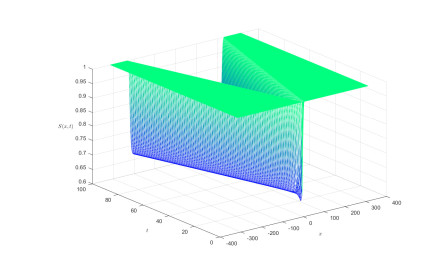
Arithmetic optimization algorithm (AOA) is a newly proposed meta-heuristic method which is inspired by the arithmetic operators in mathematics. However, the AOA has the weaknesses of insufficient exploration capability and is likely to fall into local optima. To improve the searching quality of original AOA, this paper presents an improved AOA (IAOA) integrated with proposed forced switching mechanism (FSM). The enhanced algorithm uses the random math optimizer probability (RMOP) to increase the population diversity for better global search. And then the forced switching mechanism is introduced into the AOA to help the search agents jump out of the local optima. When the search agents cannot find better positions within a certain number of iterations, the proposed FSM will make them conduct the exploratory behavior. Thus the cases of being trapped into local optima can be avoided effectively. The proposed IAOA is extensively tested by twenty-three classical benchmark functions and ten CEC2020 test functions and compared with the AOA and other well-known optimization algorithms. The experimental results show that the proposed algorithm is superior to other comparative algorithms on most of the test functions. Furthermore, the test results of two training problems of multi-layer perceptron (MLP) and three classical engineering design problems also indicate that the proposed IAOA is highly effective when dealing with real-world problems.
Citation: Rong Zheng, Heming Jia, Laith Abualigah, Qingxin Liu, Shuang Wang. An improved arithmetic optimization algorithm with forced switching mechanism for global optimization problems[J]. Mathematical Biosciences and Engineering, 2022, 19(1): 473-512. doi: 10.3934/mbe.2022023
[1] | Sarafa A. Iyaniwura, Musa Rabiu, Jummy F. David, Jude D. Kong . Assessing the impact of adherence to Non-pharmaceutical interventions and indirect transmission on the dynamics of COVID-19: a mathematical modelling study. Mathematical Biosciences and Engineering, 2021, 18(6): 8905-8932. doi: 10.3934/mbe.2021439 |
[2] | Seyedeh Nazanin Khatami, Chaitra Gopalappa . Deep reinforcement learning framework for controlling infectious disease outbreaks in the context of multi-jurisdictions. Mathematical Biosciences and Engineering, 2023, 20(8): 14306-14326. doi: 10.3934/mbe.2023640 |
[3] | Gabriel McCarthy, Hana M. Dobrovolny . Determining the best mathematical model for implementation of non-pharmaceutical interventions. Mathematical Biosciences and Engineering, 2025, 22(3): 700-724. doi: 10.3934/mbe.2025026 |
[4] | Zi Sang, Zhipeng Qiu, Xiefei Yan, Yun Zou . Assessing the effect of non-pharmaceutical interventions on containing an emerging disease. Mathematical Biosciences and Engineering, 2012, 9(1): 147-164. doi: 10.3934/mbe.2012.9.147 |
[5] | Luyu Zhang, Zhaohua Zhang, Sen Pei, Qing Gao, Wei Chen . Quantifying the presymptomatic transmission of COVID-19 in the USA. Mathematical Biosciences and Engineering, 2024, 21(1): 861-883. doi: 10.3934/mbe.2024036 |
[6] | M. C. J. Bootsma, K. M. D. Chan, O. Diekmann, H. Inaba . Separable mixing: The general formulation and a particular example focusing on mask efficiency. Mathematical Biosciences and Engineering, 2023, 20(10): 17661-17671. doi: 10.3934/mbe.2023785 |
[7] | Rhiannon Loster, Sarah Smook, Lia Humphrey, David Lyver, Zahra Mohammadi, Edward W. Thommes, Monica G. Cojocaru . Behaviour quantification of public health policy adoption - the case of non-pharmaceutical measures during COVID-19. Mathematical Biosciences and Engineering, 2025, 22(4): 920-942. doi: 10.3934/mbe.2025033 |
[8] | Stefanie Fuderer, Christina Kuttler, Michael Hoelscher, Ludwig Christian Hinske, Noemi Castelletti . Data suggested hospitalization as critical indicator of the severity of the COVID-19 pandemic, even at its early stages. Mathematical Biosciences and Engineering, 2023, 20(6): 10304-10338. doi: 10.3934/mbe.2023452 |
[9] | Fulian Yin, Xinyi Tang, Tongyu Liang, Yanjing Huang, Jianhong Wu . External intervention model with direct and indirect propagation behaviors on social media platforms. Mathematical Biosciences and Engineering, 2022, 19(11): 11380-11398. doi: 10.3934/mbe.2022530 |
[10] | Bruno Buonomo, Eleonora Messina . Impact of vaccine arrival on the optimal control of a newly emerging infectious disease: A theoretical study. Mathematical Biosciences and Engineering, 2012, 9(3): 539-552. doi: 10.3934/mbe.2012.9.539 |
Arithmetic optimization algorithm (AOA) is a newly proposed meta-heuristic method which is inspired by the arithmetic operators in mathematics. However, the AOA has the weaknesses of insufficient exploration capability and is likely to fall into local optima. To improve the searching quality of original AOA, this paper presents an improved AOA (IAOA) integrated with proposed forced switching mechanism (FSM). The enhanced algorithm uses the random math optimizer probability (RMOP) to increase the population diversity for better global search. And then the forced switching mechanism is introduced into the AOA to help the search agents jump out of the local optima. When the search agents cannot find better positions within a certain number of iterations, the proposed FSM will make them conduct the exploratory behavior. Thus the cases of being trapped into local optima can be avoided effectively. The proposed IAOA is extensively tested by twenty-three classical benchmark functions and ten CEC2020 test functions and compared with the AOA and other well-known optimization algorithms. The experimental results show that the proposed algorithm is superior to other comparative algorithms on most of the test functions. Furthermore, the test results of two training problems of multi-layer perceptron (MLP) and three classical engineering design problems also indicate that the proposed IAOA is highly effective when dealing with real-world problems.
The spreading of communicable diseases is affected by many factors including infectious agents, modes of contact, and latent periods. Since different diseases may have distinctive features of infection, it is necessary to model the evolutionary of various diseases by different mathematical models. Following the pioneer work of Kermack and McKendrick [1], the total population is often classified into the susceptible (S), the infective (I), and the recovered (R), and the corresponding models are called SIR models. The contact pattern between the susceptible and the infective is often described by the incidence function. Nonlinear incidence functions have been utilized in many epidemic systems including the following SIR model [2]
{˙S(t)=μ−h(S(t))g(I(t))−μS(t),˙I(t)=h(S(t))g(I(t))−(δ+μ)I(t),˙R(t)=δI(t)−μR(t), | (1.1) |
in which all the parameters are positive and h,g:R+→R+ describe the contact pattern, μ represents the constant death ratio and death rate and the constant recruitment rate. Here, the nonlinear incidence is partly due to the effect of media, government behavior. For some typical examples of h,g and their biological backgrounds, we may refer to [2]. Clearly, some properties of this system can be obtained by only investigating S,I.
In epidemic models, time delay may reflect that a susceptible individual was attacked by the pathogen (by contacting an infective) at an earlier time but becomes infective after several days, which may be reflected by delayed effect of the infective. Since an ostensible susceptible may be infected one that may transmit the pathogen to other true susceptible, the delayed effect must be involved in some cases. When the delayed effect on latent periods is concerned in (1.1), Huang et al. [3] studied the following model
{˙S(t)=μ−h(S(t))g(I(t−τ))−μS(t),˙I(t)=h(S(t))g(I(t−τ))−(σ+μ)I(t),˙R(t)=σI(t)−μR(t), |
in which all the parameters are positive, τ reflects the latent period. By comparing [2,3], time delay may be harmless to the stability of the system but decrease the basic reproduction ratio, which indicates the nontrivial role of time delay. Moreover, the latent periods may be different, and distributed delays may be useful, see a model of hematopoiesis by Adimy et al. [4]. In particular, by assuming that each individual of the susceptible class has the same probability being infected, h(u) is in proportion to u in many models in the above works.
Over a period of time there has been a growing awareness of the importance that includes a spatial aspect in constructing realistic models of biological systems, with a consequent development of both approximate and mathematically rigorous methods of analysis [5]. It is important to characterize the speed of spatial expansion of diseases [6,7,8], and the expansion speed may be a constant [9,Chapter 13]. In literature, many reaction-diffusion systems have been established to reflect the process [5]. Due to the deficiency of monotone semiflows in many epidemic models, the long time behavior of the initial value problems of epidemic models can not be studied by the theory of monotone semiflows [10], we may refer to Ducrot [11,12].
When both the spatial factor and delayed effect are involved in mathematical epidemiology, one important factor is the effect of nonlocal delays [13,14]. Here the nonlocal delay may reflect the history movement ability of the infective, which includes the cases of discrete delays and distributed delays [15,16]. De Mottoni et al. [17] studied the following model with spatial nonlocality
{∂S∂t=ΔS+μ−σS(x,t)−S(x,t)∫ΩI(y,t)K(x,y)dy∂I∂t=dΔI+S(x,t)∫ΩI(y,t)K(x,y)dy−γI(x,t), |
in which all the parameters are positive, Ω is the spatial domain, K is a nonnegative function. For more epidemic models with nonlocal delays, we refer to Ruan [16]. The purpose of this paper is to study diffusive epidemic models with nonlocal delays, nonlinear incidence rate and constant recruitment rate, during which the effect of time delay and spatial nonlocality will be also presented.
For simplicity, we firstly consider the initial value problem after scaling
{∂S(x,t)∂t=d∂2S(x,t)∂x2+σ−σS(x,t)−βS(x,t)(J∗I)(x,t)1+α(J∗I)(x,t),∂I(x,t)∂t=∂2I(x,t)∂x2+βS(x,t)(J∗I)(x,t)1+α(J∗I)(x,t)−(μ+γ)I(x,t),S(x,0)=1,I(x,s)=ϕ(x,s) | (1.2) |
with
(J∗I)(x,t)=∫∞0∫RJ(y,s)I(x−y,t−s)dyds, |
where x∈R,t>0,s∈(−∞,0]. In model (1.2), S(x,t), I(x,t) denote the densities of susceptible and infective individuals at time t and location x, respectively, d>0 is a constant describing the spatial diffusive motility of the susceptible, σ>0 represents the entering flux as well as the death rate of the individuals, μ is the death rates of infective, β>0 reflects the infection rate, γ>0 is the recovery rate of the infective individuals, and the positive constant α measures the saturation level, ϕ(x,s)≥0 is a continuous bounded function. The nonlocal delayed term βS(x,t)(J∗I)(x,t)1+α(J∗I)(x,t) describes the interaction between the infected individuals at an earlier time t−s at location y and susceptible individuals at location x at time t, which implies that only the susceptible individuals at location x at the present time t affect the change rate of the susceptible class although the contact leading to infection maybe occurred at location y at an earlier time t−s.
Clearly, model (1.2) is a subsystem of the following SIR model
{∂S(x,t)∂t=d∂2S(x,t)∂x2+σ−σS(x,t)−βS(x,t)(J∗I)(x,t)1+α(J∗I)(x,t),∂I(x,t)∂t=∂2I(x,t)∂x2+βS(x,t)(J∗I)(x,t)1+α(J∗I)(x,t)−(μ+γ)I(x,t),∂R(x,t)∂t=d3∂2R(x,t)∂x2+γI(x,t)−μ1R(x,t), |
where R denotes the removed individuals, d3>0,μ1>0 are constants on spatial diffusion ratio and death rate of the removed, respectively. Clearly, after investigating (1.2), some properties of the class R can be obtained (see. e.g., Li et al. [18,Section 5]) since
R(x,t)=e−μ1t√4πd3t∫Re−(x−y)24d3tR(y,0)dy+∫t0e−μ1(t−s)√4πd3(t−s)ds∫Re−(x−y)24d3(t−s)I(y,s)dy |
for any t>0,x∈R, and we only study system (1.2) in this paper. These systems may model the evolutionary of the epidemic with nonlinear incidence rate and constant recruitment rate. For the dynamics in the corresponding functional differential equations with discrete or distribute delay, we may refer to Enatsu et al. [19], Huang et al. [3], in which the persistence and the extinction of I were studied. In particular, by [3], we may find that the so-called basic reproduction ratio is βμ+γ.
Recently, traveling wave solutions of different versions for (1.2) have been studied [18,20,21], in which these solutions take the form
S(x,t)=S(ξ),I(x,t)=I(ξ),ξ=x+ct∈R |
for some c>0 and S,I satisfy
limξ→−∞(S(ξ),I(ξ))=(1,0),lim infξ→∞S(ξ)>0,lim infξ→∞I(ξ)>0. |
By letting ξ=x+ct, such a solution indicates that at any fixed location x∈R, there was no infective long time ago (ξ→−∞ iff t→−∞) while the infective and susceptible will coexist eventually (ξ→∞ iff t→∞). However, from the viewpoint of initial value problems, these traveling wave solutions formulate that the infective individuals live in a habitat of infinite size at any fixed time. This contradicts to the outbreak of many diseases, during which the initial habitat of the infective individuals is finite, see a similar process in biological invasion by [22,23,24]. Motivated by this, we will study the corresponding initial value problem of (1.2) by the following index [25].
Definition 1.1. Assume that u(x,t) is a nonnegative function for x∈R,t>0. Then c′ is called the spreading speed of u(x,t) if
a) limt→∞sup|x|>(c′+ϵ)tu(x,t)=0 for any given ϵ>0;
b) lim inft→∞inf|x|<(c′−ϵ)tu(x,t)>0 for any given ϵ∈(0,c′).
The spreading speeds of unknown functions governed by reaction-diffusion equations and other parabolic type systems have been widely studied since [25], and there are some important results for monotone semiflows [10,26,27,28,29] and nonmonotone scalar equations [30,31,32]. For noncooperative systems, Ducrot [11] estimated the asymptotic spreading in a predator-prey system of Holling-Tanner type, Ducrot [12] considered the spatial propagation of an epidemic model with recruitment rate and bilinear incidence rate, Lin et al. [33,34] studied the spreading speeds of two competitive invaders in competitive systems, Lin and Wang [35] and Pan [36] investigated the invasion speed of predators in a predator-prey system of Lotka-Volterra type. For coupled systems in [11,12,33,34,35,36], the comparison principle plays the crucial role.
In this paper, to focus on our main idea, we first study (1.2) by estimating the limit behavior of I(x,t) motivated by [35,36]. With the uniform boundedness of S,I, we try to estimate S, by which we may obtain an auxiliary equation on I(x,t). However, this equation involves the nonlocal delay [14,15,16,37] and does not generate monotone semiflows. Further by the smoothness of I(x,t), we obtain an auxiliary equation with quasimonotonicity, of which the spreading speed has been established. Then we obtain the spreading speed of I(x,t). Here, the spreading speed is coincident with the minimal wave speed of traveling wave solutions in some well studied cases. For example, when it just involves discrete delays, the spreading speed in this paper equals the minimal wave speed of traveling wave solutions in Li et al. [18].
Subsequently, we study the general model
{∂S(x,t)∂t=d∂2S(x,t)∂x2+σ−σS(x,t)−S(x,t)f((J∗I)(x,t)),∂I(x,t)∂t=∂2I(x,t)∂x2+S(x,t)f((J∗I)(x,t))−(μ+γ)I(x,t),S(x,0)=1,I(x,s)=ϕ(x,s), | (1.3) |
where x∈R,t>0,s∈(−∞,0],f:[0,+∞)→[0,+∞) is Lipscitz continuous. We also obtain the spreading speed of I(x,t) even if f is not monotone, and the convergence of (S,I) is established from the viewpoint of asymptotic spreading. Furthermore, to show the effect of time delay and spatial nonlocality and estimate the spreading speeds, we present some numerical examples.
The rest of this paper is organized as follows. In Section 2, we show some necessary preliminaries on reaction-diffusion systems with nonlocal delays. Section 3 is concerned with the spreading speed of I(x,t) in (1.2). We then consider the spreading speed in the general model (1.3) in Section 4, and show the convergence result of I(x,t). To further illustrate our analysis results, we present some numerical results in Section 5. Finally, we provide a discussion on the epidemic backgrounds and mathematical conclusions.
In this section, we introduce some concepts and review some relevant results. Firstly, when a partial order in R2 is concerned, it is the standard partial order in R2. That is, if
u=(u1,u2),v=(v1,v2)∈R2, |
then u≤v iff u1≤v1,u2≤v2. For the kernel function J that is integrable, we make the following assumptions.
(J1) J(y,s)=J(−y,s)≥0,∫∞0∫RJ(y,s)dyds=1,y∈R,s≥0;
(J2) for each fixed c>0, there exists λc∈(0,∞] such that
∫∞0∫RJ(y,s)eλ(y−cs)dyds<∞ for all λ∈(0,λc). |
For f, we make the following assumptions.
(f) There exists I′>0 such that f(I)<(μ+γ)I,I>I′.f:[0,∞)→R+ is C1. f′(0)>μ+γ, and there exist positive constants L,α such that
f′(0)I−LI1+α≤f(I)≤f′(0)I,I∈[0,I′]. |
Besides by taking
f(I)=βI1+αI |
as in (1.2), there are also many other functions satisfying (f), e.g., the following function
f(I)=βI1+αIγ, |
where γ>0. By taking different parameters, it may be nonmonotone, the corresponding kinetic model was proposed and analyzed by Liu et al. [38], Xiao and Ruan [39]. By the above assumptions, we define
Λ(λ,c)=λ2−cλ+f′(0)∫∞0∫RJ(y,s)eλ(y−cs)dyds−(μ+γ) |
for each fixed c>0 with λ∈[0,λc). From (J1)-(J2) and (f), Λ(λ,c) satisfies the following property.
Lemma 2.1. There exists a constant c∗>0 such that
(R1) Λ(λ,c)=0 has no real roots if c<c∗,
(R2) if c≥c∗, then Λ(λ,c)=0 has positive real roots.
Evidently, we see that time delay and spatial nonlocality may affect the threshold c∗, a similar conclusion was reported by Li et al. [40] (we may refer to a recent paper by Li and Pan [41] and references cited therein for the traveling wave solutions of delayed models). To further quantificationally illustrate the role, we first consider the case of discrete delay such that
(J∗I)(x,t)=I(x,t−τ), |
and
Λ(λ,c)=λ2−cλ+f′(0)e−λcτ−(μ+γ), |
in which τ>0. Let c∗(τ) be the threshold depending on τ, then we have
c∗(τ1)<c∗(τ2) iff τ1>τ2>0 |
and
limτ→∞c∗(τ)=0. |
That is, the time delay may decrease the threshold, we also refer to Zou [42]. On the other hand, to quantificationally show the effect of spatial nonlocality, we consider a simple nonlocal case with
(J∗I)(x,t)=I(x+l,t)+I(x−l,t)2,l≥0, |
and so
Λ(λ,c)=λ2−cλ+f′(0)[eλl+e−λl]/2−(μ+γ). |
Let c∗(l) be the threshold depending on l>0, then
c∗(l1)<c∗(l2) iff l2>l1≥0 |
and
liml→∞c∗(l)=∞. |
For this type of kernels, we say the nonlocality is stronger if l>0 is larger, so the spatial nonlocality may increase the threshold, which will be further illustrated by numerical simulations.
Let
(T1(t)v(⋅,r))(x)=1√4πdt∫Re−y24dtv(x−y,r)dy,(T2(t)v(⋅,r))(x)=1√4πt∫Re−y24tv(x−y,r)dy |
for any bounded and continuous function v(x,t),x∈R,t>0. Then by the theory of abstract functional differential equations with application to delayed reaction-diffusion systems [43,44,45,46], we have the following existence and uniqueness of mild solutions.
Lemma 2.2. (1.3) admits a mild solution (S(x,t),I(x,t)),x∈R,t>0 such that
S(x,t)>0,I(x,t)>0,x∈R,t>0, |
and (S(x,t),I(x,t)) takes the form
S(x,t)=(T1(t−r)S(⋅,r))(x)+∫tr[T1(t−s)[σ−σS(⋅,s)−S(⋅,s)f((J∗I)(⋅,s))]]ds(x),I(x,t)=(T2(t−r)I(⋅,r))(x)+∫tr[T2(t−s)[S(⋅,s)f((J∗I)(⋅,s))−(μ+γ)I(⋅,s)]]ds(x) |
for x∈R and any given r∈[0,t). If t>1, then S(x,t),I(x,t) are uniformly continuous in x∈R,t>1. In particular, if ϕ(x,0) admits nonempty compact support in (1.2), then
0<S(x,t)<1,0<I(x,t)<max{supx∈R,s≤0ϕ(x,s),β−(μ+γ)α(μ+γ)}:=¯I,x∈R,t>0. |
Moreover, if τ>0 such that
∫τ0∫RJ(y,s)dyds=1, |
then t>τ implies that (S,I) is the classical solution of (1.3) in the sense of partial derivatives.
The local existence of mild solutions is ensured by the theory of analytic semigroups as well as abstract functional differential equations [43,47], and the global existence is guaranteed due to the existence of positive invariant regions because of the existence of I′.
In addition, the proof depends on the comparison principle and asymptotic spreading of the following equation with nonlocal delays
{∂u(x,t)∂t=∂2u(x,t)∂x2+f((J∗u)(x,t))−ϵu2(x,t)−(μ+γ)u(x,t),u(x,s)=φ(x,s), | (2.1) |
where ϵ≥0, all the other parameters are the same as those in (f) and φ(x,s) is a positive continuous function satisfying
φ(x,s)=0,|x|>L,s≤0 |
for some L>0. By results in Martin and Smith [47], Ruan and Wu [43], (2.1) satisfies the following comparison principle.
Lemma 2.3. Let T≤∞ hold and f be nondecreasing. Assume that a continuous function ¯u(x,t):R×(−∞,T)→R+ satisfies
¯u(x,s)≥φ(x,s),x∈R,s≤0, |
and
¯u(x,t)≥(T2(t−r)¯u(⋅,r))(x)+∫tr[T2(t−s)[f((J∗¯u)(⋅,s))−ϵ¯u2(⋅,s)−(μ+γ)¯u(⋅,s)]]ds(x) |
for x∈R and any given r∈[0,t). Then
¯u(x,t)≥u(x,t),x∈R,t∈(0,T). |
Furthermore, results by Liang and Zhao [10], Yi et al. [48] imply the following spreading property even if f(u) is not monotone for all u>0.
Lemma 2.4. Let u(x,t) be the unique mild solution of (2.1) with φ(x,0)>0 for some x∈R. Then for any given c>c∗,u(x,t) satisfies limt→∞,|x|>ctu(x,t)=0, and for any given c∈(0,c∗),u(x,t) satisfies lim inft→∞inf|x|<ctu(x,t)>0.
In this section, we establish the main results of (1.2). In particular, we take f′(0)=β in this section, ϕ(x,0) admits nonempty compact support and
ϕ(x,s)=0,|x|>L,s≤0 | (3.1) |
with some L>0. The following is the main result of this section.
Theorem 3.1. Assume that I(x,t) is defined by (1.2). Then for any given c>c∗,I(x,t) satisfies
limt→∞,|x|>ctI(x,t)=0, | (3.2) |
and for any given c∈(0,c∗),I(x,t) satisfies
lim inft→∞inf|x|<ctI(x,t)>0. | (3.3) |
Proof. By Lemma 2.2, we have
S(x,t)≤1,x∈R,t>0, |
which implies that
I(x,t)≤(T2(t−r)I(⋅,r))(x)+∫tr[T2(t−s)[β(J∗I)(⋅,s)1+α(J∗I)(⋅,s)−(μ+γ)I(⋅,s)]]ds(x) | (3.4) |
for all x∈R,r∈[0,t). Further by Lemmas 2.3 and 2.4, we obtain (3.2).
We now prove (3.3) for any fixed c∈(0,c∗). Since it involves long time behavior, we assume that t>1 is large and (S(x,t),I(x,t)) is uniformly continuous in x∈R,t>1. Define S(x,t)=1−S(x,t), then Lemma 2.2 indicates that
S(x,0)=0,0<S(x,t)<1,x∈R,t>0. |
Therefore, if S is smooth enough, then it satisfies
∂S(x,t)∂t=d∂2S(x,t)∂x2−σS(x,t)+β(1−S(x,t))(J∗I)(x,t)1+α(J∗I)(x,t)≤d∂2S(x,t)∂x2−σS(x,t)+β(J∗I)(x,t)1+α(J∗I)(x,t)≤d∂2S(x,t)∂x2−σS(x,t)+β(J∗I)(x,t) |
and similarly, we can verify that
S(x,t)≤(T(t)S(⋅,0))(x)+β∫t0[T(t−r)(J∗I)(⋅,r)]dr(x)=β∫t0[T(t−r)(J∗I)(⋅,r)]dr(x), |
where
(T(t)v(⋅,r))(x)=e−σt√4πdt∫Re−y24dtv(x−y,r)dy,t>0 |
for any bounded and continuous function v(x,r),x∈R,r>0.
Returning to the equation of I(x,t), we have
I(x,t)=(T2(t−r)I(⋅,r))(x)+∫tr[T2(t−s)[βS(⋅,s)(J∗I)(⋅,s)1+α(J∗I)(⋅,s)−(μ+γ)I(⋅,s)]]ds(x)=(T2(t−r)I(⋅,r))(x)+∫tr[T2(t−s)[β(1−S(⋅,s))(J∗I)(⋅,s)1+α(J∗I)(⋅,s)−(μ+γ)I(⋅,s)]]ds(x)=(T2(t−r)I(⋅,r))(x)+∫tr[T2(t−s)[β(J∗I)(⋅,s)1+α(J∗I)(⋅,s)−(μ+γ)I(⋅,s)]]ds(x)−β∫tr[T2(t−s)[S(⋅,s)(J∗I)(⋅,s)1+α(J∗I)(⋅,s)]]ds(x)≥(T2(t−r)I(⋅,r))(x)+∫tr[T2(t−s)[β(J∗I)(⋅,s)1+α(J∗I)(⋅,s)−(μ+γ)I(⋅,s)]]ds(x)−β∫tr[T2(t−s)[S(⋅,s)(J∗I)(⋅,s)]]ds(x)≥(T2(t−r)I(⋅,r))(x)+∫tr[T2(t−s)[β(J∗I)(⋅,s)1+α(J∗I)(⋅,s)−(μ+γ)I(⋅,s)]]ds(x)−β2∫tr[T2(t−s)[(J∗I)(⋅,s)v(⋅,s)]]ds(x) |
with
v(x,s)=∫s0[T(t−r)(J∗I)(⋅,r)]dr(x). |
In what follows, we prove the following claim.
(C) For any ϵ∈(0,β), there exists M(ϵ)>0 such that
I(x,t)≥(T2(t−r)I(⋅,r))(x)+∫tr[T2(t−s)[(β−ϵ)(J∗I)(⋅,s)1+α(J∗I)(⋅,s)−M2I(⋅,s)−(μ+γ)I(⋅,s)]]ds(x) | (3.5) |
if t>1 is large.
From Lemmas 2.3 and 2.4, (C) implies that
lim inft→∞inf|x|<ctI(x,t)>0. |
by selecting and fixing ϵ>0 small enough, which implies what we wanted.
Subsequently, we verify (3.5) for any fixed ϵ>0. If
β2∫t0[T(t−r)I(⋅,r)]dr(x)≤ϵ, |
then (3.5) is true. Otherwise, let x∈R and t>1 be large enough such that
β2∫t0[T(t−r)I(⋅,r)]dr(x)>ϵ. | (3.6) |
Firstly, we fix T>1 such that
β2∫t−T0e−σ(t−r)√4πd(t−r)∫Re−y24d(t−r)(J∗I)(x−y,r)dydr≤β2¯I∫t−T0e−σ(t−r)√4πd(t−r)∫Re−y24d(t−r)dydr=β2¯I∫t−T0e−σ(t−r)dr<ϵ6 for any t>T. |
For such a fixed T>1, we further select N>0 such that
β2∫tt−Te−σ(t−r)√4πd(t−r)[∫−N−∞+∫∞N]e−y24d(t−r)(J∗I)(x−y,r)dydr≤β2¯I∫tt−Te−σ(t−r)√4πd(t−r)[∫−N−∞+∫∞N]e−y24d(t−r)dydr≤β2¯I∫tt−Te−σ(t−r)√4πd(t−r)[∫−N−∞+∫∞N]e−y24dTdydr<ϵ6 for any t>T, |
which is admissible since
∫∞0e−σt√4πdtdt<∞,limN→∞[∫−N−∞+∫∞N]e−y24dTdy=0. |
Further choose sufficiently small δ>0 such that
β2∫tt−δe−σ(t−r)√4πd(t−r)∫Re−y24d(t−r)(J∗I)(x−y,r)dydr≤β2¯I∫tt−δe−σ(t−r)√4πd(t−r)∫Re−y24d(t−r)dydr=β2¯I∫tt−δe−σ(t−r)dr<β2¯Iδ≤ϵ6. |
So we obtain
β2∫t0[T(t−r)(J∗I)(⋅,r)]dr(x)=β2∫t0e−σ(t−r)√4πd(t−r)∫Re−y24d(t−r)(J∗I)(x−y,r)dydr=β2∫tt−Te−σ(t−r)√4πd(t−r)∫Re−y24d(t−r)(J∗I)(x−y,r)dydr+β2∫t−T0e−σ(t−r)√4πd(t−r)∫Re−y24d(t−r)(J∗I)(x−y,r)dydr=β2∫t−δt−Te−σ(t−r)√4πd(t−r)[∫N−N+∫−N−∞+∫∞N]e−y24d(t−r)(J∗I)(x−y,r)dydr+β2[∫t−T0+∫tt−δ]e−σ(t−r)√4πd(t−r)∫Re−y24d(t−r)(J∗I)(x−y,r)dydr=β2∫t−δt−Te−σ(t−r)√4πd(t−r)∫N−Ne−y24d(t−r)(J∗I)(x−y,r)dydr+β2∫t−δt−Te−σ(t−r)√4πd(t−r)[∫−N−∞+∫∞N]e−y24d(t−r)(J∗I)(x−y,r)dydr+β2[∫t−T0+∫tt−δ]e−σ(t−r)√4πd(t−r)∫Re−y24d(t−r)(J∗I)(x−y,r)dydr≤β2∫t−δt−Te−σ(t−r)√4πd(t−r)∫N−Ne−y24d(t−r)(J∗I)(x−y,r)dydr+ϵ/2 |
for any t>T+δ+1, and (3.6) implies
β2∫t−δt−Te−σ(t−r)√4πd(t−r)∫N−Ne−y24d(t−r)(J∗I)(x−y,r)dydr>ϵ/2. | (3.7) |
The left side of (3.7) is
β2∫t−δt−Te−σ(t−r)√4πd(t−r)∫N−Ne−y24d(t−r)(J∗I)(x−y,r)dydr=β2∫t−δt−Te−σ(t−r)√4πd(t−r)∫N−Ne−y24d(t−r)[∫τ0∫RJ(z,s)I(x−y−z,t−s)dzds]dydr+β2∫t−δt−Te−σ(t−r)√4πd(t−r)∫N−Ne−y24d(t−r)[∫∞τ∫RJ(z,s)I(x−y−z,t−s)dzds]dydr=β2∫t−δt−Te−σ(t−r)√4πd(t−r)∫N−Ne−y24d(t−r)[∫τ0∫K−KJ(z,s)I(x−y−z,t−s)dzds]dydr+β2∫t−δt−Te−σ(t−r)√4πd(t−r)∫N−Ne−y24d(t−r)[∫τ0∫|z|≥KJ(z,s)I(x−y−z,t−s)dzds]dydr+β2∫t−δt−Te−σ(t−r)√4πd(t−r)∫N−Ne−y24d(t−r)[∫∞τ∫RJ(z,s)I(x−y−z,t−s)dzds]dydr |
for any τ>0,K>0. By the convergence in (J1) and (J2), if τ>0,K>0 (independent on x,t) are large enough, then
β2∫t−δt−Te−σ(t−r)√4πd(t−r)∫N−Ne−y24d(t−r)[∫τ0∫K−KJ(z,s)I(x−y−z,r−s)dzds]dydr>ϵ4, | (3.8) |
and we now fix constants τ,K.
Note that e−σ(t−r)√4πd(t−r),e−y24d(t−r) are uniformly continuous and uniformly bounded for r∈[t−T,t−δ],y∈[−N,N], then (3.8) implies that we can choose constants
λ′>0,x0−y0∈[x−N,x+N],r0∈[t−T,t−δ] |
such that
∫τ0∫K−KJ(z,s)I(x0−y0−z,r0−s)dzds>λ′, |
here λ′ depends on ϵ and is independent of x∈R,t≥T+1+τ. Again by the uniform continuity of I(x,t) and convergence of J, we fix λ,ι depending on λ′ such that
I(z0,s0)>λ |
for some
z0∈[x−N−K,x+N+K],s0∈[t−T−τ,t−δ], |
and the uniform continuity further implies that
I(x,s0)≥λ,x∈[z0−ι,z0+ι]. |
From the equation of I(x,t), we see that
I(x,t)≥(T2(t−r)I(⋅,r))(x)−(μ+γ)∫tr[T2(t−s)I(⋅,s)]ds(x) |
for any 0≤r<t. By the property of semigroup, we also have
I(x,t)≥e−(μ+γ)(t−s)√4π(t−s)∫Re−y24(t−s)I(y,s)dy |
for any s∈[0,t). So for any given s0≥0, if
|x−z0|<N+K+ι,t∈[s0+δ,s0+T+τ], |
then we have
I(x,t)≥e−(μ+γ)(t−s0)√4π(t−s0)∫Re−y24(t−s)I(y,s0)dy≥e−(μ+γ)(T+τ)√4π(T+τ)∫Re−y24δI(y,s0)dy≥e−(μ+γ)(T+τ)√4π(T+τ)∫N+K+ι−N−K−ιe−y24δI(y,s0)dy≥e−(μ+γ)(T+τ)√4π(T+τ)e−(N+K+ι)24δ∫N+K+ι−N−K−ιI(y,s0)dy≥2λιe−(μ+γ)(T+τ)√4π(T+τ)e−(N+K+ι)24δ. |
By what we have done, x,t satisfying (3.6) lead to
I(x,t)≥2λιe−(μ+γ)(T+τ)√4π(T+τ)e−(N+K+ι)24δ=:I_, |
where I_ is uniform for any x∈R,t≥T+τ+1 such that (3.6) holds. Again by the uniform boundedness of I(x,t) and
β2(J∗I)(x,t)1+α(J∗I)(x,t)∫t0[T(t−r)(J∗I)(⋅,r)]dr(x), |
we obtain the existence of M, which is also independent on x,t once (3.7) is true and t≥T+τ+1. The proof is complete.
Remark 3.2. From (3.4) and Lemma 2.4, we see that
f′(0)≤μ+γ |
implies
limt→∞supx∈RI(x,t)=0. |
That is, the sign of f′(0)−(μ+γ) determines the persistence and extinction of I, which is similar to the case in the corresponding functional differential systems. Therefore, the basic reproduction ratio of the corresponding functional differential systems may determine the failure or propagation of the disease, and also may affect the spreading speed if the ratio is larger than 1.
In this section, we first study the spreading speed and then estimate the convergence of (S,I) in (1.3), in which f satisfies (f). The first result on spreading speed is formulated as follows.
Theorem 4.1. c∗ is the spreading speed of I(x,t) if I(x,s) satisfies (3.1).
Proof. Due to the proof of Theorem 3.1, we just give a sketch. Firstly, we define
¯f(u)=supv∈[0,u]f(v),u∈[0,I′], |
then there exists v_1>0 such that
¯f(u)=f(u),u∈[0,v_1], |
and ¯f(u) is nondecreasing such that
¯f(u)≤f′(0)u,u∈[0,I′]. |
Therefore, I(x,t) satisfies
I(x,t)≤(T2(t−r)I(⋅,r))(x)+∫tr[T2(t−s)[¯f((J∗I)(⋅,s))−(μ+γ)I(⋅,s)]]ds(x) |
for any r∈[0,t). So for any given c>c∗,I(x,t) satisfies
limt→∞,|x|>ctI(x,t)=0. |
Further define
f_(u)=infv∈[u,I′]f(v),u∈[0,I′], |
then there exists v_2>0 such that
f_(u)=f(u),u∈[0,v_2], |
and
f_(u)≤f′(0)u,u∈[0,I′]. |
So I(x,t) satisfies
I(x,t)≥(T2(t−r)I(⋅,r))(x)+∫tr[T2(t−s)[S(⋅,s)f_((J∗I)(⋅,s))−(μ+γ)I(⋅,s)]]ds(x) |
for any r∈[0,t). Similar to that in Section 3, we obtain
lim inft→∞,|x|<ctI(x,t)>0 |
for any given c∈(0,c∗). The proof is complete.
Theorems 3.1 and 4.1 imply that the infective individuals successfully invade the habitat of the susceptible. We now estimate the limit behavior of S(x,t),I(x,t). Firstly, we consider (1.3) when S(x,0),I(x,s) satisfies
infx∈RS(x,0)>0,infx∈RI(x,0)>0, | (4.1) |
and make the following assumption.
(C) Assume that (4.1) holds. Then
limt→∞supx∈R(|S(x,t)−S∗|+|I(x,t)−I∗|)=0, |
where (S∗,I∗) is the unique positive spatially homogeneous steady state of (1.3).
Remark 4.2. In some cases, (C) is true. For example, if f is monotone such that f′(0)>μ+γ and (1.3) with (4.1) is persistent, further define
lim supt→∞supx∈RS(x,t)=¯S,lim inft→∞infx∈RS(x,t)=S_,lim supt→∞supx∈RI(x,t)=¯I,lim inft→∞infx∈RI(x,t)=I_, |
then the persistence implies that
¯S≥S_>0,¯I≥I_>0. |
By dominated convergence theorem, we have
σ−σ¯S−¯Sf(I_)≥0,¯Sf(¯I)−(μ+γ)¯I≥0,σ−σS_−S_f(¯I)≤0,S_f(I_)−(μ+γ)I_≤0. |
If these inequalities imply ¯S=S_,¯I=I_, then (C) is true by applying the dominated convergence theorem in the corresponding integral equations [18].
Now, we formulate the limit behavior of S(x,t),I(x,t) defined by (1.3) if I(x,0) satisfies (3.1).
Theorem 4.3. Assume that there exist τ≥0,K>0 such that
∫τ0∫K−KJ(z,s)dzds=1 |
and if τ>0, then
∫τ′0∫K−KJ(z,s)dzds<1 |
for any τ′∈(0,τ). If (C) holds, then
lim supt→∞sup|x|<ct(|S(x,t)−S∗|+|I(x,t)−I∗|)=0, |
which holds for any given c∈(0,c∗).
Proof. We prove that for any given c∈(0,c∗),ϵ>0, there exists T>0 such that
sup|x|<ct(|S(x,t)−S∗|+|I(x,t)−I∗|)<ϵ,t>T | (4.2) |
by the idea in [49]. Firstly, by Theorem 4.1, there exist δ>0,T1>0 such that
inf|x|<(c+2c∗)t/3S(x,t)>δ,inf|x|<(c+2c∗)t/3I(x,t)>δ,t>T1. |
Then (4.2) is true if we can prove that for any T2>T1 large enough, there exists T3>0 independent on T2 such that
sup|x|<(2c+c∗)(t+T3+1)/3(|S(x,t)−S∗|+|I(x,t)−I∗|)<ϵ,t∈[T2+T3,T2+T3+1] | (4.3) |
since
ct<(2c+c∗)(t+T3+1)/3<(c+2c∗)t/3 |
when t(>T2) is large enough.
Let S,I be the solution of (1.3) with initial value satisfying
infx∈RS(x,0)>δ,infx∈RI(x,0)>δ. |
Then (C) implies that there exists T3>0 such that
supx∈R(|S(x,t)−S∗|+|I(x,t)−I∗|)<ϵ/4,t>T3. |
Let
s(x,t)=S(x,t)−S(x,t),i(x,t)=I(x,t)−I(x,t), |
then the boundedness indicates that there exists L>0 satisfying
|s(x,t)|≤(T1(t−r)|s(⋅,r)|)(x)+L∫trT1(t−s)[|s(⋅,z)|+|i(⋅,z)|+|(J∗i)(⋅,z)|]dz(x),|i(x,t)|≤(T2(t−r)|i(⋅,r)|)(x)+L∫trT2(t−s)[|s(⋅,z)|+|i(⋅,z)|+|(J∗i)(⋅,z)|]dz(x) |
for any r∈[0,t). Then s(x,t),i(x,t) satisfy
|s(x,t)|≤X(x,t),|i(x,t)|≤Y(x,t),x∈R,t>0, |
where (X(x,t),Y(x,t)) is defined by
X(x,t)=(T1(t−r)X(⋅,r))(x)+L∫trT1(t−s)[X(⋅,s)+Y(⋅,s)+(J∗Y)(⋅,s)]ds(x),Y(x,t)=(T2(t−r)Y(⋅,r))(x)+L∫trT2(t−s)[X(⋅,s)+Y(⋅,s)+(J∗Y)(⋅,s)]ds(x) |
with
X(x,0)=|s(x,0)|,Y(x,s)=|i(x,s)|,s∈[−τ,0]. |
Let N>0 be a fixed constant (clarified later) such that
X(x,0)=Y(x,s)=0,|x|≤N,x∈R,s∈[−τ,0], |
and
supx∈RX(x,0)+supx∈R,s∈[−τ,0]Y(x,s)<I′+1. |
Let C>0 be large and λ>0 be small such that
(d+1)λ2−Cλ+L[2+∫τ0∫K−KJ(z,s)eλ(z−Cs)dzds]≤0, |
of which the admissibility is clear by the existence of τ,K. Define a continuous function
Φ(x,t)=min{(I′+1)[eλ(x−N+Ct)+eλ(−x−N+Ct)],(I′+1)e3L(t+τ)} |
for t≥−τ,x∈R, which implies that
Φ(x,0)≥X(x,0),Φ(x,s)≥Y(x,s),x∈R,s∈[−τ,0]. |
Then we have
Φ(x,t)≥(T1(t−r)Φ(⋅,r))(x)+L∫trT1(t−s)[2Φ(⋅,s)+(J∗Φ)(⋅,s)]ds(x),Φ(x,t)≥(T2(t−r)Φ(⋅,r))(x)+L∫trT2(t−s)[2Φ(⋅,s)+(J∗Φ)(⋅,s)]ds(x) |
for any 0≤r<t<∞,x∈R, and so
Φ(x,t)≥X(x,t),Φ(x,t)≥Y(x,t),x∈R,t≥0. |
Then we fix N=N(T3)>0 such that
X(0,T3)≤ϵ4,Y(0,T3)≤ϵ4, |
and for any T2>0 such that
(2c+c∗)(T2+T3+1)/3+N<(c+2c∗)T2/3, |
we have (4.3), so for (4.2). The proof is complete.
In this section, we shall illustrate the analytic conclusion by some numerical examples. Due to Theorem 3.1, we simulate the dynamics in a large spatial interval [−N,N] and take the following boundary conditions
I(x,t)=0,S(x,t)=1,t≥−τ,|x|=N. |
Moreover, I(x,0) is defined by
I(x,0)={sinx,x∈[0,π],0,x∉[0,π]. |
Firstly, we consider (1.2) without nonlocal delay and taking
{∂S(x,t)∂t=∂2S(x,t)∂x2+1−S(x,t)−2S(x,t)I(x,t)1+I(x,t),∂I(x,t)∂t=∂2I(x,t)∂x2+2S(x,t)I(x,t)1+I(x,t)−I(x,t). | (5.1) |
With these parameters, we see that
Λ(λ,c)=λ2−cλ+1 |
and c∗=2. We now show the numerical results as follows.
From Figures 1 and 2, we see that I almost invades and S almost decreases at a constant speed. To further show the invasion speed of I, we present the following Figure 3 on the distribution at t=100, by which we see that the invasion speed is close to c∗=2.
We now consider the effect of time delay in the following model
{∂S(x,t)∂t=∂2S(x,t)∂x2+1−S(x,t)−2S(x,t)I(x,t−τ)1+I(x,t−τ),∂I(x,t)∂t=∂2I(x,t)∂x2+2S(x,t)I(x,t−τ)1+I(x,t−τ)−I(x,t),S(x,0)=1,I(x,s)=I(x,0),s∈[−τ,0], | (5.2) |
such that
Λ(λ,c)=λ2−cλ+2e−λcτ−1. |
When τ=1, we show the graphs of Λ(λ,c) by the following Figures 4 and 5, from which we see that c∗ is close to 1.
The evolution of S,I with τ=1 is presented by Figures 6 and 7.
To estimate the invasion speed with different τ, we give the distribution of t=100 by Figures 8 and 9, by which we see the role of time delay.
To show the effect of nonlocality, we consider
{∂S(x,t)∂t=∂2S(x,t)∂x2+1−S(x,t)−2S(x,t)[I(x+l,t−τ)+I(x−l,t−τ)]2+[I(x+l,t−τ)+I(x−l,t−τ)],∂I(x,t)∂t=∂2I(x,t)∂x2+2S(x,t)[I(x+l,t−τ)+I(x−l,t−τ)]2+[I(x+l,t−τ)+I(x−l,t−τ)]−I(x,t) | (5.3) |
and
Λ(λ,c)=λ2−cλ+[elλ−cτλ+e−lλ−cτλ]−(μ+γ). |
We first show the graph of Λ(λ,c) as follows when τ=1,l=1 by Figures 10 and 11.
Comparing Figures 4 and 5 with Figures 10-11, we see that the nonlocality increases the invasion speed. We τ=l=2, we see that c∗ is close to 1 from the following Figure 12.
Although τ=2>1,c∗ is close to 1 due to the role of spatial nonlocality. The evolution of S,I with τ=l=2 is presented as follows.
To further show the effect of spatial nonlocality, we also simulate S,I by l=4,τ=2 by the following Figures 15 and 16.
Clearly, although τ=2>1 in Figures 6 and 7, the spreading speed is close to that in Figures 6 and 7, which indicates the role of spatial nonlocality.
To show the role of spatial nonlocality, we fix τ=2 and take l=2,l=4 and compare the distribution. From the following Figures 17 and 18, we see that the stronger nonlocality implies larger spreading threshold.
Finally, different from that in the monotone model (5.1), we consider the following system without comparison principle
{∂S(x,t)∂t=∂2S(x,t)∂x2+1−S(x,t)−2S(x,t)I(x,t)e−6I(x,t),∂I(x,t)∂t=∂2I(x,t)∂x2+2S(x,t)I(x,t)e−6I(x,t)−I(x,t). | (5.4) |
Clearly, the spreading speed of (5.4) is the same as that in (5.1), and the following figures imply the spreading speed of I is close to 2.
The spatial spreading of epidemic models attracts much attention since it greatly affects the human behavior in modern days. In population dynamics, there are many works on the study of spatial dynamics of epidemic models. Since the interesting works of [7,8], the threshold of spatial spreading of epidemic models has been widely studied. Thus the analysis of parameters dependence of propagation threshold may show the factors on disease spreading and control. In this paper, we have shown the effect of time delay and spatial nonlocality by theoretical analysis and numerical simulations. But it is difficult to change the latent periods in many cases. Due to our analysis, the spatial movement of the infective in history may increase the spreading speed of the disease, and it is reasonable to restrain the spatial movement of potential infective individuals to control some diseases. The recipe has been utilized in controlling many disease including SARS.
As we have mentioned in Section 1, the traveling wave solutions can not well illustrate the outbreak of some diseases, of which the initial prevalent district is small. To model the feature, asymptotic spreading is a suitable index. Comparing with the traveling wave solutions, there are a few results on the asymptotic spreading of nonmonotone epidemic models. In this paper, we obtained the spreading speed in a class of epidemic models. In fact, the methodology in this work can be extended to more general systems. For example,
{∂S(x,t)∂t=d∂2S(x,t)∂x2+f(S(x,t))−S(x,t)f1((J1∗I)(x,t)),∂I(x,t)∂t=∂2I(x,t)∂x2+S(x,t)f2((J2∗I)(x,t))−(μ+γ)I(x,t),S(x,0)=1,I(x,s)=ϕ(x,s), |
in which f1≠f2 is admissible and kernel functions J1,J2 may be different, f may be a function including f(u)=ru(1−u). Under proper assumptions, we could estimate the spreading speed of I.
The spreading speed in this paper equals the minimal wave speed of traveling wave solutions in some known results. For some models similar to (2.1), it has been proven that the traveling wave solutions with minimal wave speed is stable in the sense of weighted functional spaces, see [51,52] and references cited therein. Therefore, it is possible to understand the dynamics of the corresponding initial value problems by traveling wave solutions. In this paper, we show a rough speed on the branch I(x,t). Very likely, the shape of spreading of I governed by (1.2) can be approximated by the shape of traveling wave solutions with minimal wave speed in proper weighted functional spaces.
We are grateful to three referees and Professor Shigui Ruan for their valuable comments. Lin was supported by NSF of China (11731005) and Fundamental Research Funds for the Central Universities (lzujbky-2018-113), Yan was supported by NSF of China (61763024) and Foundation of a Hundred Youth Talents Training Program of Lanzhou Jiaotong University (152022).
The authors declare no conflict of interest in this paper.
[1] |
L. Abualigah, Multi-verse optimizer algorithm: A comprehensive survey of its results, variants, and applications, Neural Comput. Appl., 32 (2020), 12381–12401. doi: 10.1007/s00521-020-04839-1. doi: 10.1007/s00521-020-04839-1
![]() |
[2] |
K. Hussain, M. N. Mohd Salleh, S. Cheng, Y. Shi, Metaheuristic research: a comprehensive survey, Artif. Intell. Rev., 52 (2019), 2191–2233. doi: 10.1007/s10462-017-9605-z. doi: 10.1007/s10462-017-9605-z
![]() |
[3] |
L. B. Booker, D. E. Goldberg, J. H. Holland, Classifier systems and genetic algorithms, Artif. Intell., 40 (1989), 235-282. doi: 10.1016/0004-3702(89)90050-7. doi: 10.1016/0004-3702(89)90050-7
![]() |
[4] | J. R. Koza, J. P. Rice, Automatic programming of robots using genetic programming, in Proceedings Tenth National Conference on Artificial Intelligence, (1992), 194–201. |
[5] |
S. Das, P. N. Suganthan, Differential evolution: a survey of the state-of-the-art, IEEE Trans. Evol. Comput., 15 (2011), 4–31. doi: 10.1109/TEVC.2010.2059031. doi: 10.1109/TEVC.2010.2059031
![]() |
[6] | J. Kennedy, R. Eberhart, Particle swarm optimization, in Proceedings of ICNN'95-International Conference on Neural Networks, 4 (1995), 1942–1948. doi: 10.1109/ICNN.1995.488968. |
[7] |
S. Mirjalili, S. M. Mirjalili, A. Lewis, Grey Wolf Optimizer, Adv. Eng. Softw., 69 (2014), 46–61. doi: 10.1016/j.advengsoft.2013.12.007. doi: 10.1016/j.advengsoft.2013.12.007
![]() |
[8] |
D. Zhao, L. Liu, F. H. Yu, A. A. Heidari, M. J. Wang, G. X. Liang, et al., Chaotic random spare ant colony optimization for multi-threshold image segmentation of 2D Kapur entropy, Knowl. Based Syst., 216 (2020), 106510. doi: 10.1016/j.knosys.2020.106510. doi: 10.1016/j.knosys.2020.106510
![]() |
[9] |
D. Karaboga, B. Basturk, On the performance of artificial bee colony (ABC) algorithm, Appl. Soft. Comput., 8 (2008), 687–697. doi: 10.1016/j.asoc.2007.05.007. doi: 10.1016/j.asoc.2007.05.007
![]() |
[10] |
S. Mirjalili, A. Lewis, The whale optimization algorithm, Adv. Eng. Softw., 95 (2016), 51–67. doi: 10.1016/j.advengsoft.2016.01.008. doi: 10.1016/j.advengsoft.2016.01.008
![]() |
[11] |
S. M. Li, H. L. Chen, M. J. Wang, A. A. Heidari, S. Mirjalili, Slime mould algorithm: a new method for stochastic optimization, Futur. Gener. Comput. Syst., 111 (2020), 300–323. doi: 10.1016/j.future.2020.03.055. doi: 10.1016/j.future.2020.03.055
![]() |
[12] |
A. Faramarzi, M. Heidarinejad, S. Mirjalili, A. H. Gandomi, Marine predators algorithm: a nature-inspired metaheuristic, Expert Syst. Appl., 152 (2020), 113377. doi: 10.1016/j.eswa.2020.113377. doi: 10.1016/j.eswa.2020.113377
![]() |
[13] |
H. M. Jia, X. X. Peng, C. B. Lang, Remora optimization algorithm, Expert Syst. Appl., 185 (2021), 115665. doi: 10.1016/j.eswa.2021.115665. doi: 10.1016/j.eswa.2021.115665
![]() |
[14] |
C. R. Hwang, Simulated annealing: Theory and applications, Acta. Appl. Math., 12 (1988), 108–111. doi: 10.1016/0378-4754(88)90023-7. doi: 10.1016/0378-4754(88)90023-7
![]() |
[15] |
E. Rashedi, H. Nezamabadi-pour, S. Saryazdi, GSA: a gravitational search algorithm, Inf. Sci., (Ny) 179 (2009), 2232–2248. doi: 10.1016/j.ins.2009.03.004. doi: 10.1016/j.ins.2009.03.004
![]() |
[16] |
S. Mirjalili, S. M. Mirjalili, A. Hatamlou, Multi-verse optimizer: a nature-inspired algorithm for global optimization, Neural Comput. Appl., 27 (2015), 495–513. doi: 10.1007/s00521-015-1870-7. doi: 10.1007/s00521-015-1870-7
![]() |
[17] |
F. Asef, V. Majidnezhad, M. R. Feizi-Derakhshi, S. Parsa, Heat transfer relation-based optimization algorithm (HTOA), Soft. Comput., 25 (2021), 8129–8158. doi: 10.1007/s00500-021-05734-0. doi: 10.1007/s00500-021-05734-0
![]() |
[18] |
B. Alatas, ACROA: Artificial Chemical Reaction Optimization Algorithm for global optimization, Expert Syst. Appl., 38 (2011), 13170–13180. doi: 10.1016/j.eswa.2011.04.126. doi: 10.1016/j.eswa.2011.04.126
![]() |
[19] | F. F. Moghaddam, R. F. Moghaddam, M. Cheriet, Curved Space Optimization: A Random Search based on General Relativity Theory, preprint, arXiv: 1208.2214. |
[20] |
Z. W. Geem, J. H. Kim, G. Loganathan, A new heuristic optimization algorithm: harmony search, Simulation, 76 (2001), 60–68. doi: 10.1177/003754970107600201. doi: 10.1177/003754970107600201
![]() |
[21] |
R. V. Rao, V. J. Savsani, D. P. Vakharia, Teaching-Learning-Based optimization: an optimization method for continuous non-linear large scale problems, Inf. Sci., 183 (2012), 1–15. doi: 10.1016/j.ins.2011.08.006. doi: 10.1016/j.ins.2011.08.006
![]() |
[22] |
R. V. Rao, V. J. Savsani, D. P. Vakharia, Teaching-Learning-Based Optimization: A novel method for constrained mechanical design optimization problems, Computer-Aided Des., 43 (2011), 303–15. doi: 10.1016/j.cad.2010.12.015. doi: 10.1016/j.cad.2010.12.015
![]() |
[23] |
F. Ramezani, S. Lotfi, Social-Based Algorithm (SBA), Appl. Soft. Comput., 13 (2013), 2837–2856. doi: 10.1016/j.asoc.2012.05.018. doi: 10.1016/j.asoc.2012.05.018
![]() |
[24] |
Q. Fan, Z. J. Chen, Z. Li, Z. H. Xia, J. Y. Yu, D. Z. Wang, A new improved whale optimization algorithm with joint search mechanisms for high-dimensional global optimization problems, Eng. Comput., 37 (2021), 1851–1878. doi: 10.1007/s00366-019-00917-8. doi: 10.1007/s00366-019-00917-8
![]() |
[25] |
A. Abbasi, B. Firouzi, P. Sendur, A. A. Heidari, H. L. Chen, R. Tiwari, Multi-strategy Gaussian Harris hawks optimization for fatigue life of tapered roller bearings, Eng. Comput., 2021 (2021). doi: 10.1007/s00366-021-01442-3. doi: 10.1007/s00366-021-01442-3
![]() |
[26] |
Y. Li, Y. Zhao, J. Liu, Dynamic sine cosine algorithm for large-scale global optimization problems, Expert Syst. Appl., 173 (2021), 114950. doi: 10.1016/j.eswa.2021.114950. doi: 10.1016/j.eswa.2021.114950
![]() |
[27] |
C. Y. Yu, A. A. Heidari, X. Xue, L. J. Zhang, H. L. Chen, W. B. Chen, Boosting Quantum Rotation Gate Embedded Slime Mould Algorithm, Expert Syst. Appl., 181 (2021), 115082. doi: 10.1016/j.eswa.2021.115082. doi: 10.1016/j.eswa.2021.115082
![]() |
[28] |
Q. S. Fan, H. S. Huang, Q. P. Chen, L. G. Yao, D. Huang, A modified self-adaptive marine predators algorithm: framework and engineering applications, Eng. Comput., 2021 (2021). doi: 10.1007/s00366-021-01319-5. doi: 10.1007/s00366-021-01319-5
![]() |
[29] |
D. H. Wolpert, W. G. Macready, No free lunch theorems for optimization, IEEE Trans. Evol. Comput., 1 (1997), 67–82. doi: 10.1109/4235.585893 doi: 10.1109/4235.585893
![]() |
[30] |
L. Abualigah, A. Diabat, S. Mirjalili, M. A. Elaziz, A. H. Gandomi, The arithmetic optimization algorithm, Comput. Methods Appl. Mech. Eng., 376 (2021), 113609. doi: 10.1016/j.cma.2020.113609. doi: 10.1016/j.cma.2020.113609
![]() |
[31] |
S. Mirjalili, Moth-flame optimization algorithm: a novel nature-inspired heuristic paradigm, Knowl.-Based Syst., 89 (2015), 228–249. doi: 10.1016/j.knosys.2015.07.006. doi: 10.1016/j.knosys.2015.07.006
![]() |
[32] |
P. Manoharan, P. Jangir, D. S. Kumar, S. Ravichandran, S. Mirjalili, A new arithmetic optimization algorithm for solving real-world multiobjective CEC-2021 constrained optimization problems: diversity analysis and validations, IEEE Access, 9 (2021), 84263–84295. doi: 10.1109/ACCESS.2021.3085529. doi: 10.1109/ACCESS.2021.3085529
![]() |
[33] |
A. Žilinskas, J. Calvin, Bi-objective decision making in global optimization based on statistical models, J. Glob. Optim., 74 (2018), 599–609. doi: 10.1007/s10898-018-0622-5. doi: 10.1007/s10898-018-0622-5
![]() |
[34] |
L. Abualigah, A. Diabat, P. Sumari, A. H. Gandomi, A novel evolutionary arithmetic optimization algorithm for multilevel thresholding degmentation of COVID-19 CT images, Processes, 9 (2021), 1155. doi: 10.3390/pr9071155. doi: 10.3390/pr9071155
![]() |
[35] |
S. Khatir, S. Tiachacht, C. L. Thanh, E. Ghandourah, M. A. Wahab, An improved artificial neural network using arithmetic optimization algorithm for damage assessment in FGM composite plates, Compos. Struct., 273 (2021), 114287. doi: 10.1016/j.compstruct.2021.114287. doi: 10.1016/j.compstruct.2021.114287
![]() |
[36] |
J. G. Digalakis, K. G. Margaritis, On benchmarking functions for genetic algorithms, Int. J. Comput. Math., 77 (2001), 481–506. doi: 10.1080/00207160108805080. doi: 10.1080/00207160108805080
![]() |
[37] | C. T. Yue, K. V. Price, P. N. Suganthan, J. J. Liang, M. Z. Ali, B. Y. Qu, et al., Problem definitions and evaluation criteria for the CEC 2020 special session and competition on single objective bound constrained numerical optimization, (2020). |
[38] |
S. García, A. Fernández, J. Luengo, F. Herrera, Advanced nonparametric tests for multiple comparisons in the design of experiments in computational intelligence and data mining: experimental analysis of power, Inf. Sci. (Ny), 180 (2010), 2044–2064. doi: 10.1016/j.ins.2009.12.010 doi: 10.1016/j.ins.2009.12.010
![]() |
[39] |
E. Theodorsson-Norheim, Friedman and Quade tests: BASIC computer program to perform nonparametric two-way analysis of variance and multiple comparisons on ranks of several related samples, Comput. Biol. Med., 17 (1987), 85–99. doi: 10.1016/0010-4825(87)90003-5. doi: 10.1016/0010-4825(87)90003-5
![]() |
[40] |
S. Mirjalili, SCA: a sine cosine algorithm for solving optimization problems, Knowl. Based Syst., 96 (2016), 120–133. doi: 10.1016/j.knosys.2015.12.022. doi: 10.1016/j.knosys.2015.12.022
![]() |
[41] |
S. Mirjalili, A. H. Gandomi, S. Z. Mirjalili, S. Saremi, H. Faris, S. M. Mirjalili, Salp swarm algorithm: a bio-inspired optimizer for engineering design problems, Adv. Eng. Softw., 114 (2017), 163–191. doi: 10.1016/j.advengsoft.2017.07.002. doi: 10.1016/j.advengsoft.2017.07.002
![]() |
[42] |
S. K. Wang, K. J. Sun, W. Y. Zhang, H. M. Jia, Multilevel thresholding using a modified ant lion optimizer with opposition-based learning for color image segmentation, Math. Biosci. Eng., 18 (2021), 3092–3143. doi: 10.3934/mbe.2021155. doi: 10.3934/mbe.2021155
![]() |
[43] |
W. Long, J. J. Jiao, X. M. Liang, S. H. Cai, M. Xu, A Random Opposition-Based Learning Grey Wolf Optimizer, IEEE Access, 7 (2019), 113810–113825. doi: 10.1109/ACCESS.2019.2934994. doi: 10.1109/ACCESS.2019.2934994
![]() |
[44] |
A. Seyyedabbasi, R. Aliyev, F. Kiani, M. U. Gulle, H. Basyildiz, M. A. Shah, Hybrid algorithms based on combining reinforcement learning and metaheuristic methods to solve global optimization problems, Knowl. Based Syst., 223 (2021), 107044. doi: 10.1016/j.knosys.2021.107044. doi: 10.1016/j.knosys.2021.107044
![]() |
[45] |
R. Zheng, H. M. Jia, L. Abualigah; Q. X. Liu, S. Wang, Deep Ensemble of Slime Mold Algorithm and Arithmetic Optimization Algorithm for Global Optimization, Processes, 9 (2021), 1774. doi: 10.3390/pr9101774. doi: 10.3390/pr9101774
![]() |
[46] |
S. Wang, Q. X. Liu, Y. X. Liu, H. M. Jia, L. Abualigah, R. Zheng, et al., A Hybrid SSA and SMA with mutation opposition-based learning for constrained engineering problems, Comput. Intel. Neurosc., 2021 (2021), 6379469. doi: 10.1155/2021/6379469. doi: 10.1155/2021/6379469
![]() |
[47] |
S. Mirjalili, How effective is the grey wolf optimizer in training multi-layer perceptrons, Appl. Intell., 43 (2015), 150–161. doi: 10.1007/s10489-014-0645-7. doi: 10.1007/s10489-014-0645-7
![]() |
[48] |
T. Ray, P. Saini, Engineering design optimization using a swarm with an intelligent information sharing among individuals, Eng. Optim., 33 (2001), 735–748. doi: 10.1080/03052150108940941. doi: 10.1080/03052150108940941
![]() |
[49] |
A. H. Gandomi, X. S. Yang, A. H. Alavi, Cuckoo search algorithm: a metaheuristic approach to solve structural optimization problems, Eng. Comput., 29 (2013), 17–35. doi: 10.1007/s00366-011-0241-y. doi: 10.1007/s00366-011-0241-y
![]() |
[50] |
A. Sadollah, A. Bahreininejad, H. Eskandar, M. Hamdi, Mine blast algorithm: a new population based algorithm for solving constrained engineering optimization problems, Appl. Soft. Comput., 13 (2013), 2592–612. doi: 10.1016/j.asoc.2012.11.026. doi: 10.1016/j.asoc.2012.11.026
![]() |
[51] |
S. Saremi, S. Mirjalili, A. Lewis, Grasshopper Optimization Algorithm: Theory and application, Adv. Eng. Softw., 105 (2017), 30–47. doi: 10.1016/j.advengsoft.2017.01.004. doi: 10.1016/j.advengsoft.2017.01.004
![]() |
[52] |
H. Liu, Z. Cai, Y. Wang, Hybridizing particle swarm optimization with differential evolution for constrained numerical and engineering optimization, Appl. Soft. Comput., 10 (2010), 629–640. doi: 10.1016/j.asoc.2009.08.031. doi: 10.1016/j.asoc.2009.08.031
![]() |
[53] |
N. Singh, J. Kaur, Hybridizing sine-cosine algorithm with harmony search strategy for optimization design problems, Soft. Comput., 25 (2021), 11053–11075. doi: 10.1007/s00500-021-05841-y. doi: 10.1007/s00500-021-05841-y
![]() |
[54] |
B. K. Kannan, S. N. Kramer, An augmented lagrange multiplier based method for mixed integer discrete continuous optimization and its applications to mechanical design, J. Mech. Des., 116 (1994), 405–411. doi: 10.1115/1.2919393. doi: 10.1115/1.2919393
![]() |
[55] |
R. M. Rizk-Allah, Hybridizing sine cosine algorithm with multi-orthogonal search strategy for engineering design problems, J. Comput. Des. Eng., 5 (2018), 249–273. doi: 10.1016/j.jcde.2017.08.002. doi: 10.1016/j.jcde.2017.08.002
![]() |
[56] |
Y. Ling, Y. Q. Zhou, Q. F. Luo, Lévy flight trajectory-based whale optimization algorithm for global optimization, IEEE Acess, 5 (2017), 6168–6186. doi: 10.1109/ACCESS.2017.2695498. doi: 10.1109/ACCESS.2017.2695498
![]() |
[57] |
D. Pelusi, R. Mascella, L. Tallini, J. Nayak, B. Naik, Y. Deng, An improved moth-flame optimization algorithm with hybrid search phase, Knowl. Based Syst., 191 (2020), 105277. doi: 10.1016/j.knosys.2019.105277. doi: 10.1016/j.knosys.2019.105277
![]() |
[58] |
A. Baykasoğlu, S. Akpinar, Weighted superposition attraction (WSA): A swarm intelligence algorithm for optimization problems-part2: Constrained optimization, Appl. Soft. Comput., 37 (2015), 396–415. doi: 10.1016/j.asoc.2015.08.052. doi: 10.1016/j.asoc.2015.08.052
![]() |