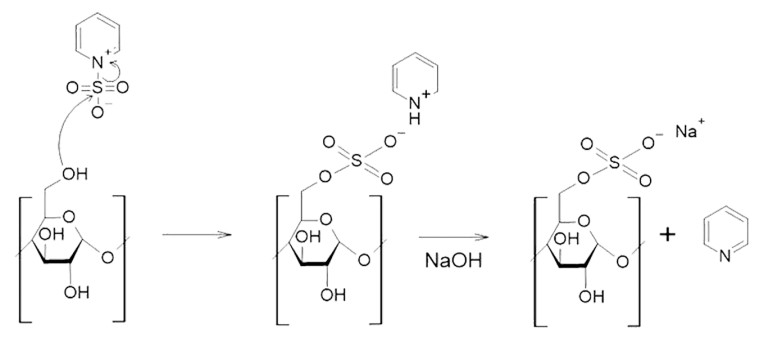
Waterproofing coatings are composite materials made of different layers with complementary functionalities. They may suffer damage that can modify their aesthetic appearance and/or their functionality. In this study, dark stains appearing on a waterproofing coating of a public swimming pool were mapped and characterized at a macroscopic scale through visual observation and by colorimetric analysis, as well as at a microscopic scale with a digital microscope, a confocal laser scanning microscope, and a scanning electron microscope. Five stains were differentiated macroscopically and characterized using colorimetry and principal component analysis. Microscopic observations showed the presence of microorganisms of varied morphology, some filamentous but mostly unicellular. Biofilms consisting of ovoid fluorescent cells with the morphology of Chlorophyta and unicellular cyanobacteria were particularly abundant. The pigmented stains were located at top coat disorders where microbial colonization and biofilm development were observed. The microscopic observations suggested that physical degradation of the surface of the material would have constituted a prerequisite for colonization by pigmented microorganisms which would have led to the development of macroscopically visible pigmented areas. In this case study, the damage remained superficial and did not alter the watertightness of the material so far.
Citation: Clotilde Maestri, Ronan L. Hébert, Patrick Di Martino. Biofilm associated with pigmented areas on a waterproofing coating surface[J]. AIMS Microbiology, 2025, 11(1): 74-86. doi: 10.3934/microbiol.2025005
[1] | Yulia M. Panchenko, Andrey I. Marshakov, Ludmila A. Nikolaeva, Victoria V. Kovtanyuk . Prediction of first-year corrosion losses of copper and aluminum in continental regions. AIMS Materials Science, 2018, 5(4): 624-649. doi: 10.3934/matersci.2018.4.624 |
[2] | Krzysztof Gargul . Ammonia leaching of slag from direct-to-blister copper smelting technology. AIMS Materials Science, 2020, 7(5): 565-580. doi: 10.3934/matersci.2020.5.565 |
[3] | Omar Bataineh, Abdullah F. Al-Dwairi, Zaid Ayoub, Mohammad Al-Omosh . DOE-based experimental investigation and optimization of hardness and corrosion rate for Cu-x%Al2O3 as processed by powder metallurgy. AIMS Materials Science, 2021, 8(3): 416-433. doi: 10.3934/matersci.2021026 |
[4] | Hiroki Nara, Keisuke Morita, Tokihiko Yokoshima, Daikichi Mukoyama, Toshiyuki Momma, Tetsuya Osaka . Electrochemical impedance spectroscopy analysis with a symmetric cell for LiCoO2 cathode degradation correlated with Co dissolution. AIMS Materials Science, 2016, 3(2): 448-459. doi: 10.3934/matersci.2016.2.448 |
[5] | Narayan Poudyal, Kinjal Gandha, Kevin Elkins, J. Ping Liu . Anisotropic SmCo5/FeCo core/shell nanocomposite chips prepared via electroless coating. AIMS Materials Science, 2015, 2(3): 294-302. doi: 10.3934/matersci.2015.3.294 |
[6] | Leydi J. Cardenas F., Josep Ma. Chimenos, Luis C. Moreno A., Elaine C. Paris, Miryam R. Joya . Enhancing Co3O4 nanoparticles: Investigating the impact of nickel doping and high-temperature annealing on NiCo2O4/CoO heterostructures. AIMS Materials Science, 2023, 10(6): 1090-1104. doi: 10.3934/matersci.2023058 |
[7] | Mahmoud A Rabah . Lead, zinc and copper fine powder with controlled size and shape. AIMS Materials Science, 2017, 4(6): 1358-1371. doi: 10.3934/matersci.2017.6.1358 |
[8] | Mundher Al-Shakban, Naktal Al-Dulaimi, Thokozani Xaba, Ahmad Raheel . Rapid facile synthesis of Cu2ZnSnS4 films from melt reactions. AIMS Materials Science, 2020, 7(3): 302-311. doi: 10.3934/matersci.2020.3.302 |
[9] | Abdulkader A. Annaz, Saif S. Irhayyim, Mohanad L. Hamada, Hashim Sh. Hammood . Comparative study of mechanical performance between Al–Graphite and Cu–Graphite self-lubricating composites reinforced by nano-Ag particles. AIMS Materials Science, 2020, 7(5): 534-551. doi: 10.3934/matersci.2020.5.534 |
[10] | Lucangelo Dimesso, Michael Wussler, Thomas Mayer, Eric Mankel, Wolfram Jaegermann . Inorganic alkali lead iodide semiconducting APbI3 (A = Li, Na, K, Cs) and NH4PbI3 films prepared from solution: Structure, morphology, and electronic structure. AIMS Materials Science, 2016, 3(3): 737-755. doi: 10.3934/matersci.2016.3.737 |
Waterproofing coatings are composite materials made of different layers with complementary functionalities. They may suffer damage that can modify their aesthetic appearance and/or their functionality. In this study, dark stains appearing on a waterproofing coating of a public swimming pool were mapped and characterized at a macroscopic scale through visual observation and by colorimetric analysis, as well as at a microscopic scale with a digital microscope, a confocal laser scanning microscope, and a scanning electron microscope. Five stains were differentiated macroscopically and characterized using colorimetry and principal component analysis. Microscopic observations showed the presence of microorganisms of varied morphology, some filamentous but mostly unicellular. Biofilms consisting of ovoid fluorescent cells with the morphology of Chlorophyta and unicellular cyanobacteria were particularly abundant. The pigmented stains were located at top coat disorders where microbial colonization and biofilm development were observed. The microscopic observations suggested that physical degradation of the surface of the material would have constituted a prerequisite for colonization by pigmented microorganisms which would have led to the development of macroscopically visible pigmented areas. In this case study, the damage remained superficial and did not alter the watertightness of the material so far.
Abbreviations: OP: Orange peel waste; AP: Apple pomace; UF: Ultrafiltration
Heavy metals are the most common contaminants found in water. They can cause severe health effects even at low concentrations due to their accumulation in living organisms, and severely interfere within the aquatic ecosystem. Toxic heavy metals can be present in water bodies due to natural causes or human actions, e.g., industrial activity and mining. Thus, effluents produced at the industry level must be treated before releasing wastewater to the natural water bodies [1,2].
There is a wide range of different methods for heavy metal removal [3]. Methods such as coagulation and chemical precipitation are simple and inexpensive but generate large volumes of sludge which needs to be disposed of or processed further. In addition, precipitation requires neutral to basic pH. Thus, it cannot be utilized in every case. Precipitation is also ineffective for low metal concentrations (1–100 mg/L) [4,5]. Ion exchange, in turn, is a fast and effective process and can be carried out as batch or continuous flow process, but fouling due to the organic matter can cause challenges in the process. Also, treating large volumes of water requires large ion exchange columns [4]. Adsorption is a simple method with fast kinetics and can be applied for a wide range of contaminants [3]. Carbon-based adsorbents like activated carbons are widely used for heavy metal removal due to their high surface area and uptake capacity but can be expensive. Also, uptake capacity of activated carbon adsorbents depends on the pore size distribution and functional groups on the surface [3,4].
Lignocellulosic biomass is an inexpensive and abundant raw material, which can be used for heavy metal removal [6] or modified via surface functional groups e.g., by sulfonation to enhance binding efficiency [7]. Sulfonation increases the amount of negatively charged binding sites on the surface of the biomass which improves binding of cationic species such as heavy metal ions from aqueous solutions [8]. However, lignocellulose is poorly soluble in water and common organic solvents. Therefore, sulfonation reactions have been carried out in solvents like dimethyl sulfoxide, pyridine or toluene, which have undesired properties like carcinogenicity [9].
Ionic liquids are considered as green alternatives for organic solvents. They are practically nonvolatile and nonflammable and have good thermal and chemical stability. They can also dissolve well organic and inorganic compounds, such as natural polymers [10]. Dissolving biomass in the reaction medium prior to the modification enhances reaction efficiency and even distribution of the attached groups [8,9]. Sulfonation with SO3-pyridine complex has been studied previously e.g., with starch, cellulose and pectin and it has shown high reactivity but has not been used for more heterogeneous biomass waste materials such as fruit waste [9,11,12,13].
Fruit waste, such as orange peel waste (OP) and apple pomace (AP), is generated in large amounts globally every year. According to food and agricultural organization (FAO) the global production of orange and apple in 2020 was 75 and 86 million tons, respectively [14]. From produced orange approximately 50%–60% is lost as waste, mainly peels [15]. AP generated from apple processing (juicing etc.) is 20%–35% of the fresh apple weight [16]. The produced peels and pomace are mainly discarded as waste, which causes pollution of the environment and is a waste of resources. Fruit wastes contain typically significant amount of holocellulose, which is rich in hydroxyl groups [17]. Hence, fruit waste is a suitable raw material for sulfonation where SO3-groups are attached to those hydroxyl groups. During work-up the sulfated product can be precipitated as sodium salt with cold ethanol [9,13,18]. Sulfonation reaction with SO3-pyridine complex and formation of sodium salt is described in Figure 1.
In this study, OP and AP were sulfonated with SO3-pyridine complex in ionic liquid, 1-allyl-3-methylimidazolium chloride [AMIM]Cl. The occurrence of the sulfonation reaction was verified with 1H NMR analysis by monitoring a signal at 4.94 ppm. The intensity of the signal increased with increasing amount of SO3-pyridine complex and reaction temperature. Therefore, the product which spectrum had the highest signal at 4.94 ppm was considered as the most promising material and was selected for the cobalt and copper removal experiments performed with sulfonated fruit waste enhanced ultrafiltration. Based on the ICP-OES analyses, both studied materials bound cobalt and copper well, both from a single metal solution and from a binary metal solution. To our knowledge, sulfonation of OP or AP or the use of similarly prepared sulfonation products has not been studied before.
OP was obtained from Finnish grocery store (Prisma, Oulu, Finland) and AP from local juicing facility (Marjarannikko, Tupos, Finland). Materials were stored in a freezer. Before use, flavedo, i.e., the orange surface layer of the peel, was removed and only albedo was used in the study. OP and AP were dried until constant weight in the oven at 45 ℃ and then ground to a fine powder with a coffee grinder. The moisture content of OP (79.4%) and AP (82.3%) was determined by calculating mass loss during drying. Dried and ground biomasses were extracted by Soxhlet extraction for 4 h with acetone (anhydrous, BDH chemicals). After the extraction, powders were dried again at 45 ℃ until constant weight. Dried powders were stored in a desiccator. The mass% of extractives of OP (10.5%) and AP (15.6%) were determined gravimetrically by calculating mass loss due to extraction.
An estimate of the composition of dry OP and AP was obtained using known methods [19]. The ash content determination was made for unextracted OP and AP but for other determinations extracted biomass was used. All analyses were performed in duplicate.
Other reagents used in the study were allyl chloride (99%, Sigma Aldrich), 1-methylimidazole (≥99%, Sigma Aldrich), ethyl acetate (≥99.7%, Honeywell), DMF (99.8%, Labscan), SO3-pyridine complex (98%, Sigma Aldrich), ethanol (96%, BDH chemicals), NaOH (>95%, FF-Chemicals), NaCl (99.5%, Merck), cobalt chloride hexahydrate (≥98%, Riedel de Haën), copper sulphate pentahydrate (≥99%, Merck) and anhydrous sodium sulphate (≥99%, Sigma Aldrich). Elix water was used for preparation of aqueous solutions. Unless otherwise mentioned, all chemicals were used as received.
[AMIM]Cl was prepared using a previously described method [20] from allyl chloride and 1-methylimidazole which was distilled prior to use. The molar ratio of allyl chloride and 1-methylimidazole was 1:1.3. The reaction was carried out in a round bottom flask under argon with continuous stirring. Allyl chloride was added slowly to cooled 1-methylimidazole while keeping temperature under 10 ℃ using ice/salt bath. After the addition, the reaction mixture was allowed to warm to room temperature prior to heating to 55 ℃ in an oil bath. The reaction was allowed to proceed for 12 hours while keeping the temperature constant. After the reaction, the crude [AMIM]Cl was purified by extracting it five times with ethyl acetate. Traces of water and ethyl acetate were removed by evaporation in a high vacuum. The dark brown colored and highly viscous [AMIM]Cl was stored under argon gas in desiccator.
Sulfonation was based on a method previously described by Kärkkäinen et al. [9]. OP (0.125 g) and [AMIM]Cl (2.5 g) were mixed in a microwave reactor tube (size 2–5 mL) equipped with a magnetic stirring bar. The mixture was heated in a microwave reactor (Biotage initiator) at 80 ℃ for 20 min. After the pretreatment 1 mL of DMF was added to the mixture to reduce the viscosity, and the mixture was transferred to a round bottom flask (25 mL) equipped with a magnetic stirring bar. The sulfonation reaction was started by mixing the SO3-pyridine complex to the reaction mixture. The amount of the complex was such that the molar ratio of SO3-pyridine complex to the anhydroglucose unit (AGU) was 1.5:1, 3.35:1 or 5:1. The reaction mixture was heated in an oil bath at 30, 50 or 70 ℃ for 60, 90 or 120 min. After the reaction, the mixture was allowed to cool to room temperature and 8 mL of water was added rapidly while stirring. NaOH (1 M) was added with molar ratio of 1.6:1 to SO3-pyridine complex. The mixture was stirred for 5 min at room temperature. The crude product was precipitated by adding 50 mL of cold ethanol and allowing to set for 1 h. Light colored precipitate was filtrated with nylon membrane (Whatman 0.45 µm, 47 mm). The isolated crude product was purified from organic impurities by dissolving it in 15 mL of 2% NaCl and precipitating again with 50 mL of cold ethanol. The mixture was allowed to set for 30 min and filtrated again. Dissolving to NaCl, precipitation with cold ethanol, settling and filtration were repeated, and the product was dried at 45 ℃ until constant weight.
Sulfonated AP was prepared similarly at selected reaction conditions (reaction time 60 min, reaction temperature 70 ℃, molar ratio of SO3-pyridine complex to AGU 5:1).
The prepared sulfonated OP and AP were treated with ultrafiltration (Millipore Amican 8400) to remove the Na2SO4 salt. 1 g of OP or AP was mixed with 300 mL of water in an ultrafiltration cell, and the mixture was filtrated through regenerated cellulose membrane (Millipore Ultracel with 3 kDa cut-off weight). The pressure (3 kPa) was generated using nitrogen gas and kept constant during the filtration. Filtration was stopped when 200 mL was filtrated. Washing was carried out by adding 100 mL of water into the cell and filtration was continued until 100 mL was filtrated. The washing step was repeated five times. After the ultrafiltration, the remaining 100 mL was transferred from the cell to the round bottom flask and the filtration system was rinsed with 50 mL of water. Rinsing water was combined with the remaining 100 mL. Excess water was evaporated until the total volume was 10–30 mL. The product was precipitated with 5-fold amount of cold ethanol. The precipitated product was isolated by vacuum filtration using nylon membrane as described above and dried at 45 ℃ until constant weight.
Decrease of salt in ultrafiltration cell was monitored by collecting samples during the ultrafiltration and analyzing them with ICP-OES.
Cobalt and copper solutions (8 and 6 mg/L, respectively) used in metal removal experiments were prepared from stock solutions (80 mg/L). Unmodified OP and AP were used as reference materials. In 100 mL of 8 mg/L cobalt solution, 25, 12.5 or 6 mg of modified or unmodified biomass was weighted. In 6 mg/L Cu-solution 6 mg of biomass was weighted. Experiments were carried out in pH 4 and 5. pH was adjusted with 0.1 M HCl. Contact time was 35 min due to the experimental setup even though the adsorption itself has been studied to be rapid [21]. Cobalt solution without any adsorbent was also filtrated to evaluate the effect of the membrane itself on the cobalt concentration. Samples were collected by ultrafiltrating 20 mL from the mixture. The membrane was washed between each experiment by rinsing with water and filtrating 20 mL of water through the membrane. All metal removal experiments were done in duplicate and results are reported as an average of those parallel experiments.
[AMIM]Cl, sulfonated OP and unmodified and pretreated OP were characterized with 1H NMR (Bruker Avance 400) in room temperature. [AMIM]Cl was dissolved in CDCl3 and peel materials in D2O. Spectra were processed with ACDLabs Spectrus Processor.
Thermo Elemental Flash 2000 CHNS/O Analyzer was used for the determination of the degree of substitution (DS). DS was calculated using Eq 1 [22]:
DS=(162.1×S%)/(3207−102.2×S%) | (1) |
where S% is sulfur mass percentage of sulfonated and purified OP determined with elemental analysis. Standard error of the mean (SEM) was calculated using Eq 2 [23]:
SEM=√((∑(xi−¯x)2)/(n(n−1))) | (2) |
where xi is DS value based on single measurement of sulfur content, x is the mean value for DS based on three replicate measurements of sulfur content and n is number of measurements.
Samples collected from ultrafiltration and metal removal experiments were analyzed with ICP-OES (Agilent 5110) equipped with SPS 4 autosampler, concentric nebulizer and cyclonic spray chamber. Each sample was measured in triplicate at three different wavelengths. Selected wavelengths were 568.821,588.995 and 589.592 nm; 238.892,228.615 and 230.786 nm and 327.395,324.745 and 213.598 nm for sodium, cobalt and copper, respectively. Automatic background correction was used, and argon gas was used in all gas flows. Measuring parameters were: vertical dual view, measuring time 5 s, plasma power 1.2 kW, stabilization time 25/20 s, pump speed 12 rpm, plasma gas flow rate 12 L/min, auxiliary gas flow rate 1 L/min, viewing height in radial measurements 8 mm.
OP and AP were subjected to compositional analysis before use to get an estimate of their composition. The results of the analyses are presented in Table 1. The presented lignin amounts contain both the soluble and insoluble lignin. The determined polysaccharide (holocellulose + pectin) amounts (78% for OP, 56% for AP) were used as an approximation for the amount of anhydroglucose units of studied biomasses.
OP, wt% | AP, wt% | |
Extractives | 4 | 28 |
Ash | 2 | 2 |
Lignin, total | 16 | 14 |
Holocellulose | 27 | 21 |
α-cellulose | 11 | 12 |
Hemicellulosea | 16 | 9 |
Other (mainly pectin)b | 51 | 35 |
a calculated by subtracting α-cellulose from holocellulose; b calculated by subtracting extractives, ash, lignin and holocellulose from 100. |
It was noted during the sulfonation reactions and the isolation of the product that reaction temperature and the amount of SO3-pyridine complex had an evident impact on the reaction itself and on the obtained solid products. The viscosity of reaction mixture reduced with increasing reaction temperature and higher temperatures resulted in more efficient stirring. The homogeneity of the precipitated product increased with increasing SO3-pyridine complex amount. With 5:1 molar ratio of the complex to anhydroglucose unit (AGU) the product was powder like and had a small particle size, while the molar ratio of 1.5:1 (complex:AGU) produced also larger flake like structures. With molar ratio of 3.25:1, the obtained product contained fine powder mixed with fiber like particles. When the product was dissolved to NaCl it was noted that the solubility increased with increasing amount of SO3-pyridine complex and reaction temperature, which may indicate that the reactions performed in low temperatures produced products with uneven sulfate group distribution due to the inefficient stirring. In Richter's and Klemm's study cellulose sulfates were prepared homogeneously with several sulfating agents and all the products were completely water soluble [21,24]. Also, in the Qin et al. and Gericke et al., studies it was noted that cellulose sulfates with DS > 0.3–0.4 with even distribution of sulfate groups were soluble in water [11,12].
According to ICP-OES measurements approximately 40%–50% of the masses of the prepared sulfonated fruit wastes was Na2SO4 salt. The amount of salt was cut in half during the first three washes with ultrafiltration. During the last two washes the amount of salt was reduced by two-thirds. In total, sodium concentration was reduced from 1000 mg/L to under 10 mg/L by washing. According to ICP-OES results the amount of salt in the product was so low after purification that it was not considered in biomass dosage in heavy metal removal experiments.
All sulfonated OP products as well as unmodified and pretreated OP were analyzed with 1H NMR. 1H NMR was used to confirm that sulfonation reaction had occurred and to evaluate the effect of the reaction conditions on the structure and DS of polysaccharides. Unmodified OP was poorly soluble in D2O producing a poor-quality NMR spectrum (Supplementary). Therefore, the spectrum of pretreated OP (Figure 2a) was used as the reference spectrum for the spectra of sulfonated OPs. The pretreatment of OP in ionic liquid broke the structure of OP slightly resulting in better solubility of pretreated OP than unmodified OP.
Peak broadening and overlapping were observed due to the heterogeneous nature of the raw material, which caused challenges for the interpretation of the spectra. According to the literature the signal of nanocellulose H1 is observed at 4.4 ppm and the signal of anomeric H1 at 4.4–5.8 ppm while the signals of polysaccharides' H2 and H6 can usually be observed at 3.2–4.5 ppm. According to the literature β-anomeric H1 is observed more up field than ɑ-anomeric H1 [25,26].
Signals of the sulfonated OP were broad and not clearly separated at 4–4.7 ppm (Figure 2b) which made interpretation of spectra challenging. However, a new signal was observed at 4.94 ppm which was concluded to prove that change in chemical environment of certain hydrogen had occurred due to the sulfonation (Figure 2b). The intensity of that signal increased with the increasing amount of SO3-pyridine complex (Figure 2c). On the other hand, increasing the reaction time did not increase the intensity of the signal at 4.94 ppm. The studied signal was most likely caused by β-anomeric H1 of substituted product. Thus, it was concluded that stronger signal with corresponding shift indicated higher DS. Based on that conclusion the product from the reaction with following conditions: reaction time 60 min, reaction temperature 70 ℃ and the molar ratio of the SO3-pyridine complex to AGU 5:1 was selected for the metal removal experiments. The product of the selected reaction had the most significant increase in signal intensity at 4.94 ppm. Increasing the amount of sulfating reagent to AGU has been reported by Kärkkäinen et al. and Gericke et al. to increase the DS of starch and cellulose, respectively [9,11].
Reference spectrum for sulfonated OP could not be found in the literature. However, signals corresponding to cellulose and cellulose sulfates were described by Kowsaka et al. [27]. According to their data, the substitution had occurred to the OH group at C2 position [25] even though the OH group at the primary C6 has been reported to be the most reactive site at least in starch [9]. C2 has been reported to be the second most reactive site in starch [9]. According to Kowsaka et al., the signal of H1 of C2 and C6 monosubstituted cellulose sulfate should be observed at 4.93 and 4.53 ppm, respectively. H1 of 2, 6-disubstitued cellulose sulfate was also proposed to be observed at 4.93 ppm. Unsubstituted cellulose H1 was observed at 4.57 ppm [27]. Also, sulfonation agent has been reported to influence the substitution site. When trimethylsilyl cellulose was sulfonated with SO3-pyridine complex, substitution occurred at C2 and C6 positions without clear preference on either site while SO3-DMF preferred C6 position [24]. It must be acknowledged that in addition to cellulose, OP also contains hemicellulose and pectin which may have effect on the reactivity of different sites, and which can also undergo sulfonation making the interpretation of the NMR spectra more complex. Based on the 1H NMR data, it was concluded that the sulfonation reaction had been successful when the reaction conditions were sufficient, i.e., the molar ratio of the SO3-pyridine complex to AGU 3.25–5:1, temperature 50–70 ℃ and reaction time 60–90 min (1H NMR spectra shown in Supplementary).
The product that was used in metal removal experiments was selected based on the 1H NMR spectra (60 min, 70 ℃, 5:1 for molar ratio of SO3-pyridine complex to AGU). The DS of the selected product was determined afterwards with elemental analysis due to the complexity of the obtained NMR spectrum. According to elemental analysis, the product, which was purified from sulfate salt by ultrafiltration prior analysis, contained 0.23%, 48.18%, 20.51%, 3.03% and 10.68% of nitrogen, oxygen, carbon, hydrogen and sulfur, respectively. The calculated DS was 0.82 (SEM ± 0.087). With the used method, i.e., ionic liquid as the reaction medium and SO3-pyridine complex as the reagent, similar DS values have been reported for barley starch and cellulose [9,11].
Metal removal was first studied with cobalt. The initial cobalt concentration was 8 mg/L, and the amount of sulfonated OP was varied (6, 12.5 or 25 mg). The pH of the metal solution was adjusted to 4 or 5 due to the precipitation of metal in neutral to alkaline pH. In pH 4 a slight but not significant decrease in binding of cobalt was observed compared to pH 5 due to the higher H+ content in more acidic media. Thus, binding was not particularly sensitive to pH change from 5 to 4. This was also observed in the study of Dong et al. [21] They modeled the effect of pH on metal removal efficiency and found that in pH below 4 the efficiency decreased significantly due to increasing H+ content in solutions [21].
Unmodified OP was used as a reference material to confirm that binding improved with sulfonation. In addition, cobalt solution was ultrafiltrated without any biomass to study the effect of the ultrafiltration itself. According to ICP-OES measurements, the effect of ultrafiltration or unmodified OP in binding was minor compared to the sulfonated OP. Ultrafiltration alone removed 0–11% and unmodified OP with ultrafiltration removed 2%–15% of cobalt. Sulfonated OP in turn bound over 89% of cobalt in pH 5 and 4 with 12.5 and 25 mg/100 mL dosage. Since lower dosage (12.5 mg) did not decrease the removal-% significantly, it was concluded that using dosage < 25 mg makes the process more cost-effective. When sulfonated OP dosage was reduced to 6 mg/100 mL, over 60% of cobalt was still bound. The results of the cobalt removal experiments are presented in Table 2.
Binding method | pH | mpeel (mg) | Co removal-%a |
Ultrafiltration | 5 | 0 | 11 |
4 | 0 | 0 | |
Unmodified OP + UF | 5 | 6 | 6 |
5 | 12.5 | 2 | |
5 | 25 | 15 | |
Sulfonated OP + UF | 5 | 6 | 63 |
5 | 12.5 | 91 | |
5 | 25 | 98 | |
Sulfonated OP + UF | 4 | 6 | 56 |
4 | 12.5 | 89 | |
4 | 25 | 95 | |
aSD ± 4%. |
Because removal of cobalt was effective with relatively low biomass dosage, the removal of another metal, i.e., copper, was also studied. The initial copper solution concentrations of 6 and 8 mg/L were used to evaluate the effect of the initial metal concentration on the removal. The studied sulfonated OP dosages were 12.5 and 6 mg/100 mL, since it was noted earlier that < 25 mg is enough for efficient metal removal. Copper removal followed a similar trend than cobalt removal. The change of pH from 5 to 4 did not have a significant effect on binding. It was observed that copper removal-% was even higher compared to cobalt removal-% despite it was noted in Dong et al., study that copper removal was poorest compared to Fe and Pb from single metal solution as well as from ternary solution [21]. In addition, Lappalainen et. al., noted that copper bound to sulfated starch was unstable compared to other studied metals (Fe, Zn, Ni) [28]. The difference between copper and cobalt binding was more notable with 6 mg than 12.5 mg dosage. With 6 mg dosage of sulfonated OP, 27% more copper was bound compared to cobalt and, with 12.5 mg dosage, the difference was 7%. It was noted that binding was slightly more efficient with higher initial metal concentration. It has been studied that higher metal concentration increases the interactions between metal ions and adsorbent increasing the adsorption capacity. However, Sirviö et al. found that the highest metal removal was achieved with low concentrations [29]. Similar behavior was observed also in the study of Dong et al., where binding of metals with sulfonated cellulose decreased with increasing metal concentration [21]. The decrease of heavy metal removal with increasing metal concentration may be due to the lack of free adsorption sites. In Dong et al., study DS of cellulose sulfate was 0.56 which is lower compared to 0.82 determined in this study for sulfonated OP. Adsorbent dose was considerably higher (50 mg) in most experiments performed by Dong et al. when considering the volume and the metal content of the solution to be treated (volume 25 mL, metal concentration 25–200 mg/L) [21]. Results of copper binding are presented in Table 3.
Binding method | pH | mpeel (mg) | Cuinitial (mg/L) | Cu removal-%a |
Sulfonated OP + UF | 5 | 12.5 | 8 | 98 |
5 | 6 | 6 | 74 | |
5 | 6 | 8 | 89 | |
4 | 12.5 | 8 | 97 | |
4 | 6 | 6 | 64 | |
Sulfonated AP + UF | 5 | 12.5 | 8 | 97 |
5 | 6 | 6 | 89 | |
5 | 6 | 8 | 87 | |
4 | 12.5 | 8 | 98 | |
4 | 6 | 6 | 85 | |
aSD ±6%. |
Due to the effective binding of both cobalt and copper with sulfonated OP, a preliminary binding experiment was performed with another fruit waste, AP. AP was sulfonated in the same conditions which were previously found best for OP (molar ratio of SO3-pyridine complex to AGU 1.5:1, reaction time 60 min, reaction temperature 70 ℃). Sulfonated AP was tested for copper removal first. No notable difference was observed between sulfonated OP and AP when initial metal concentration was 8 mg/L. However, sulfonated AP bound 15% and 21% more copper than sulfonated OP in pH 5 and 4, respectively, when initial metal concentration was 6 mg/L (Table 3).
The results achieved with metal removal from single metal solution with sulfonated fruit wastes were promising, and therefore the binding was also studied from binary solution. The initial concentration of both metals was 8 mg/L. Since the total concentration of metals doubled compared to single metal solutions, the dose of OP and AP was increased to 12.5 mg. It was noted in single metal experiments that < 25 mg dosage was clearly enough. On the other hand, 6 mg proved to be a slight underestimate for effective metal removal in part of the experiments (Tables 2 and 3). From binary metal solution copper bound more effectively than cobalt (Table 4). Also, sulfonated OP seemed to bind both metals somewhat better in both pH conditions than sulfonated AP. Despite the more efficient removal of copper, the removal-% of both metals was 70%–82% in pH 4 and 77%–93% in pH 5.
Binding method | pH | Co removal-%a | Cu removal-%a |
Sulfonated OP + UF | 5 | 83 | 93 |
4 | 73 | 82 | |
Sulfonated AP + UF | 5 | 77 | 82 |
4 | 70 | 73 | |
aSD ±4%. |
Sulfonation with SO3-pyridine complex in ionic liquid was observed to be an effective way to attach SO3-groups on the surface of powdered OP. Based on the 1H NMR analysis the best reaction conditions for the sulfonation reaction were 60 min for reaction temperature, 70 ℃ for reaction time and 5:1 for SO3-pyridine complex to AGU. The DS of the product prepared in those conditions was 0.82.
The prepared sulfonated OP with DS of 0.82, combined with ultrafiltration, was effective in removal of cobalt and copper from single metal as well as from binary metal solutions with a relatively small dosage. From the single metal solution 91% of cobalt and 98% of copper was removed when pH of the solution was 5, the initial metal concentration 8 mg/L and the dosage of the adsorbent 12.5 mg/100 mL. From binary metal solution (pH 5, 8 mg/L/metal) the removal-% were 93% and 83% for copper and cobalt, respectively, when the adsorbent dosage was 12.5 mg/100 mL.
The successful experiments performed with OP were repeated with AP. Based on the preliminary results the sulfonated AP proved to be almost as effective in cobalt and copper removal as sulfonated OP. From binary metal solution (pH 5, 8 mg/L/metal) the removal-% were 82% and 77% for copper and cobalt, respectively, when the adsorbent dosage was 12.5 mg/100 mL.
The analysis of the prepared sulfonated fruit waste proved to be challenging due to the heterogeneity of the material. Therefore, an effective and accurate method for DS determination of sulfonated fruit wastes with NMR should be studied further. Further characterization of sulfonated fruit waste is needed also for evaluating the stability of the material in different conditions (thermal, chemical). In addition, regeneration of sulfonated fruit waste should be included in future studies.
The authors declare they have not used Artificial Intelligence (AI) tools in the creation of this article.
The study was supported by K.H. Renlund foundation, Kone Foundation (Grant number 201903073), Maj and Tor Nessling Foundation (Grant number 201800070), and Oulun läänin talousseuran maataloussäätiö.
The authors declare no conflict of interest.
[1] | Khanna S, Sah R, Hooda S, et al. (2024) Water proofing system in concrete structures. Int J Res Civ Eng Technol 5: 32-34. https://doi.org/10.48175/ijarsct-17845 |
[2] | Kavitha R, Ram Vivekananthan M, Dhanagopal K, et al. (2023) An overview of water proofing system in concrete structures. Mater Today Proc . https://doi.org/10.1016/j.matpr.2023.03.515 |
[3] | Saxena PK, Raut KG, Srinivasan SR, et al. (1991) Polyurethane waterproofing coating for building applications. Constr Build Mater 5: 208-210. https://doi.org/10.1016/0950-0618(91)90052-M |
[4] | Maestri C, Plancher L, Duthoit A, et al. (2023) Fungal Biodegradation of Polyurethanes. J Fungi (Basel) 9: 760. http://doi.org/10.3390/jof9070760 |
[5] | Hébert R, Beouch L, Fichet O, et al. (2012) Cracks and stains on façade-cladding made of carbonate rock thin panels. Struct Survey 30: 130-144. https://doi.org/10.1108/02630801211228734 |
[6] | Newby PT, Mansfield TA, Hamilton RS (1991) Sources and economic implications of building soiling in urban areas. Sci Total Environ 100: 347-365. https://doi.org/10.1016/0048-9697(91)90385-R |
[7] | Brimblecombe P, Grossi CM (2005) Aesthetic thresholds and blackening of stone buildings. Sci Total Environ 349: 175-189. https://doi.org/10.1016/j.scitotenv.2005.01.009 |
[8] | Cutler NA, Viles HA, Ahmad S, et al. (2013) Algal ‘greening’ and the conservation of stone heritage structures. Sci Total Environ 442: 152-164. https://doi.org/10.1016/j.scitotenv.2012.10.050 |
[9] | Di Martino P (2016) What about biofilms on the surface of stone monuments?. Open Conf Proc J 7: 14-28. https://doi.org/10.2174/2210289201607020014 |
[10] | Liu X, Koestler RJ, Warscheid T, et al. (2020) Microbial deterioration and sustainable conservation of stone monuments and buildings. Nat Sustain 3: 991-1004. https://doi.org/10.1038/s41893-020-00602-5 |
[11] | Hernández Mariné M, Clavero E, Roldán M (2004) Microscopy methods applied to research on cyanobacteria. Limnetica 23: 179-186. https://doi.org/10.23818/limn.23.16 |
[12] | Peisker H, Michels J, Gorb S (2013) Evidence for a material gradient in the adhesive tarsal setae of the ladybird beetle Coccinella septempunctata. Nat Commun 4: 1661. https://doi.org/10.1038/ncomms2576 |
[13] | Hobisch MA, Bossu J, Mandlez D, et al. (2019) Localization of cellulosic fines in paper via fluorescent labeling. Cellulose 26: 6933-6942. https://doi.org/10.1007/s10570-019-02556-0 |
[14] | Lewin RA (2006) Black algae. J Appl Phycol 18: 699-702. https://doi.org/10.1007/s10811-005-9018-2 |
[15] | Miller AZ, Laiz L, Gonzalez JM, et al. (2008) Reproducing stone monument photosynthetic-based colonization under laboratory conditions. Sci Total Environ 405: 278-285. https://doi.org/10.1016/j.scitotenv.2008.06.066 |
[16] | Macedo MF, Miller AZ, Dionísio A, et al. (2009) Biodiversity of cyanobacteria and green algae on monuments in the Mediterranean Basin: an overview. Microbiology (Reading) 155: 3476-3490. https://doi.org/10.1099/mic.0.032508-0 |
[17] | Li S, Fanesi A, Martin T, et al. (2024) Physiological transition of Chlorella vulgaris from planktonic to immobilized conditions. Algal Res 77: 103354. https://doi.org/10.1016/j.algal.2023.103354 |
[18] | Garcia-Pichel F, Sherry ND, Castenholtz RW (1992) Evidence for an ultraviolet sunscreen role of the extracellular pigment scytonemin in the terrestrial cyanobacterium Chlorogloeopsis sp. Photochem Photobiol 56: 17-23. https://doi.org/10.1111/j.1751-1097.1992.tb09596.x |
[19] | Garcia-Pichel F, Castenholz RW (1993) Occurrence of UV-absorbing mycosporine-like compounds among cyanobacterial isolates, and an estimate of their screening capacity. App Env Microbiol 59: 163-169. https://doi.org/10.1128/aem.59.1.163-169.1993 |
[20] | Proteau PJ, Gerwick WH, Garcia-Pichel F, et al. (1993) The structure of scytonemin, an ultraviolet sunscreen pigment from the sheaths of cyanobacteria. Experientia 49: 825-829. https://doi.org/10.1007/BF01923559 |
[21] | Gaylarde CC, Gaylarde PM (2005) A comparative study of the major microbial biomass of biofilms on exteriors of buildings in Europe and Latin America. Int Biodeterior Biodegrad 55: 131-139. https://doi.org/10.1016/j.ibiod.2004.10.001 |
[22] | Bruno L, Valle V (2017) Effect of white and monochromatic lights on cyanobacteria and biofilms from Roman Catacombs. Int Biodeterior Biodegrad 123: 286-295. https://doi.org/10.1016/j.ibiod.2017.07.013 |
[23] | Komar M, Nowicka-Krawczyk P, Ruman T, et al. (2022) Metabolomic analysis of photosynthetic biofilms on building façades in temperate climate zones. Int Biodeterior Biodegrad 169: 105374. https://doi.org/10.1016/j.ibiod.2022.105374 |
[24] | Nowicka-Krawczyk P, Komar M, Gutarowska B (2022) Towards understanding the link between the deterioration of building materials and the nature of aerophytic green algae. Sci Total Environ 802: 149856. https://doi.org/10.1016/j.scitotenv.2021.149856 |
[25] | Viles HA, Taylor MP, Yates TJS, et al. (2002) Soiling and decay of N.M.E.P. limestone tablets. Sci Total Environ 292: 215-229. https://doi.org/10.1016/S0048-9697(01)01124-X |
[26] | Viles HA, Gorbushina AA (2003) Soiling and microbial colonisation on urban roadside limestone: a three-year study in Oxford, England. Build Environ 38: 1217-1224. https://doi.org/10.1016/S0360-1323(03)00078-7 |
[27] | Rajkowska K, Otlewska A, Kozirog A, et al. (2014) Assessment of biological colonization of historic buildings in the former Auschwitz II-Birkenau concentration camp. Ann Microbiol 64: 799-808. https://doi.org/10.1007/s13213-013-0716-8 |
[28] | Komar M, Nowicka-Krawczyk P, Ruman T, et al. (2023) Biodeterioration potential of algae on building materials-Model study. Int Biodeterior Biodegrad 180: 105593. https://doi.org/10.1016/j.ibiod.2023.105593 |
[29] | Saiz-Jimenez C (1999) Biogeochemistry of weathering processes in Monuments. Geomicrobiol J 16: 27-37. https://doi.org/10.1080/014904599270721 |
[30] | Błaszczyński T, Łowińska-Kluge A (2007) Experimental investigation and assessment of damage in the case of swimming-pool repairs. Archiv Civ Mech Eng 7: 5-20. https://doi.org/10.1016/S1644-9665(12)60001-6 |
[31] | Maj M, Ubysz A (2022) The reasons for the loss of polyurea coatings adhesion to the concrete substrate in chemically aggressive water tanks. Eng Fail Anal 142: 106774. https://doi.org/10.1016/j.engfailanal.2022.106774 |
[32] | Almusallam AA, Khan FM, Dulaijan SU, et al. (2003) Effectiveness of surface coatings in improving concrete durability. Cem Concr Compos 25: 473-481. https://doi.org/10.1016/S0958-9465(02)00087-2 |
[33] | Tsukagoshi M, Miyauchi H, Tanaka K (2012) Protective performance of polyurethane waterproofing membrane against carbonation in cracked areas of mortar substrate. Constr Build Mater 36: 895-905. https://doi.org/10.1016/j.conbuildmat.2012.06.072 |
[34] | Somarathna HMCC, Raman SN, Mohotti D, et al. (2018) The use of polyurethane for structural and infrastructural engineering applications: A state-of-the-art review. Constr Build Mater 190: 995-1014. https://doi.org/10.1016/j.conbuildmat.2018.09.166 |
[35] | Khatua S, Hsieh YL (1997) Chlorine degradation of polyether-based polyurethane. J Polym Sci A: Polym Chem 35: 3263-3273. https://doi.org/10.1002/(SICI)1099-0518(19971115)35:15%3C3263::AID-POLA20%3E3.0.CO;2-8 |
OP, wt% | AP, wt% | |
Extractives | 4 | 28 |
Ash | 2 | 2 |
Lignin, total | 16 | 14 |
Holocellulose | 27 | 21 |
α-cellulose | 11 | 12 |
Hemicellulosea | 16 | 9 |
Other (mainly pectin)b | 51 | 35 |
a calculated by subtracting α-cellulose from holocellulose; b calculated by subtracting extractives, ash, lignin and holocellulose from 100. |
Binding method | pH | mpeel (mg) | Co removal-%a |
Ultrafiltration | 5 | 0 | 11 |
4 | 0 | 0 | |
Unmodified OP + UF | 5 | 6 | 6 |
5 | 12.5 | 2 | |
5 | 25 | 15 | |
Sulfonated OP + UF | 5 | 6 | 63 |
5 | 12.5 | 91 | |
5 | 25 | 98 | |
Sulfonated OP + UF | 4 | 6 | 56 |
4 | 12.5 | 89 | |
4 | 25 | 95 | |
aSD ± 4%. |
Binding method | pH | mpeel (mg) | Cuinitial (mg/L) | Cu removal-%a |
Sulfonated OP + UF | 5 | 12.5 | 8 | 98 |
5 | 6 | 6 | 74 | |
5 | 6 | 8 | 89 | |
4 | 12.5 | 8 | 97 | |
4 | 6 | 6 | 64 | |
Sulfonated AP + UF | 5 | 12.5 | 8 | 97 |
5 | 6 | 6 | 89 | |
5 | 6 | 8 | 87 | |
4 | 12.5 | 8 | 98 | |
4 | 6 | 6 | 85 | |
aSD ±6%. |
Binding method | pH | Co removal-%a | Cu removal-%a |
Sulfonated OP + UF | 5 | 83 | 93 |
4 | 73 | 82 | |
Sulfonated AP + UF | 5 | 77 | 82 |
4 | 70 | 73 | |
aSD ±4%. |
OP, wt% | AP, wt% | |
Extractives | 4 | 28 |
Ash | 2 | 2 |
Lignin, total | 16 | 14 |
Holocellulose | 27 | 21 |
α-cellulose | 11 | 12 |
Hemicellulosea | 16 | 9 |
Other (mainly pectin)b | 51 | 35 |
a calculated by subtracting α-cellulose from holocellulose; b calculated by subtracting extractives, ash, lignin and holocellulose from 100. |
Binding method | pH | mpeel (mg) | Co removal-%a |
Ultrafiltration | 5 | 0 | 11 |
4 | 0 | 0 | |
Unmodified OP + UF | 5 | 6 | 6 |
5 | 12.5 | 2 | |
5 | 25 | 15 | |
Sulfonated OP + UF | 5 | 6 | 63 |
5 | 12.5 | 91 | |
5 | 25 | 98 | |
Sulfonated OP + UF | 4 | 6 | 56 |
4 | 12.5 | 89 | |
4 | 25 | 95 | |
aSD ± 4%. |
Binding method | pH | mpeel (mg) | Cuinitial (mg/L) | Cu removal-%a |
Sulfonated OP + UF | 5 | 12.5 | 8 | 98 |
5 | 6 | 6 | 74 | |
5 | 6 | 8 | 89 | |
4 | 12.5 | 8 | 97 | |
4 | 6 | 6 | 64 | |
Sulfonated AP + UF | 5 | 12.5 | 8 | 97 |
5 | 6 | 6 | 89 | |
5 | 6 | 8 | 87 | |
4 | 12.5 | 8 | 98 | |
4 | 6 | 6 | 85 | |
aSD ±6%. |
Binding method | pH | Co removal-%a | Cu removal-%a |
Sulfonated OP + UF | 5 | 83 | 93 |
4 | 73 | 82 | |
Sulfonated AP + UF | 5 | 77 | 82 |
4 | 70 | 73 | |
aSD ±4%. |