
[18] |
Gijsen FJ, Wentzel JJ, Thury A, et al. (2008) Strain distribution over plaques in human coronary arteries relates to shear stress. Am J Physiol Heart Circ Physiol 295: H1608-H1614. https://doi.org/10.1152/ajpheart.01081.2007 ![]() |
[19] |
Sukhova GK, Schonbeck U, Rabkin E, et al. (1999) Evidence for increased collagenolysis by interstitial collagenases-1 and -3 in vulnerable human atheromatous plaques. Circulation 99: 2503-2509. https://doi.org/10.1161/01.cir.99.19.2503 ![]() |
[20] |
Vengrenyuk Y, Carlier S, Xanthos S, et al. (2006) A hypothesis for vulnerable plaque rupture due to stress-induced debonding around cellular microcalcifications in thin fibrous caps. Proc Natl Acad Sc U S A 103: 14678-14683. https://doi.org/10.1073/pnas.0606310103 ![]() |
[21] |
Farb A, Burke AP, Tang AL, et al. (1996) Coronary plaque erosion without rupture into a lipid core. A frequent cause of coronary thrombosis in sudden coronary death. Circulation 93: 1354-1363. https://doi.org/10.1161/01.cir.93.7.1354 ![]() |
[22] |
Kolodgie FD, Burke AP, Farb A, et al. (2002) Differential accumulation of proteoglycans and hyaluronan in culprit lesions: insights into plaque erosion. Arterioscler Thromb Vasc Biol 22: 1642-1648. https://doi.org/10.1161/01.atv.0000034021.92658.4c ![]() |
[23] |
Torii S, Sato Y, Otsuka F, et al. (2021) Eruptive calcified nodules as a potential mechanism of acute coronary thrombosis and sudden death. J Am Coll Cardiol 77: 1599-1611. https://doi.org/10.1016/j.jacc.2021.02.016 ![]() |
[24] |
Mann J, Davies MJ (1999) Mechanisms of progression in native coronary artery disease: role of healed plaque disruption. Heart 82: 265-268. https://doi.org/10.1136/hrt.82.3.265 ![]() |
[25] |
Mauriello A, Sangiorgi GM, Virmani R, et al. (2010) A pathobiologic link between risk factors profile and morphological markers of carotid instability. Atherosclerosis 208: 572-580. https://doi.org/10.1016/j.atherosclerosis.2009.07.048 ![]() |
[26] |
Detrano R, Guerci AD, Carr JJ, et al. (2008) Coronary calcium as a predictor of coronary events in four racial or ethnic groups. N Engl J Med 358: 1336-1345. https://doi.org/10.1056/NEJMoa072100 ![]() |
[27] |
Nandalur KR, Baskurt E, Hagspiel KD, et al. (2006) Carotid artery calcification on CT may independently predict stroke risk. AJR Am J Roentgenol 186: 547-552. https://doi.org/10.2214/AJR.04.1216 ![]() |
[28] |
Kuntz SH, Torii S, Jinnouchi H, et al. (2020) Pathology and multimodality imaging of acute and chronic femoral stenting in humans. JACC Cardiovasc Interv 13: 418-427. https://doi.org/10.1016/j.jcin.2019.10.060 ![]() |
[29] |
Kawai K, Sato Y, Hokama JY, et al. (2023) Histology, OCT, and Micro-CT evaluation of coronary calcification treated with intravascular lithotripsy: atherosclerotic cadaver study. JACC Cardiovasc Interv 16: 2097-2108. https://doi.org/10.1016/j.jcin.2023.06.021 ![]() |
[30] |
Jinnouchi H, Sato Y, Sakamoto A, et al. (2020) Calcium deposition within coronary atherosclerotic lesion: implications for plaque stability. Atherosclerosis 306: 85-95. https://doi.org/10.1016/j.atherosclerosis.2020.05.017 ![]() |
[31] |
Buckler AJ, Gotto AM, Rajeev A, et al. (2023) Atherosclerosis risk classification with computed tomography angiography: A radiologic-pathologic validation study. Atherosclerosis 366: 42-48. https://doi.org/10.1016/j.atherosclerosis.2022.11.013 ![]() |
[32] | Buckler AJ, Abbara S, Budoff MJ, et al. (2024) Special report on the consensus QIBA profile for objective analytical validation of non-calcified and high-risk plaque and other biomarkers using computed tomography angiography. Acad Radiol . https://doi.org/10.1016/j.acra.2024.07.014 |
[33] |
Razavi AC, van Assen M, De Cecco CN, et al. (2022) Discordance between coronary artery calcium area and density predicts long-term atherosclerotic cardiovascular disease risk. JACC Cardiovasc Imaging 15: 1929-1940. https://doi.org/10.1016/j.jcmg.2022.06.007 ![]() |
[34] |
Bhatia HS, McClelland RL, Denenberg J, et al. (2023) Coronary artery calcium density and cardiovascular events by volume level: the MESA. Circ Cardiovasc Imaging 16: e014788. https://doi.org/10.1161/CIRCIMAGING.122.014788 ![]() |
[35] |
Nakano M, Yahagi K, Yamamoto H, et al. (2016) Additive value of integrated backscatter IVUS for detection of vulnerable plaque by optical frequency domain imaging: an ex vivo autopsy study of human coronary arteries. JACC Cardiovasc Imaging 9: 163-172. https://doi.org/10.1016/j.jcmg.2015.07.011 ![]() |
[36] |
Sakakura K, Nakano M, Otsuka F, et al. (2014) Comparison of pathology of chronic total occlusion with and without coronary artery bypass graft. Eur Heart J 35: 1683-1693. https://doi.org/10.1093/eurheartj/eht422 ![]() |
[37] |
Burke AP, Farb A, Malcom G, et al. (2001) Effect of menopause on plaque morphologic characteristics in coronary atherosclerosis. Am Heart J 141: S58-S62. https://doi.org/10.1067/mhj.2001.109946 ![]() |
[38] |
Sato Y, Kawakami R, Sakamoto A, et al. (2022) Sex differences in coronary atherosclerosis. Curr Atheroscler Rep 24: 23-32. https://doi.org/10.1007/s11883-022-00980-5 ![]() |
[39] |
Rajendran A, Minhas AS, Kazzi B, et al. (2023) Sex-specific differences in cardiovascular risk factors and implications for cardiovascular disease prevention in women. Atherosclerosis 384: 117269. https://doi.org/10.1016/j.atherosclerosis.2023.117269 ![]() |
[40] |
Budoff MJ, Young R, Burke G, et al. (2018) Ten-year association of coronary artery calcium with atherosclerotic cardiovascular disease (ASCVD) events: the multi-ethnic study of atherosclerosis (MESA). Eur Heart J 39: 2401-2408. https://doi.org/10.1093/eurheartj/ehy217 ![]() |
[41] |
Shaw LJ, Min JK, Nasir K, et al. (2018) Sex differences in calcified plaque and long-term cardiovascular mortality: observations from the CAC Consortium. Eur Heart J 39: 3727-3735. https://doi.org/10.1093/eurheartj/ehy534 ![]() |
[42] |
Maas AH, van der Schouw YT, Atsma F, et al. (2007) Breast arterial calcifications are correlated with subsequent development of coronary artery calcifications, but their aetiology is predominantly different. Eur J Radiol 63: 396-400. https://doi.org/10.1016/j.ejrad.2007.02.009 ![]() |
[43] |
Quispe R, Al-Rifai M, Di Carlo PA, et al. (2019) Breast arterial calcium: a game changer in women's cardiovascular health?. JACC Cardiovasc Imaging 12: 2538-2548. https://doi.org/10.1016/j.jcmg.2018.07.035 ![]() |
[44] |
Koh TJW, Tan HJH, Ravi PRJ, et al. (2023) Association between breast arterial calcifications and cardiovascular disease: a systematic review and meta-analysis. Can J Cardiol 39: 1941-1950. https://doi.org/10.1016/j.cjca.2023.07.024 ![]() |
[45] |
Burke AP, Kolodgie FD, Zieske A, et al. (2004) Morphologic findings of coronary atherosclerotic plaques in diabetics: a postmortem study. Arterioscler Thromb Vasc Biol 24: 1266-1271. https://doi.org/10.1161/01.ATV.0000131783.74034.97 ![]() |
[46] |
Yahagi K, Kolodgie FD, Lutter C, et al. (2017) Pathology of human coronary and carotid artery atherosclerosis and vascular calcification in diabetes mellitus. Arterioscler Thromb Vasc Biol 37: 191-204. https://doi.org/10.1161/ATVBAHA.116.306256 ![]() |
[47] |
Lamprea-Montealegre JA, McClelland RL, Astor BC, et al. (2013) Chronic kidney disease, plasma lipoproteins, and coronary artery calcium incidence: the Multi-Ethnic Study of Atherosclerosis. Arterioscler Thromb Vasc Biol 33: 652-658. https://doi.org/10.1161/ATVBAHA.112.300624 ![]() |
[48] |
Kestenbaum BR, Adeney KL, de Boer IH, et al. (2009) Incidence and progression of coronary calcification in chronic kidney disease: the Multi-Ethnic Study of Atherosclerosis. Kidney Int 76: 991-998. https://doi.org/10.1038/ki.2009.298 ![]() |
[49] |
Shroff GR, Sanchez OA, Miedema MD, et al. (2020) Coronary artery calcium progresses rapidly and discriminates incident cardiovascular events in chronic kidney disease regardless of diabetes: The Multi-Ethnic Study of Atherosclerosis (MESA). Atherosclerosis 310: 75-82. https://doi.org/10.1016/j.atherosclerosis.2020.07.026 ![]() |
[50] |
Hutcheson JD, Goettsch C (2023) Cardiovascular calcification heterogeneity in chronic kidney disease. Circ Res 132: 993-1012. https://doi.org/10.1161/CIRCRESAHA.123.321760 ![]() |
[51] |
Lee SE, Chang HJ, Sung JM, et al. (2018) Effects of statins on coronary atherosclerotic plaques: the PARADIGM study. JACC Cardiovasc Imaging 11: 1475-1484. https://doi.org/10.1016/j.jcmg.2018.04.015 ![]() |
[52] |
van Rosendael AR, van den Hoogen IJ, Gianni U, et al. (2021) Association of statin treatment with progression of coronary atherosclerotic plaque composition. JAMA Cardiol 6: 1257-1266. https://doi.org/10.1001/jamacardio.2021.3055 ![]() |