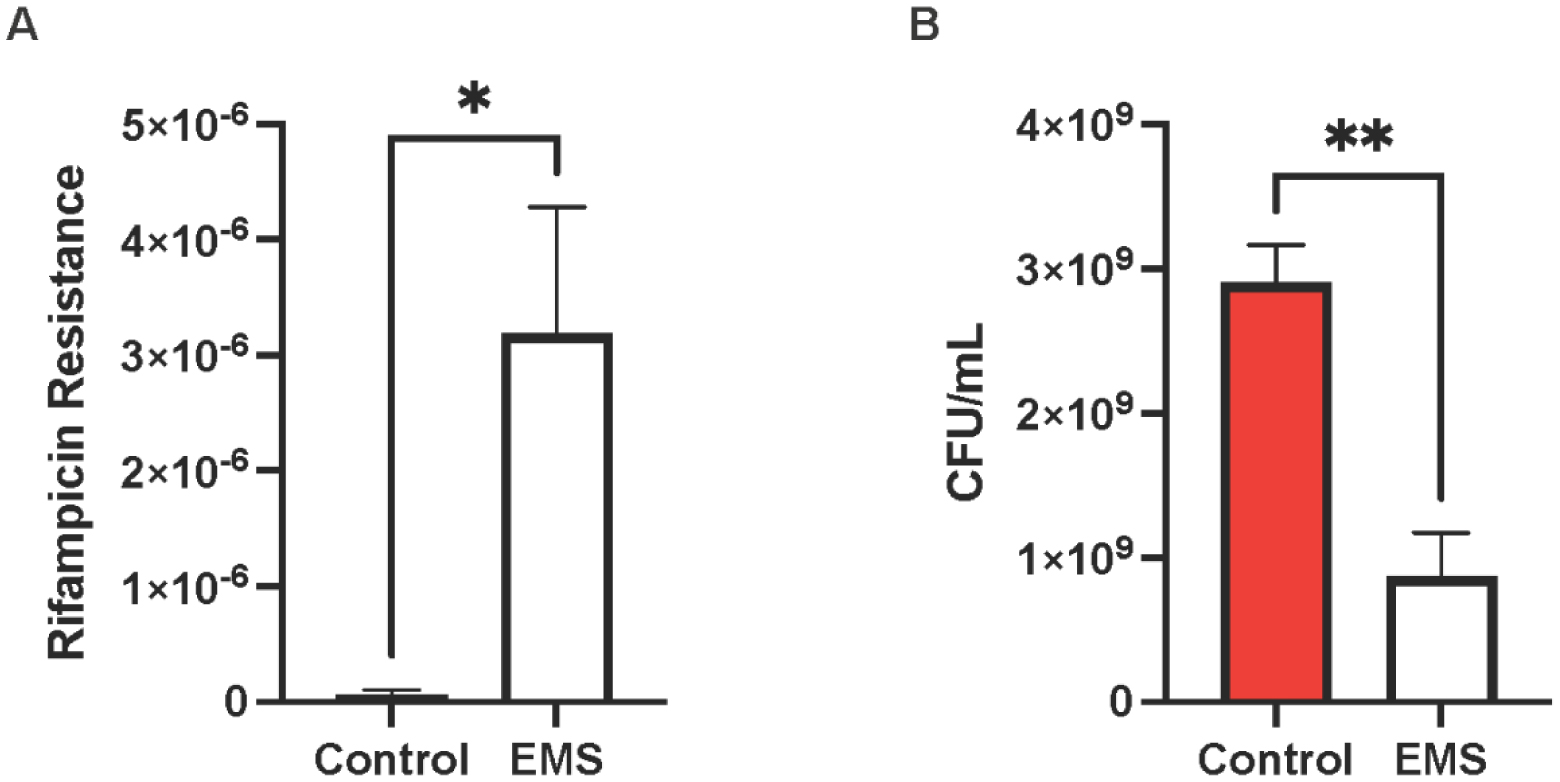
Staphylococcus lugdunensis is a coagulase-negative species responsible for a multitude of infections. These infections often resemble those caused by the more pathogenic staphylococcal species, Staphylococcus aureus, such as skin and soft tissue infections, prosthetic joint infections, and infective endocarditis. Despite a high mortality rate and infections that differ from other coagulase-negative species, little is known regarding S. lugdunensis pathogenesis. The objective of this study is to identify the essential factors for biofilm formation in S. lugdunensis. S. lugdunensis was mutagenized through ethyl methanesulfonate (EMS) exposure, and the individual cells were separated using a cell sorter and examined for biofilm formation at 8 hr and 24 hr timepoints. Mutations that resulted in either increased or decreased biofilm formation were sequenced to identify the genes responsible for the respective phenotypes. A mutation within the S. lugdunensis surface protein A (slsA) gene was common among all of the low biofilm formers, thus suggesting that high expression of this protein is important in biofilm formation. However, other mutations common among the mutants with decreased biofilm formation were in the putative divalent cation transport gene, mgtE. Conversely, a mutation in the gene that codes for the von Willebrand factor binding protein, vwbl, was common among the mutants with increased biofilm formation. Following proteinase K treatment, a significant dispersal of the S. lugdunensis biofilm matrix occurred, thus confirming the presence of primarily protein-mediated biofilms; this is in agreement with previous S. lugdunensis studies. Additionally, all low biofilm formers exhibited decreased protein levels (1.95–2.77 fold change) within the biofilm matrix, while no difference was observed with extracellular DNA (eDNA) or polysaccharides. This study presents a unique methodology to identify genes that affect biofilm formation and sheds light on S. lugdunensis pathogenesis.
Citation: McKenna J. Cruikshank, Justine M. Pitzer, Kimia Ameri, Caleb V. Rother, Kathryn Cooper, Austin S. Nuxoll. Characterization of Staphylococcus lugdunensis biofilms through ethyl methanesulfonate mutagenesis[J]. AIMS Microbiology, 2024, 10(4): 880-893. doi: 10.3934/microbiol.2024038
[1] | Babak Elyasi Far, Mehran Ragheb, Reza Rahbar, Ladan Mafakher, Neda Yousefi Nojookambari, Spyridon Achinas, Sajjad Yazdansetad . Cloning and expression of Staphylococcus simulans lysostaphin enzyme gene in Bacillus subtilis WB600. AIMS Microbiology, 2021, 7(3): 271-283. doi: 10.3934/microbiol.2021017 |
[2] | Kamila Tomoko Yuyama, Thaís Souto Paula da Costa Neves, Marina Torquato Memória, Iago Toledo Tartuci, Wolf-Rainer Abraham . Aurantiogliocladin inhibits biofilm formation at subtoxic concentrations. AIMS Microbiology, 2017, 3(1): 50-60. doi: 10.3934/microbiol.2017.1.50 |
[3] | Débora Luíza Albano Fulgêncio, Rosiane Andrade da Costa, Fernanda Guilhelmelli, Calliandra Maria de Souza Silva, Daniel Barros Ortega, Thiago Fellipe de Araujo, Philippe Spezia Silva, Ildinete Silva-Pereira, Patrícia Albuquerque, Cristine Chaves Barreto . In vitro antifungal activity of pelgipeptins against human pathogenic fungi and Candida albicans biofilms. AIMS Microbiology, 2021, 7(1): 28-39. doi: 10.3934/microbiol.2021003 |
[4] | Anthonia O. Oluduro, Yetunde M. Adesiyan, Olumide O. Omoboye, Adebowale T. Odeyemi . Phenotypic and molecular characterization of Staphylococcus aureus from mobile phones in Nigeria. AIMS Microbiology, 2023, 9(3): 402-418. doi: 10.3934/microbiol.2023021 |
[5] | Afraa Said Al-Adawi, Christine C. Gaylarde, Jan Sunner, Iwona B. Beech . Transfer of bacteria between stainless steel and chicken meat: A CLSM and DGGE study of biofilms. AIMS Microbiology, 2016, 2(3): 340-358. doi: 10.3934/microbiol.2016.3.340 |
[6] | Mohammad Abu-Sini, Mohammad A. Al-Kafaween, Rania M. Al-Groom, Abu Bakar Mohd Hilmi . Comparative in vitro activity of various antibiotic against planktonic and biofilm and the gene expression profile in Pseudomonas aeruginosa. AIMS Microbiology, 2023, 9(2): 313-331. doi: 10.3934/microbiol.2023017 |
[7] | Afia Anjum, Jarin Tabassum, Sohidul Islam, A. K. M. Imrul Hassan, Ishrat Jabeen, Sabbir R. Shuvo . Deciphering the genomic character of the multidrug-resistant Staphylococcus aureus from Dhaka, Bangladesh. AIMS Microbiology, 2024, 10(4): 833-858. doi: 10.3934/microbiol.2024036 |
[8] | Alexis M. Hobbs, Kennedy E. Kluthe, Kimberly A. Carlson, Austin S. Nuxoll . Interruption of the tricarboxylic acid cycle in Staphylococcus aureus leads to increased tolerance to innate immunity. AIMS Microbiology, 2021, 7(4): 513-527. doi: 10.3934/microbiol.2021031 |
[9] | Oluwafolajimi Adesanya, Tolulope Oduselu, Oluwawapelumi Akin-Ajani, Olubusuyi M. Adewumi, Olusegun G. Ademowo . An exegesis of bacteriophage therapy: An emerging player in the fight against anti-microbial resistance. AIMS Microbiology, 2020, 6(3): 204-230. doi: 10.3934/microbiol.2020014 |
[10] | Oriana Simonetti, Samuele Marasca, Matteo Candelora, Giulio Rizzetto, Giulia Radi, Elisa Molinelli, Lucia Brescini, Oscar Cirioni, Annamaria Offidani . Methicillin-resistant Staphylococcus aureus as a cause of chronic wound infections: Alternative strategies for management. AIMS Microbiology, 2022, 8(2): 125-137. doi: 10.3934/microbiol.2022011 |
Staphylococcus lugdunensis is a coagulase-negative species responsible for a multitude of infections. These infections often resemble those caused by the more pathogenic staphylococcal species, Staphylococcus aureus, such as skin and soft tissue infections, prosthetic joint infections, and infective endocarditis. Despite a high mortality rate and infections that differ from other coagulase-negative species, little is known regarding S. lugdunensis pathogenesis. The objective of this study is to identify the essential factors for biofilm formation in S. lugdunensis. S. lugdunensis was mutagenized through ethyl methanesulfonate (EMS) exposure, and the individual cells were separated using a cell sorter and examined for biofilm formation at 8 hr and 24 hr timepoints. Mutations that resulted in either increased or decreased biofilm formation were sequenced to identify the genes responsible for the respective phenotypes. A mutation within the S. lugdunensis surface protein A (slsA) gene was common among all of the low biofilm formers, thus suggesting that high expression of this protein is important in biofilm formation. However, other mutations common among the mutants with decreased biofilm formation were in the putative divalent cation transport gene, mgtE. Conversely, a mutation in the gene that codes for the von Willebrand factor binding protein, vwbl, was common among the mutants with increased biofilm formation. Following proteinase K treatment, a significant dispersal of the S. lugdunensis biofilm matrix occurred, thus confirming the presence of primarily protein-mediated biofilms; this is in agreement with previous S. lugdunensis studies. Additionally, all low biofilm formers exhibited decreased protein levels (1.95–2.77 fold change) within the biofilm matrix, while no difference was observed with extracellular DNA (eDNA) or polysaccharides. This study presents a unique methodology to identify genes that affect biofilm formation and sheds light on S. lugdunensis pathogenesis.
Staphylococcus lugdunensis, which is a coagulase-negative staphylococcal species (CoNS), can often be found on the human skin as normal microbiota [1]. While CoNS are not typically classified as aggressive human pathogens, S. lugdunensis is unique in that it exhibits virulence characteristics and infection patterns closer to that of the major human pathogen Staphylococcus aureus, with infections from minor skin and soft tissue infections to more serious and often life-threatening conditions such as infective endocarditis, prosthetic joint infections, and sepsis [2]. Retrospective clinical studies have found that this unique CoNS had an approximate global mortality rate of 39% in association with heart valve lesions, while endocarditis caused by S. aureus was estimated to have a global mortality rate closer to 20% [3],[4].
One of the primary ways that S. lugdunensis mediates infections similar to those caused by S. aureus is through biofilm formation [2]. These multicellular communities of bacteria are bound together by a matrix formed by biological materials such as polysaccharide intercellular adhesion (PIA), extracellular DNA (eDNA), and proteins [5]. This matrix produced by the bacteria is protective against antibiotics, disinfectants, and the host immune system, which makes biofilm-associated infections extremely difficult to treat [6],[7]. Following biofilm maturation, quorum sensing leads to the dissociation of the biofilm and the subsequential spreading to other areas of the host, thus potentially causing a life-threatening systemic infection [8].
While S. lugdunensis shares the ability to cause severe biofilm-mediated infections with S. aureus, the biofilms of S. lugdunensis remain relatively uncharacterized. The objective of this study is to further characterize these biofilms. Specifically, genetic factors important for S. lugdunensis biofilm formation are examined through ethyl methanesulfonate (EMS) mutagenesis, followed by biofilm screening. One gene of interest is the S. lugdunensis surface protein A (slsA) gene. SlsA is a putative LPXTG cell-wall anchored protein, which is a class of proteins commonly associated with biofilm formation in staphylococci [9]. While the slsA gene was associated with biofilm formation in the EMS screen, there are several other mutations in the low biofilm formers, including mutations in a putative divalent cation transport protein gene, mgtE. Among the high biofilm formers, a common mutation was found in the gene that encodes the von Willebrand factor binding protein, vwbl. These results identified three genes involved in biofilm formation in S. lugdunensis. Furthermore, the characterization of the biofilm matrix in low biofilm formers revealed a decrease in the overall protein composition, whereas the eDNA and polysaccharide concentrations remained unaltered.
S. lugdunensis strain N920143 was used in all experiments [10]. Methicillin susceptible S. aureus strain HG003 and Staphylococcus epidermidis strain 1457 were used as controls in the biofilm dispersal assays [11],[12]. Unless otherwise stated, growth occurred at 37 °C, 225 rpm, and in 3 mL Tryptic Soy Broth (TSB) in a 14 mL snap cap tube.
Following a 1:100 dilution, the overnight cultures were grown for 4.5 hr to mid-exponential phase (OD600 = ~ 3.5). 500 µL of the culture, 250 µL 125 mM HEPES, and 15 µL of EMS (100 µg/mL) were added to a 1.5 mL centrifuge tube, incubated for 45 min at 37 °C, washed with 1% NaCl, and then plated on either TSA or TSA containing rifampicin (10 µg/mL) to determine if mutagenesis occurred. To determine the resistance frequency following mutagenesis, the number of colonies recovered on the TSA containing rifampicin was divided by the number of colonies recovered on the TSA. Following mutagenesis, the cultures were sorted (Sony SH800) onto a rectangular TSA plate at one event per spot with a total of 1344 cells, and were subsequently incubated at 37 °C for 24 hr. The experiments were performed in triplicate and the significance was determined using a student's t-test, P ≤ 0.05.
Overnight cultures of S. lugdunensis were diluted 1:100 in 100 µL TSB within a 96-well tissue culture treated plate (Costar #3628) and were statically incubated at 37 °C for either 8 hr (immature biofilm) or 24 hr (mature biofilm). After the biofilms formed, the non-adherent cells were removed by washing with 1% NaCl and stained with 50 µL of crystal violet dye. The biofilms were stained at room temperature for 2 min. Following staining, the biofilms were washed with distilled water to remove any excess stain. To quantify the biofilm matrix, 100 µL ethanol was used to solubilize the biofilms and the absorbance was measured at 600 nm using a BioTek Synergy H1 plate reader.
Growth of the mutagenized colonies was assessed by diluting the overnight cultures 1:100 in 100 µL TSB in a 96-well microtiter plate. Growth was monitored in a microplate reader (BioTex Synergy H1, serial number 170822D) with continuous shaking at 37 °C for 24 hr. The absorbance was read at 600 nm every 30 min and growth curves were determined for each mutant. The experiments were performed in triplicate and the standard deviation is represented.
DNA (100 ng) from isolates that were found to have high and low biofilms compared to the parent strain were sent to the genomics core facility at the University of Nebraska Medical Center to identify nucleotide changes. Genomic DNA libraries were prepared using the Nextera XT Library Preparation Kit (Illumina) according to manufacturer instructions. A pool of 12 indexed libraries was sequenced using the Illumina NextSeq 550 system using a mid-output flow cell with a 75 paired-end run. vCompressed fastq files were downloaded and stored in a static read-only archive. FastQC, version 0.11.7, was used to guide the quality control and data cleaning. Trimmomatic, version 0.36, was used to clip the adapter sequences and to trim low-quality reads, primarily at the 3′ end of the reads. BWA-MEM, version 0.7.17, was used to align the sequencing reads against the reference genome (S. lugdunensis N920143 complete genome, taxnomomy ID 1034809, downloaded from the National Institutes of Health Genome Browser). Then, Samtools, v.1.9, was used to sort convert the SAM to BAM files. Then, Picard MarkDuplicates, version 2.9, was used to locate and tag the duplicate reads, and the quality was assessed using Qualimap, v.2.2.1. Variant calling was performed with Freebayes, v.0.9.10, where the output included all the predicted variants (reference + observed allele) and a brief report on their quality scores. VCFTools was used to filter the quality scores (QUAL) and the depth of each to a QUAL > 1000 and a DP > 100. The intersection of variants between high biofilm formers was found using the bcftools (version 1.8) isec function, as was the intersection of the variants from the low biofilm formers. SnpEff, v.4.3, was used to annotate the variants and calculated the effects they produced on the known genes. The inputs were predicted variants in the variant call format (VCF).
S. lugdunensis and low biofilm mutants were grown overnight, diluted 1:100 in 100 µL TSB in a 96-well microtiter plate (Costar #3628), and grown for 24 hr at 37 °C. Following incubation, the biofilms were washed with PBS and statically incubated at 37 °C in either 100 µg/mL proteinase K in proteinase K buffer (20 mM HCl Tris, 100 mM NaCl, pH 7.5) or in proteinase K buffer alone. The medium was removed, and the strains were washed again with 1% NaCl. The biofilms were stained with crystal violet dye and were statically incubated for 1 min. The stain was removed by washing with 1% NaCl twice. 100 µL ethanol was added to the wells to solubilize the biofilms, and the absorbance was read at 600 nm using a BioTek Synergy H1 plate reader. The experiments were performed in triplicate and the statistical significance was determined using a two-way ANOVA followed by a post hoc Sidak's test (p ≤ 0.05).
The overnight cultures of S. lugdunensis were diluted 1:100 in 100 µL TSB within a 96-well tissue culture-treated plate (Costar #3628) and were statically incubated at 37 °C for 24 hr. Following incubation, the biofilms were washed with 100 µL 1% NaCl and stained with either 100 µL concanavalin A (50 µg/mL for 60 min, ex488/em545, MP Biomedicals), 4′,6-diamidino-2-phenylindole-DAPI (1 µg/mL for 15 min, ex350/em465, Invitrogen), or SYPRO ruby biofilm matrix stain (1:1 ratio for 30 min, ex450/em610, Invitrogen). Following incubation with the dyes, the biofilms were washed with 100 µL NaCl and their fluorescence were measured using a BioTek Synergy H1 plate reader at each stain's respective excitation/emission spectrum. The experiments were performed in triplicate and the statistical significance was determined using a one-way ANOVA followed by a Dunnett's multiple comparison test (p ≤ 0.05).
To identify the factors involved in S. lugdunensis biofilm formation, EMS mutagenesis was performed in S. lugdunensis. EMS creates random mutations throughout the genome following guanine alkylation. To confirm if mutagenesis occurred, the rifampicin resistance frequency was determined in cultures treated with EMS (Figure 1A; p = 0.0402). The rifampicin resistance frequency in untreated cultures was 6.9 × 10−8. Following EMS treatment, the frequency increased to 3.2 × 10−6, thus indicating a significant increase in mutagenesis. Increased mutagenesis is likely to result in some loss of viability. To ensure that the cells remained viable, the survival was measured following EMS treatment (Figure 1B; p = 0.0051). As expected, there was a reduction in the number of viable cells following treatment; however, the majority of the population (1 × 109 cells/mL) remained viable.
Following the mutagenesis of S. lugdunensis cultures, individual cells were sorted onto rectangular TSA plates at one event per spot. Doublets were excluded by gating on the forward scatter area vs the forward scatter height. Biofilm formation was analyzed in 1344 mutagenized cells for either increases or decreases compared to the parent strain. We reasoned that increases in biofilm formation may be challenging to assess in mature biofilms, and that decreases in biofilm formation that resulted from decreased growth rates would be more apparent in immature biofilms. Therefore, to determine EMS mutants that led to either increased or decreased biofilm formation, screening was performed at both 8 hr (immature biofilms) and 24 hr (mature biofilms). Mutants exhibiting altered biofilm formation at either 8 hr or 24 hr were selected for a further analysis (Figure 2A,B). To ensure that the mutations were affecting biofilm formation and were not an artifact of mutations that altered the metabolism, EMS mutants were monitored for any changes in either the growth rate or the growth yield (Figure 2C,D). Mutants that exhibited significant alterations in either the growth rate or the growth yield compared to the parent strain, N920143, were excluded from further analyses, as the biofilm defects were likely a result of changes in the growth rates. Following a growth rate analysis, four low biofilm formers (5C10, 5C11, 5D7, 5H3) and eight high biofilm formers (3B5, 5C4, 8A7, 8C9, 9A2, 10F1, 10H5, 13G2) were selected for a sequence analysis to identify the mutations responsible for the respective biofilm phenotypes. While it was initially predicted that increases in biofilm formation wouldn't be apparent in mature biofilms, a significant increase in biofilm formation was apparent in all high biofilm formers at 24 hr. Therefore, low biofilm and high biofilm formers were designated by having a statistically significantly decrease or increase in biofilm formation compared to the N920143 parent strain in a mature biofilm as measured by crystal violet staining.
Sequencing data was used to search for non-synonymous variants in high and low biofilm formers. Data was high quality and 97% of reads were retained after pre-processing the sequencing data with Trimmomatic. The GC content was reported at 34%, which is in line with the reported S. lugdunensis genome GC content of 33.7953%. There was a high coverage of the genome, with an average coverage range of 130 to 290. The average read mapping after aligning the sequencing reads to the reference genome was 99.94%, with a range of 99.91–99.95% mapping across all the samples. The data was deposited with links to BioProject accession number PRJNA1140638 in the NCBI BioProject database (https://www.ncbi.nlm.nih.gov/bioproject/1140638).
SnpEff describes variant impact definitions as HIGH, MODERATE, LOW, or MODIFIER based on the anticipated impact on the analyzed protein or gene product. For this study, we focused on variants annotated as HIGH (disruptive impact or stop gain mutations) and MODERATE (some possible impact or missense variants). Among the four strains that exhibited a decreased biofilm formation, there were two common variants. A mutation in the slsA gene and the mgtE gene resulted in a missense and a frameshift mutation, respectively (Table 1). A common mutation among the eight high biofilm formers was found in the vwbl gene, which resulted in a missense mutation (Table 2).
Chromosome Position | Ref. | ALT | SnpEff Annot. | Gene Id | Transcript Id/Protein | Function | Type | Sequence |
1957808 | TTAT | TT | HIGH | SLUG_18540 (mgtE) | CCB54356.1 | Putative divalent cation transport protein | Frameshift | 1957803..1959080 |
372929 | CCAA | GGAA | MOD. | SLUG_03480 (slsA) | CCB52765.1 | Putative LPXTG cell wall-anchored protein | Missense | 368457..374249 |
Chromosome Position | Ref. | ALT | SnpEff Annot. | Gene Id | Transcript Id/Protein | Function | Type | Sequence |
2465879 | CTCTTG | TTCCTT | MOD. | SLUG_23290 (vwbl) | CCB54854.1 | Von Willebrand factor-binding protein precursor | Missense | 2464430..2470009 |
While two unique mutations were identified to be present among all the low biofilm formers and one unique mutation was identified among all the high biofilm formers, slsA was of a particular interest, as surface proteins that are anchored to the cell wall by an LPXTG motif have been shown to be important for biofilm formation in closely related species, namely S. aureus and S. epidermidis [13],[14]. Based on these sequencing results, it was hypothesized that the S. lugdunensis biofilms were primarily protein mediated. To determine if this was indeed the case, the biofilms were treated with proteinase K. Following the proteinase K challenge, the S. aureus and S. lugdunensis biofilms were significantly disrupted; alternatively, there was no significant decrease in S. epidermidis (Figure 3; p = 0.0487 and p = 0.0049, respectively). These findings support the sequence analysis which identified a gene (slsA) that coded for a LPXTG cell-wall anchored protein as a factor that could be involved with S. lugdunensis biofilm formation.
Based on the findings in the biofilm dispersal assay, we reasoned that the low biofilm formers from EMS mutagenesis would not be affected by the proteinase K treatment, as the SlsA protein was already disrupted in these mutants. Following proteinase K dispersal, the wild-type and three of the low biofilm formers, 5C11, 5D7, and 5H3, exhibited a significant dispersal of the biofilm matrix, while there was no change in 5C10 (Figure 4). These results suggest that other proteins alongside SlsA are important for S. lugdunensis biofilm formation.
Based on the unexpected findings that the proteinase K treatment further reduced bacterial burden, the biofilm composition was analyzed to determine if the low biofilm formation in the EMS mutants was a result of either decreased proteins, eDNA, polysaccharide, or a combination of those factors. As expected, the four EMS mutants with a low biofilm formation all exhibited a significant decrease in protein concentrations within the biofilm matrix (Figure 5A). When examining the composition of eDNA in the biofilm matrix, no difference was observed between the wild-type and the low biofilm formers (Figure 5B). Interestingly, when examining the polysaccharides within the biofilm matrices, the four EMS mutants showed decreased polysaccharide contents compared to the N920143 wild-type strain, albeit not significant decreases (Figure 5C). These findings support the notion that S. lugdunensis forms protein-mediated biofilms and slsA is likely involved in this process.
S. lugdunensis is responsible for infections ranging from skin and soft tissue infections to biofilm mediated infections including prosthetic joint infections and endocarditis. Among all bacterial infections, it is estimated that biofilms are associated with 60% to 80% of infections [15]. Biofilms are problematic for not only the host's immune system, but also contribute to antibiotic therapy failure [6],[16],[17]. While S. lugdunensis is classified as a coagulase-negative staphylococci, the clinical features of this pathogen more closely resemble S. aureus [18]. Despite the pathogenic nature and clinical importance, relatively little is known regarding the factors that are required for S. lugdunensis biofilm formation.
Through ethyl methanesulfonate mutagenesis coupled with cell sorting, we identified several factors that are likely to impact biofilm formation in S. lugdunensis. A common mutation in the slsA gene and the mgtE gene were found among the low biofilm formers (Table 1), while a common mutation in the vwbl gene was found among the high biofilm formers (Table 2). The mutation in the slsA gene that resulted in a decreased biofilm formation was not surprising, as it encodes a surface protein that is anchored to the cell wall through an LPXTG motif. Similar surface proteins have been found to play roles in biofilm formation in other staphylococcal species [13]. SasC, SasG, and Bap in S. aureus and Aap in S. epidermidis are surface proteins that are important for biofilm formation that contain an LPXTG motif [19]–[22]. Additionally, this is in agreement with previous work which found that S. lugdunensis biofilms were primarily protein mediated and not polysaccharide mediated [23],[24].
In this study, all low biofilm formers which were identified through EMS mutagenesis contained mutations in both mgtE and slsA, thus suggesting that both mutations were important for the observed phenotype. The mgtE encodes putative divalent cation transport proteins. Divalent cations are involved in cell-to-cell adhesion due to interactions with teichoic acids in the cell wall; additionally, divalent cation concentrations have been shown to affect extracellular polysaccharide and proteinaceous materials in biofilms [25]–[27]. It stands to reason that the observed decrease in biofilm formation may be a result of both the slsA and mgtE mutations that altered the composition of the biofilm matrix. However, a number of individual point mutations existed within each individual strain; therefore, one cannot exclude the possibility of other mutations contributing to these biofilm phenotypes. It is important to note that no mutations outside of slsA and mgtE were common among the four low biofilm formers; additionally, they were absent from the high biofilm formers mutants. Additional point mutations could explain the findings observed with 5C10 not showing a further reduction in biofilm formation following proteinase K dispersal (Figure 4). While the protein composition was significantly decreased in all four of the low biofilm formers, 5C10 exhibited a stronger phenotype compared to the other EMS mutants, thus indicating that other point mutations likely impact the biofilm formation (Figure 5). In addition to the decrease in proteins within the biofilm matrix, there was a slight reduction in the observed polysaccharides within the mutants (Figure 5), which could be the result of the mutation in mgtE.
Interestingly, a mutation within vwbl, which codes for the von Willebrand factor binding protein, resulted in an increased biofilm formation. Recently, work in Mycobacterium tuberculosis also implicated a protein that contained the von Willebrand factor domain in biofilm formation, which supports the finding that vwbl may be involved in biofilm formation [28]. In S. aureus, a number of studies highlighted the importance of the von Willebrand factor binding protein during in vivo adherence and the establishment of infection [29]–[32]. Fitting with these findings, a mutation of vwbl in S. lugdunensis resulted in reduced virulence within a rat endocarditis infection model [33].
The staphylococcal species are one of the primary causes of biofilm-mediated indwelling medical device infections. Many studies have aimed to understand the mechanism of biofilm formation in other staphylococcal species, such as S. aureus and S. epidermidis; however, the factors responsible for biofilm formation in S. lugdunensis are less studied. The last couple of decades have led to a better appreciation for the clinical importance of S. lugdunensis; however, there is much that is unknown regarding the pathogenesis of this organism. While this study identified mutations that were common among four mutants with reduced biofilm formation and one mutation that resulted in an enhanced biofilm formation, genetic deletions of these genes are needed to confirm their role in biofilm formation in S. lugdunensis. While slsA, mgtE, and vwbl were the only mutations identified in this study, many single nucleotide polymorphisms exist in each mutant sequence and could impact the observed biofilm phenotypes. Additionally, EMS mutagenesis is restricted single nucleotide polymorphisms (SNPs), many of which could be silent or result in only a partial loss of protein function, thus leading to a number of genes involved with biofilm formation going unidentified. Despite these limitations, this work highlighted common mutations among all high biofilm formers, as well as those present among all low biofilm formers, thus suggesting the importance of these factors to S. lugdunensis biofilm formation Additional experimentation is required to further understand the mechanism of biofilm formation in S. lugdunensis.
The authors declare they have not used Artificial Intelligence (AI) tools in the creation of this article.
[1] |
Heilbronner S, Foster TJ (2021) Staphylococcus lugdunensis: A skin commensal with invasive pathogenic potential. Clin Microbiol Rev 34. https://doi.org/10.1128/cmr.00205-20 ![]() |
[2] | Parthasarathy S, Shah S, Raja Sager A, et al. (2020) Staphylococcus lugdunensis: Review of epidemiology, complications, and treatment. Cureus 12: e8801. https://doi.org/10.7759/cureus.8801 |
[3] |
Liu PY, Huang YF, Tang CW, et al. (2010) Staphylococcus lugdunensis infective endocarditis: A literature review and analysis of risk factors. J Microbiol Immunol Infect 43: 478-484. https://doi.org/10.1016/S1684-1182(10)60074-6 ![]() |
[4] |
Sandre RM, Shafran SD (1996) Infective endocarditis: Review of 135 cases over 9 years. Clin Infect Dis 22: 276-286. https://doi.org/10.1093/clinids/22.2.276 ![]() |
[5] |
Karygianni L, Ren Z, Koo H, et al. (2020) Biofilm matrixome: Extracellular components in structured microbial communities. Trends Microbiol 28: 668-681. https://doi.org/10.1016/j.tim.2020.03.016 ![]() |
[6] |
Theis TJ, Daubert TA, Kluthe KE, et al. (2023) Staphylococcus aureus persisters are associated with reduced clearance in a catheter-associated biofilm infection. Front Cell Infect Microbiol 13: 1178526. https://doi.org/10.3389/fcimb.2023.1178526 ![]() |
[7] |
Otto M (2018) Staphylococcal biofilms. Microbiol Spectr 6. https://doi.org/10.1128/microbiolspec.gpp3-0023-2018 ![]() |
[8] |
Sionov RV, Steinberg D (2022) Targeting the holy triangle of quorum sensing, biofilm formation, and antibiotic resistance in pathogenic bacteria. Microorganisms 10: 1239. https://doi.org/10.3390/microorganisms10061239 ![]() |
[9] |
Hernandez-Cuellar E, Tsuchiya K, Valle-Rios R, et al. (2023) Differences in biofilm formation by methicillin-resistant and methicillin-susceptible Staphylococcus aureus strains. Diseases 11: 160. https://doi.org/10.3390/diseases11040160 ![]() |
[10] |
Heilbronner S, Holden MT, van Tonder A, et al. (2011) Genome sequence of Staphylococcus lugdunensis N920143 allows identification of putative colonization and virulence factors. FEMS Microbiol Lett 322: 60-67. https://doi.org/10.1111/j.1574-6968.2011.02339.x ![]() |
[11] |
Herbert S, Ziebandt AK, Ohlsen K, et al. (2010) Repair of global regulators in Staphylococcus aureus 8325 and comparative analysis with other clinical isolates. Infect Immun 78: 2877-2889. https://doi.org/10.1128/iai.00088-10 ![]() |
[12] |
Mack D, Siemssen N, Laufs R (1992) Parallel induction by glucose of adherence and a polysaccharide antigen specific for plastic-adherent Staphylococcus epidermidis: Evidence for functional relation to intercellular adhesion. Infect Immun 60: 2048-2057. https://doi.org/10.1128/iai.60.5.2048-2057.1992 ![]() |
[13] |
McCarthy H, Rudkin JK, Black NS, et al. (2015) Methicillin resistance and the biofilm phenotype in Staphylococcus aureus. Front Cell Infect Microbiol 5: 1. https://doi.org/10.3389/fcimb.2015.00001 ![]() |
[14] |
Khodaparast L, Khodaparast L, Shahrooei M, et al. (2016) The possible role of Staphylococcus epidermidis LPxTG surface protein SesC in biofilm formation. PLoS One 11: e0146704. https://doi.org/10.1371/journal.pone.0146704 ![]() |
[15] |
Assefa M, Amare A (2022) Biofilm-associated multi-drug resistance in hospital-acquired infections: A review. Infect Drug Resist 15: 5061-5068. https://doi.org/10.2147/IDR.S379502 ![]() |
[16] |
Jamal M, Ahmad W, Andleeb S, et al. (2018) Bacterial biofilm and associated infections. J Chin Med Assoc 81: 7-11. https://doi.org/10.1016/j.jcma.2017.07.012 ![]() |
[17] |
Lewis K (2010) Persister cells. Annu Rev Microbiol 64: 357-372. https://doi.org/10.1146/annurev.micro.112408.134306 ![]() |
[18] |
Lourtet-Hascoet J, Bicart-See A, Felice MP, et al. (2016) Staphylococcus lugdunensis, a serious pathogen in periprosthetic joint infections: Comparison to Staphylococcus aureus and Staphylococcus epidermidis. Int J Infect Dis 51: 56-61. https://doi.org/10.1016/j.ijid.2016.08.007 ![]() |
[19] |
Schroeder K, Jularic M, Horsburgh SM, et al. (2009) Molecular characterization of a novel Staphylococcus aureus surface protein (SasC) involved in cell aggregation and biofilm accumulation. PLoS One 4: e7567. https://doi.org/10.1371/journal.pone.0007567 ![]() |
[20] | Corrigan RM, Rigby D, Handley P, et al. (2007) The role of Staphylococcus aureus surface protein SasG in adherence and biofilm formation. Microbiolog 153: 2435-2446. https://doi.org/10.1099/mic.0.2007/006676-0 |
[21] |
Cucarella C, Solano C, Valle J, et al. (2001) Bap, a Staphylococcus aureus surface protein involved in biofilm formation. J Bacteriol 183: 2888-2896. https://doi.org/10.1128/jb.183.9.2888-2896.2001 ![]() |
[22] |
Rohde H, Burdelski C, Bartscht K, et al. (2005) Induction of Staphylococcus epidermidis biofilm formation via proteolytic processing of the accumulation-associated protein by staphylococcal and host proteases. Mol Microbiol 55: 1883-1895. https://doi.org/10.1111/j.1365-2958.2005.04515.x ![]() |
[23] |
Frank KL, Patel R (2007) Poly-N-acetylglucosamine is not a major component of the extracellular matrix in biofilms formed by icaADBC-positive Staphylococcus lugdunensis isolates. Infect Immun 75: 4728-4742. https://doi.org/10.1128/iai.00640-07 ![]() |
[24] |
Missineo A, Di Poto A, Geoghegan JA, et al. (2014) IsdC from Staphylococcus lugdunensis induces biofilm formation under low-iron growth conditions. Infect Immun 82: 2448-2459. https://doi.org/10.1128/iai.01542-14 ![]() |
[25] |
Steiger EL, Muelli JR, Braissant O, et al. (2020) Effect of divalent ions on cariogenic biofilm formation. BMC Microbiol 20: 1-11. https://doi.org/10.1186/s12866-020-01973-7 ![]() |
[26] |
Yang J, Wei W, Pi S, et al. (2015) Competitive adsorption of heavy metals by extracellular polymeric substances extracted from Klebsiella sp. J1. Bioresour Technol 196: 533-539. https://doi.org/10.1016/j.biortech.2015.08.011 ![]() |
[27] |
Sarkisova S, Patrauchan MA, Berglund D, et al. (2005) Calcium-induced virulence factors associated with the extracellular matrix of mucoid Pseudomonas aeruginosa biofilms. J Bacteriol 187: 4327-4337. https://doi.org/10.1128/jb.187.13.4327-4337.2005 ![]() |
[28] |
Zhao X, Duan X, Dai Y, et al. (2020) Mycobacterium Von Willebrand factor protein MSMEG_3641 is involved in biofilm formation and intracellular survival. Future Microbiol 15: 1033-1044. https://doi.org/10.2217/fmb-2020-0064 ![]() |
[29] |
Motta C, Pellegrini A, Camaione S, et al. (2023) von Willebrand factor-binding protein (vWbp)-activated factor XIII and transglutaminase 2 (TG2) promote cross-linking between FnBPA from Staphylococcus aureus and fibrinogen. Sci Rep 13: 11683. https://doi.org/10.1038/s41598-023-38972-3 ![]() |
[30] |
Viljoen A, Viela F, Mathelie-Guinlet M, et al. (2021) Staphylococcus aureus vWF-binding protein triggers a strong interaction between clumping factor A and host vWF. Commun Biol 4: 453. https://doi.org/10.1038/s42003-021-01986-6 ![]() |
[31] |
Viela F, Prystopiuk V, Leprince A, et al. (2019) Staphylococcus aureus protein A to von Willebrand factor is regulated by mechanical force. MBio 10. https://doi.org/10.1128/mbio.00555-19 ![]() |
[32] |
Thomas S, Liu W, Arora S, et al. (2019) The complex fibrinogen interactions of the Staphylococcus aureus coagulases. Front Cell Infect Microbiol 9: 106. https://doi.org/10.3389/fcimb.2019.00106 ![]() |
[33] |
Heilbronner S, Hanses F, Monk IR, et al. (2013) Sortase A promotes virulence in experimental Staphylococcus lugdunensis endocarditis. Microbiology 159: 2141-2152. https://doi.org/10.1099/mic.0.070292-0 ![]() |
Chromosome Position | Ref. | ALT | SnpEff Annot. | Gene Id | Transcript Id/Protein | Function | Type | Sequence |
1957808 | TTAT | TT | HIGH | SLUG_18540 (mgtE) | CCB54356.1 | Putative divalent cation transport protein | Frameshift | 1957803..1959080 |
372929 | CCAA | GGAA | MOD. | SLUG_03480 (slsA) | CCB52765.1 | Putative LPXTG cell wall-anchored protein | Missense | 368457..374249 |
Chromosome Position | Ref. | ALT | SnpEff Annot. | Gene Id | Transcript Id/Protein | Function | Type | Sequence |
2465879 | CTCTTG | TTCCTT | MOD. | SLUG_23290 (vwbl) | CCB54854.1 | Von Willebrand factor-binding protein precursor | Missense | 2464430..2470009 |
Chromosome Position | Ref. | ALT | SnpEff Annot. | Gene Id | Transcript Id/Protein | Function | Type | Sequence |
1957808 | TTAT | TT | HIGH | SLUG_18540 (mgtE) | CCB54356.1 | Putative divalent cation transport protein | Frameshift | 1957803..1959080 |
372929 | CCAA | GGAA | MOD. | SLUG_03480 (slsA) | CCB52765.1 | Putative LPXTG cell wall-anchored protein | Missense | 368457..374249 |
Chromosome Position | Ref. | ALT | SnpEff Annot. | Gene Id | Transcript Id/Protein | Function | Type | Sequence |
2465879 | CTCTTG | TTCCTT | MOD. | SLUG_23290 (vwbl) | CCB54854.1 | Von Willebrand factor-binding protein precursor | Missense | 2464430..2470009 |