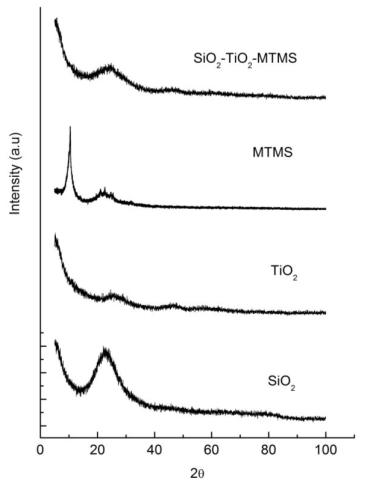
Citation: Alfa Akustia Widati, Nuryono Nuryono, Indriana Kartini. Water-repellent glass coated with SiO2–TiO2–methyltrimethoxysilane through sol–gel coating[J]. AIMS Materials Science, 2019, 6(1): 10-24. doi: 10.3934/matersci.2019.1.10
[1] | Johana Gamez, Luis Reyes-Osorio, Oscar Zapata, Roberto Cabriales, Luis Lopez, Miguel Delgado-Pamanes . Study of protective hard coatings of SiO2-TiO2 on aluminum substrates. AIMS Materials Science, 2024, 11(2): 200-215. doi: 10.3934/matersci.2024011 |
[2] | Nhung Thi-Tuyet Hoang, Anh Thi-Kim Tran, Nguyen Van Suc, The-Vinh Nguyen . Antibacterial activities of gel-derived Ag-TiO2-SiO2 nanomaterials under different light irradiation. AIMS Materials Science, 2016, 3(2): 339-348. doi: 10.3934/matersci.2016.2.339 |
[3] | Akira Nishimura, Ryuki Toyoda, Daichi Tatematsu, Masafumi Hirota, Akira Koshio, Fumio Kokai, Eric Hu . Optimum reductants ratio for CO2 reduction by overlapped Cu/TiO2. AIMS Materials Science, 2019, 6(2): 214-233. doi: 10.3934/matersci.2019.2.214 |
[4] | Julien G. Mahy, Stéphanie D. Lambert, Jérémy Geens, Alain Daniel, David Wicky, Catherine Archambeau, Benoît Heinrichs . Large scale production of photocatalytic TiO2 coating for volatile organic compound (VOC) air remediation. AIMS Materials Science, 2018, 5(5): 945-956. doi: 10.3934/matersci.2018.5.945 |
[5] | Akira Nishimura, Tadaki Inoue, Yoshito Sakakibara, Masafumi Hirota, Akira Koshio, Fumio Kokai, Eric Hu . Optimum molar ratio of H2 and H2O to reduce CO2 using Pd/TiO2. AIMS Materials Science, 2019, 6(4): 464-483. doi: 10.3934/matersci.2019.4.464 |
[6] | Ahmed Ali Farhan Ogaili, Ehsan S. Al-Ameen, Mohammed Salman Kadhim, Muhanad Nazar Mustafa . Evaluation of mechanical and electrical properties of GFRP composite strengthened with hybrid nanomaterial fillers. AIMS Materials Science, 2020, 7(1): 93-102. doi: 10.3934/matersci.2020.1.93 |
[7] | Muhammad Yakob, Hamdani Umar, Puji Wahyuningsih, Rachmad Almi Putra . Characterization of microstructural and optical CoFe2O4/SiO2 ferrite nanocomposite for photodegradation of methylene blue. AIMS Materials Science, 2019, 6(1): 45-51. doi: 10.3934/matersci.2019.1.45 |
[8] | Arbaz Sajjad, Wan Zaripah Wan Bakar, Dasmawati Mohamad, Thirumulu Ponnuraj Kannan . Characterization and enhancement of physico-mechanical properties of glass ionomer cement by incorporating a novel nano zirconia silica hydroxyapatite composite synthesized via sol-gel. AIMS Materials Science, 2019, 6(5): 730-747. doi: 10.3934/matersci.2019.5.730 |
[9] | Amadou Kouyaté, Yapo Hermann Aristide Yapi, Aliou Pohan, Ange Privat Ahoussou, Albert Trokourey . Correlation between bioactivity and thermodynamic stability of glasses of the molar formula 20.15[(2.038 + x)SiO2-(1.457 - x)Na2O]-2.6P2O5-25.73CaO-1.22MgO. AIMS Materials Science, 2020, 7(3): 323-337. doi: 10.3934/matersci.2020.3.323 |
[10] | Ahmed Z. Abdullah, Adawiya J. Haider, Allaa A. Jabbar . Pure TiO2/PSi and TiO2@Ag/PSi structures as controllable sensor for toxic gases. AIMS Materials Science, 2022, 9(4): 522-533. doi: 10.3934/matersci.2022031 |
Hydrophobic glasses are glasses with surfaces that exhibit water roll-off properties. Generally, the hydrophobicity of a glass is influenced by its surface energy and surface roughness [1]. Fluorosilane is the most frequently used low-surface-energy material. Unfortunately, fluorosilane is categorized as a toxic compound, and has been reported to be hazardous to human health and environment [2,3]. Recently, many authors have utilized alkylsilane as a milder alternative to fluorosilane that also has a low surface energy [4,5,6,7]. However, materials coated with alkylsilane exhibited lower water contact angles than those coated with fluorosilane. Coatings prepared using only alkylsilane also resulted in low water contact angles, i.e., low hydrophobicity. The coating of glasses with methyltrimethoxysilane (MTMS) also resulted in a low water contact angle; however, the angle was increased to 171 ± 1° by the addition of polymethylmethacrylate to the MTMS coating [8]. Another attempt to improve the hydrophobicity of a silica aerogel based on MTMS through modification with trimethylchlorosilane (TMCS) and cetyltrimethylammoniumbromide was reported [9].
Increasing the roughness of a surface can also enhance its hydrophobicity. This effect can be explained by the Wenzel and Cassie-Baxter equation. Wenzel stated that a rough surface has an actual surface area larger than its horizontal projection. The substitution of the actual surface area to the Young equation will provide the larger contact angle. Cassie-Baxter assumed that the rough surface is created by air entrapment in the gaps of the rough structure, so that the droplet rests on a layer of air [10,11]. A rough surface can be produced by the deposition of metal oxide on the surface. The agglomeration of the oxide generates some cavities on the surface, producing a rough structure. In previous studies, we have investigated the use of SiO2 nanoparticles, methyltrimethoxysilane, and hexadecyltrimethoxysilane to prepare hydrophobic glasses [12]. The prepared glasses were stable towards ethanol, but unstable at ambient temperature. The use of SiO2 nanoparticles in powder form decreased the transparency of the glasses. The use of a MTMS-modified SiO2 sol to coat soda lime glass resulted in high transparency, but its water contact angle was less than 90° [13].
In addition to SiO2, TiO2 has also been employed to produce rough surfaces [14,15,16]. Interestingly, the combination of TiO2 and hydrophobic agents results in tunable properties, i.e., the ability to switch the hydrophobicity to hydrophilicity under UV illumination [17,18]. The aquapel modified micro-nano TiO2/SiO2 composite films had reversible high-hydrophobic to high-hydrophilic conversion properties [17]. Crystalline TiO2 plays a main role in this behavior. The irradiation of TiO2 is known to produce electrons and holes. The electrons tend to reduce Ti4+ to the Ti3+, and the holes oxidize the O2− on the surface. Oxygen atoms are ejected, generating oxygen vacancies. At this stage, water molecules occupy these vacancies, resulting in adsorbed hydroxyl groups. This behavior created the hydrophilic properties [19]. In other words, to obtain stable hydrophobicity using modified TiO2, the TiO2 structure must be amorphous [20]. The amorphous TiO2 has a much a higher band gap (~310 nm) than crystalline TiO2 (~400 nm), therefore it is promising candidate to performance the UV-shielding ability [21]. The coating of TiO2 with inert material such as SiO2 also inhibits the photocatalytic properties of TiO2. Hybrid SiO2–amorphous TiO2 also exhibited excellent stability under UV-light irradiation. SiO2 imparts an efficient UV scattering because of the large refractive index of SiO2 [22].
The mechanical properties of coatings are an important aspect from an applications point of view. Generally, hydrophobic coatings have low mechanical stability due their high surface roughness. Liu et al. [23] reported the poor scratch resistance of such coatings; the coatings were easy removed using all pencil hardnes (hardest, average hardness, and softest pencil). TiO2 and SiO2 have been introduced into hybrid silica–epoxy coatings to improve the mechanical properties of an aluminum alloy surface. The addition of TiO2 improved the hydrophobic and adhesion properties of the coating. However, it decreased the hardness of the coated surfaces. Meanwhile, SiO2 increased the hydrophobicity and surface hardness, but the coatings exhibited poor adhesion properties [24].
In this work, we proposed the fabrication of hydrophobic glass using MTMS-modified SiO2–TiO2. MTMS contributed to the increased hydrophobicity due to replacement of the polar –OH groups of the surface by the non-polar –CH3 groups of MTMS. SiO2 and TiO2 produced a rough surface and contributed to the hardness of coating. The effect of chemical composition on water contact angle and transparency of the coated glasses was studied. The topography of the surface, the hardness properties and the stability of the hydrophobic glass were also determined.
The sol–gel system consisted tetraethylorthosilicate (TEOS) and titanium tetraisopropoxide (TTIP) as SiO2 and TiO2 precursor, methyltrimethoxysilane (MTMS) as the hydrophobic precursor, HCl as catalyst, and ethanol as solvents. All these chemicals were purchased from Sigma Aldrich, except TEOS was supplied by Merck. All reagents were commercially available in analytical grade. All these materials were used as received without any further purification.
Before the coating process, glass slides were sonicated in ethanol bath for 30 minutes and were dried. The coating solutions were prepared by the sol–gel method. SiO2, TiO2, and MTMS sol were hydrolyzed in separate containers. A 0.030 mol of SiO2 was prepared by stirring a solution consisting 3.35 mL of TEOS, 8 mL of ethanol, 1 mL of water, and 0.2 mL of 0.1 M HCl at 60 ℃ for 90 minutes. After this, the obtained SiO2 sol was added 2 mL of concentrated HCl, followed by adding ethanol to a 20 mL of solution. To clarify the effect of SiO2 on the hydrophobization, various mol SiO2 (0, 0.015, 0.030, 0.045, 0.060, 0.075 and 0.089) were employed.
The procedure for preparation of TiO2 and MTMS sol were similar with early described procedure in the preparation of SiO2 sol. The variation mol of TiO2 (0, 0.015, 0.030 and 0.045) and MTMS (0, 0.001, 0.002, 0.004 and 0.006) were also prepared to study the effect on the hydrophobization. While the mol of SiO2 was varied, we kept constant the mol of TiO2 and MTMS were 0.030 and 0.002 mole, respectively. Furthermore, the optimum mol of SiO2 and constant mol of MTMS was handled to study the effect of TiO2 amount. After that, the variation of MTMS mol was investigated using optimum mol of SiO2 and TiO2.
The deposition of SiO2, TiO2, and MTMS on glass substrates were conducted by layer by layer dip coating technique. The bottom, middle, and top layer were SiO2, TiO2, and MTMS, respectively. The glasses were immersed into the coating solution with withdrawal speed of 3 cm/min. After each coating, the films were first annealed at room temperature (33 ℃) for 10 minutes and then followed at 70° for 30 minutes.
Shimadzu UV 1800 was used to determine the transparency of coated glass. The transmissions were measured in the wavelength range 300–800 nm. Vickers microhardness Mitutoyo HM 211 was employed to measure the mechanical durability. The average of five hardness test was found for each of coated glass. FTIR spectra was recorded on Shimadzu IRTracer-100. Spectrum was recorded in the wavenumber range 4000–400 cm−1. The structure of sample performed on the XRD Pan Analytical using Cu Kα radiation of wavelength 0.15418 nm. The diffraction data were recorded between 5–100° with 2 minutes scan speed. The surface roughness of coated glass was observed AFM N8 Neos Bruker in the non-contact mode under ambient conditions. Water contact angles were determined by capturing the images of a 40 μL water droplet on coated glasses. The images were then processed using ImageJ software. The morphology and composition of surface was analyzed using SEM-EDX Carl Zeiss EVO MA 10.
The coating solutions were formed by the hydrolysis and condensation reactions of MTMS, TEOS, and TTIP. The sol–gel process of TEOS, TTIP, and MTMS was conducted using ethanol, water, and HCl. Hydrolysis occurred when TEOS was dissolved in a water–ethanol mixture. This process resulted in the formation of silanol (Si–OH) groups. The condensation of the silanol groups formed siloxane bridges (–Si–O–Si–), while the hydrolysis of TTIP produced the tetravalent cation titanol, (Ti(OH)4). Oxolation and olation processes also occurred during nucleation and growth, generating amorphous TiO2 [25]. In this research, of amorphous TiO2 was prepared to reduce the photocatalytic properties of TiO2 in order to maintain the hydrophobic properties of the coated glasses.
Simultaneously, MTMS was polymerized in a water–ethanol mixture under acidic conditions. Water was an important solvent in the hydrolysis process of MTMS. Although the process is catalyzed by HCl, the methoxy groups of MTMS cannot completely hydrolyze unless water is present in the sol–gel system [9]. The three methoxy groups of the MTMS monomers underwent hydrolysis and condensation reactions, and the monomers condensed to form dimers. After 1 hour, condensation of the dimers resulted in tetramers, which then transformed into a 4-membered ring [26].
The coating process was conducted by immersing the glass substrate into each of the sols, followed by annealing at 70 ℃. During the annealing process, the hydroxyl groups condensed, forming Si–O–Si and Si–O–Ti networks.
In order to investigate the phases of SiO2, TiO2, MTMS and SiO2–TiO2–MTMS, the sol was heated at 70 ℃, ground into a powder, and then used in XRD and FTIR analysis. This temperature was similar to the annealing temperature of the coated glass. Heating the sol at 70 ℃ did not change the crystallinity of the sample, so we used this temperature to prepare the powder form of the samples. The corresponding XRD spectra of the samples are depicted in Figure 1.
The XRD patterns reveal that none of the samples exhibited sharp peaks. In the diffractogram of SiO2, the hump at 2θ 15–35° indicates that the sample was amorphous silica. The XRD pattern of TiO2 also indicated that our sample had an amorphous structure. The amorphous structure of TiO2 was important to obtain the hydrophobic properties in our coated glasses, because amorphous TiO2 does not exhibit the strong photocatalytic properties associated with crystalline TiO2 [20]. As discussed earlier, photocatalysis produces hydrophilic OH groups. Hence, crystalline TiO2 is not a suitable structure to prepare hydrophobic materials. The MTMS pattern displayed a diffraction peak at 10° and a broad peak at 14–30°. The first peak was related to the presence of silicon atoms attached to alkyl groups, while the second peak was associated with Si–O–Si in amorphous silica [27]. Therefore, it was not surprising that the diffractogram of SiO2–TiO2–MTMS showed the amorphous phase.
The FTIR spectra of SiO2, TiO2, MTMS, and SiO2–TiO2–MTMS are shown in Figure 2. The details of the SiO2, TiO2, MTMS, and SiO2–TiO2–MTMS spectra are presented in Table 1. FTIR analysis was conducted to study the appearance of the SiO2, TiO2, and MTMS peaks in the SiO2–TiO2–MTMS sample.
Wavenumber (cm−1) | Sample | Assignment | |||
SiO2 | TiO2 | MTMS | SiO2–TiO2–MTMS | ||
3200–3500 | √ | √ | √ | √ | O–H stretching [28,29] |
2970 | √ | Antisymmetric stretching C–H bond in CH3 [30] | |||
2910 | √ | Symmetric stretching C–H bond [3] | |||
1620–1630 | √ | √ | √ | √ | O–H vibration of H2O [31] |
1400–1440 | √ | √ | Antisymmetric bending C–H bond in CH3 [30] | ||
1270 | √ | √ | Symmetric bending C–H bond in CH3 [31] | ||
1070–1160 | √ | √ | √ | Antisymmetric stretching of Si–O–C Symmetric stretching of Si–O–Si in cyclic structures [28] | |
930–960 | √ | √ | Si–O non bridging free broken [27] Vibration of Si–O–Ti [28] | ||
773–775 | √ | √ | Stretching of C–H [32,33] | ||
630 | √ | √ | Ti–O–Ti vibration [34,35] |
All the spectra displayed a broad band in the spectral region 3200–3500 cm−1, which was related to O–H stretching from silanol, titanol, absorbed water, and ethanol. The peak at 1620–1630 cm−1 corresponds to the O–H vibration of H2O. The spectra of SiO2–TiO2–MTMS showed characteristic peaks at 1438, 1271, 1120,931, and 775 cm−1. The peak at 1438 cm−1 was assigned to antisymmetric bending of the C–H bond of the CH3 of MTMS. The peak at 1271 cm−1 was the symmetric bending of the C–H bond in CH3 from MTMS. The peak at 1120 cm−1 corresponded to overlapping peaks of the antisymmetric stretching of Si–O–C and symmetric stretching of Si–O–Si in cyclic structures. The antisymmetric stretching of Si–O–C originated from the MTMS molecules. Meanwhile, the symmetric stretching of Si–O–Si in cyclic structures was attributed to the polymerization of SiO2 and MTMS, respectively. The peak at 931 cm−1 was related to non-bridging Si–O that had broken free from SiO2 and MTMS. Evidence for Si–O–Ti linkages between SiO2 and TiO2 was also obtained from this peak, i.e., in the range between 900–960 cm−1 [28]. The peak at about 775 cm−1 indicated the C–H stretch of MTMS. The peak at 630 cm−1 was confirmed to be the Ti–O–Ti vibration of TiO2.
The wettability of the coated glass was studied using various moles of the coating solutions. The changes in the contact angles and shapes of water droplets for different amount of SiO2, TiO2, and MTMS are shown in Figures 3 and 4. Figure 3a depicts the effect of the mol of SiO2 and TiO2 on the contact angle of the glass substrates. The water contact angle on the coated glasses increased when the mol of SiO2 was raised, reaching 110.14 ± 1.01° at 0.075 mol SiO2. This increase in water contact angle may be attributed to the increase in surface roughness from the network formation of silica particles.
In contrast, the contact angle decreased to 90.34 ± 0.85° when 0.089 mol SiO2 was used in the glass coatings. The abundance of hydroxyl groups on the surface caused an increase in its hydrophilicity. This tendency can be attributed to higher interaction of polar hydroxyl group with water molecule [36]. It was also assumed that the roughness tend to be smooth because the homogeneity of SiO2 coating on glass surfaces.
The influence of the mol of TiO2 on hydrophobicity and transparency can be seen in Figure 3b. Coating glasses with sols containing 0 to 0.030 mol of TiO2 caused increased hydrophobicity. This result was unsurprising, as TiO2, like SiO2, is used to enhance the surface roughness; therefore the effect of TiO2 was similar to that of SiO2. Based on the results, 0.075 mol of SiO2 and 0.030 mol of TiO2 were used in further studies.
Figure 3c shows the contact angles when different amount of MTMS were used in the glass coating. The hydrophobicity decreased with increasing amounts of MTMS, reaching 115.56 ± 1.01° when 0.002 mol of MTMS was used. This might be due to the replacement the polar OH groups by the non-polar alkyl groups of MTMS which has low surface energy. Furthermore, the water contact angle decreased when more than 0.002 mol of MTMS was used. In high concentration of silane, the surface did not show any hierarchical morphology. The surfaces with rather smooth with holes, therefore the water contact angle were decreased [37].
Figure 5 shows the optical transmission of the coated glasses prepared using various moles of SiO2, TiO2, and MTMS. The transparency of the bare glass decreased when the coating solution was deposited on the surface. Increasing the mol of SiO2, TiO2, or MTMS in the coating solution resulted in decreased transparency of the coated glass. Higher moles of these components in the coating solutions promoted the formation of thicker films. A previous study also reported that the preparation of transparent glass with high surface roughness was difficult, because the higher-roughness coatings were thicker and led to extensive light scattering and loss of transparency [38].
SiO2 and TiO2 played a crucial role in the wettability of the surfaces. The water contact angle measured for the SiO2–MTMS coated glass was 78.46 ± 1.53°. The contact angle increased when TiO2 was deposited on the surface. The SiO2–TiO2–MTMS coated glass exhibited a water contact angle about 115.56 ± 1.01°, because the use of a combination of SiO2 and TiO2 in the coating produced a rougher surface than when only SiO2 was used. This was confirmed by the AFM analysis results, as can be seen in Figure 6. The surface topography revealed that the rms of bare glass, and of glass coated using SiO2–MTMS, TiO2–MTMS, and SiO2–TiO2–MTMS were 4.10, 95.6, 76.3, and 106.0 nm, respectively.
Table 2 tabulated the height surface of coated glass based on two-dimensions of AFM images. It can be seen that the surface height of SiO2–MTMS, TiO2–MTMS, and SiO2–TiO2–MTMS were 70–200, 10–90, and 20–180 nm, respectively. This data showed that the surface height of SiO2–TiO2–MTMS was resulted from a combination of height surface between SiO2–MTMS and TiO2–MTMS. The topography and high surface roughness of SiO2–TiO2–MTMS was resulted from the agammaegation of SiO2 and TiO2 particles. In other word, SiO2 and TiO2 had a constructive effect on the surface roughness.
Sample | Height of surface (nm) | rms (nm) |
Bare glass | 0.5–12 | 4.10 |
SiO2–MTMS | 70–200 | 95.6 |
TiO2–MTMS | 10–190 | 76.3 |
SiO2–TiO2–MTMS | 20–180 | 106.0 |
SiO2–TiO2–MTMS performed rougher surface, higher water repellence, and Cassie-Baxter behavior. In the Cassie-Baxter model, air is trapped in the cavities, and the water droplets rest on top of the rough surfaces. The results of the SEM-EDX measurements also demonstrated that the SiO2–TiO2–MTMS coated glass had a rough surface with a coating thickness about 18 μm. This information is presented in Figure S1 of the supplementary material.
The surface roughness also influenced the transparency of glasses. The ability of glass to transmit light waves is called transparency. Rough surfaces have tendency to absorb and transmit the light. Therefore, the transmission process on the rough surface was lower than smooth surfaces. It caused the rough surfaces presented low transparency. Figure 7 displays the correlation between surface roughness and transparency.
The mechanical properties of the hydrophobic glasses were evaluated using the Vickers hardness test, which measures the hardness as a function of the penetration depth of an indentor. The thicker the coating is, the deeper the penetration of the indentor, and the higher the hardness is considered to be [39]. The hardness properties of bare glass and glass coated with TiO2–MTMS and SiO2–TiO2–MTMS were 552.76,612.32, and 615.1 kgf/mm2, respectively. The higher value for coated glass than bare glass might due to its higher thickness. Lakshmi et al. reported that the formation of a siloxane network enhanced the thickness of a coating [38]. Increasing the hardness of coated glasses decreased their transparency, as shown in Figure 7.
The stability of coatings is a very important property from an applications point of view. Here, we measured the changes in the water contact angle as a function the long-term outdoor exposure time, as shown in Figure 8. The water contact angle decreased slightly to 89.37° after 4 weeks. One reason for this behavior was the accumulation of dirt. The presence of the silica coating on the surface produced hydroxyl groups, and also adsorbed moisture from the atmosphere, decreasing the water contact angle [8]. Li et al. also reported that coatings fabricated using the sol–gel technique exhibited poor moisture resistance because the existence of hydroxyl groups [40].
Hydrophobic glasses were successfully synthesized from SiO2, TiO2, and MTMS precursors. The addition of TiO2 to SiO2–methyltrimethoxysilane coated glass increased the surface roughness and improved the hydrophobicity. Higher moles of SiO2, TiO2, and MTMS produced higher hydrophobicity, but lowered the transparency of the coated glasses. The hydrophobic glasses exhibited good hardness. A coating with 0.075 mol of SiO2, 0.030 mol of TiO2, and 0.002 mol of MTMS was found to have high hydrophobicity, rough surface and good mechanical properties. Further research into maintaining high transparency in the glasses through controlling the surface roughness can be proposed.
The authors are grateful for financial support from Ministry or Research, Technology and Higher Education, Indonesia through PDD Grant of Universitas Airlangga No. 122/SP2H/PTNBH/DRPM/2018. We also thank to the Department of Chemistry, Faculty of Mathematics and Natural Sciences, Universitas Gadjah Mada and Department of Chemistry, Faculty of Science and Technology, Universitas Airlangga for the use of laboratory facilities.
There is no conflict to declare.
[1] |
Song J, Rojas OJ (2013) Approaching super-hydrophobicity from cellulosic materials: A review. Nord Pulp Pap Res J 28: 216–238. doi: 10.3183/NPPRJ-2013-28-02-p216-238
![]() |
[2] | Nørgaard AW, Hansen JS, Sørli JB, et al. (2013) Pulmonary toxicity of perfluorinated silane-based nanofilm spray products: solvent dependency. Toxicol Sci 137: 179–188. |
[3] |
Pan G, Zhou Q, Luan X, et al. (2014) Distribution of perfluorinated compounds in Lake Taihu (China): Impact to human health and water standards. Sci Total Environ 487: 778–784. doi: 10.1016/j.scitotenv.2013.11.100
![]() |
[4] |
Wang H, Ding J, Lin T, et al. (2010) Super water repellent fabrics produced by silica nanoparticle-containing coating. Res J Text Apparel 14: 30–37. doi: 10.1108/RJTA-14-02-2010-B004
![]() |
[5] |
Rostamzadeh P, Mirabedini SM, Esfandeh M (2014) APS-silane modification of silica nanoparticles effect of treatment's variables on the grafting content and colloidal stability of the nanoparticles. J Coat Technol Res 11: 651–660. doi: 10.1007/s11998-014-9577-8
![]() |
[6] | Spataru CI, Purcar V, Donescu D, et al. (2013) Preparation of hydrophobic surface based on hybrid silica films by sol–gel process. UPB Sci Bull B 75: 117. |
[7] | Wang X, Chai Y, Liu J (2013) Formation of highly hydrophobic wood surfaces using silica nanoparticles modified with long-chain alkylsilane. Holzforschung 67: 667–672. |
[8] |
Kavale MS, Mahadik DB, Parale VG, et al. (2011) Optically transparent, superhydrophobic methyltrimethoxysilane based silica coatings without silylating reagent. Appl Surf Sci 258: 158–162. doi: 10.1016/j.apsusc.2011.08.023
![]() |
[9] |
He S, Chen X (2017) Flexible silica aerogel based on methyltrimethoxysilane with improved mechanical property. J Non-Cryst Solids 463: 6–11. doi: 10.1016/j.jnoncrysol.2017.02.014
![]() |
[10] |
Mohamed AM, Abdullah AM, Younan NA (2015) Corrosion behaviour of superhydrophobic surfaces: A review. Arab J Chem 8: 749–765. doi: 10.1016/j.arabjc.2014.03.006
![]() |
[11] |
Brassard JD, Sarkar DK, Perron J (2012) Fluorine based superhydrophobic coatings. Appl Sci 2: 453–464. doi: 10.3390/app2020453
![]() |
[12] | Widati AA, Nuryono N, Kartini I, et al. (2017) Silica-methyltrimethoxysilane based hydrophobic coatings on glass substrate. J Chem Technol Metall 52: 1123–1128. |
[13] | Philipavičius J, Kazadojev I, Beganskienė A, et al. (2008) Hydrophobic antireflective silica coatings via sol–gel process. Mater Sci 14: 283–287. |
[14] |
Paz Y (2011) Self-assembled monolayers and titanium dioxide: From surface patterning to potential applications. Beilstein J Nanotech 2: 845–861. doi: 10.3762/bjnano.2.94
![]() |
[15] |
Kartini I, Santosa SJ, Febriyanti E, et al. (2014) Hybrid assembly of nanosol titania and dodecylamine for superhydrophobic self-cleaning glass. J Nanopart Res 16: 2514. doi: 10.1007/s11051-014-2514-z
![]() |
[16] |
Shi YL, Feng XJ, Yang W, et al. (2011) Preparation of super-hydrophobic titanium oxide film by sol–gel on substrate of common filter paper. J Sol-Gel Sci Techn 59: 43–47. doi: 10.1007/s10971-011-2459-y
![]() |
[17] |
Li W, Guo T, Meng T, et al. (2013) Enhanced reversible wettability conversion of micro-nano hierarchical TiO2/SiO2 composite films under UV irradiation. Appl Surf Sci 283: 12–18. doi: 10.1016/j.apsusc.2013.05.085
![]() |
[18] |
Liu K, Cao M, Fujishima A, et al. (2014) Bio-inspired titanium dioxide materials with special wettability and their applications. Chem Rev 114: 10044–10094. doi: 10.1021/cr4006796
![]() |
[19] |
Wu KR, Wang JJ, Liu WC, et al. (2006) Deposition of graded TiO2 films featured both hydrophobic and photo-induced hydrophilic properties. Appl Surf Sci 252: 5829–5838. doi: 10.1016/j.apsusc.2005.08.016
![]() |
[20] |
Huang J, Lin Y, Lu L, et al. (2012) The photocatalytic properties of amorphous TiO2 composite films deposited by magnetron sputtering. Res Chem Intermediat 38: 487–498. doi: 10.1007/s11164-011-0365-0
![]() |
[21] | Gautam A, Kshirsagar AS, Banerjee S, et al. (2016) UVC-shielding by nano-TiO2/PMMA composite: A chemical approach. J Mater Sci Nanotechnol 4: 304. |
[22] |
Bai Y, Li Z, Cheng B, et al. (2017) Higher UV-shielding ability and lower photocatalytic activity of TiO2@SiO2/APTES and its excellent performance in enhancing the photostability of poly(p-phenylene sulfide). RSC Adv 7: 21758–21767. doi: 10.1039/C6RA28098F
![]() |
[23] |
Liu S, Liu X, Latthe SS, et al. (2015) Self cleaning transparent superhydrophobic coatings through simple sol–gel processing of fluoroalkylsilane. Appl Surf Sci 351: 897–903. doi: 10.1016/j.apsusc.2015.06.016
![]() |
[24] |
Gobara M (2015) Effects of TiO2/SiO2 reinforced nanoparticles on the mechanical properties of green hybrid coatings. Int Lett Chem Phys Astron 47: 56–66. doi: 10.18052/www.scipress.com/ILCPA.47.56
![]() |
[25] | Nadzirah S, Foo KL, Hashim U (2015) Moprhological reaction on the different stabilizers of titanium dioxide nanoparticles. Int J Electrochem Sc 10: 5498–5512. |
[26] |
Dong H, Lee M, Thomas RD, et al. (2003) Methyltrimethoxysilane sol–gel polymerization in acidic ethanol solutions studied by 29Si NMR spectroscopy. J Sol-Gel Sci Techn 28: 5–14. doi: 10.1023/A:1025690300105
![]() |
[27] |
Lana SLB, Seddon AB (1998) X-Ray diffraction studies of sol–gel derived ORMOSILs based on combinations of tetramethoxysilane and trimethoxysilane. J Sol-Gel Sci Techn 13: 461–466. doi: 10.1023/A:1008685614559
![]() |
[28] | Hakki A, Yang L, Wang F, et al. (2017) The effect of interfacial chemical bonding in TiO2-SiO2 composites on their photocatalytic NOx abatement performance. J Vis Exp 125: 56070. |
[29] |
Wang Y, Li B, Liu T, et al. (2014) Controllable fabrication of superhydrophobic TiO2 coating with improved transparency and thermostability. Colloid Surface A 441: 298–305. doi: 10.1016/j.colsurfa.2013.09.023
![]() |
[30] |
Rubio F, Rubio J, Oteo JL (1998) A FT-IR study of the hydrolysis of tetraethylorthosilicate (TEOS). Spectrosc Lett 31: 199–219. doi: 10.1080/00387019808006772
![]() |
[31] |
Tang Z, Hess DW, Breedveld V (2015) Fabrication of oleophobic paper with tunable hydrophilicity by treatment with non-fluorinated chemicals. J Mater Chem A 3: 14651–14660. doi: 10.1039/C5TA03520A
![]() |
[32] | Socrates G (2001) Infrared and Raman Characteristic Group Frequencies, Hoboken, USA: John Wiley & Sons Inc. |
[33] | Bahşi ZB, Büyükaksoy A, Ölmezcan SM, et al. (2009) A novel label-free optical biosensor using synthetic oligonucleotides from E. coli O157:H7: elementary sensitivity tests. Sensors 9: 4890–4900. |
[34] |
Kunst SR, Beltrami LVR, Cardoso HRP, et al. (2015) Characterization of siloxane-poly(methyl methacrylate) hybrid films obtained on a tinplate substrate modified by the addition of organic and inorganic acids. Mat Res 18: 151–163. doi: 10.1590/1516-1439.299514
![]() |
[35] | Vasconcelos DCL, Costa VC, Nunes EHM, et al. (2011) Infrared spectroscopy of titania sol–gel coatings on 316L stainless steel. Mater Sci Appl 2: 1375–1382. |
[36] |
Boudot M, Ceratti DR, Faustini M, et al. (2014) Alcohol-assisted water condensation and stabilization into hydrophobic mesoporosity. J Phys Chem C 118: 23907–23917. doi: 10.1021/jp508372d
![]() |
[37] |
Latthe SS, Demirel AL (2013) Polystirene/octadecyltrichlorosilane superhydrophobic coatings with hierarchical morphology. Polym Chem 4: 246–249. doi: 10.1039/C2PY20731A
![]() |
[38] |
Lakshmi RV, Bera P, Anandan C, et al. (2014) Effect of the size of silica nanoparticles on wettability and surface chemistry of sol–gel superhydrophobic and oleophobic nanocomposite coatings. Appl Surf Sci 320: 780–786. doi: 10.1016/j.apsusc.2014.09.150
![]() |
[39] |
Mammer F, Le Bourhis E, Rozes L, et al. (2005) Mechanical properties of hybrid organic–inorganic materials. J Mater Chem 15: 3787–3811. doi: 10.1039/b507309j
![]() |
[40] |
Li X, He J, Liu W (2013) Broadband anti-reflective and water repellent coatings on glass substrates for self-cleaning photovoltaic cells. Mater Res Bull 48: 2522–2528. doi: 10.1016/j.materresbull.2013.03.017
![]() |
1. | Mohammad Ali Mousavi, Hamid Reza Mortaheb, Mahsa Baghban Salehi, Hydrophobizing polyether sulfone membrane by sol-gel for water desalination using air gap membrane distillation, 2021, 60, 2574-0881, 47, 10.1080/25740881.2020.1784225 | |
2. | Nurul Pratiwi, Syukri Arief, Diana Vanda Wellia, A Facile Preparation of Transparent Ultrahydrophobic Glass via TiO 2 /Octadecyltrichlorosilane (ODTS) Coatings for Self‐Cleaning Material , 2020, 5, 2365-6549, 1450, 10.1002/slct.201904153 | |
3. | Yujing Liu, Xiao Han, Balati Kuerbanjiang, Vlado K. Lazarov, Lidija Šiller, Effect of sodium bicarbonate solution on methyltrimethoxysilane-derived silica aerogels dried at ambient pressure, 2021, 2095-0179, 10.1007/s11705-020-2028-4 | |
4. | Alfa Akustia Widati, Nuryono Nuryono, Indriana Kartini, Design of SiO2/TiO2 that Synergistically Increases The Hydrophobicity of Methyltrimethoxysilane Coated Glass, 2019, 17, 2391-5420, 798, 10.1515/chem-2019-0087 | |
5. | M. Janocha, E. Tsotsas, Coating layer formation from deposited droplets: A comparison of nanofluid, microfluid and solution, 2022, 399, 00325910, 117202, 10.1016/j.powtec.2022.117202 | |
6. | M. Jacobs, Y. De Vos, V. Middelkoop, Thickness controlled SiO2/TiO2 sol-gel coating by spraying, 2021, 6, 26665395, 100121, 10.1016/j.oceram.2021.100121 | |
7. | Bruno Ramos, Arthur O. G. Silva, Antonio C. S. C. Teixeira, Immobilization of ZnO nanoparticles onto glass spheres: effects of annealing temperature, zinc oxide concentration, and number of coating rounds on the photocatalytic activity under visible light, 2022, 39, 0104-6632, 403, 10.1007/s43153-021-00160-z | |
8. | Vinod Kumar Mahto, Arvind Kumar Singh, Anup Malik, Surface modification techniques of magnesium-based alloys for implant applications, 2022, 1547-0091, 10.1007/s11998-022-00716-9 | |
9. | Tomoomi SEGAWA, Koichi KAWAGUCHI, Katsunori ISHII, Masahiro SUZUKI, Joji TACHIHARA, Kiyoto TAKATO, Takatoshi OKITA, Hiroshi SATONE, Michitaka SUZUKI, Preventing nuclear fuel material adhesion on glove box components using nanoparticle coating, 2021, 8, 2187-9745, 21-00022, 10.1299/mej.21-00022 | |
10. | Sukanta Pal, Sourav Mondal, Prasanta Pal, Ajit Das, Subhamay Pramanik, Jayanta Maity, Fabrication of durable, fluorine-free superhydrophobic cotton fabric for efficient self-cleaning and heavy/light oil-water separation, 2021, 44, 22150382, 100469, 10.1016/j.colcom.2021.100469 | |
11. | Heijun Jeong, Eiyong Park, Sungjoon Lim, Three-dimensional printed and fluidic dielectric material optically transparent metasurface for switchable absorption and reflection functionality in microwave frequency region, 2022, 1745-5030, 1, 10.1080/17455030.2022.2058712 | |
12. | Nikolaos D. Papadopoulos, Pinelopi P. Falara, Polyxeni Vourna, A versatile approach towards development of easy-to-clean transparent nanocoating systems with pronounced anti-static properties for various substrates, 2023, 10, 2372-0484, 139, 10.3934/matersci.2023008 | |
13. | Johana Gamez, Luis Reyes-Osorio, Oscar Zapata, Roberto Cabriales, Luis Lopez, Miguel Delgado-Pamanes, Study of protective hard coatings of SiO2-TiO2 on aluminum substrates, 2024, 11, 2372-0484, 200, 10.3934/matersci.2024011 | |
14. | Johnson Nune Naat, Suyanta Suyanta, Nuryono Nuryono, Hydrophobic modification of naturally magnetic silica with methyltrimethoxysilane for enhanced adsorption of chloramphenicol and ciprofloxacin, 2024, 10, 26660164, 100878, 10.1016/j.cscee.2024.100878 | |
15. | Alicia Rosales, Hugo Mandujano, José Antonio Cervantes-Chávez, Karen Esquivel, Antimicrobial Hydrophobic SiO2-TiO2-PDMS Films: Effect of Indirect Ultrasonic Irradiation on the Synthesis Process, 2024, 8, 2504-477X, 104, 10.3390/jcs8030104 | |
16. | T. N. Mogila, V. M. Mikhal’chuk, R. I. Lyga, V. A. Glazunova, Effect of Silica Nanofiller on Thermal-Oxidative Degradation of Epoxy Composites Synthesized by the Sol-Gel Method, 2023, 96, 1070-4272, 484, 10.1134/S1070427223040122 | |
17. | K. Chandru, R. Elansezhian, 2023, Chapter 8, 978-981-99-1615-3, 77, 10.1007/978-981-99-1616-0_8 | |
18. | Girma Masresha, S. Anuradha Jabasingh, Shimelis Kebede, David Doo‐Arhin, Mekdim Assefa, A review of prospects and challenges of photocatalytic decomposition of volatile organic compounds (VOCs) under humid environment, 2023, 101, 0008-4034, 6905, 10.1002/cjce.24978 | |
19. | Ceren Özcan Diker, Osman Duman, Sibel Tunç, Fabrication and characterization of superhydrophobic halloysite nanotube-based colored hybrid coatings with thermal, chemical, and environmental durability and self-cleaning ability on glass substrate by spray coating technique, 2023, 244, 01691317, 107109, 10.1016/j.clay.2023.107109 | |
20. | T. N. Mogila, V. M. Mikhal'chuk, R. I. Lyga, V. A. Glazunova, Effect of Silica Nanofiller on Thermal-Oxidative Degradation of Epoxy Composites Synthesized by the Sol-Gel Method, 2023, 0044-4618, 413, 10.31857/S0044461823040126 | |
21. | Mustapha Boutamart, Salah Rafqah, Amine Hadri, Khalid Nouneh, Salma Zaidi, Hicham Bichara, Samir Briche, Design of fluorine-free superhydrophobic coating for fibred architectonic concrete, 2024, 425, 09500618, 136086, 10.1016/j.conbuildmat.2024.136086 | |
22. | Nikolaos D. Papadopoulos, Polyxeni Vourna, Kypros Milidonis, Andreas Eliades, Polycarpos Falaras, Fostering wider application of anti-soiling strategies in existing solar power plants: A comparative study of novel quaternarized silica hybrids with commercial self-cleaning coatings, 2024, 315, 02540584, 129046, 10.1016/j.matchemphys.2024.129046 |
Wavenumber (cm−1) | Sample | Assignment | |||
SiO2 | TiO2 | MTMS | SiO2–TiO2–MTMS | ||
3200–3500 | √ | √ | √ | √ | O–H stretching [28,29] |
2970 | √ | Antisymmetric stretching C–H bond in CH3 [30] | |||
2910 | √ | Symmetric stretching C–H bond [3] | |||
1620–1630 | √ | √ | √ | √ | O–H vibration of H2O [31] |
1400–1440 | √ | √ | Antisymmetric bending C–H bond in CH3 [30] | ||
1270 | √ | √ | Symmetric bending C–H bond in CH3 [31] | ||
1070–1160 | √ | √ | √ | Antisymmetric stretching of Si–O–C Symmetric stretching of Si–O–Si in cyclic structures [28] | |
930–960 | √ | √ | Si–O non bridging free broken [27] Vibration of Si–O–Ti [28] | ||
773–775 | √ | √ | Stretching of C–H [32,33] | ||
630 | √ | √ | Ti–O–Ti vibration [34,35] |
Sample | Height of surface (nm) | rms (nm) |
Bare glass | 0.5–12 | 4.10 |
SiO2–MTMS | 70–200 | 95.6 |
TiO2–MTMS | 10–190 | 76.3 |
SiO2–TiO2–MTMS | 20–180 | 106.0 |
Wavenumber (cm−1) | Sample | Assignment | |||
SiO2 | TiO2 | MTMS | SiO2–TiO2–MTMS | ||
3200–3500 | √ | √ | √ | √ | O–H stretching [28,29] |
2970 | √ | Antisymmetric stretching C–H bond in CH3 [30] | |||
2910 | √ | Symmetric stretching C–H bond [3] | |||
1620–1630 | √ | √ | √ | √ | O–H vibration of H2O [31] |
1400–1440 | √ | √ | Antisymmetric bending C–H bond in CH3 [30] | ||
1270 | √ | √ | Symmetric bending C–H bond in CH3 [31] | ||
1070–1160 | √ | √ | √ | Antisymmetric stretching of Si–O–C Symmetric stretching of Si–O–Si in cyclic structures [28] | |
930–960 | √ | √ | Si–O non bridging free broken [27] Vibration of Si–O–Ti [28] | ||
773–775 | √ | √ | Stretching of C–H [32,33] | ||
630 | √ | √ | Ti–O–Ti vibration [34,35] |
Sample | Height of surface (nm) | rms (nm) |
Bare glass | 0.5–12 | 4.10 |
SiO2–MTMS | 70–200 | 95.6 |
TiO2–MTMS | 10–190 | 76.3 |
SiO2–TiO2–MTMS | 20–180 | 106.0 |