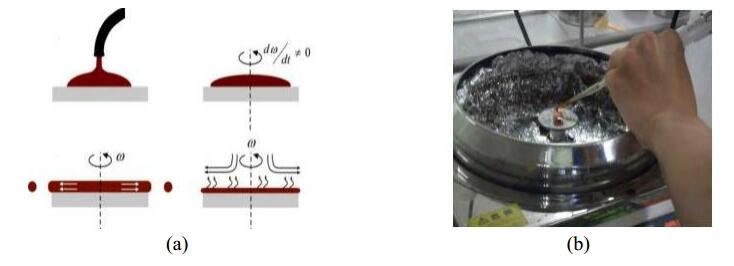
Citation: Kulpash Iskakova, Rif Akhmaltdinov, Orken Mamyrbayev. Production of thin copper oxide films and its electronic density[J]. AIMS Materials Science, 2019, 6(3): 454-463. doi: 10.3934/matersci.2019.3.454
[1] | Wiebke Langgemach, Andreas Baumann, Manuela Ehrhardt, Thomas Preußner, Edda Rädlein . The strength of uncoated and coated ultra-thin flexible glass under cyclic load. AIMS Materials Science, 2024, 11(2): 343-368. doi: 10.3934/matersci.2024019 |
[2] | Shuhan Jing, Adnan Younis, Dewei Chu, Sean Li . Resistive Switching Characteristics in Electrochemically Synthesized ZnO Films. AIMS Materials Science, 2015, 2(2): 28-36. doi: 10.3934/matersci.2015.2.28 |
[3] | Nur Jassriatul Aida binti Jamaludin, Shanmugan Subramani . Thermal performance of LED fixed on CVD processed ZnO thin film on Al substrates at various O2 gas flow rates. AIMS Materials Science, 2018, 5(2): 246-256. doi: 10.3934/matersci.2018.2.246 |
[4] | Ruijin Hong, Jialin Ji, Chunxian Tao, Daohua Zhang, Dawei Zhang . Fabrication of Au/graphene oxide/Ag sandwich structure thin film and its tunable energetics and tailorable optical properties. AIMS Materials Science, 2017, 4(1): 223-230. doi: 10.3934/matersci.2017.1.223 |
[5] | Yaorong Su, Weiguang Xie, Jianbin Xu . Towards low-voltage organic thin film transistors (OTFTs) with solution-processed high-k dielectric and interface engineering. AIMS Materials Science, 2015, 2(4): 510-529. doi: 10.3934/matersci.2015.4.510 |
[6] | Muhamed Shajudheen V P, Saravana Kumar S, Senthil Kumar V, Uma Maheswari A, Sivakumar M, Sreedevi R Mohan . Enhancement of anticorrosion properties of stainless steel 304L using nanostructured ZnO thin films. AIMS Materials Science, 2018, 5(5): 932-944. doi: 10.3934/matersci.2018.5.932 |
[7] | K. Ravindranadh, K. Durga Venkata Prasad, M.C. Rao . Spectroscopic and luminescent properties of Co2+ doped tin oxide thin films by spray pyrolysis. AIMS Materials Science, 2016, 3(3): 796-807. doi: 10.3934/matersci.2016.3.796 |
[8] | Xuan Luc Le, Nguyen Dang Phu, Nguyen Xuan Duong . Enhancement of ferroelectricity in perovskite BaTiO3 epitaxial thin films by sulfurization. AIMS Materials Science, 2024, 11(4): 802-814. doi: 10.3934/matersci.2024039 |
[9] | Vishanth Uppu, Kunal Mishra, Libin K. Babu, Ranji Vaidyanathan . Understanding the influence of graphene and nonclay on the microcracks developed at cryogenic temperature. AIMS Materials Science, 2019, 6(4): 559-566. doi: 10.3934/matersci.2019.4.559 |
[10] | Alberto Debernardi . Ab initio calculation of band alignment of epitaxial La2O3 on Si(111) substrate. AIMS Materials Science, 2015, 2(3): 279-293. doi: 10.3934/matersci.2015.3.279 |
Consider the synthesis of inorganic substances by chemical method. Let's start with the synthesis of copper sulfide and cadmium. Copper and cadmium sulfide was synthesized by passing H2S gas to copper and sulfide salts. This method is cheaper at this stage. The method differs in productivity and purity of an exit. The obtained substances were further used as elements of the "fusion" of the substance with the organic polymer.
In this paper, the conditions for the formation of copper(Ⅱ) oxide during electrolysis by alternating current of industrial frequency are studied. The electrolysis was carried out in a neutral medium in a solution of sodium sulfate using titanium wire and lamellar copper electrodes. We used the titanium electrode based on the ability of titanium to form valve oxide films. The experiment was performed using potassium sulfate as the electrolyte. There are a large number of methods for producing metal oxides based on gas-phase, plasma-chemical, thermal and other processes [1,2,3]. Detonation synthesis and electric explosion are developing. The most well-studied are methods based on the precipitation from solutions of salts and subsequent hydrolysis, which make it possible to obtain hydrated metal oxides in the form of gels, which have a high dispersion and developed porous structure. Recently, new promising directions for the synthesis of metal oxide powders have been developed, one of which is the electrochemical method. The main advantage of this method is the possibility of obtaining very pure oxides, and the regulation of the electrical parameters of the electrolysis process allows the formation of powders with a given dispersion, which further enhances its practical value.
In the present work, the conditions for the formation of copper(Ⅱ) oxide during electrolysis by alternating current of industrial frequency were studied [4,5]. The electrolysis was carried out in a neutral medium in a solution of sodium sulfate using titanium wire and lamellar copper electrodes.
At low temperatures, copper dissolves to form copper hydroxide, and at higher temperatures to form metal oxides. The values of the apparent activation energy are calculated, which testifies to the process at a low temperature in the kinetic, at a higher—in the diffusion modes.
The trace element composition of the copper(Ⅱ) oxide sample was investigated by X-ray fluorescence analysis on an X-ray fluorescence spectrometer with processing the data obtained using the program attached to the spectrometer, which forms the images of the spectrum (dependence of intensity on energy) and the table of the sample composition.
This technique for obtaining copper oxide was used for the first time with selected characteristic parameters of a spin coating apparatus (spin coating) MikasaSpinCoater 1H-D7 and electrolyte composition to obtain a uniform film of a given thickness covering the entire substrate.
In the work presented here, silicon substrates and glass with a layer of indium tin oxide (OIO) deposited on it were used as substrates. The glass is cut into rectangular pieces with a characteristic size of 2 cm, after which the etching is carried out in order to subsequently obtain the desired geometry of the device. The etching procedure includes coating the indium tin oxide site with a protective paraffin tape and the chemical etching process itself, which consists in bringing the substrate into contact with a rapidly reacting mixture of zinc powder and hydrochloric acid diluted in half. The atomic hydrogen released during the reaction will react with indium and tin oxides, which will lead to the removal of unprotected portions of indium tin oxide from the substrate. The most important step in the preparation of substrates is cleaning.
Cleaning consists of sequential washing of the substrates in an ultrasonic bath for several minutes in a detergent mixture and purified water (several times in water). Acetone and isopropanol are used as a mixture. As purified water, it is consistently used first distilled and then distilled, but also passed through two Elgacan deionizing filters. Each cleaning process lasts about 10 minutes, and after washing the substrate is dried.
Thin layers of different materials (50–100 nm) are applied using a spin coating apparatus (spin coating) MikasaSpinCoater 1H-D7 (Figure 1b). The method consists in the accumulation of a solution of a substance on a rotating substrate (or on a fixed substrate with its subsequent unwinding) [6,7]. By changing the concentration of the solution, the solvent, the rotational speed and the method of accumulation, it is possible to achieve a uniform film of a given thickness covering the entire substrate (Figure 1a). When applying several layers one after the other, it must be borne in mind that if the previous layer dissolves in the solvent applied, the first may be washed off. Of great importance is the wettability of the surface on which the film is applied, the solvent from which it is made. The higher the wettability, the easier it is to obtain a film covering the entire substrate.
We used this method of obtaining copper oxide with some changes: we selected the characteristic parameters for a spin coating apparatus (spin coating) MikasaSpinCoater 1H-D7, as well as the composition of the electrolyte, so that the output of the film of a given thickness was the same throughout its area should have been the whole substrate.
The rotation speed and rotation time is selected depending on the properties and characteristics of the applied material (ω = 1000–5000 rpm; t = 1–3 min) and also on the thickness of the film layer desired by the researcher (30–100 nm). That is, the higher the rotation speed and the rotation time, the thinner the film is. After obtaining a thin film layer, the substrate is removed from the apparatus, and a thermal annealing process (100–200 ℃, depending on the material properties) is carried out for 15–60 minutes, to remove residual solvent [8].
It is necessary to separately mention the preparation of polymer solutions. Polymer solutions were prepared by applying sound to an ultrasonic bath of mixtures of polymers and solvents for 10–20 minutes to several hours (depending on the polymer). Epoxy silane is also added to polymer solutions in ethanol to improve film-forming properties.
After cleaning and obtaining inorganic compounds, the next stage is sputtering onto the plates. Two methods are used in sputtering: vacuum spraying through decomposition using argon gas and the method of vacuum deposition using thermal decomposition. This series of experiments is applicable to obtain thin and uniform surfaces [9]. To do this, set the difference between temperature and pressure [10].
Cathode electrodes are deposited by thermal spraying of the metal in a vacuum. As a rule, aluminum was chosen as the material of the electrodes. Spray is carried out in a vacuum unit. The process lasts 2 hours, P = 2 × 10−5 Pa, Т = 800–1000 ℃. When spraying, a mask is used in order to achieve a given device geometry. The thickness of the sprayed layer is, as a rule, not less than 100 nm. Contacts from thin metal wire are attached to the electrodes with the help of silver paste.
The aluminum layer serves as a kind of energy mirror for charge carriers. Electrons and holes in semiconductors tend to recombine, i.e., move from free to bound state. Recombination takes place, for example, if two charges with different signs meet at one point. One of the extreme cases is surface recombination [11,12], since any surface is a set of dangling bonds of the crystal lattice—"traps" for free charge carriers [6,7]. It is in order to reduce the effect of this type of recombination in solar cells with the help of aluminum metallization that the so-called BSF (back side field) is formed—the back field, "mirroring" charge carriers that have not yet had time to contribute to the generation current [13].
Thin-film technology has great potential to reduce the cost of solar modules [14]. Thin-film technology has a number of specific applications that are impossible or difficult when using crystalline semiconductors (flexible modules, translucent modules, etc.) [15,16]. One of the advantages of thin-film technology is to obtain layers at low temperature [17,18]. This makes it possible to create semiconductor structures on flexible substrates [19,20]. Solar cells on a flexible basis are lightweight, mounted on any surface and can be used to make bags, covers, fit into clothes, etc. Finally, a significant advantage of thin-film technology is the ability to create instrument structures on very large areas [21].
An ultraviolet radiation apparatus, an ultrasonic radiation apparatus, a tile, an atomic-force microscope (AFM, Seiko Instruments SPA-300/SPI-3800), a spectroscopic ellipsometer (SE), a stylus profilometer (SP, AlphastepIQ) are used. Also, a sunlight stimulator (BunkoukeikiCEP-25BX) is used to remove current–voltage curves (J–V) in the dark and under illumination (parameters: AM 1.5 G, 100 mA/cm2).
During the experiments, silicon plates, ITO-plates, isopropanol, methanol, hydrofluoric acid, distilled water, anthracene, styrene, poly(pyroll sulfate), Cu2O (copper oxide Ⅱ), aluminum wires, copper wires, silver paste [22,23].
The following physico-chemical research methods used for measurements were also used: NMR spectroscopy (sample preparation, solvents, standards, instrument characteristics), EPR, IR and electron spectroscopy, cyclic voltammetry, X-ray phase analysis, microelement microscopy [24,25,26,27].
It is possible to obtain electricity from solar energy by the method of thermodynamic transformation almost the same way as from other energy sources, however, solar radiation incident on the earth has a number of characteristic features [1,28]:
1. low energy flux density;
2. daily and seasonal cyclicity;
3. weather dependence.
Therefore, in the thermodynamic conversion of this energy into electrical energy, one should strive to ensure that the use of thermal regimes does not introduce serious limitations to the operation of the system and does not arise difficulties associated with its use, i.e., such a system should have storage devices to eliminate random fluctuations in operating conditions or provide the necessary changes in energy production over time.
A number of experiments have been carried out on the listed tasks. The first work is on the synthesis of copper(Ⅰ) oxide, where the method of electrolysis of metals is used [2,4,5,29]. For electrolysis, NaCl solutions of different concentrations are used, which are cheap and affordable. Copper plates serve as an anode and cathode (Figure 2a). Obtaining samples of copper oxide through electrolysis (Figure 2b) has greater productivity and gives a net output for analysis [30].
This method is new and promising in obtaining copper(Ⅰ) oxide. Cu2O can be obtained both in crystalline form and in amorphous, in the form of powder. By this method several samples of oxide were obtained. The purity results are checked on an X-ray phase analyzer [3].
The next stage is the synthesis of ferrate and cuprate. A cell is used as in the first paper. Iron and copper were used instead of copper. The result of cuprate or ferrate production through electrolysis is the following: average productivity; the output is pure for analysis.
We investigated the trace element composition of a copper(Ⅱ) oxide sample by us using X-ray fluorescence analysis on an X-ray fluorescence spectrometer. The data obtained are processed by the program that comes with the spectrometer. This program formed the image of the spectrum (dependence of intensity on energy), as well as a table of the composition of the sample under study.
The microelement analysis of the copper oxide powders obtained is shown in Figure 3. Table 1 below lists the data on the composition of the obtained powders in the synthesis of copper oxide.
Copper | Iron | Titanium | Oxygen | Impurities |
62% | 0.5% | 0.2% | 32% | 5.3% |
Our experiments were based on the results of our calculations of the electron density for Cu2O. For Cu2O, the three-dimensional filled cell with respect to the electron density lies higher than the empty one [31,32], and so all the nanoparticles of the metal and semiconductor-cuprite with n = 16 indicate in favor of the empty cell of the structure (Figure 4), but the energy difference between empty and filled cells decreases.
For (Cu2O)n n = 16 and 23, the empty cells deform more and develop a more significant sp3 bond. That is why cell filling is more appropriate in these cases, as shown in Figure 4 (see also [31]). At 23, a significant decrease in electron density ρ is observed, and this shows that the tendency of the structure of the filled cell becomes much stronger than in the case of n = 16. (Cu2O)16 shows a remarkable transition to the three-dimensional structure of a filled cell with the highest electronic density ρ of (Cu2O)23 and a large discontinuity in the exchange bonds of lengths 1.87, 1.90, and 1.92 Å compared to n = 16. The results of this work correspond to our results in earlier labors [31,32,33,34].
The trace element analysis of the obtained copper oxide thin film shows 62% of copper and 32% of oxygen in quantitative equivalent of its composition. The remaining substances make up only 6%. When we simulate the structure and electron density of the copper oxide of the group of nearby neighbors of 10, 16, 23 positive Cu ions and negative O in the inter-sphere space, there are one electron per two spacings, which corresponds to the percentage ratios of the experimental results for thin films (Cu2O)n.
The simulated and calculated electron density in reverse space shows the following pattern. The graphic image (Figure 4) illustrates solutions with a domain of definition on the plane of variation of the projections of the wave vectors kx and ky and the electron density values ρ. In this example, an elementary cell of the inter-core space is considered, therefore kx and ky vary in the range [–0.4687, 0.4687]. The maximum of the electron density ρ is in the centers of the kx and ky ranges. The minimum values are located at the edges of these ranges [33,34].
Obtaining samples of copper oxide through electrolysis has great performance and a pure for analysis of the output material. Cu2O can be obtained both in crystalline form and in amorphous, in the form of powder. By our method of chemical electrolysis with selected experimental characteristics and certain compositions of substances, several samples of copper oxide were obtained. The results of the purity of the obtained copper oxide samples were checked on an X-ray phase analyzer. The tabulated data obtained after processing by the program show that the ratios of the structural units of the thin copper oxide film obtained in the microelement analysis and the calculated electron density in the reverse space are both approximately equal to 0.5. Obtaining samples of copper oxide through electrolysis has great performance and a pure for analysis of the output material. Cu2O can be obtained both in crystalline form and in amorphous, in the form of powder. By our method of chemical electrolysis with selected experimental characteristics and certain compositions of substances, several samples of copper oxide were obtained. The results of the purity of the obtained copper oxide samples were checked on an X-ray phase analyzer. The tabulated data obtained after processing by the program show that the ratios of the structural units of the thin copper oxide film obtained in the microelement analysis and the calculated electron density in the reverse space are both approximately equal to 0.5.
The authors declare that there is no conflict of interest regarding the publication of this paper.
[1] |
Baumeister PW (1961) Optical absorption of cuprous oxide. Phys Rev 121: 359–362. doi: 10.1103/PhysRev.121.359
![]() |
[2] |
Besenbacher F, Nørskov JK (1993) Oxygen chemisorption on metal surfaces: General trends for Cu, Ni and Ag. Prog Surf Sci 44: 5–66. doi: 10.1016/0079-6816(93)90006-H
![]() |
[3] |
Berge K, Goldmann A (2003) Electronic interchain interactions of the Cu(110)(2 × 1)O surface-an angle-resolved photoemission study. Surf Sci 540: 97–106. doi: 10.1016/S0039-6028(03)00778-7
![]() |
[4] |
Bessekhouad Y, Robert D, Weber JV (2005) Photocatalytic activity of Cu2O/TiO2, Bi2O3/TiO2 and ZnMn2O4/TiO2 heterojunctions. Catal Today 101: 315–321. doi: 10.1016/j.cattod.2005.03.038
![]() |
[5] | Blanchard NP, Martin DS, Weightman P (2005) Molecular adsorbate induced restructuring of a stepped Cu(110) surface. Phys Status Solidi C 12: 4017–4021. |
[6] |
Islam MM, Diawara B, Maurice V, et al. (2009) Bulk and surface properties of Cu2O: A first-principles investigation. J Mol Struc-THEOCHEM 903: 41–48. doi: 10.1016/j.theochem.2009.02.037
![]() |
[7] |
Islam MM, Diawara B, Maurice V, et al. (2009) First principles investigation on the stabilization mechanisms of the polar copper terminated Cu2O(111) surface. Surf Sci 603: 2087–2095. doi: 10.1016/j.susc.2009.04.005
![]() |
[8] | Galeotti M, Cortigiani B, Torrini M, et al. (1996) Epitaxy and structure of the chloride phase formed by reaction of chlorine with Cu(100). A study by X-ray photoelectron diffraction. Surf Sci 349: L164–L168. |
[9] |
Forster M, Raval R, Hodgson A, et al. (2011) c(2 × 2) water-hydroxyl layer on Cu(110): a wetting layer stabilized by Bjerrum defects. Phys Rev Lett 106: 046103. doi: 10.1103/PhysRevLett.106.046103
![]() |
[10] | Ikeda S, Takata T, Kondo T, et al. (1998) Mechano-catalytic overall water splitting. Chem Commun 2185–2186. |
[11] | Bobrov K, Guillemot L (2008) Interplay between adsorbate-induced reconstruction and local strain: Formation of phases on the Cu(110)–(2 × 1):O surface. Phys Rev B 78: 121408(R). |
[12] |
|
[13] |
12. Bohnen KP, Heid R, Pintschovius L, et al. (2009) Ab initio lattice dynamics and thermal expansion of Cu2O. Phys Rev B 80: 304. doi: 10.1103/PhysRevB.80.134304
![]() |
[14] |
13. Fornasini P, Dalba G, Grisenti R, et al. (2006) Local behaviour of negative thermal expansion materials. Nucl Instrum Meth B 246: 180–183. doi: 10.1016/j.nimb.2005.12.062
![]() |
[15] |
14. Hu JP, Payne DJ, Egdell RG, et al. (2008) On-site interband excitations in resonant inelastic X-ray scattering from Cu2O. Phys Rev B 77: 115. doi: 10.1103/PhysRevB.77.155115
![]() |
[16] |
15. Coulman DJ, Wintterlin J, Behm RJ, et al. (1990) Novel mechanism for the formation of chemisorption phases: The (2 × 1)O–Cu(110) "added row" reconstruction. Phys Rev Lett 64: 1761–1764. doi: 10.1103/PhysRevLett.64.1761
![]() |
[17] |
16. Cox DF, Schulz KH (1991) Interaction of CO with Cu+ cations: CO adsorption on Cu2O(100). Surf Sci 249: 138–148. doi: 10.1016/0039-6028(91)90839-K
![]() |
[18] |
17. Harrison MJ, Woodruff DP, Robinson J, et al. (2006) Adsorbate-induced surface reconstruction and surface-stress changes in Cu(100)/O: Experiment and theory. Phys Rev B 74: 165402. doi: 10.1103/PhysRevB.74.165402
![]() |
[19] | 18. Haugsrud R, Kofstad P (7) On the oxygen pressure dependence of high temperature oxidation of copper. Mater Sci Forum 251–254: 65–72. |
[20] |
19. Haugsrud R (2) The influence of water vapor on the oxidation of copper at intermediate temperatures. J Electrochem Soc 149: B14–B21. doi: 10.1149/1.1427076
![]() |
[21] |
20. Haugsrud R, Norby T (1999) Determination of thermodynamics and kinetics of point defects in Cu2O using the Rosenburg method. J Electrochem Soc 146: 999–1004. doi: 10.1149/1.1391712
![]() |
[22] |
21. Ho JH, Vook RW (1978) (111)Cu2O growth modes on (111)Cu surfaces. J Cryst Growth 44: 561–569. doi: 10.1016/0022-0248(78)90299-3
![]() |
[23] |
22. Ito T, Yamaguchi H, Okabe K, et al. (1998) Single-crystal growth and characterization of Cu2O and CuO. J Mater Sci 33: 3555–3566. doi: 10.1023/A:1004690809547
![]() |
[24] |
23. Ivanda M, Waasmaier D, Endriss A, et al. (1997) Low-temperature anomalies of cuprite observed by Raman spectroscopy and X-ray powder diffraction. J Raman Spectrosc 28: 487–493. doi: 10.1002/(SICI)1097-4555(199707)28:7<487::AID-JRS115>3.0.CO;2-V
![]() |
[25] |
24. Brandstetter T, Draxler M, Hohage M, et al. (2008) Oxygen-induced restructuring of Cu(19 19 1) studied by scanning tunneling microscopy. Phys Rev B 78: 075402. doi: 10.1103/PhysRevB.78.075402
![]() |
[26] |
25. Cruickshank BJ, Sneddon DD, Gewirth AA (1993) In situ observations of oxygen adsorption on a Cu(100) substrate using atomic force microscopy. Surf Sci 281: L308–L314. doi: 10.1016/0039-6028(93)90845-B
![]() |
[27] |
26. Dapiaggi M, Tiano W, Artioli G, et al. (2003) The thermal behaviour of cuprite: An XRD–EXAFS combined approach. Nucl Instrum Meth B 200: 231–236. doi: 10.1016/S0168-583X(02)01682-8
![]() |
[28] | 27. Hara M, Kondo T, Komoda M, et al. (1998) Cu2O as a photocatalyst for overall water splitting under visible light irradiation. Chem Commun 357–358. |
[29] |
28. Brattain WH (1951) The copper oxide rectifier. Rev Mod Phys 23: 203–212. doi: 10.1103/RevModPhys.23.203
![]() |
[30] | 29. De Jongh PE, Vanmaekelbergh D, Kelly JJ (1999) Cu2O: a catalyst for the photochemical decomposition of water? Chem Commun 1069–1070. |
[31] |
30. Hodgson A, Haq S (2009) Water adsorption and the wetting of metal surfaces. Surf Sci Rep 64: 381–451. doi: 10.1016/j.surfrep.2009.07.001
![]() |
[32] |
31. Iskakova K, Akhmaltdinov R, Kuketaev T (2018) Formation of (Cu)n & (Cu2O)n nanostructures with the stability of their clusters. AIMS Mater Sci 5: 543–550. doi: 10.3934/matersci.2018.3.543
![]() |
[33] |
32. Iskakova K, Akhmaltdinov R, Aliyev B (2018) Interspheral space and properties of mono- and divalent metals with FCC and BCC structures. J Comput Theor Nanos 15: 1384–1394. doi: 10.1166/jctn.2018.7245
![]() |
[34] |
33. Akhmaltdinov R (2012) Modeling of the crystal structure growth process of GaAs. Appl Phys A-Mater 109: 857–864. doi: 10.1007/s00339-012-7364-x
![]() |
[35] | 34. Akhmaltdinov RF (2012) Modeling and calculation of the algorithm structure of compound semiconductor-type A3B5. Appl Mech Mater 110–116: 2854–2858. |
1. | Kulpash Iskakova, Mohammad Rahimi-Gorji, Plasma Deposition of Gallium Arsenide Nanoclusters on a Silicon Matrix, 2022, 2022, 1687-4129, 1, 10.1155/2022/3767355 |
Copper | Iron | Titanium | Oxygen | Impurities |
62% | 0.5% | 0.2% | 32% | 5.3% |