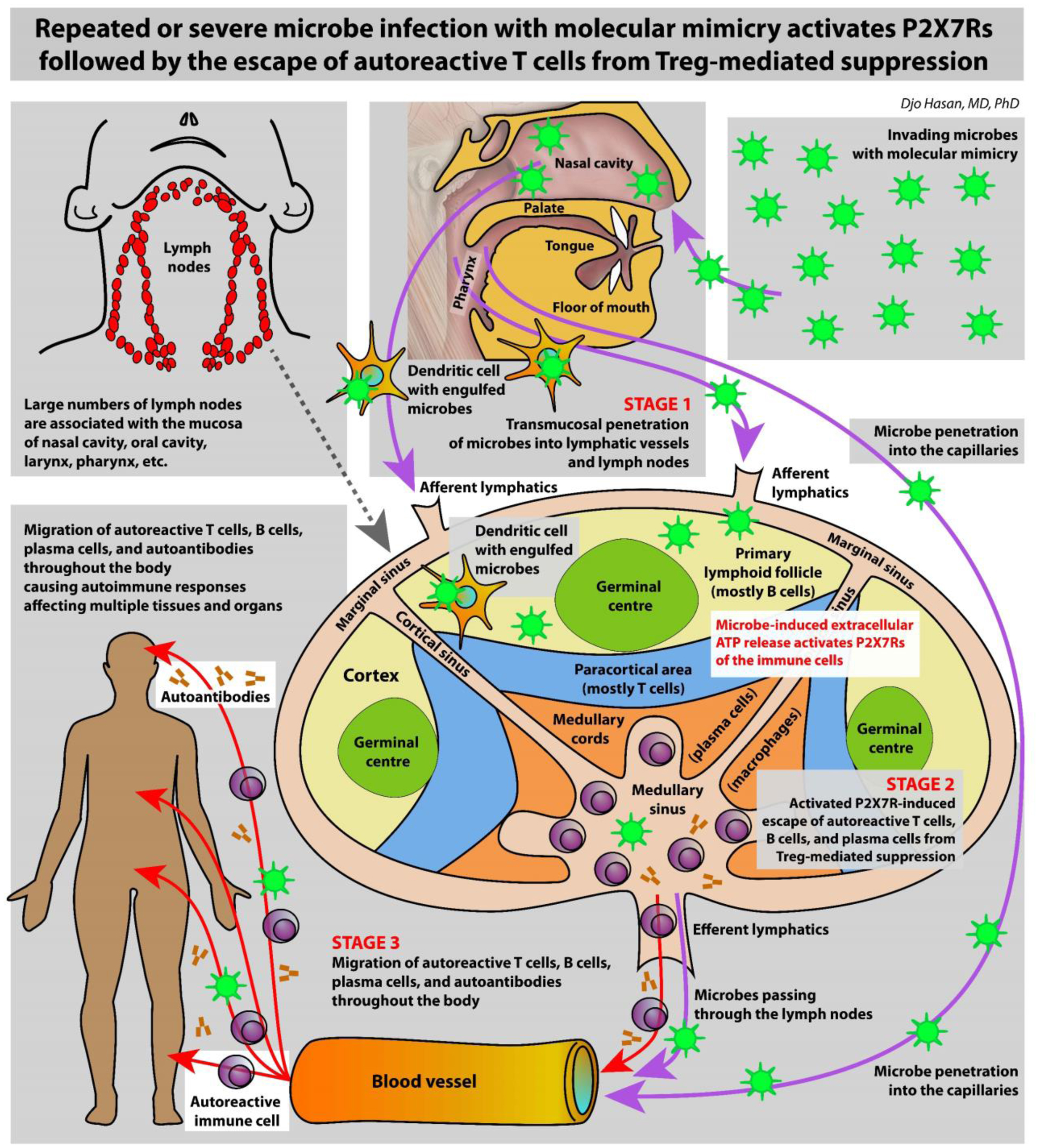
The immune system is meant to protect against invading microbes. Although this system is effective against many microbes, some can use molecular mimicry to turn the immune system against the host and activate autoimmune responses. The resulting autoimmunity has significant implications for public health and healthcare costs. It is well known that regulatory T cells (Tregs) are crucial for self-tolerance and that their function is impaired after exposure to self-antigens or antigens with molecular mimicry, leading to the activation of autoimmune responses. How molecular mimicry disrupts Tregs in this manner remains under debate. This review contributes to the field of the pathogenesis of autoimmunity by proposing that purinergic signaling in the lymph nodes, with extracellular ATP, ADP, and adenosine as ligands, plays a pivotal role in this process. Repeated or high-dose microbial infection causes the release of large amounts of extracellular ATP sufficient to reach the threshold of extracellular ATP levels for activating P2X7 purinergic receptors (P2X7Rs) on dendritic cells and Tregs. This hampers the ability of Tregs to suppress autoimmune responses. Crucially, P2X7Rs are activated at very high extracellular ATP levels, thus only after repeated or high-dose infection with microbes. Arguably, in contrast to the rapid elimination of microbes with foreign antigens, the clearance of invading microbes that employ molecular mimicry requires the activation of P2X7Rs at the expense of self-tolerance. Because all processes required to activate autoimmune responses occur in secondary lymphoid organs, this article hypothesizes that, contrary to current convention, microbes do not need to enter organs to initiate autoimmunity. However, some types of microbes can prevent P2X7R-induced Treg disruption by converting extracellular ATP to adenosine, mitigating autoimmune responses resulting in chronic diseases with less severe inflammation. The proposed hypothesized mechanism has potential implications for the understanding and treatment of autoimmune disorders.
Citation: Djo Hasan. Purinergic P2X7R expressed on regulatory T cells potentially links molecular mimicry to autoimmune responses[J]. AIMS Allergy and Immunology, 2024, 8(2): 80-123. doi: 10.3934/Allergy.2024006
[1] | Katarzyna Nazimek . The complex functions of microRNA-150 in allergy, autoimmunity and immune tolerance. AIMS Allergy and Immunology, 2021, 5(4): 195-221. doi: 10.3934/Allergy.2021016 |
[2] | Ken S. Rosenthal . Immune monitoring of the body’s borders. AIMS Allergy and Immunology, 2018, 2(3): 148-164. doi: 10.3934/Allergy.2018.3.148 |
[3] | Mansur Aliyu, Fatema Zohora, Ali Akbar Saboor-Yaraghi . Spleen in innate and adaptive immunity regulation. AIMS Allergy and Immunology, 2021, 5(1): 1-17. doi: 10.3934/Allergy.2021001 |
[4] | Ryuta Muromoto, Kenji Oritani, Tadashi Matsuda . IL-17 signaling is regulated through intrinsic stability control of mRNA during inflammation. AIMS Allergy and Immunology, 2022, 6(3): 188-199. doi: 10.3934/Allergy.2022014 |
[5] | Chunyan Li, Wojciech Dawicki, Xiaobei Zhang, Chris Rudulier, John R. Gordon . IL-10- and retinoic acid-induced regulatory dendritic cells are therapeutically equivalent in mouse models of asthma and food allergy. AIMS Allergy and Immunology, 2021, 5(2): 73-91. doi: 10.3934/Allergy.2021007 |
[6] | Ken S. Rosenthal, Daniel H. Zimmerman . J-LEAPS vaccines elicit antigen specific Th1 responses by promoting maturation of type 1 dendritic cells (DC1). AIMS Allergy and Immunology, 2017, 1(2): 89-100. doi: 10.3934/Allergy.2017.2.89 |
[7] | Xi Luo, Hehong Li, Rongshan Chen, Yinhui Zeng, Wenlong Liu, Qingxiang Zeng . MicroRNA-155 is a critical regulator of regulatory T cells in OM-85 Broncho-Vaxom treated experimental models of allergic rhinitis. AIMS Allergy and Immunology, 2024, 8(1): 8-17. doi: 10.3934/Allergy.2024002 |
[8] | James Peterson . Affinity and avidity models in autoimmune disease. AIMS Allergy and Immunology, 2018, 2(1): 45-81. doi: 10.3934/Allergy.2018.1.45 |
[9] | Tuba Karakurt, Nurhan Kasap, Kübra Aslan, Hayrunnisa Bekis Bozkurt, Fatma Bal Cetinkaya, Gizem Uslu, Zafer Bicakci, Filiz Özen, Özlem Cavkaytar, Ahmet Eken, Mustafa Arga . Suspected ALPS with clinical and laboratory findings: Three patients—three different diagnoses. AIMS Allergy and Immunology, 2023, 7(4): 304-312. doi: 10.3934/Allergy.2023020 |
[10] | Izabela Krzemień, Angelika Szatan, Paulina Skalska, Martyna Cieślik, Angelika Domagała, Magdalena Gębicka, Krzysztof Bryniarski, Katarzyna Nazimek . Serum cytokine concentrations correlate with the severity of imiquimod and IL-23-induced psoriatic inflammation in mice. AIMS Allergy and Immunology, 2024, 8(4): 265-282. doi: 10.3934/Allergy.2024016 |
The immune system is meant to protect against invading microbes. Although this system is effective against many microbes, some can use molecular mimicry to turn the immune system against the host and activate autoimmune responses. The resulting autoimmunity has significant implications for public health and healthcare costs. It is well known that regulatory T cells (Tregs) are crucial for self-tolerance and that their function is impaired after exposure to self-antigens or antigens with molecular mimicry, leading to the activation of autoimmune responses. How molecular mimicry disrupts Tregs in this manner remains under debate. This review contributes to the field of the pathogenesis of autoimmunity by proposing that purinergic signaling in the lymph nodes, with extracellular ATP, ADP, and adenosine as ligands, plays a pivotal role in this process. Repeated or high-dose microbial infection causes the release of large amounts of extracellular ATP sufficient to reach the threshold of extracellular ATP levels for activating P2X7 purinergic receptors (P2X7Rs) on dendritic cells and Tregs. This hampers the ability of Tregs to suppress autoimmune responses. Crucially, P2X7Rs are activated at very high extracellular ATP levels, thus only after repeated or high-dose infection with microbes. Arguably, in contrast to the rapid elimination of microbes with foreign antigens, the clearance of invading microbes that employ molecular mimicry requires the activation of P2X7Rs at the expense of self-tolerance. Because all processes required to activate autoimmune responses occur in secondary lymphoid organs, this article hypothesizes that, contrary to current convention, microbes do not need to enter organs to initiate autoimmunity. However, some types of microbes can prevent P2X7R-induced Treg disruption by converting extracellular ATP to adenosine, mitigating autoimmune responses resulting in chronic diseases with less severe inflammation. The proposed hypothesized mechanism has potential implications for the understanding and treatment of autoimmune disorders.
Autoimmune disorders have a major impact on the daily lives of patients and their families, as well as on public health. With their rapidly increasing prevalence, autoimmune disorders also contribute to rising healthcare costs [1]. Therefore, understanding the mechanism by which autoimmunity develops is essential and urgently needed [1]. Currently, the development of autoimmunity is attributed to a complex interaction of multiple genetic and environmental risk factors, including microbial infections [1]. In this review, I will discuss the link between microbes that mimic human antigens and autoimmunity.
The immune system should be the best ally of the host. It successfully protects against many microbes when they attempt to invade the body and proliferate in host cells. For example, Newcastle disease virus (NDV) can be a ruthless killer in birds [2] but only causes transient inflammation of the local mucosa at its entry site and flu-like symptoms in humans (avian pseudopest) [3],[4]. However, numerous microbes can manipulate the immune system by mimicking host antigens (molecular mimicry). These microbes cause the best ally of the host to turn against itself [5]–[8]. It is no surprise that these microbes are strongly related to autoimmune disorders [9].
Recently, in the context of the severe acute respiratory syndrome coronavirus 2 (SARS-CoV-2) pandemic, autoantibodies (antibodies specific for self-antigens) associated with viral infections have attracted increasing attention. However, interactions between autoantibodies and microbial infections have long been known to occur. The first hypothesis that there was an autoimmune response in the form of autoantibodies in patients with syphilis dates back to 1909 [10]. Later, in 1934, researchers found brain-specific antibodies (autoantibodies) in rabbits. These autoantibodies were induced by injections of a fresh rabbit brain emulsion and an emulsion of brain tissue infected with a vaccine virus [11]. A few years later, in 1945, autoantibodies directed against infected liver and normal liver tissue were observed in rhesus monkeys vaccinated against yellow fever [12]. Additionally, autoantibodies specific for the human heart were found in the majority of patients with streptococcal rheumatic fever in 1945 [13].
Since then, advances in immunology have shown that autoreactive T cells play a key role in autoimmune responses [14],[15]. The basic concept is that the immune system manages precursors of autoreactive T cells in the thymus (thymocytes) by eliminating the clones with the strongest responses (thymic clonal deletion) or by directing them to become regulatory T cells (Tregs) that can control the remaining autoreactive T cells [16]. However, it has become clear that molecular mimicry can disrupt the control of autoreactive T cells. Microbes that employ molecular mimicry harbor antigens with sufficient similarity to the host's antigens to be recognized as self-antigens.
There is ample evidence from research in humans and animals and in vitro studies to support the role of molecular mimicry in the development of autoimmune disorders [5],[17]–[20]. However, the precise mechanism by which molecular mimicry can cause the immune system to lose its ability to promote self-tolerance has not yet been fully clarified and is therefore still debated [1],[6],[9],[21]. This review attempts to contribute to the understanding of the mechanism of autoimmune responses by proposing a link between microbial molecular mimicry and autoimmune responses. This approach is based on the relatively newly understood immunobiological effects of purinergic signaling (see the graphical abstract in Figure 1).
In 1955, Jerne postulated that immunoglobulins could react with any antigen except for host antigens (self-antigens) because these antibodies are supposed to be eliminated (via clonal selection) [23]. Building on this hypothesis, Burnet hypothesized in 1957, using the concept of clonal selection, that it is not autoreactive antibodies but rather autoreactive lymphocytes that are eliminated [24],[25].
Tregs are known to maintain self-tolerance by controlling autoreactive lymphocytes. They are essential for the prevention of autoimmune disorders [26],[27]. Currently, the mechanisms of self-tolerance and activation of autoimmune responses are known to be complex [16], as illustrated in Figure 2. Although autoreactive thymocytes (T cell precursors) with high autoreactive T cell receptor (TCR)-peptide-MHC affinity or peptide-MHC density [the major histocompatibility complex (MHC) in mammals corresponds to human leukocyte antigen (HLA) in humans] are clonally deleted in the thymus, autoreactive thymocytes with intermediate autoreactive TCR-peptide-MHC affinity or peptide-MHC density are diverted to Tregs [16] (Figure 2B). However, a substantial proportion of autoreactive thymocytes escape from clonal deletion in the thymic cortex and medulla by dodging thymic negative selection due to their dual αβ TCR expression [28]–[31]. Tregs suppress these autoreactive T cells to prevent the activation of autoimmune responses (Figure 2C) [16],[32]–[35]. Similar to autoreactive T cells, a proportion of autoreactive B cells also escape deletion in the bone marrow [36],[37]. This is probably due to altered BCR/TLR signaling through coreceptor B cell–activating factor receptor (BAFFR) and/or coreceptor CD40 because of the presence of a single-nucleotide polymorphism (C1858T; R620W) in protein tyrosine phosphatase nonreceptor type 22 (PTPN22R620W) [36],[37]. These autoreactive B cells give rise to plasma cells that produce autoantibodies [38],[39]. Fortunately, Treg suppression is not limited to autoreactive T cells. Additionally, autoreactive B cells and the production of autoantibodies—a sensitive indicator of the activation of autoimmune responses [40],[41]—are controlled by Tregs and by follicular regulatory T cells (Tfrs) [42],[43].
Self-antigen-specific Tregs are consistently induced in the thymus [16] and in secondary lymphoid organs [44],[45]. Notably, T cells need continuous TCR signaling to remain viable. When TCR signaling decreases because antigen levels decrease, such as during the contraction phase after an immune response, the cells undergo apoptosis. This also applies to Tregs. Self-antigens are structural parts of our body. It is obvious that the ability of Tregs to promote self-tolerance and protect against autoreactive T cells must have existed from the beginning of life. Because memory Tregs can escape from apoptosis during the contraction phase of an immune response, they do not need continuous TCR signaling to survive and do not proliferate. Thus, memory Tregs can persist for decades in infected peripheral sites or secondary lymphoid organs, ensuring durable maintenance of self-tolerance [46]–[49]. It is therefore essential that a significant proportion of Tregs are memory Tregs.
It makes sense that to specifically suppress autoreactive T cells, Treg TCRs must be able to recognize epitopes of self-antigens and hence must be autoreactive [50],[51]. At first glance, reports of Tregs specific to microbes (such as Mycobacterium vaccae [52], Leishmania major [53],[54], Candida albicans [55], Bordetella pertussis [56], and Helicobacter hepaticus [57]) and to alloantigens [58],[59] seem to challenge the self-reactive nature of Tregs. However, at a closer look, it appears that all these microbes [60]–[67] and alloantigens [68]–[70] harbor pentamer peptides that have a sufficient degree of similarity to human antigens to be recognized as self-antigens by the human immune system (molecular mimicry) [71]. This also applies to a report that used a naïve T cell hybridoma and a Treg hybridoma from transgenic mice with a very limited TCR repertoire (a single Ea52–68 peptide) and analyzed hundreds of TCRs derived from Tregs. That report concluded that there is evidence opposing the tendency of Tregs to self-react [72]. However, this finding is under scrutiny and is disputed because the reported self-reactivity of the T cell hybridoma and Treg hybridoma may be below the threshold of detection [73]. Therefore, it is still reasonable to believe that Tregs are truly autoreactive.
Tregs have several tools at their disposal to complete the important task of suppressing autoreactive T cells [74]. Tregs can secrete anti-inflammatory cytokines (IL-10, IL-35, and TGF-β) that inhibit autoreactive effector T cell function. Tregs can use cytotoxic serine proteases in their cytoplasmic granules to destroy autoreactive T cells (leading to apoptosis) by “drilling” a hole through the cell membrane using the enzyme perforin and delivering deadly content. In addition, Tregs can cause metabolic disruption by depriving autoreactive T cells of IL-2 and by hydrolyzing extracellular ATP to adenosine to suppress autoreactive T cell function. Tregs can also target DCs through the binding of their cytotoxic T lymphocyte–associated protein 4 (CTLA-4) to the CD80/CD86 receptor on DCs. This induces the production of immunosuppressive indoleamine 2,3-dioxygenase (IDO) by DCs. Finally, Tregs can inhibit the maturation and function of DCs through the interaction of immunosuppressive LAG-3 molecules on Tregs with membrane-bound MHC class II molecules on DCs [74]. It is clear that Treg tools are sufficiently powerful to destroy autoreactive T cells and effectively control the activation of autoimmune responses.
In addition to Tregs, Bregs also exist. Bregs differentiate from B cells and/or from immature B cells [75],[76], and some authors have reported that regulatory Tfh cells in patients with hepatitis B infection [77] and Tfrs in ARDS [78] induce the differentiation of Bregs. Tfrs are controlled by Tregs [42],[75]; thus, the loss of Tregs affects the number and function of Bregs. Bregs contribute to immune suppression by producing soluble anti-inflammatory cytokines (IL-10, TGF-β, IL-35, and adenosine) [75],[79]–[82]. Bregs express several immunosuppressive surface molecules, such as CD1d, programmed cell death protein ligand 1 (PD-L1) [83], Fas ligand (FasL or CD95L) [84], and even T cell immunoglobulin and mucin domain 1 (TIM-1), in selected populations of Bregs [85]. Additionally, Bregs can induce the expansion of Tregs and the inhibition of Th17 cells [86]–[88]. Bregs can also release cytotoxic serine proteases [granzyme B (GrB)] after cell-to-cell contact and perforin-mediated plasma membrane perforation. The anti-inflammatory mechanism of these GrB+ Bregs involves inhibiting the proliferation of CD4+ T cells [89],[90] and the activation of Th1 and Th17 cells [91].
Thus, Tregs and Bregs play crucial roles in maintaining self-tolerance. Because Tregs and Bregs produce and release the anti-inflammatory cytokines IL-10, IL-35, TGF-β, and adenosine, the proinflammatory activity of innate immune cells [neutrophils, mast cells, macrophages, natural killer (NK) cells, etc.] is also suppressed near Tregs and Bregs [92]–[94].
A key idea in self-tolerance research is that the protective effect of Tregs is not as robust as that of thymic clonal deletion. Although moderate antigen loads increase the number and suppressive function of Tregs [33], specific circumstances (such as repeated self-antigen exposure or high self-antigen loads) can lead to the escape of autoreactive T cells from normal suppression by Tregs [32]–[35]. This occurs via impairment of the suppressive function of Tregs rather than via reduced Treg numbers [32],[34],[35] (Figure 2D1), which inevitably leads to the activation of autoimmune responses. Conveniently, this activation results in the expression of autoantibodies [40],[41], which can be measured in clinical settings, as illustrated in a recent report on patients with moderate and severe traumatic brain injury (TBI) [95]. It is reasonable to assume that in these patients, high systemic levels of free self-antigens from damaged brain tissue are sufficient to hamper Treg suppressive function. That paper described the upregulation of IgM and IgG autoantibodies in the first week, and this change persisted for several years in the majority of patients. These autoantibodies were specific to self-antigens in brain tissue, such as myelin-associated glycopeptide (MAG), myelin basic protein (MBP), paraneoplastic Ma antigen 2 (PNMA2), tight junction protein 1 (TJP1), claudin 5 (CLDN5), zinc finger protein 397 (ZNF397), and selectin E (SELE). Additionally, autoantibodies to thyroid-specific thyroid stimulating hormone receptor (TSHR) [95] and autoantibodies to ubiquitous self-antigens, such as collagen type V alpha 2 chain (COL5A2), gamma-aminobutyric acid type A receptor subunit beta3 (GABRB3), and angiotensin I-converting enzyme (ACE), were observed. Autoantibodies in TBI patients have also been reported by other authors [96]–[98], and some authors have suggested that the presence of autoantibodies is associated with worse outcomes and may predict late post-TBI neurodegeneration [95],[97],[98]. A remarkable paper described the association of the presence of autoantibodies in the pituitary and hypothalamus with inflammation and persistent male hypogonadotropic hypogonadism after TBI [99]. Moreover, autoantibodies have been reported after spinal cord injury [100]–[102], and these autoantibodies are correlated with elevated serum proinflammatory cytokine levels [103],[104].
Overall, extensive (traumatic) tissue damage can induce autoimmune responses with increased autoantibody levels, presumably due to a high load of free self-antigens. Some microbes have reportedly developed the ability to mimic human self-antigens [71]. This provides them with immune camouflage when they enter the human body. In addition, high systemic levels of these antigens may also interfere with Treg suppressive function and activate autoimmune responses.
Microbes have developed a clever method to evade recognition by our adaptive immune system. Although many microbial antigens do not exhibit molecular mimicry, some antigens may exhibit such mimicry [71]. Available data suggest that invading microbes (such as viruses, bacteria, and fungi) that possess antigenic cross-reactivity with human self-antigens and human autoreactive TCRs and BCRs have the potential to disrupt the control of autoreactivity. A paper published in 2001 reported an interesting experiment in mice infected with herpes simplex virus type 1 strain KOS (HSV-1 KOS) [105]. The cause of corneal damage [herpes stromal keratitis (HSK)] after HSV-1 KOS infection was identified as the UL6 peptide of the virus. This protein exhibits molecular mimicry with mouse corneal self-antigens. The researchers generated a replication-competent HSV-1 KOS mutant with a single amino acid substitution in the UL6 peptide (UL6S309L). Although wild-type (WT) HSV-1 KOS infection induced HSK at a specific dose, mutant HSV-1 KOS infection induced HSK only at a 1000-fold greater dose. These high doses are not typically observed in nature. On day 5, when the virus was still present, the histopathology of the damaged cornea in mice infected with mutant and WT HSV-1 KOS was comparable. By day 10, when the virus was no longer present, the corneal pathology in mice infected with the mutant virus was clearly reduced, and the pathology resolved on day 15 in these mice. In contrast, the corneal pathology in mice infected with the WT virus became progressively worse, presumably due to the activation of autoimmune responses [105]. In addition, researchers have developed transgenic mice that generate monoclonal CD4+ T cells that exclusively recognize UL-6 (C1-6 mice) and mice that generate CD4+ T cells that exclusively recognize ovalbumin (OVA mice). WT HSV-1 KOS infection caused HSK in C1-6 mice but not in OVA mice. Thus, molecular mimicry appears to play a crucial role in the development of microbe-induced HSK [105].
If invading microbes harbor non-self-antigens (foreign antigens), the microbes are swiftly eliminated, and inflammation is limited (Figure 2A). Even if an invading microbe exhibits molecular mimicry, it is unlikely that all of its antigens are similar to human antigens (these antigens that differ from human antigens are regarded as foreign). A mixture of foreign antigens and antigens with molecular mimicry is more likely. Hence, even at low infection doses, these foreign antigens may induce a proinflammatory response that clears the pathogen and infected cells (Figure 2A). Presumably, this occurs without disturbing Tregs because, at low levels of antigens with molecular mimicry, autoreactive T cells remain under Treg suppression (Figure 2C). This is a clever mechanism that stops low-dose infection by microbes with molecular mimicry from evading human adaptive immune surveillance.
In contrast, repeated or high-dose infection with microbes harboring antigens that exhibit molecular mimicry simulates repeated exposure to self-antigens or exposure to a high load of self-antigens. In this case, the suppressive function of Treg is decreased, activating autoimmune responses [32]–[35] characterized by the production of autoantibodies [41],[43],[75],[106]–[109] (Figures 1 and 2D1). Hence, microbes harboring antigens with molecular mimicry cannot be eliminated easily. Autoreactive T cells must escape Treg suppression before they can attack the invading pathogen. I propose that this type of microbe can only be eliminated at the expense of self-tolerance.
Remarkably, this hypothesis corresponds with an extraordinary but long-forgotten finding that was reported in 1945 [110]–[113]. In rabbits, 7–14 days after a single injection of rabbit kidney emulsion plus Staphylococcus, a low incidence of proteinuria, casts and red cells in the urine, and serum autoantibodies to kidney tissue were observed. However, after repeated injections of the emulsion, the incidence of urinary changes corresponding to nephritis increased dramatically, reaching 100%.
Molecular mimicry of vaccine antigens has also been reported [114],[115], and the recent pandemic provides an example of this concept in medical practice. Reportedly, in 22 healthy individuals who received at least two SARS-CoV-2 mRNA–based vaccines, the number of SARS-CoV-2 spike-specific Tregs in the peripheral blood mononuclear cell population increased. In addition, these individuals developed SARS-CoV-2 spike-specific Treg memory cells [116]. Indeed, secondary infection can quickly activate memory Tregs and cause a rapid Treg response to prevent tissue damage caused by autoimmune responses [46],[47]. This observation is consistent with the finding that in many patients, autoreactivity (in the form of autoantibodies) was not observed after administration of the SARS-CoV-2 mRNA vaccine [117]. However, in other patients, autoantibody formation after SARS-CoV-2 vaccination (including mRNA-based vaccines) was observed [118]–[121]. In a few patients, this was accompanied by severe autoimmune symptoms such as thrombotic thrombocytopenia [119],[122] or pericarditis [123]–[125]. In addition, in patients with severe COVID-19, both increased Treg numbers [126]–[129] and reduced Treg numbers [130]–[134], including reduced SARS-CoV-2-reactive Treg numbers [135], have been reported.
Thus, vaccination with antigens or infection with microbes that exhibit molecular mimicry may prevent autoimmune responses in some patients but induce autoimmune responses in other patients. The available data suggest that autoimmune responses can be induced by repeated or high-dose microbe infection, by a high-dose of vaccine antigens or by vaccination shortly after microbial infection.
Microbes harboring molecular mimics can evade immunosurveillance at low antigen loads and can activate autoimmune responses after repeated exposure to antigens or at high antigen loads [9],[17]–[21],[136],[137]. However, the underlying mechanism is unclear. Recent advances in the study of purinergic signaling in the context of immunobiology have made it clear that purinergic receptors on immune cells play a pivotal role in the activation of autoimmune responses [138],[139]. This may partially explain the mechanism of microbe-induced autoimmune responses.
Adenosine and nucleotide molecules were discovered between 1929 and 1936 [140],[141]. In 1939, researchers attributed the ability to transport intracellular energy to ATP [142]–[145]. In 1948 and 1959, it was discovered that extracellular ATP functions differently from intracellular ATP, namely through intercellular signaling (communication between cells) [146],[147], which was later termed purinergic signaling. Geoffrey Burnstock was the first to describe purinergic co-transmission in neurons in 1972 [148]. Almost 20 years passed before the relevance of purinergic signaling became widely accepted [149],[150]. The ever-so-important P2X7R (initially named P2Z receptor) was identified and cloned by researchers in Geneva, Switzerland [151], and was first reported by researchers at the University of Ferrara, Italy, in 1995 [152].
Purinergic signaling is indispensable for intercellular signaling in mammals [150],[153],[154]. A total of four adenosine receptors (AdoRs), seven P2X purinergic receptors (P2XRs), and eight P2Y purinergic receptors (P2YRs) have been identified [155]–[159] (Table 1). These receptors are expressed in almost every cell in the body. The effect of the activation of purinergic receptors depends, among other factors, on the type of receptor activated and the type of cell where the receptor is located [154]. For example, P2X7Rs play a key role in the physiological functions of several organs, such as electrical signaling in the central and peripheral nervous systems [160],[161], surfactant release in the lungs [162]–[164], mucin production in the airways [165], and insulin release in pancreatic islet beta cells [166],[167]. This paper focuses on the effect of the activation of purinergic signaling in immune cells, particularly the effect of the activation of P2X7Rs in the immune system.
Purinergic signaling, which involves all related ligands (ATP, ADP, UTP, adenosine, etc.) and all related receptors except P2X6Rs, is crucial for the regulation of inflammation [22],[168]–[172]. Some receptors are involved in proinflammatory responses, some are involved in anti-inflammatory effects, and others may be involved in both responses [22],[168]–[172]. For example, the activation of AdoRs by adenosine may promote an immune response in some cells; however, in general, it suppresses the activation of immune responses (Table 2, rows 1–3). In contrast, P2X7Rs are not involved in anti-inflammatory responses, and they stand out from other purinergic receptors [22],[152],[171],[172].
Purinergic receptors and their ligands [155]–[159] | |
Natural ligands | Receptors |
Adenosine | AdoRA1, AdoRA2A, AdoRA2B, and AdoR3 |
ATP | P2X1R, P2X2R, P2X3R, P2X4R, P2X5R, P2X7R, and P2Y11R |
ADP | P2Y12R |
ADP and ATP (ADP > ATP) | P2Y1R and P2Y13R |
UDP, UTP, and ATP (UDP > UTP >> ATP) | P2Y6R |
UDP | P2Y14R |
UTP and ATP (UTP > ATP) | P2Y2R and P2Y4R |
ATP | P2X6R molecules are mostly retained in the endoplasmic reticulum. They do not exit the endoplasmic reticulum as a homopolymer but do so as a heteropolymer (with P2X2R and/or P2X4R) or after enhanced glycosylation-induced improved trafficking of P2X6R homopolymers. |
After exiting the endoplasmic reticulum and becoming inserted into the cell membrane, P2X6R can be activated by extracellular ATP. |
Examples of the effects of extracellular adenosine, ATP, and ADP on select immune cells through the activation of three purinergic receptors (AdoRA2A, P2X7R, and P2Y12R) |
|||||
Row number | Receptor | Ligand [149] | Immune cell type or experimental model | Results of receptor signaling | Reference number |
1 | AdorA2A | CGS-21680 | Mouse naïve T cells | Promotes differentiation toward CD4+FoxP3+Lag3+ Tregs, inhibits Th1 and Th17 differentiation, inhibits IL-6 secretion, and increases TGF-β secretion. | [173] |
2 | CGS-21680 and NECA | Mouse CD4+CD25+FoxP3+ Tregs | Increases the number of Tregs and increases CTLA-4 receptor expression. Upregulates expression of the ectoenzymes CD39 and CD73, accelerating adenosine generation from extracellular ATP. | [174] | |
3 | Adenosine | Human CD4+CD25+CD127low/–Tregs and CD8+ T cells | Tregs from gastric cancer patients hydrolyze ATP to generate adenosine. Adenosine synthesized by Tregs promotes apoptosis and suppresses the proliferation of CD8+ T cells. Tregs reduce CD8+ T cell activity by promoting cAMP synthesis. Tregs inhibit the immune function of CD8+ T cells through the AdoRA2A pathway. | [175] | |
4 | P2X7R | Inactivated state in the absence of ATP | Macrophages and P2X7R-transfected HEK-293 cells | P2X7 is a scavenger receptor for apoptotic cells in the absence of its ligand ATP. | [176],[177] |
5 | P2X7R | ATP-release channel | Alveolar epithelial type I cells (AT I cells), murine osteoclast cells, murine neuroblastoma cells, astrocytic cell line, murine astrocytes, and B16 melanoma cells | Release ATP after mechanical deformation (AT I cells), spontaneously (osteoblast cells), after activation (neuroblastoma cells, astrocytic cell line), and after γ irradiation (melanoma cells). | [178]–[183] |
6 | Mouse 3T3 fibroblasts | P2X7R-mediated ATP secretion is accompanied by depletion of cytosolic ATP. | [184] | ||
7 | Bone marrow–derived DCs from WT mice and Panx1−/− C57BL/6 mice | Upon stimulation of the P2X7 receptor by ATP, Panx-1 contributes to rapid DC motility by increasing the permeability of the plasma membrane, which results in supplementary ATP release. | [185] | ||
8 | Murine macrophages and RAW 264.7 macrophages | Infection with Leishmania donovani substantially increases extracellular release of ATP. | [186] | ||
9 | A549 alveolar epithelial cells | Infection with human rhinovirus RV-16 increases basal extracellular ATP release and ATP release after a second stimulation by hypotonic and isotonic solutions. | [187] | ||
10 | Mouse influenza model | Influenza infection increases plasma ATP levels 3-fold and pulmonary ATP levels 5-fold. | [188] | ||
11 | RAW 264.7 macrophages and L929 fibroblasts | Infection with vesicular stomatitis virus (VSV) increases extracellular ATP in a time-dependent manner. | [189] | ||
12 | P2X7R | ATP, low tonic basal activation | HEK 293 and HELA cells | Increases mitochondrial calcium level, membrane potential, and cellular ATP levels, and promotes serum-independent growth. This process requires an intact pore-forming function. | [190] |
13 | In vitro scratch wound assay with HaCat cells (human skin keratinocytes) | Medium hyaluronan fragment (MMW-HA, between 100 and 300 kD) increases expression of the tight junction protein ZO-1 and induces a low activation of the P2X7 receptor, resulting in improved closure of the wound. This is accompanied by pore formation, as shown with Yo-Pro-1 cellular uptake. The P2X7R antagonist brilliant blue G (BBG) completely inhibits this process. | [191] | ||
14 | HEK293 and NIH3T3 cells | Increases the Ca2+ content of the endoplasmic reticulum, activates NFATc1, and protects from apoptosis. | [192] | ||
15 | PC3 cells LNCaP, Kelly, RPMI-8226, DU145, and SK-MEL-5 cells | Drives the expression of nfP2X7, a key mediator of cell survival. | [193] | ||
16 | Human osteoclast-like cells | Promotes an increase in extracellular adenosine concentrations. | [194] | ||
17 | HEK293 cells | Initiates anaerobic glycolysis independent of the oxygen content. Upregulates glucose transporter Glut1 (thus enhancing intracellular glycogen stores). Upregulates glycolytic enzymes (PFK, G3PDH, PKM2), phosphorylated Akt/PKB, and hypoxia-inducible factor 1a (HIF-1a) expression. Impedes the Krebs cycle independent of oxygen concentrations by promoting pyruvate dehydrogenase kinase 1 (PDHK1) and inhibiting pyruvate dehydrogenase (PDH, conversion of pyruvate to acetyl-CoA). | [195] | ||
18 | P2X7R | ATP ≥ 100 µM | C57BL/6 mice | P2X7 activation inhibits the suppressive potential and stability of Tregs and promotes the conversion of Tregs to Th17 cells in vivo. In contrast, P2X7R inhibition promotes the conversion of CD4+ T cells into Tregs after stimulation of their T cell receptors (TCRs). | [196] |
19 | C57BL/6 wild type and P2X7 knockout mice | P2X7 knockout mice show an increase in the number of CD90/CD45RBlow FoxP3+ Tregs in the colon lamina propria, with prevention of Treg death in mesenteric lymph nodes, and these Tregs produce more IL-10. Colitis is prevented or reduced in P2X7 knockout mice. Treg cells lacking the P2X7 receptor have higher levels of integrin CD103. ATP activation of P2X7R triggers Treg death. | [197] | ||
20 | C57BL/6 mice, renal ischemia–reperfusion injury | P2X7R antagonist oATP prior to renal ischemia increases renal Foxp3DCD4D Treg infiltration and reduces IL-6 and CCL2 levels. | |||
oATP treatment following injury improves renal function, decreases the infiltration of innate and adaptive effector cells, increases the renal infiltration of Foxp3+CD4+ Tregs, increases tubular cell proliferation, and reduces renal fibrosis. | [198] | ||||
21 | C57BL/6 mice | P2X7R activation reduces the frequency of Tregs, and P2X7R inhibition increases the expansion of Tregs. | [198] | ||
22 | CD4+CD25+FoxP3+ Tregs | Facilitates NAD+-induced Treg depletion through the ART2-P2X7 pathway. | [199] | ||
23 | Humanized mice model GVHD, HaCat cell, Jurkat cells (E6-1 clone), and mouse DCs | Increases CD80, CD 86, STAT-1, and P2X7R expression; IFN-β release; and T cell expansion. Reduces Treg numbers. | [200] | ||
24 | Humanized mice model GVHD | Anti-hP2X7 monoclonal antibodies increase human Treg and human NK cell numbers on Day 21 and reduce clinical and histological GVHD in the liver and lung compared to the control treatment at the disease endpoint. | [201] | ||
25 | LGMDR3 (Limb-girdle muscular dystrophy R3) mice with α-sarcoglycan gene (SGCA) knockout | P2X7 antagonist A438079 improves mouse motor function and decreases serum creatine kinase levels, reduces the percentage of central nuclei, reduces fiber size variability, and reduces the extent of local fibrosis and inflammation significantly. Flow cytometric characterization of muscle inflammatory infiltrates revealed significantly decreased numbers of innate immune cells and increased numbers of Tregs. | [202] | ||
26 | Mouse CD4+CD25+FoxP3+ Tregs | P2X7R activation facilitates NAD+-induced Treg depletion through the ART2-P2X7 pathway. | [199] | ||
27 | BALB/c mice GVHD model, DCs | Activation of P2X7Rs increases CD80, CD 86, STAT-1, and P2X7R expression, IFN-β release, and effector T cell expansion. Reduces Treg numbers. | |||
P2X7R blockade in GVHD improves survival. | [200] | ||||
28 | Mouse, human, murine B cells | Induces shedding of IgE receptor (CD23) and CXCL16. Soluble CD23 sustains growth of B cell precursors, promotes B cell and T cell differentiation, and drives cytokine release from monocytes. CXCL16 is a chemoattractant for lymphocytes. | [203],[204] | ||
29 | Jurkat cells (E6-1 clone) Naïve T cells | TCR stimulation triggers rapid release of ATP and upregulates P2X7 gene expression. Autocrine ATP stimulation through the P2X7R is required for TCR-mediated calcium influx, NFAT activation, and IL-2 production. | [205] | ||
30 | Wild-type and Panx-1 knockout C57BL/6 mice, bone marrow–derived DCs | ATP promotes the rapid migration of DCs through the activity of pannexin 1 channels and P2X7 receptors. | [185] | ||
31 | Mouse and P2X7 knockout mice, CD11c+CD103+ DCs | Mediates rapid infection-induced recruitment of CD11c+CD103+ DC subsets into the epithelial layer of the gut. | [206] | ||
32 | DCs cultured from murine bone marrow precursor cells | Induces autocrine-mediated (pannexin-1 channels) rapid migration of DCs through reorganization of the actin cytoskeleton. | [185] | ||
33 | Human monocytes | Induces MMP-9 and TIMP-1 release. | [207] | ||
34 | Wild-type and P2X7 knockout C57BL/6 mice, M1 macrophages | Induces release of 74 proinflammatory proteins detected by an antibody array and 33 inflammatory proteins detected by LC–MS/MS. | [208] | ||
35 | Wild-type and P2X7 knockout C57BL/6 mice M2 macrophages | Induces release of 21 anti-inflammatory proteins detected by LC–MS/MS. | [208] | ||
36 | CLP-induced sepsis in mice, macrophages | Enhances intracellular bacterial killing. | [209] | ||
37 | Macrophages and P2X7R-transfected HEK-293 cells | Mediates rapid uptake of beads and bacteria in the absence of serum after ATP activation. | |||
38 | Human mast cells | Induces degranulation. | [210] | ||
39 | Wild-type and P2X7 knockout C57BL/6 mice, naïve NKTs | Facilitates NAD+-induced inhibitory signaling through the ART2-P2X7 pathway, resulting in nonfunctional NKTs. | [211] | ||
40 | Wild-type and P2X7 knockout C57BL/6 mice, activated NKTs | Facilitates NAD+-induced stimulatory signaling through the ART2-P2X7 pathway, resulting in functional NKTs with increased IFN-γ and IL-4 release. | [211] | ||
41 | Human peripheral neutrophils | Human CAP18/LL-37 suppresses neutrophil apoptosis through the activation of formyl-peptide receptor-like 1 and P2X7R. | [212] | ||
42 | P2X7R | ATP > 1 mM, prolonged vigorous activation | Macrophages, HeLa cells, 1321N1 astrocytes, and HEK293 cells | Induces Panx-1-mediated large pore formation and interleukin-1 beta release. | [213] |
43 | Human neutrophils and HL-60 cells | Mediates large pore formation and superoxide generation. | [214] | ||
44 | Female C57BL/6 (B6) and BALB/c mice, ART2 knockout BALB/c mice, P2X7 knockout C57BL/6 mice, mature peripheral T cells | High-dose ATP promotes apoptosis, cell death, and CD62L shedding (homing receptor for central T cells) independent of the NAD+-induced ART2-P2X7 pathway. | [215]–[217] | ||
45 | J774 cells and HEK cells expressing the P2X7 receptor | Promotes the formation of pores permeable to very large ions, leading to cytolysis | [151] | ||
46 | P2Y12R | ADP > ATP | Murine model of sepsis, cecal ligation, and puncture (CLP). Cocultures of human platelets and T cells with or without anti-CD3/CD28 | Blockade of the P2Y12 signaling pathway restrains Treg proliferation in vivo and in vitro. | [218],[219] |
In general, P2X7Rs have several functions, and they are all present in immune cells. First, P2X7Rs expressed on the surface of monocytes and macrophages act as scavenger receptors for apoptotic cells by effectively enhancing their engulfment (Table 2, row 4). P2X7Rs are indispensable for cytoskeletal rearrangement [220] and membrane blebbing [221] during the process of phagocytosis [222]. Second, P2X7Rs act as ATP release channels after mechanical deformation, after irradiation, after activation, after stimulation with isotonic or hypotonic solutions, after stimulation by microbe-infected cells, or spontaneously, often in conjunction with pannexin 1 channels (Panx-1s) (Table 2, rows 5–11). Third, P2X7Rs act as promotors of cell proliferation—referred to as low tonic basal activation—in cells with high expression of P2X7Rs, such as cells involved in wound closure or cancer (Table 2, rows 12–17). Fourth, P2X7Rs act as intrinsic cation channels that transduce transmembrane signals, inciting a proinflammatory response in innate and adaptive immune cells [149],[223] (Table 2, rows 18–41). Finally, P2X7Rs act as promoters of cell death by promoting the formation of macropore channels (Table 2, rows 42–45).
Extracellular levels of purinergic signaling ligands are very low under resting conditions (Figure 3A). After release, extracellular ATP molecules are hydrolyzed by membrane-bound ectonucleotidases or extracellular soluble nucleotidases (CD39, CD73, tissue nonspecific alkaline phosphatase, etc.), generating extracellular ADP, AMP, adenosine, inosine, and other metabolites and returning their extracellular levels to those observed under resting conditions (Figures 3A and 4) [168]–[170]. This process, referred to as resensitization, is essential for purinergicreceptors to recover from desensitization following receptor activation, during which the receptors are unresponsive to stimuli. To a certain extent, desensitization occurs after every activation, and time is required to return to a state of complete resensitization after ligand clearance [224]–[229]. The resensitization time is dependent on, among other factors, the extent of activation. Specifically, the higher the activation level is, the longer the time required for complete resensitization to occur [227],[229] (orange boxes at the bottom of the graph in Figure 3).
Microbial infection is linked to purinergic signaling, as it increases extracellular ATP release through P2X7R combined with Panx-1 as an ATP release channel [186]–[189]. In experimental virus-infected cells, extracellular ATP concentrations and activation of P2X7Rs increase with increasing exposure time and microbe concentration [189]. Extracellular ATP hydrolysis (Figure 4) causes extracellular ADP, AMP, and adenosine levels to increase. The subsequent activation of P2Rs (P2XRs and P2YRs) and AdoRs initiates the proinflammatory immune response to counter-invading microbes (Figure 3B). However, the levels of extracellular ligands required to activate P2Rs (other than P2X7Rs) and AdoRs are quite modest [228],[230],[231]. The activation of P2X7R, an intrinsic cation channel that activates a proinflammatory response, is initiated at extracellular ATP levels that are at least 10-fold or greater than the ligand concentrations observed to activate other P2Rs [223],[226],[230]. The EC50 (the effective concentration 50, defined as the level required for the ligand to reach effective receptor activation halfway between maximal and baseline receptor activation) of AdoRs for adenosine ranges from 1 nM to 1.4 µM [228],[232], and the EC50 of P2Rs for ATP, ADP, or UTP ranges from 0.01 µM to 10 µM [155]–[159],[230]–[232]. In contrast, the activation of P2X7R is initiated at 10-fold or greater levels of extracellular ATP (≥100 µM, with an EC50 > 1 mM) [223],[226],[230] (Figures 3B and C). Thus, the P2X7R-induced proinflammatory response occurs only after a certain dose of microbes is exceeded, as occurs in repeated or severe infection (Figures 3C and D).
As summarized in Table 2 (rows 1–3 and 46), activation of AdoRA2A or P2Y12R may increase the number and suppressive function of Tregs. P2X7Rs are highly expressed in Tregs and dendritic cells (DCs) [196],[197],[199],[200]. In contrast to the activation of AdoRA2A and P2Y12R, activation of P2X7Rs causes changes in T cell balance, promoting effector T cell expansion and Treg contraction [196],[197],[199],[200]. Crucially, the disruption of Tregs occurs exclusively after the activation of P2X7Rs on DCs, Tregs, and other immune cells and not via the activation of any other purinergic receptor [22],[162]. The mechanisms by which P2X7R activation affects Tregs and DCs are presented in Table 2, rows 18–27 and 30–32, respectively. As shown in Figure 3C, P2X7Rs are activated only after high levels of extracellular ATP are reached. Activated P2X7Rs disrupt Treg suppressive function, allowing autoreactive T cells to be activated and attack invading microbes exhibiting molecular mimicry at the expense of self-tolerance. This is highlighted by the finding that although P2X7R is not required to control lung tuberculosis after aerosol infection with a low dose (~50 CFU) of M. tuberculosis (Erdman strain) in P2X7 knockout mice [233], P2X7R blockade can significantly reduce lung inflammation in WT mice intratracheally infected with a high-dose (~100 CFU) of the M. bovis MP287/03 strain in a model of severe lung tuberculosis [234].
Thus, available data suggest that the activation of purinergic receptors on immune cells activates inflammation at moderate extracellular levels of their ligands. However, the activation of P2X7Rs on immune cells, which interferes with Treg suppression of autoreactive T cells, occurs at much higher extracellular ATP levels.
Immunization with type II collagen (CII) in mice induces arthritis with IgG autoantibodies specific to CII. Intraperitoneal treatment with the P2X7R antagonist oxidized ATP (oATP) significantly decreases CII-specific IgG autoantibody levels and improves clinical outcomes [235]. Other researchers reported that immunization with a plasmid containing the M2 receptor (M2AChR) cDNA sequence induces dilated cardiomyopathy with autoantibodies to M2AChR in P2X7 knockout and WT mice. The autoantibody levels in P2X7 knockout mice are significantly lower than those in WT mice [236]. Additionally, in Sjogren's syndrome, the expression and activation of the P2X7R inflammasome axis in human salivary glands and human circulating lymphomonocytes are greater in autoantibody-positive patients than in autoantibody-negative patients [237],[238].
Infections caused by microbes that contain foreign antigens (antigens without molecular mimicry) that can be eliminated rapidly are associated with modest increases in extracellular adenosine and nucleotide levels. In these infections, all of the purinergic receptors required for an adequate immune response, except P2X7Rs, are activated (Figure 3B). However, infections caused by microbes that exhibit molecular mimicry (these microbes also harbor foreign antigens) will initially increase Treg numbers and activation [33], delaying the (auto) immune response against their antigens. When the immune response against foreign antigens is not sufficiently effective, microbes proliferate extensively. I believe that this causes an increase in ATP release and extracellular ATP levels above the threshold that allows P2X7Rs to become activated in addition to P2XRs, P2YRs, and AdoRs, hampering the ability of Tregs to suppress autoreactive T cells and B cells (Figure 3C). A faster autoimmune response is expected after repeated doses or under high-dose infection (associated with greater ATP release) with microbes that exhibit molecular mimicry. If the proinflammatory process is not resolved and severe inflammation occurs, extracellular ATP levels further increase, causing prolonged vigorous activation of P2X7Rs. This inevitably leads to macropore formation and cytolysis, with concomitant uncontrolled ATP release (Table 2, rows 42–45) [151],[172],[213],[214]. All immune cells are affected by cytolysis, except for cytotoxic T cells (CTLs), which include autoreactive CTLs, because CTLs are resistant to extracellular ATP-induced cytolysis [239], possibly due to the very high expression and activity of ATP-hydrolyzing nucleotidases on the CTL membrane [240]. This results in a state known as hyperinflammation, with maximally activated autoreactive CTLs and abundant cytokine production (cytokine storm, Figure 3D) [22],[241],[242]. Hyperinflammation is a potentially life-threatening condition. However, even hyperinflammation may resolve spontaneously. At the macropore-formation stage, P2X7R-dependent release of matrix metalloproteinase 2 (MMP-2) occurs, which can control runaway P2X7Rs by proteolytic degradation of P2X7Rs [243],[244].
As mentioned above, one intriguing phenomenon certainly deserves more attention: desensitization. At very high extracellular adenosine and nucleotide concentrations, purinergic receptors are likely to become unresponsive to stimulation by their ligands. An even more fascinating finding is that all purinergic receptors except for P2X7Rs are subject to desensitization [223],[226],[230] (Figure 3D). At very high extracellular adenosine and nucleotide concentrations, all immune responses except for autoimmune responses are paralyzed. Thus, there is simultaneous immunosuppression (against foreign antigens) and strong activation of autoimmune responses. During severe microbial infection, ATP is released beyond the capacity of extracellular hydrolyzing nucleotidases to clear it (as depicted in Figure 4), after which extracellular ATP accumulates [245] alongside elevated extracellular levels of adenosine and other nucleotides. This results in hyperinflammation, with highly activated P2X7Rs, disruption of many immune cells (except CTLs), cytokine storm, and desensitization of other purinergic receptors, which interferes with normal immune responses to microbes [22]. Hence, during hyperinflammation, the host is more susceptible to invading microbes [22],[163] (Figure 3D). I hypothesize that the desensitization of purinergic receptors on immune cells may be the underlying mechanism of secondary immune paralysis in severe diseases. For example, sepsis-induced immunosuppression [246],[247] is also known as compensatory anti-inflammatory response syndrome (CARS) in critically ill patients [248],[249].
Recently, a study reported that the blood levels of Tregs, Bregs, and autoantibodies to type I IFNs in COVID-19 patients requiring high-flow oxygen therapy or noninvasive ventilation are significantly greater than those in nonhospitalized COVID-19 patients and healthy controls. The increased Treg and Breg levels are even greater in those positive for autoantibodies to type I IFNs [129]. This phenomenon can be interpreted as the simultaneous activation of proinflammatory immune responses, autoimmune responses, and tolerogenic responses. It is quite challenging to explain this phenomenon from the perspective of immunobiology. However, when purinergic signaling is involved, we can deduce that ATP, ADP, and adenosine levels are sufficiently high to activate all purinergic receptors, including P2X7Rs, and can still achieve adequate resensitization. In this scenario, there is simultaneous activation of anti-inflammatory responses (by activated AdoRA2As and P2Y12Rs) that increase Treg numbers and activation of proinflammatory responses (against foreign antigens) fueled by the activation of other P2XRs, P2YRs, and AdoRs. In addition, the activation of P2X7Rs in immune cells hampers Treg suppressive function, leading to the escape of autoreactive T cells and B cells from Treg suppression (Figure 3C), which initiates, among other processes, the production of autoantibodies despite the increased numbers of Tregs. Thus, Treg numbers and autoantibody levels are expected to increase with increasing extracellular ATP, ADP, and adenosine levels.
In contrast, other researchers reported exactly the opposite results. In one study, healthy controls were not included, and autoantibody levels were not determined. The lack of autoantibody levels makes it difficult to assess whether Treg suppressive function is affected. The blood Treg numbers in patients who did not require oxygen therapy (those with mild disease) were slightly but still significantly greater than those of patients who required oxygen via a nasal cannula or oxygen mask (those with moderate disease). However, blood Treg numbers in patients requiring noninvasive or invasive mechanical ventilation (those with severe disease) were far lower than those in patients with moderate or mild disease [134]. Hypothetically, from the perspective of purinergic signaling, we can deduce that the activation of purinergic receptors in patients with mild and moderate disease in this cohort corresponds to the mechanism presented in Figure 3C. In patients with severe disease who are undergoing mechanical ventilation, it corresponds to a state of hyperinflammation where Treg numbers are affected by ATP-induced macropore formation and cytolysis, as depicted in Figure 3D.
On the one hand, in the case of disruption of Tregs with TCRs matching ubiquitous antigens, we would expect that symptoms would be generalized. On the other hand, in the case of tissue-restricted antigens (TRAs), symptoms are expected to be confined to certain tissues or organs. However, in reality, this is often not true; autoimmune disorders share many common symptoms, such as headache [250],[251], pruritus [252], sleep disturbances [253], fatigue [253],[254], pain [255], chronic pain [256], and neuropsychiatric symptoms [257].
There are two explanations for the common symptoms of autoimmune disorders. First, P2X7Rs are expressed on all Tregs [196],[197],[199],[200]; therefore, activation of these receptors disrupts the suppressive function of Tregs in general, irrespective of whether their TCRs are specific to microbes that mimic self-antigens. This leads to autoimmune responses against self-antigens in multiple tissues or organs. This phenomenon may be the basis of the well-known “bystander T cell activation” phenomenon in which T cells that are not specific to disease-related antigens damage host cells without proper antigen recognition [258]–[262]. Second, according to bioinformatics analysis, microbes tend to exhibit molecular mimicry with multiple human antigens rather than a single antigen [263],[264]. An interesting article reported that after severe COVID-19, the generated antibodies include autoantibodies, specific antibodies to the SARS-CoV-2 spike protein receptor-binding domain, and other nonspecific antibodies. Remarkably, the profile of autoreactive antibodies in critically ill patients with COVID-19 was very comparable to that in patients with acute respiratory distress syndrome (ARDS) following bacterial pneumonia in the ICU with similar self-antigen targets, such as antinuclear antibodies (ANAs) and anti-carbamylated protein responses (anti-CarPs). Researchers have even observed an overlap with autoantibodies found in patients with systemic lupus erythematosus (SLE) [265].
Thus, undermining the suppressive function of Tregs can easily promote the escape of autoreactive T cells and B cells specific to multiple antigens. This clearly affects multiple tissues or organs with concomitant production of autoantibodies to more than one antigen [106]. However, critically, not all microbial infections lead to immediate and severe autoimmune responses; some microbes are able to efficiently suppress autoimmune responses.
Although the activation of AdoRs by adenosine may promote an immune response in some immune cells, in general, AdoR activation reduces the number and inhibits the functions of DCs, T cells, B cells, antibody-producing plasma cells, macrophages, neutrophils, and NK cells [266]. In addition, activation of AdoRA2A increases the number and promotes the suppressive function of Tregs (Table 2, rows 1–3). Microbes, after invading the body, can reduce the extracellular concentration of ATP by converting ATP to adenosine to such an extent that the activation of P2X7Rs in immune cells may not occur. The “advantage” for microbes is that the resulting increase in extracellular adenosine levels restores the number and suppressive function of Tregs, mitigating autoimmune responses; however, for the host, this occurs at the expense of defense against these microbes (Table 2, rows 1–3 and Figure 2D2). It is therefore not a coincidence that, in patients infected with certain viruses that exhibit molecular mimicry, such as Epstein–Barr virus (EBV), human immunodeficiency virus (HIV), and hepatitis C virus (HCV) [5], the expression of the ATP-hydrolyzing enzyme CD39 (Figure 4) is upregulated in infected cells, Tregs, and other immune cells [267]–[270]. Subsequently, activation of AdoRA2A by adenosine increases Treg number and suppressive function. This mitigates autoimmune responses, resulting in a chronic condition with much less severe inflammation, such as chronic EBV infection [271], chronic HIV infection [272], and chronic HCV infection [273].
In severe infection with EBV, HIV, or HCV, a further complication develops. Presumably, ATP release [178],[180],[185] combined with upregulated CD39 expression reduce extracellular ATP concentrations, preventing P2X7R activation and converting extracellular ATP to adenosine. Because ATP release does not depend on extracellular ATP-induced P2X7R activity but rather is driven by microbial infection [186]–[189], in severe infection, high ATP release combined with upregulated ATP-hydrolyzing nucleoside activities strongly decrease ATP levels and further increase high extracellular adenosine levels [162],[163]. This potentially increases the risk of organ fibrosis [274],[275], and organ fibrosis has been reported in patients with chronic EBV [276], HIV [277], and HCV [278],[279] infection.
In short, I propose that by employing this strategy, microbes can survive and proliferate in the body for quite a long time, causing chronic inflammatory disease and increasing the risk of fibrosis without triggering severe autoimmune responses.
Blocking P2X7Rs on DCs and Tregs is an obvious next step in treating autoimmune disorders. However, to ameliorate the symptoms of autoimmune responses, P2X7R antagonists must reach adequate effective concentrations in secondary lymphoid organs, where DC-activated T cells and Tregs are induced. P2X7R activation is indispensable in many organs (the central and peripheral nervous system [160],[161], the lung [162]–[164], the airways [165], the pancreas [166],[167], etc.). Consequently, traditional approaches involving oral or intravenous administration of P2X7R antagonists cannot safely achieve adequate concentrations without disrupting essential organ functions. Indeed, despite effective ex vivo antagonism of P2X7Rs in immune cells, clinical trials have failed to demonstrate the efficacy of the P2X7R antagonists CE-224,535 (Pfizer) [280] and AZD-9056 (AstraZeneca) [281] in the treatment of rheumatoid arthritis and major depressive disorder under JNJ-54175446 (Johnson and Johnson) [282]. Apparently, the safe serum levels of these antagonists are not high enough to produce effective P2X7R inhibition of Tregs in secondary lymphoid organs. This is referred to as the very narrow therapeutic index (NTI, a narrow window between effective doses and doses causing adverse toxic effects), which is a common and difficult to overcome problem in drug development [283]. In contrast, oral AZD9056 significantly improved the Crohn's Disease Activity Index (CDAI) in patients with Crohn's disease [284]. I propose that this success can theoretically be ascribed to the effect of high local concentrations of AZD9056 in tertiary lymphoid organs in the gut, where the drug enters the mucosa, enabling effective antagonism of P2X7Rs on local immune cells involved in the pathogenesis of Crohn's disease [285].
In this review, I discuss the regulation of autoimmunity in relation to self-antigens and antigens of microbes with molecular mimicry. Under certain conditions, microbes with molecular mimicry are able to activate autoimmune responses. In addition, I have explained the mechanism of the activation of autoimmune responses by microbes with molecular mimicry using the knowledge of purinergic signaling. I found that P2X7Rs on immune cells, especially on Tregs and DCs, play a critical role by affecting the suppressive function of Tregs followed by the activation of autoimmune responses. Previously, it was thought that microbes had to reach target organs and enter cells to damage organs. However, since the activation of autoimmune responses occurs almost exclusively in the secondary lymphoid organs, it appears that it is sufficient for microbes with molecular mimicry to reach the lymph nodes to initiate autoimmune responses. The resulting autoreactive T cells that escape Treg suppression migrate via systemic circulation and cause organ damage throughout the body. As a result, depending on the mimicked self-antigens, one or more organs are affected. Consequently, the elimination of microbes with molecular mimicry occurs at the expense of self-tolerance (Figure 1).
I have also proposed that some types of microbes that express molecular mimicry, by exploiting the purinergic conversion of extracellular ATP to the anti-inflammatory adenosine, may mitigate autoimmune responses at the expense of the ability to clear microbes. This may be the basis for the development of microbe-induced chronic diseases.
As mentioned in the Introduction section of this review, autoimmune disorders resulting from the activation of autoimmune responses have a major impact on people's daily lives and public health. In addition, their rapidly increasing prevalence contributes to rising healthcare costs. Therefore, effective treatments are urgently needed. However, the development of effective treatments for autoimmune disorders is challenging due to their NTIs. This review provides an indication for targeting secondary lymphoid organs (such as local lymph nodes) with a P2X7R antagonist. I believe it is not necessary to target all lymph nodes. The drug dose required to effectively inhibit the P2X7Rs of immune cells in a small volume of local lymph nodes is relatively low. When the drug reaches systemic circulation, it is highly diluted to very low concentrations. This prevents systemic P2X7R inhibition and other adverse effects. As suggested previously [22], I hypothesize that targeting local lymph nodes with a P2X7R antagonist can increase the production and suppressive function of Tregs. These Tregs migrate through the systemic circulation to suppress autoreactive T cells and B cells throughout the body.
In my opinion, this review differs from reviews in the field of microbe-induced autoimmunity because of the involvement of purinergic signaling. This allows me to elucidate biological processes that would otherwise be nearly impossible to explain. In addition, this approach provides an opportunity to explore new avenues in the field of microbe-induced autoimmunity.
The author declares that he did not use Artificial Intelligence (AI) tools in the preparation of this article.
[1] |
Miller FW (2023) The increasing prevalence of autoimmunity and autoimmune diseases: an urgent call to action for improved understanding, diagnosis, treatment, and prevention. Curr Opin Immunol 80: 102266. https://doi.org/10.1016/j.coi.2022.102266 ![]() |
[2] | Goldhaft TM, Wernicoff N (1948) High mortality associated with a widespread outbreak of Newcastle disease. Cornell Vet 38: 181-185. |
[3] |
Nelson CB, Pomeroy BS, Schrall K, et al. (1952) An outbreak of conjunctivitis due to Newcastle disease virus (NDV) occurring in poultry workers. Am J Public Health Nations Health 42: 672-678. https://doi.org/10.2105/AJPH.42.6.672 ![]() |
[4] |
Trott DG, Pilsworth R (1965) Outbreaks of conjunctivitis due to the Newcastle disease virus among workers in chicken-broiler factories. Br Med J 2: 1514-1517. https://doi.org/10.1136/bmj.2.5477.1514 ![]() |
[5] |
Rojas M, Restrepo-Jiménez P, Monsalve DM, et al. (2018) Molecular mimicry and autoimmunity. J Autoimmun 95: 100-123. https://doi.org/10.1016/j.jaut.2018.10.012 ![]() |
[6] |
Suliman BA (2024) Potential clinical implications of molecular mimicry-induced autoimmunity. Immun Inflamm Dis 12: e1178. https://doi.org/10.1002/iid3.1178 ![]() |
[7] |
Kanduc D, Shoenfeld Y (2020) Medical, genomic, and evolutionary aspects of the peptide Sharing between Pathogens, Primates, and Humans. Glob Med Genet 7: 64-67. https://doi.org/10.1055/s-0040-1716334 ![]() |
[8] |
Oldstone MB (2014) Molecular mimicry: its evolution from concept to mechanism as a cause of autoimmune diseases. Monoclon Antib Immunodiagn Immunother 33: 158-165. https://doi.org/10.1089/mab.2013.0090 ![]() |
[9] |
Santambrogio L, Marrack P (2023) The broad spectrum of pathogenic autoreactivity. Nat Rev Immunol 23: 69-70. https://doi.org/10.1038/s41577-022-00812-2 ![]() |
[10] |
Simon CE (1909) On auto-antibody formation and antihemolysis. J Exp Med 11: 695-717. https://doi.org/10.1084/jem.11.5.695 ![]() |
[11] |
Schwentker FF, Rivers TM (1934) The antibody response of rabbits to injections of emulsions and extracts of homologous brain. J Exp Med 60: 559-574. https://doi.org/10.1084/jem.60.5.559 ![]() |
[12] |
Gear J (1945) Autoantigens and autoantibodies in the pathogenesis of disease with special refenence to blackwater fever. Trans R Soc Trop Med Hyg 39: 301-314. https://doi.org/10.1016/0035-9203(46)90041-7 ![]() |
[13] |
Cavelti PA (1945) Autoantibodies in rheumatic fever. Proc Soc Exp Biol Med 60: 379-381. https://doi.org/10.3181/00379727-60-15197P ![]() |
[14] |
Liblau RS, Wong FS, Mars LT, et al. (2002) Autoreactive CD8 T cells in organ-specific autoimmunity: Emerging targets for therapeutic intervention. Immunity 17: 1-6. https://doi.org/10.1016/S1074-7613(02)00338-2 ![]() |
[15] |
Shepard ER, Wegner A, Hill EV, et al. (2021) The mechanism of action of antigen processing independent T cell epitopes designed for immunotherapy of autoimmune diseases. Front Immunol 12: 654201. https://doi.org/10.3389/fimmu.2021.654201 ![]() |
[16] |
Klein L, Robey EA, Hsieh CS (2019) Central CD4(+) T cell tolerance: deletion versus regulatory T cell differentiation. Nat Rev Immunol 19: 7-18. https://doi.org/10.1038/s41577-018-0083-6 ![]() |
[17] |
Sharma C, Bayry J (2023) High risk of autoimmune diseases after COVID-19. Nat Rev Rheumatol 19: 399-400. https://doi.org/10.1038/s41584-023-00964-y ![]() |
[18] |
Lerner A, Benzvi C, Vojdani A (2023) Cross-reactivity and sequence similarity between microbial transglutaminase and human tissue antigens. Sci Rep 13: 17526. https://doi.org/10.1038/s41598-023-44452-5 ![]() |
[19] | Trier NH, Houen G (2023) Antibody cross-reactivity in auto-immune diseases. Int J Mol Sci 24. https://doi.org/10.3390/ijms241713609 |
[20] |
English J, Patrick S, Stewart LD (2023) The potential role of molecular mimicry by the anaerobic microbiota in the aetiology of autoimmune disease. Anaerobe 80: 102721. https://doi.org/10.1016/j.anaerobe.2023.102721 ![]() |
[21] |
Pisetsky DS (2023) Pathogenesis of autoimmune disease. Nat Rev Nephrol 19: 509-524. https://doi.org/10.1038/s41581-023-00720-1 ![]() |
[22] |
Hasan D, Shono A, van Kalken CK, et al. (2022) A novel definition and treatment of hyperinflammation in COVID-19 based on purinergic signalling. Purinergic Signal 18: 13-59. https://doi.org/10.1007/s11302-021-09814-6 ![]() |
[23] |
Jerne NK (1955) The natural-selection theory of antibody formation. Proc Natl Acad Sci USA 41: 849-857. https://doi.org/10.1073/pnas.41.11.849 ![]() |
[24] |
Burnet FM (1976) A modification of Jerne's theory of antibody production using the concept of clonal selection. CA Cancer J Clin 26: 119-121. https://doi.org/10.3322/canjclin.26.2.119 ![]() |
[25] | Burnet FM (1957) A modification of Jerne's theory of antibody production using the concept of clonal selection. Aust J Sci 20: 67-69. |
[26] |
Rajendeeran A, Tenbrock K (2021) Regulatory T cell function in autoimmune disease. J Transl Autoimmun 4: 100130. https://doi.org/10.1016/j.jtauto.2021.100130 ![]() |
[27] |
Dominguez-Villar M, Hafler DA (2018) Regulatory T cells in autoimmune disease. Nat Immunol 19: 665-673. https://doi.org/10.1038/s41590-018-0120-4 ![]() |
[28] |
Santori FR, Arsov I, Lilić M, et al. (2002) Editing autoreactive TCR enables efficient positive selection. J Immunol 169: 1729-1734. https://doi.org/10.4049/jimmunol.169.4.1729 ![]() |
[29] |
Zal T, Weiss S, Mellor A, et al. (1996) Expression of a second receptor rescues self-specific T cells from thymic deletion and allows activation of autoreactive effector function. Proc Natl Acad Sci USA 93: 9102-9107. https://doi.org/10.1073/pnas.93.17.9102 ![]() |
[30] |
Ni PP, Solomon B, Hsieh CS, et al. (2014) The ability to rearrange dual TCRs enhances positive selection, leading to increased Allo- and Autoreactive T cell repertoires. J Immunol 193: 1778-1786. https://doi.org/10.4049/jimmunol.1400532 ![]() |
[31] |
Auger JL, Haasken S, Steinert EM, et al. (2012) Incomplete TCR-β allelic exclusion accelerates spontaneous autoimmune arthritis in K/BxN TCR transgenic mice. Eur J Immunol 42: 2354-2362. https://doi.org/10.1002/eji.201242520 ![]() |
[32] |
George TC, Bilsborough J, Viney JL, et al. (2003) High antigen dose and activated dendritic cells enable Th cells to escape regulatory T cell-mediated suppression in vitro. Eur J Immunol 33: 502-511. https://doi.org/10.1002/immu.200310026 ![]() |
[33] |
Swee LK, Nusser A, Curti M, et al. (2014) The amount of self-antigen determines the effector function of murine T cells escaping negative selection. Eur J Immunol 44: 1299-1312. ![]() |
[34] |
Davis MM (2015) Not-so-negative selection. Immunity 43: 833-835. https://doi.org/10.1002/eji.201343840 ![]() |
[35] |
Legoux FP, Lim JB, Cauley AW, et al. (2015) CD4+ T cell tolerance to tissue-restricted self antigens is mediated by antigen-specific regulatory T cells rather than deletion. Immunity 43: 896-908. https://doi.org/10.1016/j.immuni.2015.10.011 ![]() |
[36] |
Rawlings DJ, Metzler G, Wray-Dutra M, et al. (2017) Altered B cell signalling in autoimmunity. Nat Rev Immunol 17: 421-436. https://doi.org/10.3390/cells10051190 ![]() |
[37] |
Bonasia CG, Abdulahad WH, Rutgers A, et al. (2021) B cell activation and escape of tolerance checkpoints: Recent insights from studying autoreactive B cells. Cells 10: 1190. https://doi.org/10.3390/cells10051190 ![]() |
[38] |
Giltiay NV, Chappell CP, Clark EA (2012) B-cell selection and the development of autoantibodies. Arthritis Res Ther 14. https://doi.org/10.1186/ar3918 ![]() |
[39] |
Suurmond J, Diamond B (2015) Autoantibodies in systemic autoimmune diseases: specificity and pathogenicity. J Clin Invest 125: 2194-2202. https://doi.org/10.1172/JCI78084 ![]() |
[40] |
Sakaguchi S, Wing K, Miyara M (2007) Regulatory T cells–a brief history and perspective. Eur J Immunol 37: S116-S123. https://doi.org/10.1002/eji.200737593 ![]() |
[41] |
Wing JB, Tekgüç M, Sakaguchi S (2018) Control of germinal center responses by T-Follicular regulatory cells. Front Immunol 9: 1910. https://doi.org/10.3389/fimmu.2018.01910 ![]() |
[42] |
Wing JB, Lim EL, Sakaguchi S (2020) Control of foreign Ag-specific Ab responses by Treg and Tfr. Immunol Rev 296: 104-119. https://doi.org/10.1111/imr.12888 ![]() |
[43] |
Ding T, Su R, Wu R, et al. (2021) Frontiers of autoantibodies in autoimmune disorders: Crosstalk between Tfh/Tfr and regulatory B cells. Front Immunol 12: 641013. https://doi.org/10.3389/fimmu.2021.641013 ![]() |
[44] |
Richards DM, Delacher M, Goldfarb Y, et al. (2015) Chapter eight-Treg cell differentiation: from thymus to peripheral tissue. Progress in Molecular Biology and Translational Science. New York: Academic Press 175-205. https://doi.org/10.1016/bs.pmbts.2015.07.014 ![]() |
[45] |
Schmitt EG, Williams CB (2013) Generation and function of induced regulatory T cells. Front Immunol 4: 152. https://doi.org/10.3389/fimmu.2013.00152 ![]() |
[46] |
Khantakova JN, Bulygin AS, Sennikov SV (2022) The regulatory-T-cell memory phenotype: What we know. Cells 11. https://doi.org/10.3390/cells11101687 ![]() |
[47] |
Sanchez AM, Zhu J, Huang X, et al. (2012) The development and function of memory regulatory T cells after acute viral infections. J Immunol 189: 2805-2814. https://doi.org/10.4049/jimmunol.1200645 ![]() |
[48] |
Rosenblum MD, Way SS, Abbas AK (2016) Regulatory T cell memory. Nat Rev Immunol 16: 90-101. https://doi.org/10.1038/nri.2015.1 ![]() |
[49] |
Kaminski A, Hager FT, Kopplin L, et al. (2023) Resident regulatory T cells reflect the immune history of individual lymph nodes. Sci Immunol 8: eadj5789. https://doi.org/10.1126/sciimmunol.adj5789 ![]() |
[50] |
Corthay A (2009) How do regulatory T cells work?. Scand J Immunol 70: 326-336. https://doi.org/10.1111/j.1365-3083.2009.02308.x ![]() |
[51] |
Sprouse ML, Scavuzzo MA, Blum S, et al. (2018) High self-reactivity drives T-bet and potentiates Treg function in tissue-specific autoimmunity. JCI Insight 3. https://doi.org/10.1172/jci.insight.97322 ![]() |
[52] |
Zuany-Amorim C, Sawicka E, Manlius C, et al. (2002) Suppression of airway eosinophilia by killed Mycobacterium vaccae-induced allergen-specific regulatory T-cells. Nat Med 8: 625-629. https://doi.org/10.1038/nm0602-625 ![]() |
[53] |
Belkaid Y, Piccirillo CA, Mendez S, et al. (2002) CD4+CD25+ regulatory T cells control Leishmania major persistence and immunity. Nature 420: 502-507. https://doi.org/10.1038/nature01152 ![]() |
[54] |
Suffia IJ, Reckling SK, Piccirillo CA, et al. (2006) Infected site-restricted Foxp3+ natural regulatory T cells are specific for microbial antigens. J Exp Med 203: 777-788. https://doi.org/10.1084/jem.20052056 ![]() |
[55] |
Montagnoli C, Bacci A, Bozza S, et al. (2002) B7/CD28-dependent CD4+CD25+ regulatory T cells are essential components of the memory-protective immunity to Candida albicans. J Immunol 169: 6298-6308. https://doi.org/10.4049/jimmunol.169.11.6298 ![]() |
[56] |
McGuirk P, McCann C, Mills KH (2002) Pathogen-specific T regulatory 1 cells induced in the respiratory tract by a bacterial molecule that stimulates interleukin 10 production by dendritic cells: a novel strategy for evasion of protective T helper type 1 responses by Bordetella pertussis. J Exp Med 195: 221-231. https://doi.org/10.1084/jem.20011288 ![]() |
[57] |
Kullberg MC, Jankovic D, Gorelick PL, et al. (2002) Bacteria-triggered CD4(+) T regulatory cells suppress Helicobacter hepaticus-induced colitis. J Exp Med 196: 505-515. https://doi.org/10.1084/jem.20020556 ![]() |
[58] |
Ochando JC, Yopp AC, Yang Y, et al. (2005) Lymph node occupancy is required for the peripheral development of alloantigen-specific Foxp3+ regulatory T cells. J Immunol 174: 6993-7005. https://doi.org/10.4049/jimmunol.174.11.6993 ![]() |
[59] |
van Maurik A, Herber M, Wood KJ, et al. (2002) Cutting edge: CD4+CD25+ alloantigen-specific immunoregulatory cells that can prevent CD8+ T cell-mediated graft rejection: implications for anti-CD154 immunotherapy. J Immunol 169: 5401-5404. https://doi.org/10.4049/jimmunol.169.10.5401 ![]() |
[60] |
Smith DG, Martinelli R, Besra GS, et al. (2019) Identification and characterization of a novel anti-inflammatory lipid isolated from Mycobacterium vaccae, a soil-derived bacterium with immunoregulatory and stress resilience properties. Psychopharmacology 236: 1653-1670. https://doi.org/10.1007/s00213-019-05253-9 ![]() |
[61] |
Múnera M, Farak J, Pérez M, et al. (2020) Prediction of molecular mimicry between antigens from Leishmania sp. and human: Implications for autoimmune response in systemic lupus erythematosus. Microb Pathog 148: 104444. https://doi.org/10.1016/j.micpath.2020.104444 ![]() |
[62] | Argov S, Jaffe CL, Krupp M, et al. (1989) Autoantibody production by patients infected with Leishmania. Clin Exp Immunol 76: 190-197. |
[63] |
Gustafson KS, Vercellotti GM, Bendel CM, et al. (1991) Molecular mimicry in Candida albicans. Role of an integrin analogue in adhesion of the yeast to human endothelium. J Clin Invest 87: 1896-1902. https://doi.org/10.1172/JCI115214 ![]() |
[64] | Vojdani A, Rahimian P, Kalhor H, et al. (1996) Immunological cross reactivity between Candida albicans and human tissue. J Clin Lab Immunol 48: 1-15. |
[65] |
Sandros J, Rozdzinski E, Zheng J, et al. (1994) Lectin domains in the toxin of Bordetella pertussis: selectin mimicry linked to microbial pathogenesis. Glycoconj J 11: 501-506. https://doi.org/10.1007/BF00731300 ![]() |
[66] |
Sandros J, Tuomanen E (1993) Attachment factors of Bordetella pertussis: mimicry of eukaryotic cell recognition molecules. Trends Microbiol 1: 192-196. https://doi.org/10.1016/0966-842X(93)90090-E ![]() |
[67] |
Alyamani EJ, Brandt P, Pena JA, et al. (2007) Helicobacter hepaticus catalase shares surface-predicted epitopes with mammalian catalases. Microbiology (Reading) 153: 1006-1016. https://doi.org/10.1099/mic.0.29184-0 ![]() |
[68] |
Macdonald WA, Chen Z, Gras S, et al. (2009) T cell allorecognition via molecular mimicry. Immunity 31: 897-908. https://doi.org/10.1016/j.immuni.2009.09.025 ![]() |
[69] |
Koyama M, Hill GR (2016) Alloantigen presentation and graft-versus-host disease: fuel for the fire. Blood 127: 2963-2970. https://doi.org/10.1182/blood-2016-02-697250 ![]() |
[70] |
Hudson KE, Wong ASL, Richards AL, et al. (2019) Alloimmunogenicity of an isolated MHC allele is affected by the context of MHC mismatch in a murine model. Transfusion 59: 744-753. https://doi.org/10.1111/trf.15109 ![]() |
[71] |
Mondino S, Schmidt S, Buchrieser C (2020) Molecular mimicry: A paradigm of host-microbe coevolution illustrated by legionella. mBio 11. https://doi.org/10.1128/mBio.01201-20 ![]() |
[72] |
Pacholczyk R, Kern J, Singh N, et al. (2007) Nonself-antigens are the cognate specificities of Foxp3+ regulatory T cells. Immunity 27: 493-504. https://doi.org/10.1016/j.immuni.2007.07.019 ![]() |
[73] |
Stephens GL, Shevach EM (2007) Foxp3+ regulatory T cells: selfishness under scrutiny. Immunity 27: 417-419. ![]() |
[74] | Vignali DA, Collison LW, Workman CJ (2008) How regulatory T cells work. NatRev Immunol 8: 523-532. https://doi.org/10.1038/nri2343 |
[75] |
Tan D, Yin W, Guan F, et al. (2022) B cell-T cell interplay in immune regulation: A focus on follicular regulatory T and regulatory B cell functions. Front Cell Dev Biol 10: 991840. https://doi.org/10.3389/fcell.2022.991840 ![]() |
[76] |
Rosser EC, Mauri C (2015) Regulatory B cells: origin, phenotype, and function. Immunity 42: 607-612. https://doi.org/10.1016/j.immuni.2015.04.005 ![]() |
[77] |
Wang R, Xie R, Song Z (2018) Circulating regulatory Tfh cells are enriched in patients with chronic hepatitis B infection and induce the differentiation of regulatory B cells. Exp Cell Res 365: 171-176. https://doi.org/10.1016/j.yexcr.2018.02.031 ![]() |
[78] |
Li H, Zhou R, Wang C, et al. (2018) T follicular regulatory cells infiltrate the human airways during the onset of acute respiratory distress syndrome and regulate the development of B regulatory cells. Immunol Res 66: 548-554. https://doi.org/10.1007/s12026-018-9014-7 ![]() |
[79] |
Catalán D, Mansilla MA, Ferrier A, et al. (2021) Immunosuppressive mechanisms of regulatory B Cells. Front Immunol 12. https://doi.org/10.3389/fimmu.2021.611795 ![]() |
[80] |
Figueiró F, Muller L, Funk S, et al. (2016) Phenotypic and functional characteristics of CD39 (high) human regulatory B cells (Breg). Oncoimmunology 5: e1082703. https://doi.org/10.1080/2162402X.2015.1082703 ![]() |
[81] |
Kaku H, Cheng KF, Al-Abed Y, et al. (2014) A novel mechanism of B cell-mediated immune suppression through CD73 expression and adenosine production. J Immunol 193: 5904-5913. https://doi.org/10.4049/jimmunol.1400336 ![]() |
[82] |
Saze Z, Schuler PJ, Hong CS, et al. (2013) Adenosine production by human B cells and B cell-mediated suppression of activated T cells. Blood 122: 9-18. https://doi.org/10.1182/blood-2013-02-482406 ![]() |
[83] |
Khan AR, Hams E, Floudas A, et al. (2015) PD-L1hi B cells are critical regulators of humoral immunity. Nat Commun 6: 5997. https://doi.org/10.1038/ncomms6997 ![]() |
[84] |
Wang K, Tao L, Su J, et al. (2017) TLR4 supports the expansion of FasL(+)CD5(+)CD1d(hi) regulatory B cells, which decreases in contact hypersensitivity. Mol Immunol 87: 188-199. https://doi.org/10.1016/j.molimm.2017.04.016 ![]() |
[85] |
Ma L, Liu B, Jiang Z, et al. (2014) Reduced numbers of regulatory B cells are negatively correlated with disease activity in patients with new-onset rheumatoid arthritis. Clin Rheumatol 33: 187-195. https://doi.org/10.1007/s10067-013-2359-3 ![]() |
[86] |
Yang M, Deng J, Liu Y, et al. (2012) IL-10-producing regulatory B10 cells ameliorate collagen-induced arthritis via suppressing Th17 cell generation. Am J Pathol 180: 2375-2385. https://doi.org/10.1016/j.ajpath.2012.03.010 ![]() |
[87] |
Flores-Borja F, Bosma A, Ng D, et al. (2013) CD19+CD24hiCD38hi B cells maintain regulatory T cells while limiting TH1 and TH17 differentiation. Sci Transl Med 5: 173ra123. https://doi.org/10.1126/scitranslmed.3005407 ![]() |
[88] |
Carter NA, Rosser EC, Mauri C (2012) Interleukin-10 produced by B cells is crucial for the suppression of Th17/Th1 responses, induction of T regulatory type 1 cells and reduction of collagen-induced arthritis. Arthritis Res Ther 14: R32. https://doi.org/10.1186/ar3736 ![]() |
[89] |
Gondek DC, Lu LF, Quezada SA, et al. (2005) Cutting edge: contact-mediated suppression by CD4+CD25+ regulatory cells involves a granzyme B-dependent, perforin-independent mechanism. J Immunol 174: 1783-1786. https://doi.org/10.4049/jimmunol.174.4.1783 ![]() |
[90] |
Jahrsdörfer B, Vollmer A, Blackwell SE, et al. (2010) Granzyme B produced by human plasmacytoid dendritic cells suppresses T-cell expansion. Blood 115: 1156-1165. https://doi.org/10.1182/blood-2009-07-235382 ![]() |
[91] |
Xu L, Liu X, Liu H, et al. (2017) Impairment of granzyme B-producing regulatory B cells correlates with exacerbated rheumatoid arthritis. Front Immunol 8: 768. https://doi.org/10.3389/fimmu.2017.00768 ![]() |
[92] | Saraiva M, Vieira P, O'Garra A (2019) Biology and therapeutic potential of interleukin-10. J Exp Med 217. https://doi.org/10.1084/jem.20190418 |
[93] |
Zysk W, Gleń J, Trzeciak M (2022) Current insight into the role of IL-35 and its potential involvement in the pathogenesis and therapy of atopic dermatitis. Int J Mol Sci 23: 15709. https://doi.org/10.3390/ijms232415709 ![]() |
[94] |
Massagué J, Sheppard D (2023) TGF-β signaling in health and disease. Cell 186: 4007-4037. https://doi.org/10.1016/j.cell.2023.07.036 ![]() |
[95] |
Needham EJ, Stoevesandt O, Thelin EP, et al. (2021) Complex autoantibody responses occur following moderate to severe traumatic brain injury. J Immunol 207: 90-100. https://doi.org/10.4049/jimmunol.2001309 ![]() |
[96] |
Zhang Z, Zoltewicz JS, Mondello S, et al. (2014) Human traumatic brain injury induces autoantibody response against glial fibrillary acidic protein and its breakdown products. PLoS One 9: e92698. https://doi.org/10.1371/journal.pone.0092698 ![]() |
[97] | Zimering MB (2023) Repeated traumatic brain injury is associated with neurotoxic plasma autoantibodies directed against the serotonin 2A and alpha 1 adrenergic receptors. Endocrinol Diabetes Metab J 7: 1-12. https://doi.org/10.31038/EDMJ.2023722 |
[98] |
Zimering MB, Pulikeyil AT, Myers CE, et al. (2020) Serotonin 2A receptor autoantibodies increase in adult traumatic brain injury in association with neurodegeneration. J Endocrinol Diabetes 7: 1-8. https://doi.org/10.15226/2374-6890/7/1/001142 ![]() |
[99] |
Vijapur SM, Yang Z, Barton DJ, et al. (2020) Anti-pituitary and anti-hypothalamus autoantibody associations with inflammation and persistent hypogonadotropic hypogonadism in men with traumatic brain injury. J Neurotrauma 37: 1609-1626. https://doi.org/10.1089/neu.2019.6780 ![]() |
[100] |
Arevalo-Martin A, Grassner L, Garcia-Ovejero D, et al. (2018) Elevated autoantibodies in subacute human spinal cord injury are naturally occurring antibodies. Front Immunol 9. https://doi.org/10.3389/fimmu.2018.02365 ![]() |
[101] |
Schwab JM, Haider C, Kopp MA, et al. (2023) Lesional antibody synthesis and complement deposition associate with de novo antineuronal antibody synthesis after spinal cord injury. Neurol Neuroimmunol Neuroinflammation 10: e200099. https://doi.org/10.1212/NXI.0000000000200099 ![]() |
[102] |
Caputo F, Barranco R, Ricci P, et al. (2021) An unusual case of coma related to glutamate receptor 3 (GluR3) auto-antibodies after a traumatic spinal cord injury: Clinical and pathological aspects. Med Leg J 89: 133-136. https://doi.org/10.1177/0025817220970069 ![]() |
[103] |
Davies AL, Hayes KC, Dekaban GA (2007) Clinical correlates of elevated serum concentrations of cytokines and autoantibodies in patients with spinal cord injury. Arch Phys Med Rehabil 88: 1384-1393. https://doi.org/10.1016/j.apmr.2007.08.004 ![]() |
[104] |
Hayes KC, Hull TC, Delaney GA, et al. (2002) Elevated serum titers of proinflammatory cytokines and CNS autoantibodies in patients with chronic spinal cord injury. J Neurotrauma 19: 753-761. https://doi.org/10.1089/08977150260139129 ![]() |
[105] |
Panoutsakopoulou V, Sanchirico ME, Huster KM, et al. (2001) Analysis of the relationship between viral infection and autoimmune disease. Immunity 15: 137-147. https://doi.org/10.1016/S1074-7613(01)00172-8 ![]() |
[106] |
Wang EY, Mao T, Klein J, et al. (2021) Diverse functional autoantibodies in patients with COVID-19. Nature 595: 283-288. https://doi.org/10.1038/s41586-021-03631-y ![]() |
[107] |
Trahtemberg U, Rottapel R, Dos Santos CC, et al. (2021) Anticardiolipin and other antiphospholipid antibodies in critically ill COVID-19 positive and negative patients. Ann Rheum Dis 80: 1236-1240. https://doi.org/10.1136/annrheumdis-2021-220206 ![]() |
[108] |
Chang SE, Feng A, Meng W, et al. (2021) New-onset IgG autoantibodies in hospitalized patients with COVID-19. Nat Commun 12: 5417. https://doi.org/10.1038/s41467-021-25509-3 ![]() |
[109] |
Arthur JM, Forrest JC, Boehme KW, et al. (2021) Development of ACE2 autoantibodies after SARS-CoV-2 infection. PLoS One 16: e0257016. https://doi.org/10.1371/journal.pone.0257016 ![]() |
[110] |
Humphrey JH (1948) The pathogenesis of glomerulonephritis; a reinvestigation of the auto-immunization hypothesis. J Pathol Bacteriol 60: 211-218. https://doi.org/10.1002/path.1700600208 ![]() |
[111] | Cavelti PA (1947) Studies on the pathogenesis of rheumatic fever; experimental production of autoantibodies to heart, skeletal muscle and connective tissue. Arch Pathol (Chic) 44: 1-12. |
[112] | Cavelti PA (1947) Studies on the pathogenesis of rheumatic fever; cardiac lesions produced in rats by means of autoantibodies to heart and connective tissues. Arch Pathol (Chic) 44: 13-27. |
[113] | Cavelti PA (1947) Pathogenesis of glomerulonephritis and rheumatic fever; in vivo activation of tissue antigens as a result of streptococcic infection and consecutive formation of autoantibodies. Arch Pathol (Chic) 44: 119-125. |
[114] |
Segal Y, Shoenfeld Y (2018) Vaccine-induced autoimmunity: the role of molecular mimicry and immune crossreaction. Cell Mol Immunol 15: 586-594. https://doi.org/10.1038/cmi.2017.151 ![]() |
[115] |
Talotta R (2023) Molecular mimicry and HLA polymorphisms may drive autoimmunity in recipients of the BNT-162b2 mRNA vaccine: A computational analysis. Microorganisms 11. https://doi.org/10.3390/microorganisms11071686 ![]() |
[116] |
Franco A, Song J, Chambers C, et al. (2023) SARS-CoV-2 spike-specific regulatory T cells (Treg) expand and develop memory in vaccine recipients suggesting a role for immune regulation in preventing severe symptoms in COVID-19. Autoimmunity 56: 2259133. https://doi.org/10.1080/08916934.2023.2259133 ![]() |
[117] |
Fiorelli D, Caruso V, Belardi R, et al. (2023) Evaluation of autoantibody profile in healthy subjects after mRNA vaccination against COVID-19. Int Immunopharmacol 122: 110592. https://doi.org/10.1016/j.intimp.2023.110592 ![]() |
[118] |
Greinacher A, Selleng K, Palankar R, et al. (2021) Insights in ChAdOx1 nCoV-19 vaccine-induced immune thrombotic thrombocytopenia. Blood 138: 2256-2268. https://doi.org/10.1182/blood.2021013231 ![]() |
[119] |
Greinacher A, Thiele T, Warkentin TE, et al. (2021) Thrombotic thrombocytopenia after ChAdOx1 nCov-19 vaccination. N Engl J Med 384: 2092-2101. https://doi.org/10.1056/NEJMoa2104840 ![]() |
[120] |
Krashias G, Pafiti A, Deeba E, et al. (2022) SARS CoV-2 vaccination induces antibodies against cardiolipin. BMC Res Notes 15: 292. https://doi.org/10.1186/s13104-022-06180-3 ![]() |
[121] |
Xu W, Wen X, Cong X, et al. (2023) COVID-19 mRNA vaccine, but not a viral vector-based vaccine, promotes neutralizing anti-type I interferon autoantibody production in a small group of healthy individuals. J Med Virol 95: e29137. https://doi.org/10.1002/jmv.29137 ![]() |
[122] |
Aladdin Y, Algahtani H, Shirah B (2021) Vaccine-induced immune thrombotic thrombocytopenia with disseminated intravascular coagulation and death following the ChAdOx1 nCoV-19 vaccine. J Stroke Cerebrovasc Dis 30: 105938. https://doi.org/10.1016/j.jstrokecerebrovasdis.2021.105938 ![]() |
[123] |
Alami A, Villeneuve PJ, Farrell PJ, et al. (2023) Myocarditis and pericarditis post-mRNA COVID-19 vaccination: Insights from a pharmacovigilance perspective. J Clin Med 12. https://doi.org/10.3390/jcm12154971 ![]() |
[124] | Di Dedda EA, Barison A, Aquaro GD, et al. (2022) Cardiac magnetic resonance imaging of myocarditis and pericarditis following COVID-19 vaccination: a multicenter collection of 27 cases. Eur Radiol : 1-9. https://doi.org/10.1007/s00330-022-08566-0 |
[125] |
Yap J, Tham MY, Poh J, et al. (2022) Pericarditis and myocarditis after COVID-19 mRNA vaccination in a nationwide setting. Ann Acad Med Singap 51: 96-100. https://doi.org/10.47102/annals-acadmedsg.2021425 ![]() |
[126] |
Galván-Peña S, Leon J, Chowdhary K, et al. (2021) Profound Treg perturbations correlate with COVID-19 severity. Proc Natl Acad Sci USA 118. https://doi.org/10.1073/pnas.2111315118 ![]() |
[127] |
Neumann J, Prezzemolo T, Vanderbeke L, et al. (2020) Increased IL-10-producing regulatory T cells are characteristic of severe cases of COVID-19. Clin Transl Immunol 9: e1204. https://doi.org/10.1002/cti2.1204 ![]() |
[128] |
Vick SC, Frutoso M, Mair F, et al. (2021) A regulatory T cell signature distinguishes the immune landscape of COVID-19 patients from those with other respiratory infections. Sci Adv 7: eabj0274. https://doi.org/10.1126/sciadv.abj0274 ![]() |
[129] |
Saheb Sharif-Askari F, Saheb Sharif-Askari N, Hafezi S, et al. (2023) Increased blood immune regulatory cells in severe COVID-19 with autoantibodies to type I interferons. Sci Rep 13: 17344. https://doi.org/10.1038/s41598-023-43675-w ![]() |
[130] | Wang F, Hou H, Luo Y, et al. (2020) The laboratory tests and host immunity of COVID-19 patients with different severity of illness. JCI Insight 5. https://doi.org/10.1172/jci.insight.137799 |
[131] | Sadeghi A, Tahmasebi S, Mahmood A, et al. (2020) Th17 and Treg cells function in SARS-CoV2 patients compared with healthy controls. J Cell Physiol 4: 2829-2839. https://doi.org/10.1002/jcp.30047 |
[132] |
Chen G, Wu D, Guo W, et al. (2020) Clinical and immunological features of severe and moderate coronavirus disease 2019. J Clin Invest 130: 2620-2629. https://doi.org/10.1172/JCI137244 ![]() |
[133] | Mohebbi SR, Baghaei K, Rostami-Nejad M, et al. (2020) Significant changes of CD4, FOXP3, CD25, and IL6 expression level in Iranian COVID-19 patients. Gastroenterol Hepatol Bed Bench 13: 388-392. |
[134] |
Caldrer S, Mazzi C, Bernardi M, et al. (2021) Regulatory T cells as predictors of clinical course in hospitalised COVID-19 patients. Front Immunol 12: 789735. https://doi.org/10.3389/fimmu.2021.789735 ![]() |
[135] |
Meckiff BJ, Ramírez-Suástegui C, Fajardo V, et al. (2020) Imbalance of regulatory and cytotoxic SARS-CoV-2-Reactive CD4(+) T Cells in COVID-19. Cell 183: 1340-1353.e16. https://doi.org/10.3410/f.738790055.793579433 ![]() |
[136] |
Rojas M, Herrán M, Ramírez-Santana C, et al. (2023) Molecular mimicry and autoimmunity in the time of COVID-19. J Autoimmun 139: 103070. https://doi.org/10.1016/j.jaut.2023.103070 ![]() |
[137] |
Gouttefangeas C, Klein R, Maia A (2023) The good and the bad of T cell cross-reactivity: challenges and opportunities for novel therapeutics in autoimmunity and cancer. Front Immunol 14. https://doi.org/10.3389/fimmu.2023.1212546 ![]() |
[138] |
Grassi F, Salina G (2023) The P2X7 receptor in autoimmunity. Int J Mol Sci 24: 14116. https://doi.org/10.3390/ijms241814116 ![]() |
[139] |
Di Virgilio F, Giuliani AL (2016) Purinergic signalling in autoimmunity: A role for the P2X7R in systemic lupus erythematosus?. Biomed J 39: 326-338. https://doi.org/10.1016/j.bj.2016.08.006 ![]() |
[140] |
Drury AN, Szent-Gyorgyi A (1929) The physiological activity of adenine compounds with especial reference to their action upon the mammalian heart. J Physiol 68: 213-237. https://doi.org/10.1113/jphysiol.1929.sp002608 ![]() |
[141] | Lohmann K (1929) Über die Pyrophosphatfraktion im Muskel. Naturwissenschaften 17: 624-625. https://doi.org/10.1007/BF01506215 |
[142] |
Engelhardt WA, Ljubimowa MN (1939) Myosine and Adenosinetriphosphatase. Nature 144: 668-669. https://doi.org/10.1038/144668b0 ![]() |
[143] |
Lipmann F (1940) A phosphorylated oxygenation product of pyruvic acid. J Biol Chem 134: 463-464. https://doi.org/10.1016/S0021-9258(18)73290-0 ![]() |
[144] |
Lipmann F (1944) Enzymatic synthesis of acetyl phosphate. J Biol Chem 155: 55-70. https://doi.org/10.1016/S0021-9258(18)43172-9 ![]() |
[145] |
Lipmann F, Jones ME, Black S, et al. (1953) The mechanism of the ATP-CoA-acetate reaction. J Cell Physiol Suppl 41: 109-112. https://doi.org/10.1002/jcp.1030410408 ![]() |
[146] |
Feldberg W, Hebb C (1948) The stimulating action of phosphate compounds on the perfused superior cervical ganglion of the cat. J Physiol 107: 210-221. https://doi.org/10.1113/jphysiol.1948.sp004264 ![]() |
[147] |
Holton P (1959) The liberation of adenosine triphosphate on antidromic stimulation of sensory nerves. J Physiol 145: 494-504. https://doi.org/10.1113/jphysiol.1959.sp006157 ![]() |
[148] | Burnstock G (1972) Purinergic nerves. Pharmacol Rev 24: 509-581. |
[149] |
Burnstock G (2012) Purinergic signalling: Its unpopular beginning, its acceptance and its exciting future. Bioessays 34: 218-225. https://doi.org/10.1002/bies.201100130 ![]() |
[150] |
Burnstock G (2014) Purinergic signalling: from discovery to current developments. Exp Physiol 99: 16-34. https://doi.org/10.1113/expphysiol.2013.071951 ![]() |
[151] |
Surprenant A, Rassendren F, Kawashima E, et al. (1996) The cytolytic P2Z receptor for extracellular ATP identified as a P2X receptor (P2X7). Science 272: 735-738. https://doi.org/10.1126/science.272.5262.735 ![]() |
[152] |
Di Virgilio F (1995) The P2Z purinoceptor: an intriguing role in immunity, inflammation and cell death. Immunol Today 16: 524-528. https://doi.org/10.1016/0167-5699(95)80045-X ![]() |
[153] |
Ai Y, Wang H, Liu L, et al. (2023) Purine and purinergic receptors in health and disease. MedComm 4: e359. https://doi.org/10.1002/mco2.359 ![]() |
[154] |
Burnstock G (2020) Introduction to purinergic signaling. Methods Mol Biol 2041: 1-15. https://doi.org/10.1007/978-1-4939-9717-6_1 ![]() |
[155] | Ralevic V, Burnstock G (1998) Receptors for purines and pyrimidines. Pharmacol Rev 50: 413-492. |
[156] |
Burnstock G (2018) Purine and purinergic receptors. Brain Neurosci Adv 2. https://doi.org/10.1177/2398212818817494 ![]() |
[157] |
Fredholm BB, Frenguelli BG, Hills R, et al. (2023) Adenosine receptors in GtoPdb v.2023.1. IUPHAR/BPS Guide to Pharmacology CITE 2023. https://doi.org/10.2218/gtopdb/F3/2023.1 ![]() |
[158] | Di Virgilio F, Falzoni S, Fortuny-Gomez A, et al. (2023) P2X receptors in GtoPdb v.2023.1. IUPHAR/BPS Guide to Pharmacology CITE . https://doi.org/10.2218/gtopdb/F77/2023.1 |
[159] | Abbracchio M-P, Boeynaems J-M, Boyer JL, et al. (2023) P2Y receptors in GtoPdb v.2023.1. IUPHAR/BPS Guide to Pharmacology CITE 1. https://doi.org/10.2218/gtopdb/F52/2023.1 |
[160] |
Cotrina ML, Nedergaard M (2009) Physiological and pathological functions of P2X7 receptor in the spinal cord. Purinergic Signal 5: 223-232. https://doi.org/10.1007/s11302-009-9138-2 ![]() |
[161] |
Alves LA, Bezerra RJ, Faria RX, et al. (2013) Physiological roles and potential therapeutic applications of the P2X7 receptor in inflammation and pain. Molecules 18: 10953-10972. https://doi.org/10.3390/molecules180910953 ![]() |
[162] |
Hasan D, Blankman P, Nieman GF (2017) Purinergic signalling links mechanical breath profile and alveolar mechanics with the pro-inflammatory innate immune response causing ventilation-induced lung injury. Purinergic Signal 13: 363-386. https://doi.org/10.1007/s11302-017-9564-5 ![]() |
[163] |
Hasan D, Satalin J, van der Zee P, et al. (2018) Excessive extracellular ATP desensitizes P2Y2 and P2X4 ATP receptors provoking surfactant impairment ending in ventilation-induced lung injury. Int J Mol Sci 19: 1185. https://doi.org/10.3390/ijms19041185 ![]() |
[164] |
Patel AS, Reigada D, Mitchell CH, et al. (2005) Paracrine stimulation of surfactant secretion by extracellular ATP in response to mechanical deformation. Am J Physiol Lung Cell MolPhysiol 289: L489-L496. https://doi.org/10.1152/ajplung.00074.2005 ![]() |
[165] |
Kim KC, Zheng QX, Van-Seuningen I (1993) Involvement of a signal transduction mechanism in ATP-induced mucin release from cultured airway goblet cells. Am J Respir Cell Mol Biol 8: 121-125. https://doi.org/10.1165/ajrcmb/8.2.121 ![]() |
[166] |
Tozzi M, Larsen AT, Lange SC, et al. (2018) The P2X7 receptor and pannexin-1 are involved in glucose-induced autocrine regulation in beta-cells. Sci Rep 8: 8926. https://doi.org/10.1038/s41598-018-27281-9 ![]() |
[167] |
Jacques-Silva MC, Correa-Medina M, Cabrera O, et al. (2010) ATP-gated P2X3 receptors constitute a positive autocrine signal for insulin release in the human pancreatic beta cell. Proc Natl Acad Sci USA 107: 6465-6470. https://doi.org/10.1073/pnas.0908935107 ![]() |
[168] |
Eltzschig HK, Sitkovsky MV, Robson SC (2012) Purinergic signaling during inflammation. N Engl J Med 367: 2322-2333. https://doi.org/10.1056/NEJMra1205750 ![]() |
[169] |
Idzko M, Ferrari D, Eltzschig HK (2014) Nucleotide signalling during inflammation. Nature 509: 310-317. https://doi.org/10.1038/nature13085 ![]() |
[170] |
Cekic C, Linden J (2016) Purinergic regulation of the immune system. Nat Rev Immunol 16: 177-192. https://doi.org/10.1038/nri.2016.4 ![]() |
[171] |
Adinolfi E, Giuliani AL, De Marchi E, et al. (2018) The P2X7 receptor: A main player in inflammation. Biochem Pharmacol 151: 234-244. https://doi.org/10.1016/j.bcp.2017.12.021 ![]() |
[172] |
Savio LEB, de Andrade Mello P, da Silva CG, et al. (2018) The P2X7 receptor in inflammatory diseases: Angel or demon?. Front Pharmacol 9: 52. https://doi.org/10.3389/fphar.2018.00052 ![]() |
[173] |
Zarek PE, Huang CT, Lutz ER, et al. (2008) A2A receptor signaling promotes peripheral tolerance by inducing T-cell anergy and the generation of adaptive regulatory T cells. Blood 111: 251-259. https://doi.org/10.1182/blood-2007-03-081646 ![]() |
[174] |
Ohta A, Kini R, Ohta A, et al. (2012) The development and immunosuppressive functions of CD4(+) CD25(+) FoxP3(+) regulatory T cells are under influence of the adenosine-A2A adenosine receptor pathway. Front Immunol 3: 190. https://doi.org/10.3389/fimmu.2012.00190 ![]() |
[175] | Shi L, Feng M, Du S, et al. (2019) Adenosine generated by regulatory T cells induces CD8(+) T cell exhaustion in gastric cancer through A2aR pathway. Biomed Res Int 2019: 4093214. https://doi.org/10.1155/2019/4093214 |
[176] |
Wiley JS, Gu BJ (2012) A new role for the P2X7 receptor: a scavenger receptor for bacteria and apoptotic cells in the absence of serum and extracellular ATP. Purinergic Signal 8: 579-586. https://doi.org/10.1007/s11302-012-9308-5 ![]() |
[177] |
Gu BJ, Saunders BM, Petrou S, et al. (2011) P2X(7) is a scavenger receptor for apoptotic cells in the absence of its ligand, extracellular ATP. J Immunol 187: 2365-2375. https://doi.org/10.4049/jimmunol.1101178 ![]() |
[178] |
Brandao-Burch A, Key ML, Patel JJ, et al. (2012) The P2X7 receptor is an important regulator of extracellular ATP levels. Front Endocrinol (Lausanne) 3: 41. https://doi.org/10.3389/fendo.2012.00041 ![]() |
[179] |
Gutierrez-Martin Y, Bustillo D, Gomez-Villafuertes R, et al. (2011) P2X7 receptors trigger ATP exocytosis and modify secretory vesicle dynamics in neuroblastoma cells. J Biol Chem 286: 11370-11381. https://doi.org/10.1074/jbc.M110.139410 ![]() |
[180] |
Suadicani SO, Brosnan CF, Scemes E (2006) P2X7 receptors mediate ATP release and amplification of astrocytic intercellular Ca2+ signaling. J Neurosci 26: 1378-1385. https://doi.org/10.1523/JNEUROSCI.3902-05.2006 ![]() |
[181] |
Henriquez M, Herrera-Molina R, Valdivia A, et al. (2011) ATP release due to Thy-1-integrin binding induces P2X7-mediated calcium entry required for focal adhesion formation. J Cell Sci 124: 1581-1588. https://doi.org/10.1242/jcs.073171 ![]() |
[182] |
Mishra A, Chintagari NR, Guo Y, et al. (2011) Purinergic P2X7 receptor regulates lung surfactant secretion in a paracrine manner. J Cell Sci 124: 657-668. https://doi.org/10.1242/jcs.066977 ![]() |
[183] |
Ohshima Y, Tsukimoto M, Takenouchi T, et al. (2010) gamma-Irradiation induces P2X(7) receptor-dependent ATP release from B16 melanoma cells. Biochim Biophys Acta 1800: 40-46. ![]() |
[184] |
Johnsen B, Kaschubowski KE, Nader S, et al. (2019) P2X7A-mediated ATP secretion is accompanied by depletion of cytosolic ATP. Purinergic Signal 15: 155-166. https://doi.org/10.1007/s11302-019-09654-5 ![]() |
[185] | Sáez PJ, Vargas P, Shoji KF, et al. (2017) ATP promotes the fast migration of dendritic cells through the activity of pannexin 1 channels and P2X(7) receptors. Sci Signal 10. https://doi.org/10.1126/scisignal.aah7107 |
[186] | Basu M, Gupta P, Dutta A, et al. (2020) Increased host ATP efflux and its conversion to extracellular adenosine is crucial for establishing Leishmania infection. J Cell Sci 133. https://doi.org/10.1242/jcs.239939 |
[187] |
Atkinson SK, Morice AH, Sadofsky LR (2020) Rhinovirus-16 increases ATP release in A549 cells without concomitant increase in production. ERJ Open Res 6. https://doi.org/10.1183/23120541.00159-2020 ![]() |
[188] | Ledderose C, Valsami EA, Elevado M, et al. (2023) ATP release from influenza-infected lungs enhances neutrophil activation and promotes disease progression. J Infect Dis . https://doi.org/10.1093/infdis/jiad442 |
[189] |
Zhang C, He H, Wang L, et al. (2017) Virus-triggered ATP release limits viral replication through facilitating IFN-β production in a P2X7-dependent manner. J Immunol 199: 1372-1381. https://doi.org/10.4049/jimmunol.1700187 ![]() |
[190] |
Adinolfi E, Callegari MG, Ferrari D, et al. (2005) Basal activation of the P2X7 ATP receptor elevates mitochondrial calcium and potential, increases cellular ATP levels, and promotes serum-independent growth. Mol Biol Cell 16: 3260-3272. https://doi.org/10.1091/mbc.e04-11-1025 ![]() |
[191] |
Ghazi K, Deng-Pichon U, Warnet JM, et al. (2012) Hyaluronan fragments improve wound healing on in vitro cutaneous model through P2X7 purinoreceptor basal activation: role of molecular weight. PLoS One 7: e48351. https://doi.org/10.1371/journal.pone.0048351 ![]() |
[192] |
Adinolfi E, Callegari MG, Cirillo M, et al. (2009) Expression of the P2X7 receptor increases the Ca2+ content of the endoplasmic reticulum, activates NFATc1, and protects from apoptosis. J Biol Chem 284: 10120-10128. https://doi.org/10.1074/jbc.M805805200 ![]() |
[193] |
Gilbert SM, Oliphant CJ, Hassan S, et al. (2019) ATP in the tumour microenvironment drives expression of nfP2X7, a key mediator of cancer cell survival. Oncogene 38: 194-208. https://doi.org/10.1038/s41388-018-0426-6 ![]() |
[194] |
Pellegatti P, Falzoni S, Donvito G, et al. (2011) P2X7 receptor drives osteoclast fusion by increasing the extracellular adenosine concentration. Faseb j 25: 1264-1274. https://doi.org/10.1096/fj.10-169854 ![]() |
[195] |
Amoroso F, Falzoni S, Adinolfi E, et al. (2012) The P2X7 receptor is a key modulator of aerobic glycolysis. Cell Death Dis 3: e370. https://doi.org/10.1038/cddis.2012.105 ![]() |
[196] |
Schenk U, Frascoli M, Proietti M, et al. (2011) ATP inhibits the generation and function of regulatory T cells through the activation of purinergic P2X receptors. Sci Signal 4: ra12. https://doi.org/10.1126/scisignal.2001270 ![]() |
[197] |
Figliuolo VR, Savio LEB, Safya H, et al. (2017) P2X7 receptor promotes intestinal inflammation in chemically induced colitis and triggers death of mucosal regulatory T cells. Biochim Biophys Acta Mol Basis Dis 1863: 1183-1194. https://doi.org/10.1016/j.bbadis.2017.03.004 ![]() |
[198] |
Koo TY, Lee JG, Yan JJ, et al. (2017) The P2X7 receptor antagonist, oxidized adenosine triphosphate, ameliorates renal ischemia-reperfusion injury by expansion of regulatory T cells. Kidney Int 92: 415-431. https://doi.org/10.1016/j.kint.2017.01.031 ![]() |
[199] |
Hubert S, Rissiek B, Klages K, et al. (2010) Extracellular NAD+ shapes the Foxp3+ regulatory T cell compartment through the ART2-P2X7 pathway. J Exp Med 207: 2561-2568. https://doi.org/10.1084/jem.20091154 ![]() |
[200] |
Wilhelm K, Ganesan J, Muller T, et al. (2010) Graft-versus-host disease is enhanced by extracellular ATP activating P2X7R. Nat Med 16: 1434-1438. https://doi.org/10.1038/nm.2242 ![]() |
[201] |
Elhage A, Cuthbertson P, Sligar C, et al. (2023) A species-specific anti-human P2X7 monoclonal antibody reduces Graft-versus-Host disease in humanised mice. Pharmaceutics 15. https://doi.org/10.3390/pharmaceutics15092263 ![]() |
[202] |
Raffaghello L, Principi E, Baratto S, et al. (2022) P2X7 receptor antagonist reduces fibrosis and inflammation in a mouse model of alpha-sarcoglycan muscular dystrophy. Pharmaceuticals (Basel) 15. https://doi.org/10.3390/ph15010089 ![]() |
[203] |
Pupovac A, Foster CM, Sluyter R (2013) Human P2X7 receptor activation induces the rapid shedding of CXCL16. Biochem Biophys Res Commun 432: 626-631. https://doi.org/10.1016/j.bbrc.2013.01.134 ![]() |
[204] |
Pupovac A, Geraghty NJ, Watson D, et al. (2015) Activation of the P2X7 receptor induces the rapid shedding of CD23 from human and murine B cells. Immunol Cell Biol 93: 77-85. https://doi.org/10.1038/icb.2014.69 ![]() |
[205] |
Yip L, Woehrle T, Corriden R, et al. (2009) Autocrine regulation of T-cell activation by ATP release and P2X7 receptors. FASEB J 23: 1685-1693. https://doi.org/10.1096/fj.08-126458 ![]() |
[206] |
Huang SW, Walker C, Pennock J, et al. (2017) P2X7 receptor-dependent tuning of gut epithelial responses to infection. Immunol Cell Biol 95: 178-188. https://doi.org/10.1038/icb.2016.75 ![]() |
[207] |
Gu BJ, Wiley JS (2006) Rapid ATP-induced release of matrix metalloproteinase 9 is mediated by the P2X7 receptor. Blood 107: 4946-4953. https://doi.org/10.1182/blood-2005-07-2994 ![]() |
[208] |
de Torre-Minguela C, Barbera-Cremades M, Gomez AI, et al. (2016) Macrophage activation and polarization modify P2X7 receptor secretome influencing the inflammatory process. Sci Rep 6: 22586. https://doi.org/10.1038/srep22586 ![]() |
[209] |
Csoka B, Nemeth ZH, Toro G, et al. (2015) Extracellular ATP protects against sepsis through macrophage P2X7 purinergic receptors by enhancing intracellular bacterial killing. Faseb j 29: 3626-3637. https://doi.org/10.1096/fj.15-272450 ![]() |
[210] |
Wareham KJ, Seward EP (2016) P2X7 receptors induce degranulation in human mast cells. Purinergic Signal 12: 235-246. https://doi.org/10.1007/s11302-016-9497-4 ![]() |
[211] |
Kawamura H, Aswad F, Minagawa M, et al. (2006) P2X7 receptors regulate NKT cells in autoimmune hepatitis. J Immunol 176: 2152-2160. https://doi.org/10.4049/jimmunol.176.4.2152 ![]() |
[212] |
Martel-Gallegos G, Rosales-Saavedra MT, Reyes JP, et al. (2010) Human neutrophils do not express purinergic P2X7 receptors. Purinergic Signal 6: 297-306. https://doi.org/10.1007/s11302-010-9178-7 ![]() |
[213] |
Pelegrin P, Surprenant A (2006) Pannexin-1 mediates large pore formation and interleukin-1beta release by the ATP-gated P2X7 receptor. Embo j 25: 5071-5082. https://doi.org/10.1038/sj.emboj.7601378 ![]() |
[214] |
Suh BC, Kim JS, Namgung U, et al. (2001) P2X7 nucleotide receptor mediation of membrane pore formation and superoxide generation in human promyelocytes and neutrophils. J Immunol 166: 6754-6763. https://doi.org/10.4049/jimmunol.166.11.6754 ![]() |
[215] |
Tsukimoto M, Maehata M, Harada H, et al. (2006) P2X7 receptor-dependent cell death is modulated during murine T cell maturation and mediated by dual signaling pathways. J Immunol 177: 2842-2850. https://doi.org/10.4049/jimmunol.177.5.2842 ![]() |
[216] |
Aswad F, Dennert G (2006) P2X7 receptor expression levels determine lethal effects of a purine based danger signal in T lymphocytes. Cell Immunol 243: 58-65. https://doi.org/10.1016/j.cellimm.2006.12.003 ![]() |
[217] |
Scheuplein F, Schwarz N, Adriouch S, et al. (2009) NAD+ and ATP released from injured cells induce P2X7-dependent shedding of CD62L and externalization of phosphatidylserine by murine T cells. J Immunol 182: 2898-2908. https://doi.org/10.4049/jimmunol.0801711 ![]() |
[218] |
Albayati S, Vemulapalli H, Tsygankov AY, et al. (2021) P2Y(12) antagonism results in altered interactions between platelets and regulatory T cells during sepsis. J Leukoc Biol 110: 141-153. https://doi.org/10.1002/JLB.3A0220-097R ![]() |
[219] |
Vemulapalli H, Samara A, Tsygankov AY, et al. (2018) Blockade of the P2Y12 signaling pathway with clopidogrel restrains treg proliferation in vivo during sepsis and in Vitro. Blood 132: 2413. https://doi.org/10.1182/blood-2018-99-114884 ![]() |
[220] |
Minns MS, Trinkaus-Randall V (2016) Purinergic signaling in corneal wound healing: A tale of 2 receptors. J Ocul Pharmacol Ther 32: 498-503. https://doi.org/10.1089/jop.2016.0009 ![]() |
[221] |
Hwang SM, Koo NY, Choi SY, et al. (2009) P2X7 receptor-mediated membrane blebbing in salivary epithelial cells. Korean J Physiol Pharmacol 13: 175-179. https://doi.org/10.4196/kjpp.2009.13.3.175 ![]() |
[222] |
Gu BJ, Wiley JS (2018) P2X7 as a scavenger receptor for innate phagocytosis in the brain. Br J Pharmacol 175: 4195-4208. https://doi.org/10.1111/bph.14470 ![]() |
[223] |
Okura D, Horishita T, Ueno S, et al. (2015) Lidocaine preferentially inhibits the function of purinergic P2X7 receptors expressed in Xenopus oocytes. Anesth Analg 120: 597-605. https://doi.org/10.1213/ANE.0000000000000585 ![]() |
[224] |
North RA (2016) P2X receptors. Philos Trans R Soc Lond B Biol Sci 371. https://doi.org/10.1098/rstb.2015.0427 ![]() |
[225] |
Coddou C, Stojilkovic SS, Huidobro-Toro JP (2011) Allosteric modulation of ATP-gated P2X receptor channels. Rev Neurosci 22: 335-354. https://doi.org/10.1515/rns.2011.014 ![]() |
[226] |
Coddou C, Yan Z, Obsil T, et al. (2011) Activation and regulation of purinergic P2X receptor channels. Pharmacol Rev 63: 641-683. https://doi.org/10.1124/pr.110.003129 ![]() |
[227] |
Sanabria P, Ross E, Ramirez E, et al. (2008) P2Y2 receptor desensitization on single endothelial cells. Endothelium 15: 43-51. https://doi.org/10.1080/10623320802092294 ![]() |
[228] |
Schulte G, Fredholm BB (2000) Human adenosine A(1), A(2A), A(2B), and A(3) receptors expressed in Chinese hamster ovary cells all mediate the phosphorylation of extracellular-regulated kinase 1/2. Mol Pharmacol 58: 477-482. https://doi.org/10.1124/mol.58.3.477 ![]() |
[229] |
Klaasse EC, Ijzerman AP, de Grip WJ, et al. (2008) Internalization and desensitization of adenosine receptors. Purinergic Signal 4: 21-37. https://doi.org/10.1007/s11302-007-9086-7 ![]() |
[230] |
Illes P, Müller CE, Jacobson KA, et al. (2021) Update of P2X receptor properties and their pharmacology: IUPHAR Review 30. Br J Pharmacol 178: 489-514. https://doi.org/10.1111/bph.15299 ![]() |
[231] |
Jacobson KA, Delicado EG, Gachet C, et al. (2020) Update of P2Y receptor pharmacology: IUPHAR Review 27. Br J Pharmacol 177: 2413-2433. https://doi.org/10.1111/bph.15005 ![]() |
[232] |
Jacobson KA, Muller CE (2016) Medicinal chemistry of adenosine, P2Y and P2X receptors. Neuropharmacology 104: 31-49. https://doi.org/10.1016/j.neuropharm.2015.12.001 ![]() |
[233] |
Myers AJ, Eilertson B, Fulton SA, et al. (2005) The purinergic P2X7 receptor Is not required for control of pulmonary Mycobacterium tuberculosis infection. Infect Immun 73: 3192-3195. https://doi.org/10.1128/IAI.73.5.3192-3195.2005 ![]() |
[234] |
Santiago-Carvalho I, de Almeida-Santos G, Bomfim CCB, et al. (2021) P2x7 receptor signaling blockade reduces lung inflammation and necrosis during severe experimental tuberculosis. Front Cell Infect Microbiol 11: 672472. https://doi.org/10.3389/fcimb.2021.672472 ![]() |
[235] |
Ardissone V, Radaelli E, Zaratin P, et al. (2011) Pharmacologic P2X purinergic receptor antagonism in the treatment of collagen-induced arthritis. Arthritis Rheum 63: 3323-3332. https://doi.org/10.1002/art.30556 ![]() |
[236] |
Martinez CG, Zamith-Miranda D, da Silva MG, et al. (2015) P2×7 purinergic signaling in dilated cardiomyopathy induced by auto-immunity against muscarinic M2 receptors: autoantibody levels, heart functionality and cytokine expression. Sci Rep 5: 16940. https://doi.org/10.1038/srep16940 ![]() |
[237] |
Baldini C, Santini E, Rossi C, et al. (2017) The P2X7 receptor-NLRP3 inflammasome complex predicts the development of non-Hodgkin's lymphoma in Sjogren's syndrome: a prospective, observational, single-centre study. J Intern Med 282: 175-186. https://doi.org/10.1111/joim.12631 ![]() |
[238] |
Baldini C, Rossi C, Ferro F, et al. (2013) The P2X7 receptor-inflammasome complex has a role in modulating the inflammatory response in primary Sjögren's syndrome. J Intern Med 274: 480-489. https://doi.org/10.1111/joim.12115 ![]() |
[239] |
Di Virgilio F, Bronte V, Collavo D, et al. (1989) Responses of mouse lymphocytes to extracellular adenosine 5′-triphosphate (ATP). Lymphocytes with cytotoxic activity are resistant to the permeabilizing effects of ATP. J Immunol 143: 1955-1960. https://doi.org/10.4049/jimmunol.143.6.1955 ![]() |
[240] |
Filippini A, Taffs RE, Agui T, et al. (1990) Ecto-ATPase activity in cytolytic T-lymphocytes. Protection from the cytolytic effects of extracellular ATP. J Biol Chem 265: 334-340. https://doi.org/10.1016/S0021-9258(19)40234-2 ![]() |
[241] |
Falcone C, Caracciolo M, Correale P, et al. (2020) Can adenosine fight COVID-19 acute respiratory distress syndrome?. J Clin Med 9. https://doi.org/10.20944/preprints202007.0426.v1 ![]() |
[242] |
Fajgenbaum DC, June CH (2020) Cytokine Storm. N Engl J Med 383: 2255-2273. https://doi.org/10.1056/NEJMra2026131 ![]() |
[243] |
Young CNJ, Chira N, Róg J, et al. (2018) Sustained activation of P2X7 induces MMP-2-evoked cleavage and functional purinoceptor inhibition. J Mol Cell Biol 10: 229-242. https://doi.org/10.1093/jmcb/mjx030 ![]() |
[244] |
Young CNJ, Górecki DC (2018) P2RX7 purinoceptor as a therapeutic target-the second coming?. Front Chem 6: 248. https://doi.org/10.3389/fchem.2018.00248 ![]() |
[245] |
Zhao R, Qiao J, Zhang X, et al. (2019) Toll-like receptor-mediated activation of CD39 internalization in BMDCs leads to extracellular ATP accumulation and facilitates P2X7 receptor activation. Front Immunol 10: 2524. https://doi.org/10.3389/fimmu.2019.02524 ![]() |
[246] |
Hotchkiss RS, Monneret G, Payen D (2013) Immunosuppression in sepsis: a novel understanding of the disorder and a new therapeutic approach. Lancet Infect Dis 13: 260-268. https://doi.org/10.1016/S1473-3099(13)70001-X ![]() |
[247] |
Hotchkiss RS, Monneret G, Payen D (2013) Sepsis-induced immunosuppression: from cellular dysfunctions to immunotherapy. Nat Rev Immunol 13: 862-874. https://doi.org/10.1038/nri3552 ![]() |
[248] |
Ward NS, Casserly B, Ayala A (2008) The compensatory anti-inflammatory response syndrome (CARS) in critically ill patients. Clin Chest Med 29: 617-625. https://doi.org/10.1016/j.ccm.2008.06.010 ![]() |
[249] |
Bone RC (1996) Sir Isaac Newton, sepsis, SIRS, and CARS. Crit Care Med 24: 1125-1128. https://doi.org/10.1097/00003246-199607000-00010 ![]() |
[250] |
Biscetti L, De Vanna G, Cresta E, et al. (2021) Headache and immunological/autoimmune disorders: a comprehensive review of available epidemiological evidence with insights on potential underlying mechanisms. J Neuroinflammation 18: 259. https://doi.org/10.1186/s12974-021-02229-5 ![]() |
[251] |
John S, Hajj-Ali RA (2014) Headache in autoimmune diseases. Headache 54: 572-582. https://doi.org/10.1111/head.12306 ![]() |
[252] |
Kim HJ (2021) Pruritus in autoimmune connective tissue diseases. Ann Transl Med 9: 441. https://doi.org/10.21037/atm-20-4894 ![]() |
[253] |
Zielinski MR, Systrom DM, Rose NR (2019) Fatigue, sleep, and autoimmune and related disorders. Front Immunol 10: 1827. https://doi.org/10.3389/fimmu.2019.01827 ![]() |
[254] |
Morris G, Berk M, Walder K, et al. (2015) Central pathways causing fatigue in neuro-inflammatory and autoimmune illnesses. BMC Med 13: 28. https://doi.org/10.1186/s12916-014-0259-2 ![]() |
[255] |
Mifflin KA, Kerr BJ (2017) Pain in autoimmune disorders. J Neurosci Res 95: 1282-1294. https://doi.org/10.1002/jnr.23844 ![]() |
[256] |
Lacagnina MJ, Heijnen CJ, Watkins LR, et al. (2021) Autoimmune regulation of chronic pain. Pain Rep 6: e905. https://doi.org/10.1097/PR9.0000000000000905 ![]() |
[257] |
Joaquim AF, Appenzeller S (2015) Neuropsychiatric manifestations in rheumatoid arthritis. Autoimmun Rev 14: 1116-1122. https://doi.org/10.1016/j.autrev.2015.07.015 ![]() |
[258] |
Shim CH, Cho S, Shin YM, et al. (2022) Emerging role of bystander T cell activation in autoimmune diseases. BMB Rep 55: 57-64. https://doi.org/10.5483/BMBRep.2022.55.2.183 ![]() |
[259] |
Fujinami RS, von Herrath MG, Christen U, et al. (2006) Molecular mimicry, bystander activation, or viral persistence: infections and autoimmune disease. Clin Microbiol Rev 19: 80-94. https://doi.org/10.1128/CMR.19.1.80-94.2006 ![]() |
[260] |
van Aalst S, Ludwig IS, van der Zee R, et al. (2017) Bystander activation of irrelevant CD4+ T cells following antigen-specific vaccination occurs in the presence and absence of adjuvant. PLoS One 12: e0177365. https://doi.org/10.1371/journal.pone.0177365 ![]() |
[261] |
Bergamaschi L, Mescia F, Turner L, et al. (2021) Longitudinal analysis reveals that delayed bystander CD8+ T cell activation and early immune pathology distinguish severe COVID-19 from mild disease. Immunity 54: 1257-1275.e1258. https://doi.org/10.1016/j.immuni.2021.05.010 ![]() |
[262] |
Lindenbergh MFS, Koerhuis DGJ, Borg EGF, et al. (2019) Bystander T-Cells support clonal T-cell activation by controlling the release of dendritic cell-derived immune-stimulatory extracellular vesicles. Front Immunol 10: 448. https://doi.org/10.3389/fimmu.2019.00448 ![]() |
[263] |
Wang JY, Zhang W, Roehrl VB, et al. (2022) An autoantigen atlas from human lung HFL1 cells offers clues to neurological and diverse autoimmune manifestations of COVID-19. Front Immunol 13: 831849. https://doi.org/10.3389/fimmu.2022.831849 ![]() |
[264] |
Felipe Cuspoca A, Isaac Estrada P, Velez-van-Meerbeke A (2022) Molecular mimicry of SARS-CoV-2 spike protein in the nervous system: A bioinformatics approach. Comput Struct Biotechnol J 20: 6041-6054. https://doi.org/10.1016/j.csbj.2022.10.022 ![]() |
[265] |
Woodruff MC, Ramonell RP, Haddad NS, et al. (2022) Dysregulated naive B cells and de novo autoreactivity in severe COVID-19. Nature 611: 139-147. https://doi.org/10.1038/s41586-022-05273-0 ![]() |
[266] |
Milne GR, Palmer TM (2011) Anti-inflammatory and immunosuppressive effects of the A2A adenosine receptor. Sci World J 11: 320-339. https://doi.org/10.1100/tsw.2011.22 ![]() |
[267] |
Elsaghir A, El-Sabaa EMW, Ahmed AK, et al. (2023) The role of cluster of differentiation 39 (CD39) and purinergic signaling pathway in viral infections. Pathogens 12. https://doi.org/10.3390/pathogens12020279 ![]() |
[268] |
Auclair H, Ouk-Martin C, Roland L, et al. (2019) EBV latency III-transformed B cells are inducers of conventional and unconventional regulatory T cells in a PD-L1-dependent manner. J Immunol 203: 1665-1674. https://doi.org/10.4049/jimmunol.1801420 ![]() |
[269] | Song J-W, Huang H-H, Zhang C, et al. (2019) Expression of CD39 is correlated with HIV DNA levels in naïve tregs in chronically infected ART naïve patients. Front Immunol 10. https://doi.org/10.3389/fimmu.2019.02465 |
[270] | Gupta PK, Godec J, Wolski D, et al. (2015) CD39 expression identifies terminally exhausted CD8+ T cells. PLoSPathog 11: e1005177. https://doi.org/10.1371/journal.ppat.1005177 |
[271] |
Wingate PJ, McAulay KA, Anthony IC, et al. (2009) Regulatory T cell activity in primary and persistent Epstein-Barr virus infection. J Med Virol 81: 870-877. https://doi.org/10.1002/jmv.21445 ![]() |
[272] |
Chevalier MF, Weiss L (2013) The split personality of regulatory T cells in HIV infection. Blood 121: 29-37. https://doi.org/10.1182/blood-2012-07-409755 ![]() |
[273] |
Wang L, Qiu J, Yu L, et al. (2014) Increased numbers of CD5+CD19+CD1dhighIL-10+ Bregs, CD4+Foxp3+ Tregs, CD4+CXCR5+Foxp3+ follicular regulatory T (TFR) cells in CHB or CHC patients. J Transl Med 12: 251. https://doi.org/10.1186/s12967-014-0251-9 ![]() |
[274] |
Cash E, Goodwin AT, Tatler AL (2023) Adenosine receptor signalling as a driver of pulmonary fibrosis. Pharmacol Ther 249: 108504. https://doi.org/10.1016/j.pharmthera.2023.108504 ![]() |
[275] |
Fausther M (2018) Extracellular adenosine: a critical signal in liver fibrosis. Am J Physiol Gastrointest Liver Physiol 315: G12-G19. https://doi.org/10.1152/ajpgi.00006.2018 ![]() |
[276] |
Sheng G, Chen P, Wei Y, et al. (2020) Viral infection increases the risk of idiopathic pulmonary fibrosis: A meta-analysis. Chest 157: 1175-1187. https://doi.org/10.1016/j.chest.2019.10.032 ![]() |
[277] |
Androutsakos T, Schina M, Pouliakis A, et al. (2020) Causative factors of liver fibrosis in HIV-infected patients. A single center study. BMC Gastroenterol 20: 91. https://doi.org/10.1186/s12876-020-01230-1 ![]() |
[278] |
McCaughan GW, George J (2004) Fibrosis progression in chronic hepatitis C virus infection. Gut 53: 318-321. https://doi.org/10.1136/gut.2003.026393 ![]() |
[279] |
Rockey DC, Friedman SL (2021) Fibrosis regression after eradication of hepatitis C virus: From bench to bedside. Gastroenterology 160: 1502-1520.e1501. https://doi.org/10.1053/j.gastro.2020.09.065 ![]() |
[280] |
Stock TC, Bloom BJ, Wei N, et al. (2012) Efficacy and safety of CE-224,535, an antagonist of P2X7 receptor, in treatment of patients with rheumatoid arthritis inadequately controlled by methotrexate. J Rheumatol 39: 720-727. https://doi.org/10.3899/jrheum.110874 ![]() |
[281] |
Keystone EC, Wang MM, Layton M, et al. (2012) Clinical evaluation of the efficacy of the P2X7 purinergic receptor antagonist AZD9056 on the signs and symptoms of rheumatoid arthritis in patients with active disease despite treatment with methotrexate or sulphasalazine. Ann Rheum Dis 71: 1630-1635. https://doi.org/10.1136/annrheumdis-2011-143578 ![]() |
[282] |
Recourt K, de Boer P, van der Ark P, et al. (2023) Characterization of the central nervous system penetrant and selective purine P2X7 receptor antagonist JNJ-54175446 in patients with major depressive disorder. Transl Psychiatry 13: 266. https://doi.org/10.1038/s41398-023-02557-5 ![]() |
[283] |
Tamargo J, Le Heuzey JY, Mabo P (2015) Narrow therapeutic index drugs: a clinical pharmacological consideration to flecainide. Eur J Clin Pharmacol 71: 549-567. https://doi.org/10.1007/s00228-015-1832-0 ![]() |
[284] | Eser A, Colombel JF, Rutgeerts P, et al. (2015) Safety and efficacy of an oral inhibitor of the purinergic receptor P2X7 in adult patients with moderately to severely active crohn's disease: A randomized placebo-controlled, double-blind, phase IIa study. Inflamm Bowel Dis 21: 2247-2253. https://doi.org/10.1097/MIB.0000000000000514 |
[285] |
Neves AR, Castelo-Branco MT, Figliuolo VR, et al. (2014) Overexpression of ATP-activated P2X7 receptors in the intestinal mucosa is implicated in the pathogenesis of Crohn's disease. Inflamm Bowel Dis 20: 444-457. https://doi.org/10.1097/01.MIB.0000441201.10454.06 ![]() |
Purinergic receptors and their ligands [155]–[159] | |
Natural ligands | Receptors |
Adenosine | AdoRA1, AdoRA2A, AdoRA2B, and AdoR3 |
ATP | P2X1R, P2X2R, P2X3R, P2X4R, P2X5R, P2X7R, and P2Y11R |
ADP | P2Y12R |
ADP and ATP (ADP > ATP) | P2Y1R and P2Y13R |
UDP, UTP, and ATP (UDP > UTP >> ATP) | P2Y6R |
UDP | P2Y14R |
UTP and ATP (UTP > ATP) | P2Y2R and P2Y4R |
ATP | P2X6R molecules are mostly retained in the endoplasmic reticulum. They do not exit the endoplasmic reticulum as a homopolymer but do so as a heteropolymer (with P2X2R and/or P2X4R) or after enhanced glycosylation-induced improved trafficking of P2X6R homopolymers. |
After exiting the endoplasmic reticulum and becoming inserted into the cell membrane, P2X6R can be activated by extracellular ATP. |
Examples of the effects of extracellular adenosine, ATP, and ADP on select immune cells through the activation of three purinergic receptors (AdoRA2A, P2X7R, and P2Y12R) |
|||||
Row number | Receptor | Ligand [149] | Immune cell type or experimental model | Results of receptor signaling | Reference number |
1 | AdorA2A | CGS-21680 | Mouse naïve T cells | Promotes differentiation toward CD4+FoxP3+Lag3+ Tregs, inhibits Th1 and Th17 differentiation, inhibits IL-6 secretion, and increases TGF-β secretion. | [173] |
2 | CGS-21680 and NECA | Mouse CD4+CD25+FoxP3+ Tregs | Increases the number of Tregs and increases CTLA-4 receptor expression. Upregulates expression of the ectoenzymes CD39 and CD73, accelerating adenosine generation from extracellular ATP. | [174] | |
3 | Adenosine | Human CD4+CD25+CD127low/–Tregs and CD8+ T cells | Tregs from gastric cancer patients hydrolyze ATP to generate adenosine. Adenosine synthesized by Tregs promotes apoptosis and suppresses the proliferation of CD8+ T cells. Tregs reduce CD8+ T cell activity by promoting cAMP synthesis. Tregs inhibit the immune function of CD8+ T cells through the AdoRA2A pathway. | [175] | |
4 | P2X7R | Inactivated state in the absence of ATP | Macrophages and P2X7R-transfected HEK-293 cells | P2X7 is a scavenger receptor for apoptotic cells in the absence of its ligand ATP. | [176],[177] |
5 | P2X7R | ATP-release channel | Alveolar epithelial type I cells (AT I cells), murine osteoclast cells, murine neuroblastoma cells, astrocytic cell line, murine astrocytes, and B16 melanoma cells | Release ATP after mechanical deformation (AT I cells), spontaneously (osteoblast cells), after activation (neuroblastoma cells, astrocytic cell line), and after γ irradiation (melanoma cells). | [178]–[183] |
6 | Mouse 3T3 fibroblasts | P2X7R-mediated ATP secretion is accompanied by depletion of cytosolic ATP. | [184] | ||
7 | Bone marrow–derived DCs from WT mice and Panx1−/− C57BL/6 mice | Upon stimulation of the P2X7 receptor by ATP, Panx-1 contributes to rapid DC motility by increasing the permeability of the plasma membrane, which results in supplementary ATP release. | [185] | ||
8 | Murine macrophages and RAW 264.7 macrophages | Infection with Leishmania donovani substantially increases extracellular release of ATP. | [186] | ||
9 | A549 alveolar epithelial cells | Infection with human rhinovirus RV-16 increases basal extracellular ATP release and ATP release after a second stimulation by hypotonic and isotonic solutions. | [187] | ||
10 | Mouse influenza model | Influenza infection increases plasma ATP levels 3-fold and pulmonary ATP levels 5-fold. | [188] | ||
11 | RAW 264.7 macrophages and L929 fibroblasts | Infection with vesicular stomatitis virus (VSV) increases extracellular ATP in a time-dependent manner. | [189] | ||
12 | P2X7R | ATP, low tonic basal activation | HEK 293 and HELA cells | Increases mitochondrial calcium level, membrane potential, and cellular ATP levels, and promotes serum-independent growth. This process requires an intact pore-forming function. | [190] |
13 | In vitro scratch wound assay with HaCat cells (human skin keratinocytes) | Medium hyaluronan fragment (MMW-HA, between 100 and 300 kD) increases expression of the tight junction protein ZO-1 and induces a low activation of the P2X7 receptor, resulting in improved closure of the wound. This is accompanied by pore formation, as shown with Yo-Pro-1 cellular uptake. The P2X7R antagonist brilliant blue G (BBG) completely inhibits this process. | [191] | ||
14 | HEK293 and NIH3T3 cells | Increases the Ca2+ content of the endoplasmic reticulum, activates NFATc1, and protects from apoptosis. | [192] | ||
15 | PC3 cells LNCaP, Kelly, RPMI-8226, DU145, and SK-MEL-5 cells | Drives the expression of nfP2X7, a key mediator of cell survival. | [193] | ||
16 | Human osteoclast-like cells | Promotes an increase in extracellular adenosine concentrations. | [194] | ||
17 | HEK293 cells | Initiates anaerobic glycolysis independent of the oxygen content. Upregulates glucose transporter Glut1 (thus enhancing intracellular glycogen stores). Upregulates glycolytic enzymes (PFK, G3PDH, PKM2), phosphorylated Akt/PKB, and hypoxia-inducible factor 1a (HIF-1a) expression. Impedes the Krebs cycle independent of oxygen concentrations by promoting pyruvate dehydrogenase kinase 1 (PDHK1) and inhibiting pyruvate dehydrogenase (PDH, conversion of pyruvate to acetyl-CoA). | [195] | ||
18 | P2X7R | ATP ≥ 100 µM | C57BL/6 mice | P2X7 activation inhibits the suppressive potential and stability of Tregs and promotes the conversion of Tregs to Th17 cells in vivo. In contrast, P2X7R inhibition promotes the conversion of CD4+ T cells into Tregs after stimulation of their T cell receptors (TCRs). | [196] |
19 | C57BL/6 wild type and P2X7 knockout mice | P2X7 knockout mice show an increase in the number of CD90/CD45RBlow FoxP3+ Tregs in the colon lamina propria, with prevention of Treg death in mesenteric lymph nodes, and these Tregs produce more IL-10. Colitis is prevented or reduced in P2X7 knockout mice. Treg cells lacking the P2X7 receptor have higher levels of integrin CD103. ATP activation of P2X7R triggers Treg death. | [197] | ||
20 | C57BL/6 mice, renal ischemia–reperfusion injury | P2X7R antagonist oATP prior to renal ischemia increases renal Foxp3DCD4D Treg infiltration and reduces IL-6 and CCL2 levels. | |||
oATP treatment following injury improves renal function, decreases the infiltration of innate and adaptive effector cells, increases the renal infiltration of Foxp3+CD4+ Tregs, increases tubular cell proliferation, and reduces renal fibrosis. | [198] | ||||
21 | C57BL/6 mice | P2X7R activation reduces the frequency of Tregs, and P2X7R inhibition increases the expansion of Tregs. | [198] | ||
22 | CD4+CD25+FoxP3+ Tregs | Facilitates NAD+-induced Treg depletion through the ART2-P2X7 pathway. | [199] | ||
23 | Humanized mice model GVHD, HaCat cell, Jurkat cells (E6-1 clone), and mouse DCs | Increases CD80, CD 86, STAT-1, and P2X7R expression; IFN-β release; and T cell expansion. Reduces Treg numbers. | [200] | ||
24 | Humanized mice model GVHD | Anti-hP2X7 monoclonal antibodies increase human Treg and human NK cell numbers on Day 21 and reduce clinical and histological GVHD in the liver and lung compared to the control treatment at the disease endpoint. | [201] | ||
25 | LGMDR3 (Limb-girdle muscular dystrophy R3) mice with α-sarcoglycan gene (SGCA) knockout | P2X7 antagonist A438079 improves mouse motor function and decreases serum creatine kinase levels, reduces the percentage of central nuclei, reduces fiber size variability, and reduces the extent of local fibrosis and inflammation significantly. Flow cytometric characterization of muscle inflammatory infiltrates revealed significantly decreased numbers of innate immune cells and increased numbers of Tregs. | [202] | ||
26 | Mouse CD4+CD25+FoxP3+ Tregs | P2X7R activation facilitates NAD+-induced Treg depletion through the ART2-P2X7 pathway. | [199] | ||
27 | BALB/c mice GVHD model, DCs | Activation of P2X7Rs increases CD80, CD 86, STAT-1, and P2X7R expression, IFN-β release, and effector T cell expansion. Reduces Treg numbers. | |||
P2X7R blockade in GVHD improves survival. | [200] | ||||
28 | Mouse, human, murine B cells | Induces shedding of IgE receptor (CD23) and CXCL16. Soluble CD23 sustains growth of B cell precursors, promotes B cell and T cell differentiation, and drives cytokine release from monocytes. CXCL16 is a chemoattractant for lymphocytes. | [203],[204] | ||
29 | Jurkat cells (E6-1 clone) Naïve T cells | TCR stimulation triggers rapid release of ATP and upregulates P2X7 gene expression. Autocrine ATP stimulation through the P2X7R is required for TCR-mediated calcium influx, NFAT activation, and IL-2 production. | [205] | ||
30 | Wild-type and Panx-1 knockout C57BL/6 mice, bone marrow–derived DCs | ATP promotes the rapid migration of DCs through the activity of pannexin 1 channels and P2X7 receptors. | [185] | ||
31 | Mouse and P2X7 knockout mice, CD11c+CD103+ DCs | Mediates rapid infection-induced recruitment of CD11c+CD103+ DC subsets into the epithelial layer of the gut. | [206] | ||
32 | DCs cultured from murine bone marrow precursor cells | Induces autocrine-mediated (pannexin-1 channels) rapid migration of DCs through reorganization of the actin cytoskeleton. | [185] | ||
33 | Human monocytes | Induces MMP-9 and TIMP-1 release. | [207] | ||
34 | Wild-type and P2X7 knockout C57BL/6 mice, M1 macrophages | Induces release of 74 proinflammatory proteins detected by an antibody array and 33 inflammatory proteins detected by LC–MS/MS. | [208] | ||
35 | Wild-type and P2X7 knockout C57BL/6 mice M2 macrophages | Induces release of 21 anti-inflammatory proteins detected by LC–MS/MS. | [208] | ||
36 | CLP-induced sepsis in mice, macrophages | Enhances intracellular bacterial killing. | [209] | ||
37 | Macrophages and P2X7R-transfected HEK-293 cells | Mediates rapid uptake of beads and bacteria in the absence of serum after ATP activation. | |||
38 | Human mast cells | Induces degranulation. | [210] | ||
39 | Wild-type and P2X7 knockout C57BL/6 mice, naïve NKTs | Facilitates NAD+-induced inhibitory signaling through the ART2-P2X7 pathway, resulting in nonfunctional NKTs. | [211] | ||
40 | Wild-type and P2X7 knockout C57BL/6 mice, activated NKTs | Facilitates NAD+-induced stimulatory signaling through the ART2-P2X7 pathway, resulting in functional NKTs with increased IFN-γ and IL-4 release. | [211] | ||
41 | Human peripheral neutrophils | Human CAP18/LL-37 suppresses neutrophil apoptosis through the activation of formyl-peptide receptor-like 1 and P2X7R. | [212] | ||
42 | P2X7R | ATP > 1 mM, prolonged vigorous activation | Macrophages, HeLa cells, 1321N1 astrocytes, and HEK293 cells | Induces Panx-1-mediated large pore formation and interleukin-1 beta release. | [213] |
43 | Human neutrophils and HL-60 cells | Mediates large pore formation and superoxide generation. | [214] | ||
44 | Female C57BL/6 (B6) and BALB/c mice, ART2 knockout BALB/c mice, P2X7 knockout C57BL/6 mice, mature peripheral T cells | High-dose ATP promotes apoptosis, cell death, and CD62L shedding (homing receptor for central T cells) independent of the NAD+-induced ART2-P2X7 pathway. | [215]–[217] | ||
45 | J774 cells and HEK cells expressing the P2X7 receptor | Promotes the formation of pores permeable to very large ions, leading to cytolysis | [151] | ||
46 | P2Y12R | ADP > ATP | Murine model of sepsis, cecal ligation, and puncture (CLP). Cocultures of human platelets and T cells with or without anti-CD3/CD28 | Blockade of the P2Y12 signaling pathway restrains Treg proliferation in vivo and in vitro. | [218],[219] |
Purinergic receptors and their ligands [155]–[159] | |
Natural ligands | Receptors |
Adenosine | AdoRA1, AdoRA2A, AdoRA2B, and AdoR3 |
ATP | P2X1R, P2X2R, P2X3R, P2X4R, P2X5R, P2X7R, and P2Y11R |
ADP | P2Y12R |
ADP and ATP (ADP > ATP) | P2Y1R and P2Y13R |
UDP, UTP, and ATP (UDP > UTP >> ATP) | P2Y6R |
UDP | P2Y14R |
UTP and ATP (UTP > ATP) | P2Y2R and P2Y4R |
ATP | P2X6R molecules are mostly retained in the endoplasmic reticulum. They do not exit the endoplasmic reticulum as a homopolymer but do so as a heteropolymer (with P2X2R and/or P2X4R) or after enhanced glycosylation-induced improved trafficking of P2X6R homopolymers. |
After exiting the endoplasmic reticulum and becoming inserted into the cell membrane, P2X6R can be activated by extracellular ATP. |
Examples of the effects of extracellular adenosine, ATP, and ADP on select immune cells through the activation of three purinergic receptors (AdoRA2A, P2X7R, and P2Y12R) |
|||||
Row number | Receptor | Ligand [149] | Immune cell type or experimental model | Results of receptor signaling | Reference number |
1 | AdorA2A | CGS-21680 | Mouse naïve T cells | Promotes differentiation toward CD4+FoxP3+Lag3+ Tregs, inhibits Th1 and Th17 differentiation, inhibits IL-6 secretion, and increases TGF-β secretion. | [173] |
2 | CGS-21680 and NECA | Mouse CD4+CD25+FoxP3+ Tregs | Increases the number of Tregs and increases CTLA-4 receptor expression. Upregulates expression of the ectoenzymes CD39 and CD73, accelerating adenosine generation from extracellular ATP. | [174] | |
3 | Adenosine | Human CD4+CD25+CD127low/–Tregs and CD8+ T cells | Tregs from gastric cancer patients hydrolyze ATP to generate adenosine. Adenosine synthesized by Tregs promotes apoptosis and suppresses the proliferation of CD8+ T cells. Tregs reduce CD8+ T cell activity by promoting cAMP synthesis. Tregs inhibit the immune function of CD8+ T cells through the AdoRA2A pathway. | [175] | |
4 | P2X7R | Inactivated state in the absence of ATP | Macrophages and P2X7R-transfected HEK-293 cells | P2X7 is a scavenger receptor for apoptotic cells in the absence of its ligand ATP. | [176],[177] |
5 | P2X7R | ATP-release channel | Alveolar epithelial type I cells (AT I cells), murine osteoclast cells, murine neuroblastoma cells, astrocytic cell line, murine astrocytes, and B16 melanoma cells | Release ATP after mechanical deformation (AT I cells), spontaneously (osteoblast cells), after activation (neuroblastoma cells, astrocytic cell line), and after γ irradiation (melanoma cells). | [178]–[183] |
6 | Mouse 3T3 fibroblasts | P2X7R-mediated ATP secretion is accompanied by depletion of cytosolic ATP. | [184] | ||
7 | Bone marrow–derived DCs from WT mice and Panx1−/− C57BL/6 mice | Upon stimulation of the P2X7 receptor by ATP, Panx-1 contributes to rapid DC motility by increasing the permeability of the plasma membrane, which results in supplementary ATP release. | [185] | ||
8 | Murine macrophages and RAW 264.7 macrophages | Infection with Leishmania donovani substantially increases extracellular release of ATP. | [186] | ||
9 | A549 alveolar epithelial cells | Infection with human rhinovirus RV-16 increases basal extracellular ATP release and ATP release after a second stimulation by hypotonic and isotonic solutions. | [187] | ||
10 | Mouse influenza model | Influenza infection increases plasma ATP levels 3-fold and pulmonary ATP levels 5-fold. | [188] | ||
11 | RAW 264.7 macrophages and L929 fibroblasts | Infection with vesicular stomatitis virus (VSV) increases extracellular ATP in a time-dependent manner. | [189] | ||
12 | P2X7R | ATP, low tonic basal activation | HEK 293 and HELA cells | Increases mitochondrial calcium level, membrane potential, and cellular ATP levels, and promotes serum-independent growth. This process requires an intact pore-forming function. | [190] |
13 | In vitro scratch wound assay with HaCat cells (human skin keratinocytes) | Medium hyaluronan fragment (MMW-HA, between 100 and 300 kD) increases expression of the tight junction protein ZO-1 and induces a low activation of the P2X7 receptor, resulting in improved closure of the wound. This is accompanied by pore formation, as shown with Yo-Pro-1 cellular uptake. The P2X7R antagonist brilliant blue G (BBG) completely inhibits this process. | [191] | ||
14 | HEK293 and NIH3T3 cells | Increases the Ca2+ content of the endoplasmic reticulum, activates NFATc1, and protects from apoptosis. | [192] | ||
15 | PC3 cells LNCaP, Kelly, RPMI-8226, DU145, and SK-MEL-5 cells | Drives the expression of nfP2X7, a key mediator of cell survival. | [193] | ||
16 | Human osteoclast-like cells | Promotes an increase in extracellular adenosine concentrations. | [194] | ||
17 | HEK293 cells | Initiates anaerobic glycolysis independent of the oxygen content. Upregulates glucose transporter Glut1 (thus enhancing intracellular glycogen stores). Upregulates glycolytic enzymes (PFK, G3PDH, PKM2), phosphorylated Akt/PKB, and hypoxia-inducible factor 1a (HIF-1a) expression. Impedes the Krebs cycle independent of oxygen concentrations by promoting pyruvate dehydrogenase kinase 1 (PDHK1) and inhibiting pyruvate dehydrogenase (PDH, conversion of pyruvate to acetyl-CoA). | [195] | ||
18 | P2X7R | ATP ≥ 100 µM | C57BL/6 mice | P2X7 activation inhibits the suppressive potential and stability of Tregs and promotes the conversion of Tregs to Th17 cells in vivo. In contrast, P2X7R inhibition promotes the conversion of CD4+ T cells into Tregs after stimulation of their T cell receptors (TCRs). | [196] |
19 | C57BL/6 wild type and P2X7 knockout mice | P2X7 knockout mice show an increase in the number of CD90/CD45RBlow FoxP3+ Tregs in the colon lamina propria, with prevention of Treg death in mesenteric lymph nodes, and these Tregs produce more IL-10. Colitis is prevented or reduced in P2X7 knockout mice. Treg cells lacking the P2X7 receptor have higher levels of integrin CD103. ATP activation of P2X7R triggers Treg death. | [197] | ||
20 | C57BL/6 mice, renal ischemia–reperfusion injury | P2X7R antagonist oATP prior to renal ischemia increases renal Foxp3DCD4D Treg infiltration and reduces IL-6 and CCL2 levels. | |||
oATP treatment following injury improves renal function, decreases the infiltration of innate and adaptive effector cells, increases the renal infiltration of Foxp3+CD4+ Tregs, increases tubular cell proliferation, and reduces renal fibrosis. | [198] | ||||
21 | C57BL/6 mice | P2X7R activation reduces the frequency of Tregs, and P2X7R inhibition increases the expansion of Tregs. | [198] | ||
22 | CD4+CD25+FoxP3+ Tregs | Facilitates NAD+-induced Treg depletion through the ART2-P2X7 pathway. | [199] | ||
23 | Humanized mice model GVHD, HaCat cell, Jurkat cells (E6-1 clone), and mouse DCs | Increases CD80, CD 86, STAT-1, and P2X7R expression; IFN-β release; and T cell expansion. Reduces Treg numbers. | [200] | ||
24 | Humanized mice model GVHD | Anti-hP2X7 monoclonal antibodies increase human Treg and human NK cell numbers on Day 21 and reduce clinical and histological GVHD in the liver and lung compared to the control treatment at the disease endpoint. | [201] | ||
25 | LGMDR3 (Limb-girdle muscular dystrophy R3) mice with α-sarcoglycan gene (SGCA) knockout | P2X7 antagonist A438079 improves mouse motor function and decreases serum creatine kinase levels, reduces the percentage of central nuclei, reduces fiber size variability, and reduces the extent of local fibrosis and inflammation significantly. Flow cytometric characterization of muscle inflammatory infiltrates revealed significantly decreased numbers of innate immune cells and increased numbers of Tregs. | [202] | ||
26 | Mouse CD4+CD25+FoxP3+ Tregs | P2X7R activation facilitates NAD+-induced Treg depletion through the ART2-P2X7 pathway. | [199] | ||
27 | BALB/c mice GVHD model, DCs | Activation of P2X7Rs increases CD80, CD 86, STAT-1, and P2X7R expression, IFN-β release, and effector T cell expansion. Reduces Treg numbers. | |||
P2X7R blockade in GVHD improves survival. | [200] | ||||
28 | Mouse, human, murine B cells | Induces shedding of IgE receptor (CD23) and CXCL16. Soluble CD23 sustains growth of B cell precursors, promotes B cell and T cell differentiation, and drives cytokine release from monocytes. CXCL16 is a chemoattractant for lymphocytes. | [203],[204] | ||
29 | Jurkat cells (E6-1 clone) Naïve T cells | TCR stimulation triggers rapid release of ATP and upregulates P2X7 gene expression. Autocrine ATP stimulation through the P2X7R is required for TCR-mediated calcium influx, NFAT activation, and IL-2 production. | [205] | ||
30 | Wild-type and Panx-1 knockout C57BL/6 mice, bone marrow–derived DCs | ATP promotes the rapid migration of DCs through the activity of pannexin 1 channels and P2X7 receptors. | [185] | ||
31 | Mouse and P2X7 knockout mice, CD11c+CD103+ DCs | Mediates rapid infection-induced recruitment of CD11c+CD103+ DC subsets into the epithelial layer of the gut. | [206] | ||
32 | DCs cultured from murine bone marrow precursor cells | Induces autocrine-mediated (pannexin-1 channels) rapid migration of DCs through reorganization of the actin cytoskeleton. | [185] | ||
33 | Human monocytes | Induces MMP-9 and TIMP-1 release. | [207] | ||
34 | Wild-type and P2X7 knockout C57BL/6 mice, M1 macrophages | Induces release of 74 proinflammatory proteins detected by an antibody array and 33 inflammatory proteins detected by LC–MS/MS. | [208] | ||
35 | Wild-type and P2X7 knockout C57BL/6 mice M2 macrophages | Induces release of 21 anti-inflammatory proteins detected by LC–MS/MS. | [208] | ||
36 | CLP-induced sepsis in mice, macrophages | Enhances intracellular bacterial killing. | [209] | ||
37 | Macrophages and P2X7R-transfected HEK-293 cells | Mediates rapid uptake of beads and bacteria in the absence of serum after ATP activation. | |||
38 | Human mast cells | Induces degranulation. | [210] | ||
39 | Wild-type and P2X7 knockout C57BL/6 mice, naïve NKTs | Facilitates NAD+-induced inhibitory signaling through the ART2-P2X7 pathway, resulting in nonfunctional NKTs. | [211] | ||
40 | Wild-type and P2X7 knockout C57BL/6 mice, activated NKTs | Facilitates NAD+-induced stimulatory signaling through the ART2-P2X7 pathway, resulting in functional NKTs with increased IFN-γ and IL-4 release. | [211] | ||
41 | Human peripheral neutrophils | Human CAP18/LL-37 suppresses neutrophil apoptosis through the activation of formyl-peptide receptor-like 1 and P2X7R. | [212] | ||
42 | P2X7R | ATP > 1 mM, prolonged vigorous activation | Macrophages, HeLa cells, 1321N1 astrocytes, and HEK293 cells | Induces Panx-1-mediated large pore formation and interleukin-1 beta release. | [213] |
43 | Human neutrophils and HL-60 cells | Mediates large pore formation and superoxide generation. | [214] | ||
44 | Female C57BL/6 (B6) and BALB/c mice, ART2 knockout BALB/c mice, P2X7 knockout C57BL/6 mice, mature peripheral T cells | High-dose ATP promotes apoptosis, cell death, and CD62L shedding (homing receptor for central T cells) independent of the NAD+-induced ART2-P2X7 pathway. | [215]–[217] | ||
45 | J774 cells and HEK cells expressing the P2X7 receptor | Promotes the formation of pores permeable to very large ions, leading to cytolysis | [151] | ||
46 | P2Y12R | ADP > ATP | Murine model of sepsis, cecal ligation, and puncture (CLP). Cocultures of human platelets and T cells with or without anti-CD3/CD28 | Blockade of the P2Y12 signaling pathway restrains Treg proliferation in vivo and in vitro. | [218],[219] |