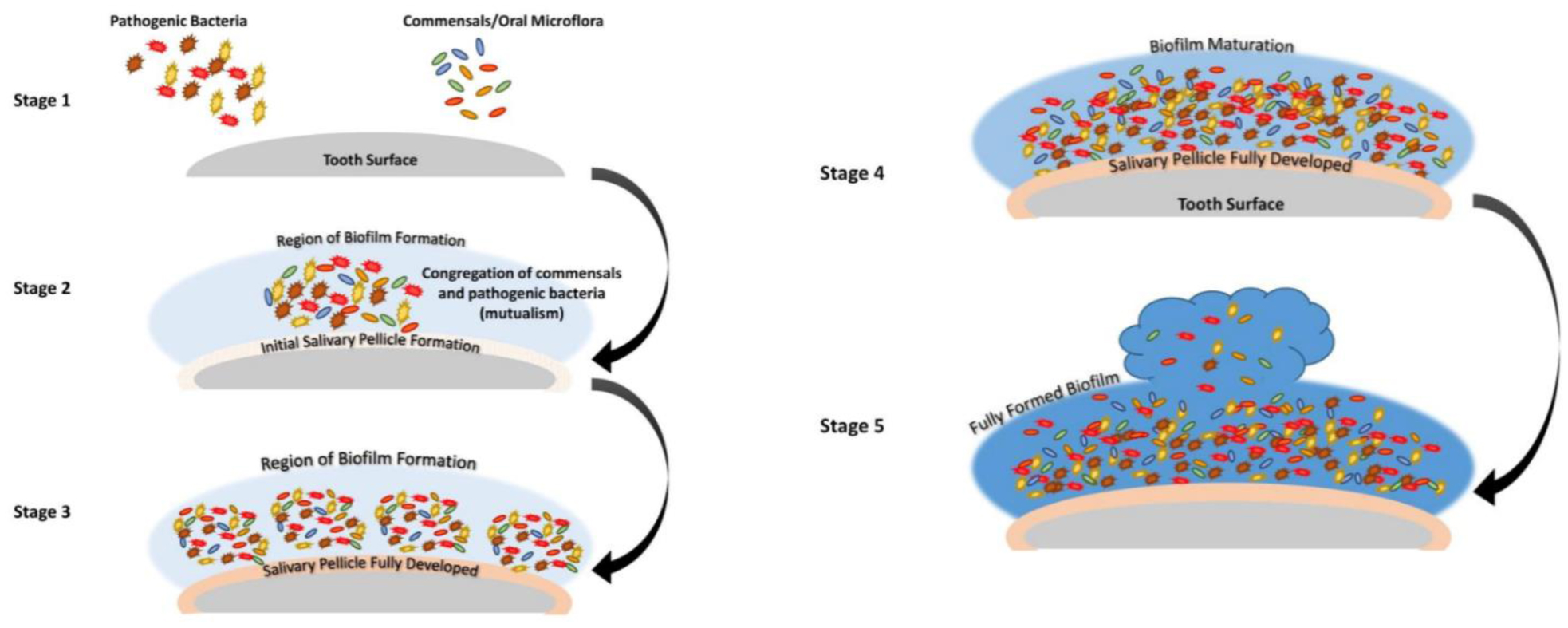
The oral microbiome represents an essential component of the oral ecosystem whose symbiotic relationship contributes to health maintenance. The biofilm represents a state of living of microorganisms surrounding themselves with a complex and tridimensional organized polymeric support and defense matrix. The substrates where the oral biofilm adhere can suffer from damages due to the microbial community metabolisms. Therefore, microbial biofilm represents the main etiological factor of the two pathologies of dental interest with the highest incidence, such as carious pathology and periodontal pathology. The study, analysis, and understanding of the characteristics of the biofilm, starting from the macroscopic structure up to the microscopic architecture, appear essential. This review examined the morphological methods used through the years to identify species, adhesion mechanisms that contribute to biofilm formation and stability, and how the action of microbicidal molecules is effective against pathological biofilm. Microscopy is the primary technique for the morphological characterization of biofilm. Light microscopy, which includes the stereomicroscope and confocal laser microscopy (CLSM), allows the visualization of microbial communities in their natural state, providing valuable information on the spatial arrangement of different microorganisms within the biofilm and revealing microbial diversity in the biofilm matrix. The stereomicroscope provides a three-dimensional view of the sample, allowing detailed observation of the structure, thickness, morphology, and distribution of the various species in the biofilm while CLSM provides information on its three-dimensional architecture, microbial composition, and dynamic development. Electron microscopy, scanning (SEM) or transmission (TEM), allows the high-resolution investigation of the architecture of the biofilm, analyzing the bacterial population, the extracellular polymeric matrix (EPS), and the mechanisms of the physical and chemical forces that contribute to the adhesion of the biofilm to the substrates, on a nanometric scale. More advanced microscopic methodologies, such as scanning transmission electron microscopy (STEM), high-resolution transmission electron microscopy (HR-TEM), and correlative microscopy, have enabled the evaluation of antibacterial treatments, due to the potential to reveal the efficacy of different molecules in breaking down the biofilm. In conclusion, evidence based on scientific literature shows that established microscopic methods represent the most common tools used to characterize biofilm and its morphology in oral microbiology. Further protocols and studies on the application of advanced microscopic techniques are needed to obtain precise details on the microbiological and pathological aspects of oral biofilm.
Citation: Davide Gerardi, Sara Bernardi, Angelo Bruni, Giovanni Falisi, Gianluca Botticelli. Characterization and morphological methods for oral biofilm visualization: where are we nowadays?[J]. AIMS Microbiology, 2024, 10(2): 391-414. doi: 10.3934/microbiol.2024020
[1] | Stephen H. Kasper, Ryan Hart, Magnus Bergkvist, Rabi A. Musah, Nathaniel C. Cady . Zein nanocapsules as a tool for surface passivation, drug delivery and biofilm prevention. AIMS Microbiology, 2016, 2(4): 422-433. doi: 10.3934/microbiol.2016.4.422 |
[2] | Neetu Sharma, Sonu Bhatia, Abhinashi Singh Sodhi, Navneet Batra . Oral microbiome and health. AIMS Microbiology, 2018, 4(1): 42-66. doi: 10.3934/microbiol.2018.1.42 |
[3] | Alejandro Borrego-Ruiz, Juan J. Borrego . Human oral microbiome and its influence on mental health and brain disorders. AIMS Microbiology, 2025, 11(2): 242-294. doi: 10.3934/microbiol.2025013 |
[4] | Clotilde Maestri, Ronan L. Hébert, Patrick Di Martino . Biofilm associated with pigmented areas on a waterproofing coating surface. AIMS Microbiology, 2025, 11(1): 74-86. doi: 10.3934/microbiol.2025005 |
[5] | Afraa Said Al-Adawi, Christine C. Gaylarde, Jan Sunner, Iwona B. Beech . Transfer of bacteria between stainless steel and chicken meat: A CLSM and DGGE study of biofilms. AIMS Microbiology, 2016, 2(3): 340-358. doi: 10.3934/microbiol.2016.3.340 |
[6] | Yunfeng Xu, Attila Nagy, Gary R. Bauchan, Xiaodong Xia, Xiangwu Nou . Enhanced biofilm formation in dual-species culture of Listeria monocytogenes and Ralstonia insidiosa. AIMS Microbiology, 2017, 3(4): 774-783. doi: 10.3934/microbiol.2017.4.774 |
[7] | O. Roger Anderson . Marine and estuarine natural microbial biofilms: ecological and biogeochemical dimensions. AIMS Microbiology, 2016, 2(3): 304-331. doi: 10.3934/microbiol.2016.3.304 |
[8] | Manuela Oliveira, Eva Cunha, Luís Tavares, Isa Serrano . P. aeruginosa interactions with other microbes in biofilms during co-infection. AIMS Microbiology, 2023, 9(4): 612-646. doi: 10.3934/microbiol.2023032 |
[9] | Ariel J. Santiago, Maria L. Burgos-Garay, Leila Kartforosh, Mustafa Mazher, Rodney M. Donlan . Bacteriophage treatment of carbapenemase-producing Klebsiella pneumoniae in a multispecies biofilm: a potential biocontrol strategy for healthcare facilities. AIMS Microbiology, 2020, 6(1): 43-63. doi: 10.3934/microbiol.2020003 |
[10] | Alexandra Soares, Ana Azevedo, Luciana C. Gomes, Filipe J. Mergulhão . Recombinant protein expression in biofilms. AIMS Microbiology, 2019, 5(3): 232-250. doi: 10.3934/microbiol.2019.3.232 |
The oral microbiome represents an essential component of the oral ecosystem whose symbiotic relationship contributes to health maintenance. The biofilm represents a state of living of microorganisms surrounding themselves with a complex and tridimensional organized polymeric support and defense matrix. The substrates where the oral biofilm adhere can suffer from damages due to the microbial community metabolisms. Therefore, microbial biofilm represents the main etiological factor of the two pathologies of dental interest with the highest incidence, such as carious pathology and periodontal pathology. The study, analysis, and understanding of the characteristics of the biofilm, starting from the macroscopic structure up to the microscopic architecture, appear essential. This review examined the morphological methods used through the years to identify species, adhesion mechanisms that contribute to biofilm formation and stability, and how the action of microbicidal molecules is effective against pathological biofilm. Microscopy is the primary technique for the morphological characterization of biofilm. Light microscopy, which includes the stereomicroscope and confocal laser microscopy (CLSM), allows the visualization of microbial communities in their natural state, providing valuable information on the spatial arrangement of different microorganisms within the biofilm and revealing microbial diversity in the biofilm matrix. The stereomicroscope provides a three-dimensional view of the sample, allowing detailed observation of the structure, thickness, morphology, and distribution of the various species in the biofilm while CLSM provides information on its three-dimensional architecture, microbial composition, and dynamic development. Electron microscopy, scanning (SEM) or transmission (TEM), allows the high-resolution investigation of the architecture of the biofilm, analyzing the bacterial population, the extracellular polymeric matrix (EPS), and the mechanisms of the physical and chemical forces that contribute to the adhesion of the biofilm to the substrates, on a nanometric scale. More advanced microscopic methodologies, such as scanning transmission electron microscopy (STEM), high-resolution transmission electron microscopy (HR-TEM), and correlative microscopy, have enabled the evaluation of antibacterial treatments, due to the potential to reveal the efficacy of different molecules in breaking down the biofilm. In conclusion, evidence based on scientific literature shows that established microscopic methods represent the most common tools used to characterize biofilm and its morphology in oral microbiology. Further protocols and studies on the application of advanced microscopic techniques are needed to obtain precise details on the microbiological and pathological aspects of oral biofilm.
Oral biofilm is a complex and dynamic microbial community that forms on the surfaces of teeth and other oral structures, identified as niches, thus the tongue, the subgingival niche, the dental prosthesis, and the niche around dental implants [1].
The process of oral biofilm formation begins with the attachment of early colonizers, such as Streptococcus species, to the tooth surfaces. The chemical and physical characteristics of the substrates play an essential role in the formation of oral biofilm, which is mediated by the different forces of adhesion, such as Van der Waals forces, ionic interactions, quorum sensing, Brownian motion, surface tension, adhesion, and cohesion. These initial adhesions pave the way for establishing a diverse microbial ecosystem. As the biofilm matures, other bacteria, including Actinomyces and Fusobacterium species, join the community. Therefore, microorganisms exist in a structured and organized manner, with distinct layers of bacteria based on their metabolic activities. While some bacteria thrive in oxygen-rich environments near the tooth surface, others, such as anaerobic species like Porphyromonas gingivalis, prefer deeper layers where oxygen is limited [2]. The matrix created by the extracellular polymeric substances (EPS) is the oral biofilm's main characteristic and protects the microbial community [3] (Figure 1).
The health of the oral cavity as an ecosystem depends on the equilibrium between the microorganisms and the structures of the oral cavity. Thus, the oral biofilm is a normal and essential component of the oral ecosystem. It aids in maintaining oral health by preventing the colonization of harmful bacteria and promoting a balanced microbial community. However, if not adequately controlled through oral hygiene practices, the biofilm can become pathogenic, leading to dental diseases such as caries and periodontal disease. In the presence of different oral pathologies, oral biofilm changes the types of microorganism species and their organization [5].
Oral biofilm, which primarily constitutes bacteria, EPS, and salivary proteins, results from the interactions of different chemical, physical, and biological components within the framework. Bacteria, both gram-positive and gram-negative, form the bulk of the biofilm; Streptococcus mutans, Porphyromonas gingivalis, and Actinomyces species are among the numerous microbial species in oral biofilm. These bacteria adhere to the tooth surfaces and each other through various mechanisms, creating a diverse and organized community.
EPS is pivotal in the structural integrity of oral biofilm, forming a matrix composed of polysaccharides, proteins, and nucleic acids that encases and protects bacterial cells. This matrix also aids in adhesion to tooth surfaces, facilitating the establishment and persistence of the biofilm, and represents a dynamic scaffold that supports the three-dimensional architecture of oral biofilm [6].
Salivary proteins, such as mucins and enzymes, contribute to the chemical composition of oral biofilm. Mucins provide a lubricating and protective layer on tooth surfaces, fostering initial bacterial adhesion, while enzymes in saliva participate in the breakdown of dietary substances, influencing the metabolic activities of bacteria within the biofilm. Additionally, antimicrobial proteins in saliva play a role in the host's defense against pathogenic bacteria in the oral cavity [7].
The formation and stability of oral biofilm involves a combination of various chemical and physical forces. The chemical forces that contribute to the adhesion of bacteria to surfaces, the maintenance of the biofilm structure, and the interactions between microbial cells are Van der Waals forces, hydrogen bonding, ionic interactions, and quorum sensing (Table 1) and the physical forces are Brownian motion, surface tension, adhesion, and cohesion (Table 2).
Chemical Forces | Description of their actions and roles in biofilm formation |
Van der Waals forces | Weak attractive forces between molecules, including those present on bacterial surfaces, playing a key role in the initial adhesion of bacteria to tooth surfaces [8]. |
Hydrogen bonds | Forces between molecules with hydrogen and electronegative atoms. Regarding the oral biofilm, hydrogen bonding can occur between water molecules, proteins, and other biomolecules, influencing the structure and stability of the biofilm [8]. |
Ionic interactions | Electrostatic interactions between charged molecules or groups which can affect bacterial adhesion. The charged molecules on bacterial and tooth surfaces can lead to attractive or repulsive forces [9]. |
Quorum sensing | A chemical communication system used by bacteria to coordinate gene expression based on population density. Quorum sensing allows bacteria within the biofilm to respond collectively to environmental changes and regulate biofilm formation [10]. |
Physical Forces | Description of their actions and roles in biofilm formation |
Brownian motion | The random motion of particles, such as bacteria, due to thermal energy contributing to bacteria transport within the oral environment and colliding with tooth surfaces [11]. |
Surface tension | The tendency of the surface of a liquid to resist external forces. In the oral environment, the surface tension of saliva can influence the spreading and wetting of oral biofilm on tooth surfaces [12]. |
Adhesion and cohesion forces | Forces contributing to the structural integrity of the biofilm. Adhesion forces refer to the attachment of bacteria to tooth surfaces or other structures, while the cohesion ones involve bacteria binding to each other within the biofilm [13]. |
The bacterial composition of the oral microbiome is primarily dominated by six phyla: Firmicutes, Bacteroidetes, Proteobacteria, Actinobacteria, Spirochaetes, and Fusobacteria, representing oral cavity bacteria. Other significant constituents of the oral microbiome include Actinobacteria (genera Actinomyces, Atopobium, Corynebacterium, and Rothia), Bacteroidetes (Bergeyella, Capnocytophaga, and Prevotella), Firmicutes (Streptococcus, Granulicatella, and Veilonella), Proteobacteria (Campylobacter, Cardiobacterium, Haemophilus, Aggregatibacter, and Neisseria), and Fusobacteria. The initial colonizers of the oral cavity belong to the genera Streptococci (e.g., S. mitis and S. salivarius) and Fusobacterium [14],[15].
These early colonizers play a significant role in the proliferation of other species and the formation of the biofilm facilitated by the products of their metabolism.
The oral biofilm is classified, in relation to the main niches, into 5 types: supragingival, plaque on the tongue, subgingival plaque, plaque on the dental prosthesis, and plaque around the implant (Table 3).
Supragingival | Plaque on the tongue | Subgingival |
· Actinomyes | · Prevotella | - The outside layer: Treponemes |
· Aggregatibacter | · Rothia | |
· Fusobacterium | · Neisseria | - The first layer (on the top of the biofilm): |
· Leptotrichia | · Veillonella | · Actinomyces species |
· Capnocytophaga | · Porphyromonas | · Bacteria belonging to the Cytophaga-Flavobacterium-Bacteroides cluster |
· Corynebacterium | · Granulicatella | |
· Lautropia | · Alloprevotella | - The intermediate layer: |
· Campylobacter Tannerella | · Rothia | · F. nucleatum |
· T. forsythia | ||
· Bacteroidetes | ||
· Prevotella species | ||
· Tannerella species | ||
- The fourth layer: | ||
· Spirochaetes and other bacterial aggregates |
Furthermore, dental implants and dental restorative material could be present in the oral cavity, whose chemical and morphological properties enhance the proliferation of bacteria, thus the formation of a new biofilm on dental restorations; in particular, different factors influence the oral biofilm, such as surface roughness of hydrophilicity and material composition [18].
Due to the complexity and variability of oral microorganisms, different methods of qualitative and quantitative analysis should be carried out to study the structure of oral biofilm and its constituents. The oral microbiota composition can be analyzed at various levels, namely at the phylum, genus, species, and genotype levels of the microorganisms constituting the biofilm. Therefore, the techniques for microbiological investigation vary; the techniques of microscopic analysis of the oral biofilm could be divided into two groups: light microscopy and electron microscopy.
Microscopy serves as a fundamental tool in biofilm analysis, offering insights into the structural organization of microbial communities. Different microscopic analysis methods of oral biofilm help assess the microbial community's metabolic activity and viability.
A comprehensive morphological analysis of biofilms necessitates a multi-faceted approach that combines various analytical methods since microscopy techniques offer visual insights, molecular methods provide genetic information, metagenomics elucidates community dynamics, and biochemical analyses unveil the composition of the matrix.
Integrating these diverse methods allows us to understand the physiology of biofilm contributing to advancements in fields ranging from medicine to environmental science [19].
As technology continues to evolve, the synergy of these analytical tools promises even more significant strides in biofilm research.
This review aims to summarize the structure of oral biofilm and illustrate the different microscopy techniques available for biofilm investigation. Indeed, the characterization and the morphological investigation are fundamental from both diagnostic and therapeutic points of view. Thanks to the morphological methods, bacteria could be identified and the efficacy of different treatments to remove oral biofilm could be investigated.
The oral biofilm and the microbial community have been studied thanks to the different techniques of light microscopy, such as the optical microscopy associated with Gram stains, the stereomicroscopy, confocal laser scanning microscopy (CLSM), and the methods of electron microscopy, such as transmission electron microscopy (TEM), scanning electron microscopy (SEM), variable pressure scanning electron microscopy (VP-SEM), high resolution-transmission electron microscopy (HR-TEM), scanning and transmission electron microscopy (STEM), scanning electrochemical microscopy (SECM), and the correlative microscopy.
Light microscopy is an imaging technique that uses visible light to observe and analyze specimens. In the field of oral biofilm investigation, light microscopy offers several advantages: It allows the visualization of the microbial communities in their natural state, providing valuable information about the spatial arrangement of different microorganisms within the biofilm. Another advantage of light microscopy is its ability to reveal the microbial diversity in the biofilm matrix [20]. Disadvantages include the limitation in identifying bacterial ultrastructure components as well as cell counting.
The tools on which light microscopy can rely are stains (Gram and fluorescence probes) and different type of microscopes (light microscope, stereomicroscope, and confocal laser scanning microscope).
Researchers can differentiate and identify various bacterial species by using stains and fluorescent dyes specific to different microorganisms.
Gram staining is a microbiological technique that helps to differentiate bacterial species into two groups based on the characteristics of their cell walls, thus Gram- and Gram+ bacteria, and helps microbiologists to classify bacteria into two major groups, Gram-positive and Gram-negative, based on their ability to retain or lose the crystal violet-iodine complex during decolorization.
Unfortunately, Gram staining is not useful for microorganisms without cell walls (such as mycoplasma) or for microorganisms of reduced dimensions (such as the Chlamydia and Rickettsia species).
The prominent stains used in the Gram-staining procedure are crystal violet, iodine, safranin, and ethanol. Crystal violet is the primary stain used in the Gram-staining process; it stains all cells blue-purple. Iodine (Gram's iodine or Lugol's solution) is a mordant stain applied after the initial staining with crystal violet; it forms a complex with crystal violet within the bacterial cells, enhancing the retention of the stain. Safranin is the counterstain used in Gram staining. After decolorization, gram-negative bacteria are stained pink/red with safranin, while gram-positive bacteria retain the blue-purple color from the crystal violet-iodine complex [21].
Ethanol or ethyl alcohol (a decolorizing agent) is a staining method that differentiates bacteria based on their cell wall structure; in particular, Gram-positive bacteria retain the crystal violet-iodine complex. In contrast, Gram-negative bacteria are decolorized [22].
Stereomicroscopy provides a three-dimensional view of the sample, allowing researchers to observe the biofilm structure in detail, thus seeing the morphology, thickness, and distribution of microbial communities within the oral biofilm. An advantage of some stereomicroscopes is the live observation, enabling researchers to study dynamic processes within the oral biofilm, such as microbial movement or interactions.
Advanced stereomicroscopes may be combined with features like polarized light, fluorescence, or differential interference contrast, providing additional capabilities for specific analyses [23].
However, the low magnification and the stereo-view do not allow us to appreciate the fine structures of the microbial community, especially on prosthetic surfaces (Figure 2).
In the study of Hirohata et al., the authors conducted an in vitro study to assess the role of soluble factors produced by P. gingivalis on trophoblasts to confirm the correlation between periodontitis and the adverse outcomes of pregnancy. The authors evaluated the morphological changes after cell invasion by using the stereomicroscope. They demonstrated that after 24 hours of culture, the P. gingivalis supernatant inhibited the cell invasion without affecting cell viability or inducing apoptosis, but it caused changes in the structural stability of spheroid formations which appeared weak and irregular [24].
Fluorescence in situ hybridization (FISH) has become a fundamental tool, allowing researchers to visualize and directly identify specific microbial populations in their natural habitat.
FISH is a molecular cytogenetic technique that utilizes fluorescently labeled nucleic acid probes to bind complementary target sequences within microbial cells. The method involves the hybridization of these probes under stringent conditions, followed by fluorescence microscopy to visualize the labeled cells. In the context of oral biofilm research, FISH provides a unique opportunity to examine the spatial arrangement and taxonomic distribution of microorganisms within the complex biofilm matrix [25].
FISH enables the spatial mapping of microbial populations in their native spatial context within the oral biofilm and, by targeting specific taxa with fluorescent probes, researchers can observe the distribution and organization of microorganisms on various oral surfaces, providing insights into biofilm structure and architecture. Different probes exist, and many during a microbiological investigation vary according to different needs and the type of microorganisms. In the study of Bernardi et al., three different probes were used in order to visualize the microbial community and, in particular, the Streptococcus spp. and the Fusobacterium nucleatum. The first probe was the EUB 338 probe, which was used to visualize the entire bacterial population within the plaque specimen (Amman 1990; Al-Ahmad et al., 2007), the second one was the FUS 664 probe used to target F. nucleatum, and the third one was the STR 405 probe to visualize Streptococcus spp. [26].
Furthermore, Karygianni et al. used a method of multiplex fluorescence in situ hybridization (M-FISH) combined with confocal laser scanning microscopy (CLSM) in order to analyze multispecies oral biofilms. The multiplex fluorescence method consisted of using five different probes, which were the oligonucleotide probes EUB 338, E 79, FUS 664, IF 201, and STR 405, applied on Streptococcus spp., Actinomyces naeslundii, as early colonizers, and Fusobacterium nucleatum, Veillonella spp., as late colonizers of in situ-formed oral biofilms [27].
The specificity of FISH probes allows for identifying individual microbial species or groups, facilitating a detailed taxonomic analysis of the oral biofilm. In addition, FISH can be tailored to target specific functional groups or metabolic activities within the oral biofilm, allowing researchers to link microbial identity with functional roles, shedding light on the contributions of different taxa to overall biofilm functionality.
Besides, FISH, when combined with image analysis, provides a means to quantify the abundance of specific microbial populations in the oral biofilm [28].
This quantitative information is essential for studying microbial dynamics and the impact of various factors on biofilm composition.
As regards live/dead stains, this method provides a real-time assessment of microbial vitality within oral biofilms, offering insights into the proportion of viable and non-viable cells. Live/dead methods are employed to evaluate the efficacy of antimicrobial agents or therapeutic interventions in altering biofilm viability, such as chlorhexidine gluconate (CHX), hypochlorous acid (HOCl) stabilized with acetic acid (HAc). In the study of Aherne et al., the authors investigated the effects of stabilized hypochlorous acid (HOCl) on oral biofilm bacteria. In particular, they exposed Streptococcus mutans, Streptococcus gordonii, Actinomyces odontolyticus, Veillonella parvula, Parvimonas micra, and Porphyromonas gingivalis within a flow-cell HOCl stabilized with 0.14% or 2% HAc, pH 4.6, as well as HOCl or HAc alone. The specimen were analyzed through confocal laser scanning microscopy following LIVE/DEAD® BacLight™ staining (Figure 3). They demonstrated that low concentrations of HOCl (5 ppm), stabilized with 0.14% or 2% HAc, significantly reduced the viability of the oral communities, after 5 minutes, without causing erosion of the HA surfaces [29].
The challenges of these methods are represented by the sample dimensions, the limit of species detection, and the cost of the probes.
Live/dead methods utilize fluorescent dyes that differentially stain live and dead microbial cells based on their membrane integrity. Typically, these dyes include green fluorescent nucleic acid stains for live cells and red fluorescent nucleic acid stains for dead cells. The selective permeability of intact cell membranes allows live cells to retain the green dye, while compromised membranes in dead cells permit the entry of the red dye.
The biofilm is stained with a combination of green and red fluorescent dyes. The choice of dyes depends on the specific assay, with commonly used dyes including SYTO 9 and propidium iodide. In this regard, Tawakoli et al. compared five different live/dead assays, thus fluorescein diacetate (FDA)/propidium iodide (PI), Syto 9/PI (BacLight®), FDA/Sytox red, Calcein acetoxymethyl (AM)/Sytox red, and carboxyfluorescein diacetate (CFDA)/Sytox red, as alternatives to traditional assays which could be instable, such as the ethidium bromide staining method. They demonstrated that BacLight, FDA/Sytox red, Calcein AM/Sytox red, and CFDA/Sytox red were good alternatives to the traditional method based on ethidium bromide staining [30].
Confocal laser scanning microscopy (CLSM) provides information on its three-dimensional architecture, microbial composition, and dynamic development. The insights gained through CLSM contribute to our understanding of oral health and hold promise for developing targeted therapeutic strategies to manage biofilm-related diseases [31].
CLSM enables three-dimensional visualization of biofilms, allowing researchers to observe the spatial distribution of different microbial species within the matrix [32].
One of the primary advantages of CLSM lies in its ability to provide high-resolution, three-dimensional images of biofilm structures. In the study of oral biofilm, this capability allows researchers to visualize the arrangement of microbial cells and EPS in unprecedented detail. The non-invasive nature of CLSM permits imaging of live biofilms, capturing the dynamics of biofilm development and response to environmental stimuli.
CLSM facilitates species-specific labeling within oral biofilms through fluorescent probes [33]. This targeted approach enables researchers to distinguish between microbial species and assess their spatial distribution within the biofilm matrix. Fluorescence in situ hybridization (FISH) techniques, when combined with CLSM, allow for the precise identification of specific bacteria within the complex oral microbial community [34].
The real-time imaging capabilities of CLSM make it an invaluable tool for studying the dynamic processes involved in biofilm development. Researchers can observe the initial attachment of bacteria to tooth surfaces, the formation of microcolonies, and the maturation of biofilm architecture over time. This temporal dimension enhances our understanding of the factors influencing biofilm growth and provides crucial insights for developing strategies to manage oral health [35].
Furthermore, CLSM facilitates quantitative analysis of various biofilm parameters, including biomass, thickness, and spatial distribution of microbial populations. Image analysis software allows for quantifying fluorescence signals, providing researchers with data on the abundance and distribution of specific bacteria or biofilm components. This quantitative approach contributes to a more comprehensive understanding of the heterogeneity within oral biofilms [36].
The application of CLSM in studying oral biofilm extends to investigations of biofilm-related diseases, such as dental caries and periodontal diseases. By examining biofilm architecture in disease states, researchers can identify potential targets for therapeutic interventions. Additionally, CLSM is instrumental in evaluating the efficacy of antimicrobial agents or other interventions in disrupting biofilm formation or promoting biofilm removal [37].
Confocal microscopy or flow cytometry can be employed to visualize and quantify the stained cells within the biofilm.
Atomic force microscopy (AFM) is a high-resolution imaging technique widely used to study various biological samples, including oral biofilms. AFM operates by scanning a sharp tip over the surface of a sample and measuring the interactions between the tip and the sample's surface to create detailed topographical maps, providing a powerful and versatile approach for investigating oral biofilm's nanoscale structure, mechanical properties, and dynamic behavior. Its ability to operate in liquid environments makes it particularly suitable for studying hydrated biological samples like oral biofilms [37]. Its disadvantages are represented by the time employed to get the images and the quality of the image, which can be affected by the geometry of the probe.
AFM produces high-resolution topographical images of the oral biofilm surface revealing details, such as the three-dimensional structure of microbial cells, extracellular polymeric substances (EPS), and biofilm's roughness, thickness, and morphology, and allows us to detect and measure the interactions between microorganisms and substrates and other biofilm components [38].
AFM can be used for force spectroscopy studies, where the tip is used to apply controlled forces to the biofilm surface, allowing researchers to measure the mechanical properties of the biofilm, such as its stiffness or adhesion forces between microbial cells and the substrate [39].
In the study of Tang et al., the mechanism of adhesion of the early colonizers of the oral cavity, Actynomices spp., to a substrate at nano newton (nN) range force levels was investigated. The authors measured the interactive forces between the bacterial biofilm formed by fimbriated and non-fimbriated bacteria belonging to the Actynomices spp., thus A. bovis, A. gerencseriae, A. israelii, A. Meyer, A. naeslundii genospecies 1 and 2, A. odontolyticus, and A. viscous, and a silicon nitride tip, through a Nanoscope IIIA atomic force microscope. The authors demonstrated that A. naeslundii genospecies 1, 2, and A. viscosus, which are fimbriated, develop higher cell-surface interactive forces than fimbriated Actynimoces spp. [40].
Furthermore, AFM-based techniques, such as AFM-IR (infrared spectroscopy), can provide chemical information about biofilm components by integrating AFM with infrared spectroscopy, allowing researchers to identify specific molecular structures within the biofilm [41].
Transmission electron microscopy (TEM) stands as a fundamental tool in the field of microbiology, allowing the visualization of individual bacterial cells and extracellular polymeric substances (EPS) within oral biofilms at the nanometer scale. This high-resolution imaging capability is crucial for discerning the fine details of biofilm architecture, including the arrangement of bacterial cells, the composition of EPS, and the interactions between microorganisms and matrix components [42],[43].
TEM facilitates a detailed examination of the biofilm matrix, shedding light on the composition and organization of EPS, and allows researchers to identify the different components, such as polysaccharides, proteins, and nucleic acids, contributing to the structural integrity of the biofilm, by visualizing the matrix at the nanoscale. This information is essential to understanding the adhesive and cohesive forces that maintain biofilm stability.
TEM could be applied in the study of antimicrobial treatments, how a molecule may influence the structural organization of the biofilm, and how it acts on microorganisms. The disadvantages of the TEM are represented by the cutting and the protocol used for embedding and the stains.
In the study of Vitkov et al., authors investigated the alterations of the biofilm caused by their exposition to chlorhexidine. The main alterations induced were the disruption of the bacterial membrane integrity and the disintegration of the fimbria, associated with partial matrix disintegration in some cases, but each alteration induced by the chlorhexidine did not induce a whole disruption of the organization of the biofilm, demonstrating that chlorhexidine alone does not have a sufficient efficacy against the oral biofilm [44].
In addition, researchers can explore how bacteria adhere to each other and tooth surfaces, forming microcolonies and establishing the intricate network characteristic of biofilm architecture [45].
Another advantage of TEM in the study of oral biofilm lies in its ability to visualize ultrastructural changes associated with biofilm-related diseases, such as dental caries and periodontal diseases. It allows for the examination of the process on which the alteration of the biofilm matrix and microbial cells is based in disease states, providing critical information for understanding the pathogenesis of these conditions at the nanoscale and investigating new treatments [46]. In the study of Zhao et al., the authors investigated the antimicrobial properties of Mg-Cu alloy grafts in the treatment of bone defects caused by periodontal disease. P. gingivalis and A. actinomycetemcomitans were cultured in Mg-Cu alloy extracts to evaluate their viability using SEM and TEM. SEM was used to investigate the biofilm changes, while TEM allowed researchers to study its structure. The electron microscopy revealed the complete destruction of the biofilm, resulting in the disruption of cell membranes and the subsequent bacterial apoptosis. In this case, using TEM allowed us to analyze new materials, such as Mg-Cu alloy grafts, against the periodontal bacteria in tissue regeneration [47].
Scanning electron microscopy (SEM) is a technique used to study the surface structure of cells and tissues at a high resolution. The SEM technique involves scanning a sample with a focused beam of electrons, and the resulting signals are used to create detailed images of the sample's surface [48].
In the case of oral biofilm, this enables scientists to observe the arrangement, size, and shape of individual bacteria within the biofilm. It provides valuable insights into the composition and organization of microbial communities in the oral cavity, contributing to a better understanding of oral health and diseases. SEM's ability to produce high-resolution images allows for the detailed examination of the surface features of oral biofilms [49].
In the study of Georgiev et al., authors analyzed demineralized enamel specimens infiltrated with triethylene glycol dimethacrylate (TEGDMA) resin and exposed to a biofilm formed by S. mutans, S.oralis, and Actinomices oris in group 1, to the biofilm in group 2, and only resin infiltrated in group 3. A fourth group was used as a control. The authors used SEM and CLSM to study the biofilm and the material's autofluorescence after 24 hours. SEM and CLSM analysis showed reduced biofilm formation on resin-infiltrated specimens (group 1) compared to group 2, while no biofilm was detectable in groups 3 and 4. Thanks to the use of SEM, authors demonstrated that freshly resin-infiltrated enamel surfaces have an effect on biofilm reduction, while monomer leakage was not affected by bacterial presence [50].
The high magnification and depth of field offered by SEM allow researchers to examine the fine details of EPS, including the web-like structures that encapsulate microbial cells. This information is crucial for understanding the biofilm matrix's adhesion, cohesion, and mechanical properties. SEM is employed in detecting, through the auxiliary of other methods like immunohistochemistry, different molecules that could be in the oral biofilm and, in particular, in its structure, such as the EPS, revealing the association between oral pathologies and systemic disease, [51]. In the study of Kanagasingam et al., authors made an SEM analysis in order to detect Amyloid-β (Aβ) in naturally formed oral biofilm: 87 freshly extracted teeth affected by the periodontal disease were selected and collected in two groups, thus, group A of 11 teeth with primary root canal infection and group B with 21 teeth whose endodontic treatment was failed. The biofilm to be analyzed was immune-stained with the anti-Aβ antibody to reveal the eventual presence of Aβ. SEM images helped to characterize the biofilm (Figure 4). Light microscopy associated with immunohistochemistry showed the presence of Aβ in group A, while no specific results were detected in group B [52].
SEM is often coupled with energy-dispersive X-ray spectroscopy (EDS) for elemental analysis, allowing researchers to identify the elemental composition of biofilm components. This integration provides additional information about the chemical composition of microbial cells and the matrix [52]. An important problem during the observation of oral biofilm images with SEM is the dehydration procedure of the specimens, which could induce artifacts such as matrix EPS collapse and misunderstanding in the results. In order to overcome this problem, a recent technique allows us to visualize images with SEM avoiding the process of dehydration but using ionic liquid.
Variable pressure scanning electron microscopy (VP-SEM) is a technique used to overcome the issues in the SEM observations, since it does not require dehydration or drying, but a different fixation with different fixatives represented by aldehydes as glutaraldehyde or paraformaldehyde cross-links proteins and osmium tetroxide (OsO4), which have the advantage of binding to lipids, so that the bacterial and fungal biofilm characterization is more efficient [53].
In the study of Bossù et al. (2020), authors used a different method of characterization of S. wiggsiase, which is one of the most representative microorganisms, which composes the early childhood caries (ECC), using SEM combined with a protocol that preserves the biofilm architecture. The protocol developed by the authors, named OsO4-RR-TA-IL, which consists of adopting osmium tetroxide (OsO4), ruthenium red (RR), tannic acid (TA) impregnation, and ionic liquid (IL) drop casting, and allows us to avoid dehydration, drying, and sputter coating. The result of this method is the achievement of high-magnification imaging (from 10000 x to 35000 x) in high-vacuum and high-voltage conditions [54].
Scanning transmission electron microscopy (STEM) is an advanced microscopy technique that combines the principles of TEM and SEM. STEM is beneficial for imaging thin sections of samples with high spatial resolution and is employed in studying various biological specimens, including oral biofilms [55].
STEM provides high spatial resolution imaging, allowing researchers to visualize fine details of the oral biofilm at the nanoscale. It is particularly effective in revealing the internal structures of microbial cells, extracellular polymeric substances (EPS), and other biofilm components. Similar to HR TEM, STEM can be used for three-dimensional imaging by acquiring a series of images from different perspectives. This information can be used to reconstruct three-dimensional representations of the oral biofilm [56].
Hickey et al. used a particular STEM technique, which is bright-field scanning transmission electron microscopy (BF-STEM), combined with tomography to study nanostructures in the biofilm, such as the characteristics of cells that influence the adhesion process and the specific structure that mediate the extracellular communication. The main structures involved are pili, flagella, and membrane tubules. In this kind of investigation, STEM is valuable because it overcomes the limitations of standard TEM, like its defocus issues, so it is possible to reach a high resolution of 4–10 nm in the visualization of the biofilm. STEM images allow us to visualize nanopod structures, deepening the fibrillar formations' shapes and architectures. The advantage of this technique is that it allows for the analysis of the nanorods of bacteria, which contributes to the stability of the biofilm [57].
Another application of STEM is to visualize the antibacterial activity of polymeric nanoparticles on a biofilm with an ionic liquid. Takahashi et al. developed a method to analyze organic polymeric nanoparticles (NPs) in a biofilm formed by Staphylococcus epidermis. STEM can show the interaction of drugs with target molecules, which is helpful in studying new aspects of treatments against biofilms [58]. However, few studies are available in the literature, making this a new technique to be explored in the field of biofilm morphological research.
High-resolution transmission electron microscopy (HR-TEM) is an advanced microscopy technique that uses a beam of electrons to create high-resolution images of thin sections of samples. In the study of oral biofilm, HR-TEM can provide detailed insights into the ultrastructure and composition of microbial cells [59].
HR-TEM offers extremely high magnification, allowing researchers to visualize the nanoscale details of microbial cells, EPS, and other structures within the oral biofilm, such as the cell membrane, cell wall, nucleoid, pili, and other cellular organelles. Thanks to its high resolution, HR-TEM is also helpful in studying the antibacterial role of nanoparticles, paving the way for new technologies against oral biofilm. In the study of Azad et al., a new treatment against E. faecalis, which is one of the most representative microorganisms in the periapical lesion, based on using bismuth nanoparticles, was analyzed through HR-TEM images showing an actual efficacy against not only S. mutans, but also E. faecalis whose growth inhibition was demonstrated at a concentration range between 0.625 µg/mL and 20 µg/mL [60].
Another study conducted by Ahmed et al. [61] focused on the antibacterial properties of gum Arabic-silver nanoparticles against Streptococcus sanguinis (S. sanguinis), Streptococcus mutans (S. mutans), Lactobacillus acidophilus (L. acidophilus), and Candida albicans (C. albicans). In this case, HR-TEM was applied to characterize the substrates composed of the gum Arabic-silver nanoparticles, thus its spherical shape with core sizes between 4 and 26 nm. In particular, HR-TEM visualization allowed us to understand that the antimicrobial effect depends on the shape and size of nanoparticles (Figure 5). Moreover, the smaller sizes of gum Arabic-silver nanoparticles showed higher activity than the larger ones [61].
Another example of the application of HR-TEM to study nanoparticles was the research of Jardón-Romero et al., which evaluated the antimicrobial effect of biogenic silver nanoparticles obtained from Syzygium aromaticum against E. coli, S. aureus, E. faecalis, S. mutans, and C. albicans. The HR-TEM images showed that the nanoparticles mentioned above had a crystalline nature and a shape similar to a sphere. Besides, the antibacterial effect had inhibition zones of 2–4 mm in diameter [62].
Some HR-TEM systems are equipped with EDS detectors, which can be used to analyze the elemental composition of the oral biofilm. EDS provides information about the distribution of elements within the biofilm, aiding in identifying specific components [63].
As the chemical microenvironment is crucial for the development of chemical interactions between the oral biofilm and the surface of the niches existing in the oral cavity, an advanced microscopic method that allows researchers to investigate this outstanding aspect of the oral biofilm with a better precision is represented by scanning electrochemical microscopy (SECM). SECM is equipped with a tip represented by a platinum or gold-ultramicroelectrode of about 25 mm [64]. In particular, this method is based on the use of electrochemical sensors, which differs from the traditional ones thanks to their properties of miniaturization, stability, and selectivity. The main electrochemical sensors are Ca2+ and hydrogen peroxide, and they are used as probes applied to the scanning electrochemical microscope. The advantage of this method is to obtain the revelation of real-time interaction between the bacterial biofilm and the dental surface [65]. Regarding hydrogen peroxide (H2O2), its application to the microscopic method is crucial due to its wide presence in many cellular processes as a metabolite. In the study of Joshi et al. [66], the H2O2 was used as a probe for the electrochemical microscope. In particular, the authors applied Pt nanoparticles on a multi-walled carbon nanotube and conducting ionic liquid matrix in order to obtain high sensitivity for H2O2 oxidation.
Another type of probe applied to SECM is the dual-tip glucose sensor composed of the glucose oxidase (GOD) enzyme blocked in an ultramicroelectrode (UME). In the study of Jayathilake et al. [67], the above-described glucose microsensor was used in order to obtain the level of local glucose uptake of S. mutans biofilms in the presence of sucrose that was metabolised by S. mutans to adhere to the tooth surface.
Furthermore, another electrochemical microsensor developed by Joshi et al. in 2017 [68] is a carbon-based pH microsensor. This sensor was applied in order to investigate the microbial metabolic exchange between two prevalent bacteria of the oral biofilm, thus, the commensal S. gordonii and pathogenic S. mutans. The authors demonstrated that S. gordonii was the dominant microorganism producing H2O2, while S. mutans is dominant in pH changes of saliva, resulting in the pH decreasing to 5.0 or less due to the production of lactic acid.
Finally, the combination of different microsensors is possible in scanning electrochemical microscopy, allowing the detection of new real-time events on which the interaction between oral biofilm and tooth surface is based. Developed by Park et al. [69], it combined three sensors—redex, pH, and H2O2—in order to simultaneously map the pH and the H2O2 concentration produced by dental plaque on a surface composed of hydroxyapatite.
Quantitative microscopy in the study of oral biofilm includes each method combined with the microscopic techniques in order to quantitatively analyze various aspects of oral biofilms, e.g., quantitative microscopy exploits the CLSM and the digital images in order to count the number of nuclei of microorganisms in the specific specimen, and once identified, it allows the counting of the bacterial species. Regarding this type of investigation, another tool used to identify the bacteria and count them is the above-described FISH method.
In the study of Dige et al. [70], in situ biofilm was collected from healthy individuals for 12 hours, divided into two groups in relation to the timing (during the day and during the night). Then, the specimen was treated with the FISH method and bacteria were visualized using confocal laser scanning microscopy. Thanks to the combination of stereological methods and digital images, the authors demonstrated a statistically significant difference between the total number of bacteria and the biovolume in the two 12-hour groups; specifically, the highest accumulation of bacteria was detected during the day. Regarding the use of the FISH method, the authors exploited specific probes for streptococci and Actinomyces naeslundii to count them in both daytime and nighttime, showing a higher number of streptococci in biofilms during daytime than nighttime (while there were no differences regarding the amount of A.Naeslundii).
Another study conducted by Dige et al. in 2009 [71] showed how the combination of stereological methods with the FISH method was effective in quantifying bacteria. The authors collected samples of intact dental biofilm at 6, 12, 24, 48 hours, which was analyzed through CLSM, while the quantification of bacteria was conducted using 16S ribosomal RNA oligonucleotide probes. The study demonstrated the efficacy of the applied method and showed that the total number of bacteria and streptococci increased over time.
Furthermore, thanks to the development of artificial intelligence (AI) [72], new protocols have been studied in the field of quantitative microscopy. In a recent study conducted by Ding et al., an AI method combined with SEM was applied in order to quantify the initial bacterial adhesion on different dental materials. In particular, authors measured the amount of Porphyromonas gingivalis and Fusobacterium nucleatum on dental zirconia surfaces using SEM images at 1, 7, and 24 hours to which Fiji software, as the AI method, was applied. The same software was used on SEM images of Streptococcus mutans cultured on a PMMA nanostructured surface at 1, 24, 72, and 168 hours. It was then compared with the traditional quantitative microscopy applied to the live/dead CLSM method.
The results showed an increasing amount of bacteria over time, while both the AI method and CLSM were comparable, paving the way for a reduction of time, cost, and labor thanks to the use of AI software combined with SEM images.
Correlative microscopy involves the synergistic application of multiple imaging techniques to study biological specimens [73].
In oral biofilm research, this approach combines the strengths of different microscopy modalities, such as light, electron, and fluorescence. By employing techniques such as confocal laser scanning microscopy in tandem with scanning electron microscopy or light microscopy combined with high-resolution electron microscopy, researchers can achieve a seamless transition from macro- to micro-scale observations [74].
The combination of fluorescence and electron microscopy in correlative studies enables researchers to unravel the microbial diversity within oral biofilm. Fluorescence microscopy, capable of selectively labeling specific microbial groups, can identify critical bacterial species. Subsequently, electron microscopy provides ultrastructural details, allowing for a more in-depth analysis of microbial interactions and spatial organization within the biofilm matrix [75].
As demonstrated by Daddi Oubekka et al., correlative time-resolved fluorescence microscopy is a method to describe the diffusion of antibiotics in biofilm. Many factors related to the microorganism and the architecture of the biofilm can be obstacles to the diffusion of antibiotics, so the advantage of this technique is to obtain images in an accurate spatiotemporal resolution, overcoming the limitations of the time-lapse confocal imaging microscopy, in order to assess the characteristics of the biofilm and how it reacts to antibiotics. The set of techniques used in this study was composed of fluorescence recovery after photobleaching, fluorescence correlation spectroscopy, and fluorescence lifetime imaging. The correlative analysis was conducted on a biofilm formed by two S. aureus to assess the reaction to the diffusion of vancomycin in the biofilm. The authors showed that the matrix that composes the biofilm did not obstruct the diffusion of vancomycin, which could penetrate through all cells. However, biofilm components could be obstacles for the bioavailability of vancomycin, which is the real reason why many antibiotics have a low efficacy when their target is the biofilm [76].
The complexity of oral biofilm requires different methods of investigation, both for understanding its origins and how to control it. The above-described microscopy techniques have been developed to exploit the advantages of each one in the study of the different microscopical levels of biofilm. The established microscopy methods (light, fluorescence, and electron) have been widely used, with the development of specific protocols to allow for a high-quality morphological characterization and visualization. On the other hand, the most recent microscopy methods, such as STEM, HR-TEM, and correlative microscopy, have yet to be fully exploited for studying oral biofilm and the efficacy of nanomolecules used for microbicidal purposes. Further protocols and studies on applying advanced microscopic techniques and image analysis are strongly necessary to obtain fine details on the microbiological, pathological, and therapeutic aspects of oral biofilm.
The authors declare they have not used Artificial Intelligence (AI) tools in the creation of this article.
[1] |
Verma D, Garg PK, Dubey AK (2018) Insights into the human oral microbiome. Arch Microbiol 200: 525-540. https://doi.org/10.1007/s00203-018-1505-3 ![]() |
[2] |
Dewhirst FE, Chen T, Izard J, et al. (2010) The human oral microbiome. J Bacteriol 192: 5002-17. https://doi.org/10.1128/JB.00542-10 ![]() |
[3] |
Reese S, Guggenheim B (2007) A novel TEM contrasting technique for extracellular polysaccharides in In Vitro biofilms. Microsc Res Tech 70: 816-822. https://doi.org/10.1002/jemt.20471 ![]() |
[4] |
Rath S, Bal SCB, Dubey D (2021) Oral biofilm: development mechanism, multidrug resistance, and their effective management with novel techniques. Rambam Maimonides Med J 12: e0004. https://doi.org/10.5041/RMMJ.10428 ![]() |
[5] |
Wade WG (2013) The oral microbiome in health and disease. Pharmacol Res 69: 137-43. https://doi.org/10.1016/j.phrs.2012.11.006 ![]() |
[6] |
Karygianni L, Ren Z, Koo H (2020) Biofilm matrixome: extracellular components in structured microbial communities. Trends Microbiol 28: 668-681. https://doi.org/10.1016/j.tim.2020.03.016 ![]() |
[7] |
Marsh PD, Do T, Beighton D, et al. (2016) Influence of saliva on the oral microbiota. Periodontol 2000 70: 80-92. https://doi.org/10.1111/prd.12098 ![]() |
[8] |
Wessel SW, Chen Y, Maitra A, et al. (2014) Adhesion forces and composition of planktonic and adhering oral microbiomes. J Dent Res 93: 84-8. https://doi.org/10.1177/0022034513511822 ![]() |
[9] |
Badihi Hauslich L, Sela MN, Steinberg D, et al. (2013) The adhesion of oral bacteria to modified titanium surfaces: role of plasma proteins and electrostatic forces. Clin Oral Implants Res 24: 49-56. https://doi.org/10.1111/j.1600-0501.2011.02364.x ![]() |
[10] |
Shao H, Demuth DR (2010) Quorum sensing regulation of biofilm growth and gene expression by oral bacteria and periodontal pathogens. Periodontol 2000 52: 53-67. https://doi.org/10.1111/j.1600-0757.2009.00318.x ![]() |
[11] |
Song L, Hou J, van der Mei HC, et al. (2016) Antimicrobials influence bond stiffness and detachment of oral bacteria. J Dent Res 95: 793-799. https://doi.org/10.1177/0022034516634631 ![]() |
[12] |
Quirynen M, Bollen CM (1995) The influence of surface roughness and surface-free energy on supra- and subgingival plaque formation in man. A review of the literature. J Clin Periodontol 22: 1-14. https://doi.org/10.1111/j.1600-051x.1995.tb01765.x ![]() |
[13] |
Carniello V, Peterson BW, van der Mei HC, et al. (2018) Physico-chemistry from initial bacterial adhesion to surface-programmed biofilm growth. Adv Colloid Interface Sci 261: 1-14. https://doi.org/10.1016/j.cis.2018.10.005 ![]() |
[14] |
Tuominen H, Collado MC, Rautava J, et al. (2019) Composition and maternal origin of the neonatal oral cavity microbiota. J Oral Microbiol 11: 1663084. https://doi.org/10.1080/20002297.2019.1663084 ![]() |
[15] |
Zijnge V, van Leeuwen MB, Degener JE, et al. (2010) Oral biofilm architecture on natural teeth. PLoS One 5: e9321. https://doi.org/10.1371/journal.pone.0009321 ![]() |
[16] |
Seidel CL, Gerlach RG, Wiedemann P, et al. (2020) Defining metaniches in the oral cavity according to their microbial composition and cytokine profile. Int J Mol Sci 21: 8218. https://doi.org/10.3390/ijms21218218 ![]() |
[17] |
Gao L, Xu T, Huang G, et al. (2018) Oral microbiomes: more and more importance in oral cavity and whole body. Protein Cell 9: 488-500. https://doi.org/10.1007/s13238-018-0548-1 ![]() |
[18] |
Hao Y, Huang X, Zhou X, et al. (2018) Influence of dental prosthesis and restorative materials interface on oral biofilms. Int J Mol Sci 19: 3157. https://doi.org/10.3390/ijms19103157 ![]() |
[19] |
Shitomi K, Miyaji H, Miyata S, et al. (2020) Photodynamic inactivation of oral bacteria with silver nanoclusters/rose bengal nanocomposite. Photodiagnosis Photodyn Ther 30: 101647. https://doi.org/10.1016/j.pdpdt.2019.101647 ![]() |
[20] |
Kriem LS, Wright K, Ccahuana-Vasquez RA, et al. (2020) Confocal Raman microscopy to identify bacteria in oral subgingival biofilm models. PLoS One 15: e0232912. https://doi.org/10.1371/journal.pone.0232912 ![]() |
[21] |
Coico R (2005) Gram staining. Curr Protoc Microbiol . https://doi.org/10.1002/9780471729259.mca03cs00 ![]() |
[22] | Moyes RB, Reynolds J, Breakwell DP (2009) Differential staining of bacteria: gram stain. Curr Protoc Microbiol . https://doi.org/10.1002/9780471729259.mca03cs15 |
[23] |
Wang J, Chen W, Jiang Y, et al. (2013) Imaging of extraradicular biofilm using combined scanning electron microscopy and stereomicroscopy. Microsc Res Tech 76: 979-983. https://doi.org/10.1002/jemt.22257 ![]() |
[24] |
Hirohata N, Komine-Aizawa S, Tamura M, et al. (2017) Porphyromonas gingivalis Suppresses Trophoblast Invasion by Soluble Factors. J Periodontol 88: 1366-1373. https://doi.org/10.1902/jop.2017.170193 ![]() |
[25] |
Gu J, Wang H, Zhang M, et al. (2022) Application of fluorescence in situ hybridization (FISH) in oral microbial detection. Pathogens 11: 1450. https://doi.org/10.3390/pathogens11121450 ![]() |
[26] | Bernardi S, Continenza MA, Al-Ahmad A, et al. (2019) Streptococcus spp. and Fusobacterium nucleatum in tongue dorsum biofilm from halitosis patients: a fluorescence in situ hybridization (FISH) and confocal laser scanning microscopy (CLSM) study. New Microbiol 42: 108-113. |
[27] |
Karygianni L, Hellwig E, Al-Ahmad A (2014) Multiplex fluorescence in situ hybridization (M-FISH) and confocal laser scanning microscopy (CLSM) to analyze multispecies oral biofilms. Methods Mol Biol 1147: 65-72. https://doi.org/10.1007/978-1-4939-0467-9_5 ![]() |
[28] |
Shen Y, Yu F, Qiu L, et al. (2023) Ecological influence by colonization of fluoride-resistant Streptococcus mutans in oral biofilm. Front Cell Infect Microbiol 12: 1106392. https://doi.org/10.3389/fcimb.2022.1106392 ![]() |
[29] |
Aherne O, Ortiz R, Fazli MM, et al. (2022) Effects of stabilized hypochlorous acid on oral biofilm bacteria. BMC Oral Health 22: 415. https://doi.org/10.1186/s12903-022-02453-2 ![]() |
[30] |
Tawakoli PN, Al-Ahmad A, Hoth-Hannig W, et al. (2013) Comparison of different live/dead stainings for detection and quantification of adherent microorganisms in the initial oral biofilm. Clin Oral Investig 17: 841-50. https://doi.org/10.1007/s00784-012-0792-3 ![]() |
[31] |
Wang Z, de la Fuente-Núñez C, Shen Y, et al. (2015) Treatment of oral multispecies biofilms by an anti-biofilm peptide. PLoS One 10: e0132512. https://doi.org/10.1371/journal.pone.0132512 ![]() |
[32] |
Alovisi M, Pasqualini D, Mandras N, et al. (2022) Confocal laser scanner evaluation of bactericidal effect of chitosan nanodroplets loaded with benzalkonium chloride. J Clin Med 11: 1650. https://doi.org/10.3390/jcm11061650 ![]() |
[33] |
Wood SR, Kirkham J, Marsh PD, et al. (2000) Architecture of intact natural human plaque biofilms studied by confocal laser scanning microscopy. J Dent Res 79: 21-27. ![]() |
[34] |
Di Pippo F, Venezia C, Sighicelli M, et al. (2020) Microplastic-associated biofilms in lentic Italian ecosystems. Water Res 187: 116429. https://doi.org/10.1016/j.watres.2020.116429 ![]() |
[35] | Zhang YS, Li NY (2007) Current application of confocal laser scanning microscope (CLSM) in stomatology. Shanghai Kou Qiang Yi Xue 16: 219-24. |
[36] |
Carvalho FG, Puppin-Rontani RM, Fúcio SB, et al. (2012) Analysis by confocal laser scanning microscopy of the MDPB bactericidal effect on S. mutans biofilm CLSM analysis of MDPB bactericidal effect on biofilm. J Appl Oral Sci 20: 568-75. https://doi.org/10.1590/s1678-77572012000500013 ![]() |
[37] |
Zago LHP, de Annunzio SR, de Oliveira KT, et al. (2020) Antimicrobial photodynamic therapy against metronidazole-resistant dental plaque bactéria. J Photochem Photobiol B 209: 111903. https://doi.org/10.1016/j.jphotobiol.2020.111903 ![]() |
[38] |
Li H, Liu H, Zhang L, et al. (2023) Evaluation of extracellular polymeric substances matrix volume, surface roughness and bacterial adhesion property of oral biofilm. J Dent Sci 18: 1723-1730. https://doi.org/10.1016/j.jds.2022.12.022 ![]() |
[39] |
Salavadhi SS, Chintalapani S, Ramachandran R, et al. (2017) Atomic Force Microscopy: A three-dimensional reconstructive tool of oral microbiota in gingivitis and periodontitis. J Indian Soc Periodontol 21: 264-269. https://doi.org/10.4103/jisp.jisp_209_17 ![]() |
[40] |
Wang R, Wang Y, Lei Z, et al. (2022) Glucosyltransferase-modulated Streptococcus mutans adhesion to different surfaces involved in biofilm formation by atomic force microscopy. Microbiol Immunol 66: 493-500. https://doi.org/10.1111/1348-0421.13025 ![]() |
[41] |
Tang G, Yip HK, Samaranayake LP, et al. (2004) Direct detection of cell surface interactive forces of sessile, fimbriated and non-fimbriated Actinomyces spp. using atomic force microscopy. Arch Oral Biol 49: 727-38. https://doi.org/10.1016/j.archoralbio.2004.04.003 ![]() |
[42] |
Shan T, Huang L, Tay FR, et al. (2022) Retention of intrafibrillar minerals improves resin-dentin bond durability. J Dent Res 101: 1490-1498. https://doi.org/10.1177/00220345221103137 ![]() |
[43] |
Ioannidis K, Niazi S, Mylonas P, et al. (2019) The synthesis of nano silver-graphene oxide system and its efficacy against endodontic biofilms using a novel tooth model. Dent Mater 35: 1614-1629. https://doi.org/10.1016/j.dental.2019.08.105 ![]() |
[44] | Fathi F, Sadrnia M, Arjomandzadegan M, et al. (2021) In vitro and in vivo evaluation of antibacterialantibacterial and anti-biofilm properties of five ethnomedicinal plants against oral bacteria by TEM. Avicenna J Phytomed 11: 180-189. |
[45] |
Vitkov L, Hermann A, Krautgartner WD, et al. (2005) Chlorhexidine-induced ultrastructural alterations in oral biofilm. Microsc Res Tech 68: 85-89. https://doi.org/10.1002/jemt.20238 ![]() |
[46] |
Keleş A, Keskin C, Kalkan M, et al. (2021) Visualization and characterization of Enterococcus faecalis biofilm structure in bovine dentin using 2D and 3D microscopic techniques. Arch Microbiol 203: 269-277. https://doi.org/10.1007/s00203-020-02031-6 ![]() |
[47] | Zhao X, Wan P, Wang H, et al. (2020) An antibacterialantibacterial strategy of mg-cu bone grafting in infection-mediated periodontics. Biomed Res Int 2020: 7289208. https://doi.org/10.1155/2020/7289208 |
[48] |
Postek MT, Vladár AE (2013) Does your SEM really tell the truth?--How would you know? Part 1. Scanning 35: 355-361. https://doi.org/10.1002/sca.21075 ![]() |
[49] |
Jaeggi M, Gyr S, Astasov-Frauenhoffer M, et al. (2022) Influence of different zirconia surface treatments on biofilm formation in vitro and in situ. Clin Oral Implants Res 33: 424-432. https://doi.org/10.1111/clr.13902 ![]() |
[50] |
Georgiev KG, Filipov IA, Dobrev IN (2018) In Vivo Collection and SEM identification of oral biofilm using indirect composite prototype restorations. Folia Med (Plovdiv) 60: 300-307. https://doi.org/10.1515/folmed-2017-0092 ![]() |
[51] |
Li YY, Li BS, Liu WW, et al. (2020) Effects of D-arginine on Porphyromonas gingivalis biofilm. J Oral Sci 62: 57-61. https://doi.org/10.2334/josnusd.19-0075 ![]() |
[52] |
Kanagasingam S, von Ruhland C, Welbury R, et al. (2022) Ex vivo detection of amyloid-β in naturally formed oral biofilm. J Alzheimers Dis Rep 6: 757-773. https://doi.org/10.3233/ADR-220076 ![]() |
[53] | Relucenti M, Familiari G, Donfrancesco O, et al. (2021) Microscopy methods for biofilm imaging: focus on SEM and VP-SEM pros and cons. Biology (Basel) 10: 51. https://doi.org/10.3390/biology10010051 |
[54] |
Bossù M, Selan L, Artini M, et al. (2020) Characterization of Scardovia wiggsiae biofilm by original scanning electron microscopy protocol. Microorganisms 8: 807. https://doi.org/10.3390/microorganisms8060807 ![]() |
[55] |
Saglie FR, Carranza FA, Newman MG (1985) The presence of bacteria within the oral epithelium in periodontal disease. I. A scanning and transmission electron microscopic study. J Periodontol 56: 618-624. https://doi.org/10.1902/jop.1985.56.10.618 ![]() |
[56] |
AA, Leapman RD (2012) Development and application of STEM for the biological sciences. Ultramicroscopy 123: 38-49. https://doi.org/10.1016/j.ultramic.2012.04.005 ![]() |
[57] |
Hickey WJ, Shetty AR, Massey RJ, et al. (2017) Three-dimensional bright-field scanning transmission electron microscopy elucidate novel nanostructure in microbial biofilms. J Microsc 265: 3-10. https://doi.org/10.1111/jmi.12455 ![]() |
[58] |
Takahashi C, Muto S, Yamamoto H (2017) A microscopy method for scanning transmission electron microscopy imaging of the antibacterialantibacterial activity of polymeric nanoparticles on a biofilm with an ionic liquid. J Biomed Mater Res Part B 105: 1432-1437. https://doi.org/10.1002/jbm.b.33680 ![]() |
[59] |
Pathak A, Pv S, Stanley J, et al. (2019) Multicolor emitting N/S-doped carbon dots as a fluorescent probe for imaging pathogenic bacteria and human buccal epithelial cells. Mikrochim Acta 186: 157. https://doi.org/10.1007/s00604-019-3270-7 ![]() |
[60] |
Azad A, Rostamifar S, Modaresi F, et al. (2020) Assessment of the antibacterialantibacterial effects of bismuth nanoparticles against Enterococcus faecalis. Biomed Res Int 2020: 5465439. https://doi.org/10.1155/2020/5465439 ![]() |
[61] |
Ahmed O, Sibuyi NRS, Fadaka AO, et al. (2022) Antimicrobial effects of gum arabic-silver nanoparticles against oral pathogens. Bioinorg Chem Appl 2022: 9602325. https://doi.org/10.1155/2022/9602325 ![]() |
[62] |
Jardón-Romero EA, Lara-Carrillo E, González-Pedroza MG, et al. (2022) Antimicrobial activity of biogenic silver nanoparticles from Syzygium aromaticumagainst the five most common microorganisms in the oral cavity. Antibiotics (Basel) 11: 834. https://doi.org/10.3390/antibiotics11070834 ![]() |
[63] |
Liu W, Jing C, Liu X, et al. (2022) 3D imaging of single bacterial cells using surface-enhanced Raman spectroscopy with a multivariate curve resolution model. Analyst 147: 223-229. https://doi.org/10.1039/d1an01879e ![]() |
[64] |
Darch SE, Koley D (2018) Quantifying microbial chatter: scanning electrochemical microscopy as a tool to study interactions in biofilms. Proc R Soc A 474: 20180405. http://dx.doi.org/10.1098/rspa.2018.0405 ![]() |
[65] |
Koley D (2022) Electrochemical sensors for oral biofilm-biomaterials interface characterization: A review. Mol Oral Microbiol 37: 292-298. https://doi.org/10.1111/omi.12396 ![]() |
[66] |
Joshi VS, Kreth J, Koley D (2017) Pt-decorated MWCNTs-Ionic liquid composite-based hydrogen peroxide sensor to study microbial metabolism using scanning electrochemical microscopy. Anal Chem 89: 7709-7718. https://doi.org/10.1021/acs.analchem.7b01677 ![]() |
[67] |
Jayathilake NM, Koley D (2020) Glucose microsensor with covalently immobilized glucose oxidase for probing bacterial glucose uptake by scanning electrochemical microscopy. Anal Chem 92: 3589-3597. https://doi.org/10.1021/acs.analchem.9b04284 ![]() |
[68] |
Joshi VS, Sheet PS, Cullin N, et al. (2017) Real-time metabolic interactions between two bacterial species using a carbon-based ph microsensor as a scanning electrochemical microscopy probe. Anal Chem 89: 11044-11052. https://doi.org/10.1021/acs.analchem.7b03050 ![]() |
[69] |
Park S, Kumar S, Maier CS, et al. (2023) Simultaneous chemical mapping of live biofilm microenvironmental ph and hydrogen peroxide in real time with a triple scanning electrochemical microscopy tip. Anal Chem 95: 6332-6340. https://doi.org/10.1021/acs.analchem.2c05258 ![]() |
[70] |
Dige I, Schlafer S, Nyvad B (2012) Difference in initial dental biofilm accumulation between night and day. Acta Odontol Scand 70: 441-447. https://doi.org/10.3109/00016357.2011.634833 ![]() |
[71] |
Dige I, Nyengaard JR, Kilian M, et al. (2009) Application of stereological principles for quantification of bacteria in intact dental biofilms. Oral Microbiol Immunol 24: 69-75. https://doi.org/10.1111/j.1399-302X.2008.00482.x ![]() |
[72] | Ding H, Yang Y, Li X, et al. (2022) A simple AI-enabled method for quantifying bacterial adhesion on dental materials. Biomater Investig Dent 9: 75-83. https://doi.org/10.1080/26415275.2022.2114479 |
[73] |
Mironov AA, Beznoussenko GV (2013) Correlative microscopy. Methods Cell Biol 113: 209-255. https://doi.org/10.1016/B978-0-12-407239-8.00011-2 ![]() |
[74] |
Howes SC, Koning RI, Koster AJ (2018) Correlative microscopy for structural microbiology. Curr Opin Microbiol 43: 132-138. https://doi.org/10.1016/j.mib.2018.01.009 ![]() |
[75] |
Lange F, Agüi-Gonzalez P, Riedel D, et al. (2021) Correlative fluorescence microscopy, transmission electron microscopy and secondary ion mass spectrometry (CLEM-SIMS) for cellular imaging. PLoS One 16: e0240768. https://doi.org/10.1371/journal.pone.0240768 ![]() |
[76] |
Daddi Oubekka S, Briandet R, Fontaine-Aupart MP, et al. (2012) Correlative time-resolved fluorescence microscopy to assess antibiotic diffusion-reaction in biofilms. Antimicrob Agents Chemother 56: 3349-58. https://doi.org/10.1128/AAC.00216-12 ![]() |
1. | Felix Thol, Felix Benjamin Warwas, Nikolai Spuck, Franz-Josef Kramer, Nils Heim, Microbial spectrum and resistance of odontogenic abscesses - microbiological analysis using next generation sequencing, 2024, 29, 1436-3771, 10.1007/s00784-024-06097-0 | |
2. | Tasha Octaricha, Cimi Ilmiawati, Nila Kasuma, Salivary microbiome profile shifts after scaling in stunted children, 2025, 18, 1756-0500, 10.1186/s13104-025-07147-w | |
3. | Talita Tartari, Carlos Estrela, Larissa Barbosa Borges de Araújo, Márcia Sirlene Zardin Graeff, Flaviana Bombarda de Andrade, Marco Antonio Hungaro Duarte, Use of confocal laser scanning microscopy to evaluate the metal ion removal and destabilization of Enterococcus faecalis biofilms by EDTA and etidronic acid, 2025, 1618-1247, 10.1007/s10266-025-01082-9 | |
4. | Gianluca Botticelli, Giovanni Falisi, Sofia Rastelli, Enzo Iacomino, Angelo Bruni, Davide Gerardi, Giuseppe Di Fabio, Marco Severino, Sara Bernardi, A Morphological Evaluation of the Antibiofilm Activity on an Implant Surface Using a New Electric Device: An In Vitro Study, 2025, 13, 2304-6767, 140, 10.3390/dj13040140 |
Chemical Forces | Description of their actions and roles in biofilm formation |
Van der Waals forces | Weak attractive forces between molecules, including those present on bacterial surfaces, playing a key role in the initial adhesion of bacteria to tooth surfaces [8]. |
Hydrogen bonds | Forces between molecules with hydrogen and electronegative atoms. Regarding the oral biofilm, hydrogen bonding can occur between water molecules, proteins, and other biomolecules, influencing the structure and stability of the biofilm [8]. |
Ionic interactions | Electrostatic interactions between charged molecules or groups which can affect bacterial adhesion. The charged molecules on bacterial and tooth surfaces can lead to attractive or repulsive forces [9]. |
Quorum sensing | A chemical communication system used by bacteria to coordinate gene expression based on population density. Quorum sensing allows bacteria within the biofilm to respond collectively to environmental changes and regulate biofilm formation [10]. |
Physical Forces | Description of their actions and roles in biofilm formation |
Brownian motion | The random motion of particles, such as bacteria, due to thermal energy contributing to bacteria transport within the oral environment and colliding with tooth surfaces [11]. |
Surface tension | The tendency of the surface of a liquid to resist external forces. In the oral environment, the surface tension of saliva can influence the spreading and wetting of oral biofilm on tooth surfaces [12]. |
Adhesion and cohesion forces | Forces contributing to the structural integrity of the biofilm. Adhesion forces refer to the attachment of bacteria to tooth surfaces or other structures, while the cohesion ones involve bacteria binding to each other within the biofilm [13]. |
Supragingival | Plaque on the tongue | Subgingival |
· Actinomyes | · Prevotella | - The outside layer: Treponemes |
· Aggregatibacter | · Rothia | |
· Fusobacterium | · Neisseria | - The first layer (on the top of the biofilm): |
· Leptotrichia | · Veillonella | · Actinomyces species |
· Capnocytophaga | · Porphyromonas | · Bacteria belonging to the Cytophaga-Flavobacterium-Bacteroides cluster |
· Corynebacterium | · Granulicatella | |
· Lautropia | · Alloprevotella | - The intermediate layer: |
· Campylobacter Tannerella | · Rothia | · F. nucleatum |
· T. forsythia | ||
· Bacteroidetes | ||
· Prevotella species | ||
· Tannerella species | ||
- The fourth layer: | ||
· Spirochaetes and other bacterial aggregates |
Chemical Forces | Description of their actions and roles in biofilm formation |
Van der Waals forces | Weak attractive forces between molecules, including those present on bacterial surfaces, playing a key role in the initial adhesion of bacteria to tooth surfaces [8]. |
Hydrogen bonds | Forces between molecules with hydrogen and electronegative atoms. Regarding the oral biofilm, hydrogen bonding can occur between water molecules, proteins, and other biomolecules, influencing the structure and stability of the biofilm [8]. |
Ionic interactions | Electrostatic interactions between charged molecules or groups which can affect bacterial adhesion. The charged molecules on bacterial and tooth surfaces can lead to attractive or repulsive forces [9]. |
Quorum sensing | A chemical communication system used by bacteria to coordinate gene expression based on population density. Quorum sensing allows bacteria within the biofilm to respond collectively to environmental changes and regulate biofilm formation [10]. |
Physical Forces | Description of their actions and roles in biofilm formation |
Brownian motion | The random motion of particles, such as bacteria, due to thermal energy contributing to bacteria transport within the oral environment and colliding with tooth surfaces [11]. |
Surface tension | The tendency of the surface of a liquid to resist external forces. In the oral environment, the surface tension of saliva can influence the spreading and wetting of oral biofilm on tooth surfaces [12]. |
Adhesion and cohesion forces | Forces contributing to the structural integrity of the biofilm. Adhesion forces refer to the attachment of bacteria to tooth surfaces or other structures, while the cohesion ones involve bacteria binding to each other within the biofilm [13]. |
Supragingival | Plaque on the tongue | Subgingival |
· Actinomyes | · Prevotella | - The outside layer: Treponemes |
· Aggregatibacter | · Rothia | |
· Fusobacterium | · Neisseria | - The first layer (on the top of the biofilm): |
· Leptotrichia | · Veillonella | · Actinomyces species |
· Capnocytophaga | · Porphyromonas | · Bacteria belonging to the Cytophaga-Flavobacterium-Bacteroides cluster |
· Corynebacterium | · Granulicatella | |
· Lautropia | · Alloprevotella | - The intermediate layer: |
· Campylobacter Tannerella | · Rothia | · F. nucleatum |
· T. forsythia | ||
· Bacteroidetes | ||
· Prevotella species | ||
· Tannerella species | ||
- The fourth layer: | ||
· Spirochaetes and other bacterial aggregates |