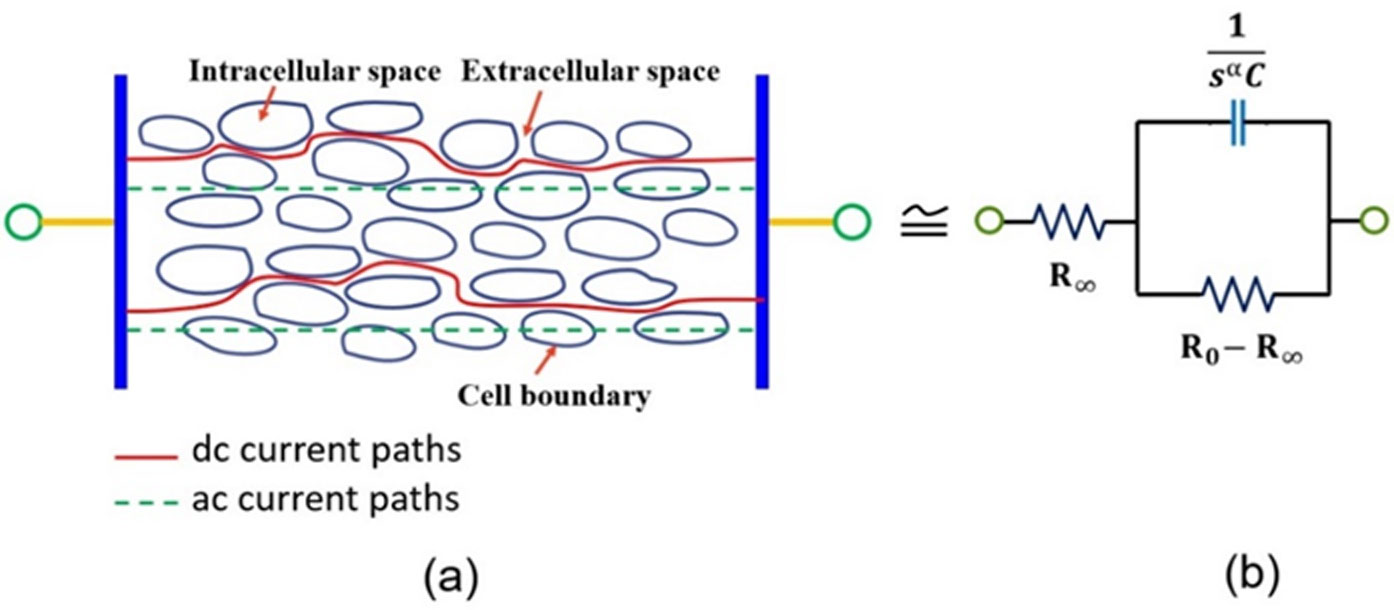
Citation: Alain Mauger, Haiming Xie, Christian M. Julien. Composite anodes for lithium-ion batteries: status and trends[J]. AIMS Materials Science, 2016, 3(3): 1054-1106. doi: 10.3934/matersci.2016.3.1054
[1] | Svetlana Kashina, Andrea Monserrat del Rayo Cervantes-Guerrero, Francisco Miguel Vargas-Luna, Gonzalo Paez, Jose Marco Balleza-Ordaz . Tissue-specific bioimpedance changes induced by graphene oxide ex vivo: a step toward contrast media development. AIMS Biophysics, 2025, 12(1): 54-68. doi: 10.3934/biophy.2025005 |
[2] | Abhishek Mallick, Atanu Mondal, Somnath Bhattacharjee, Arijit Roy . Application of nature inspired optimization algorithms in bioimpedance spectroscopy: simulation and experiment. AIMS Biophysics, 2023, 10(2): 132-172. doi: 10.3934/biophy.2023010 |
[3] | Arijit Roy, Somnath Bhattacharjee, Soumyajit Podder, Advaita Ghosh . Measurement of bioimpedance and application of Cole model to study the effect of moisturizing cream on human skin. AIMS Biophysics, 2020, 7(4): 362-379. doi: 10.3934/biophy.2020025 |
[4] | D Kelly, L Mackenzie, David A. Saint . Mechanoelectric feedback does not contribute to the Frank-Starling relation in the rat and guinea pig heart. AIMS Biophysics, 2014, 1(1): 16-30. doi: 10.3934/biophy.2014.1.16 |
[5] | Moataz M. Fahmy, Sohier M. El-Kholey, Seham Elabd, Mamdouh M. Shawki . Effect of changing the alternating electric current frequency on the viability of human liver cancer cell line (HEPG2). AIMS Biophysics, 2025, 12(1): 1-13. doi: 10.3934/biophy.2025001 |
[6] | Alexander G. Volkov, Yuri B. Shtessel . Propagation of electrotonic potentials in plants: Experimental study and mathematical modeling. AIMS Biophysics, 2016, 3(3): 358-379. doi: 10.3934/biophy.2016.3.358 |
[7] | Alaa AL-Rahman Gamal, El-Sayed Mahmoud El-Sayed, Tarek El-Hamoly, Heba Kahil . Development and bioevaluation of controlled release 5-aminoisoquinoline nanocomposite: a synergistic anticancer activity against human colon cancer. AIMS Biophysics, 2022, 9(1): 21-41. doi: 10.3934/biophy.2022003 |
[8] | Zachary Mekus, Jessica Cooley, Aaron George, Victoria Sabo, Morgan Strzegowski, Michelle Starz-Gaiano, Bradford E. Peercy . Effects of cell packing on chemoattractant distribution within a tissue. AIMS Biophysics, 2018, 5(1): 1-21. doi: 10.3934/biophy.2018.1.1 |
[9] |
Maria Teresa Caccamo, Giuseppe Mavilia, Letterio Mavilia, Pietro Calandra, Domenico Lombardo, Salvatore Magazù .
Thermal investigation of montmorillonite/BSA by fourier transform infrared spectroscopy measurements |
[10] | Enrica Serretiello, Martina Iannaccone, Federica Titta, Nicola G. Gatta, Vittorio Gentile . Possible pathophysiological roles of transglutaminase-catalyzed reactions in the pathogenesis of human neurodegenerative diseases. AIMS Biophysics, 2015, 2(4): 441-457. doi: 10.3934/biophy.2015.4.441 |
The method of monitoring physiological condition by means of bioimpedance remains a novel technique for more than 50 years or so. The non-destructive method of bioimpedance measurement for fruits and vegetables is extensively used for various purposes such as to access health conditions [1],[2], effect of storage conditions [3], physiological changes including assessing the quality of fruits and vegetables [4]–[8]. On the other hand, investigations are also carried out to understand the science behind the characteristics of bioimpedance of plant tissues [9]–[17]
The necessity of invariability of bioimpedance parameters is extremely important in practical application of Cole model for discriminating fruits (or vegetables) from a reference fruit (or vegetable). Say for example someone wants to compare the Cole parameters for two different species of a given fruit. In such cases, the parameters can be compared if the parameters are not dependent on position (of a given fruit or sample). If the position (of measurement) dependent variability is high, one cannot distinguish two different samples under investigation. Thus, the measurement method should be robust enough to establish invariability of bioimpedance parameters which can be treated as reference parameters for comparison purpose.
Cole model is widely applied for living biological tissue [15],[18]–[21]. In general, lump tissue is represented by Cole equivalent circuit and single dispersion model is accepted for its simplicity [7],[14] (Figure 1). Among the various method of bioimpedance measurements, direct measurement (i.e. using LCR meter) with two-electrode configuration is the most generic one due to its high degree of reliability [5],[20]. The electrical current paths inside a tissue is schematically shown in Figure 1 (a). It is intuitive from this figure, that if impedance is measured for the same tissue at other position, keeping the distance between the electrode invariant, one should obtain the same results (within the limits of experimental error for living tissue). On the other hand, if the distance between the electrodes is not kept same for measuring impedance at two different positions, the variability in measured parameters are clearly observed [14],[15],[22]. Thus, in order to obtain the comparable results among the samples, it is quite obvious to keep the distance between the electrode invariant. The reasons of choosing apple and potato are as follows. First of all, apple is a fruit whereas potato is a vegetable (tuber) and both of them are extensively used as food materials and therefore have importance in agriculture sector. Further, apple grows in open atmosphere whereas potato grows under soil. Not only these, the tissue of apple will have a natural tendency towards ripen (or to be eaten) so that seed can be dispersed. On the other hand, potato tissue will have tendency to fight against external environment to sustain so as to produce the next generation potato. The porosity (fraction of empty space) of apple is highly different than that of potato.
In this work, a specific electrode-pair is designed and is used to measure bioimpedance of apple and potato tissues. It is needless to mention that, though apple is a fruit, potato is a vegetable and providing us two different kind of living tissue to be investigated under this study. Applying single dispersion Cole model, impedance parameters are extracted by curve fitting. It is established here that the tissue of apple and potato can be specified in terms of bioimpedance parameters which in turn can be used to discriminate similar tissue of different species with 98% confidence. Further, with 99% confidence, it is evidently shown here that the position of measurement of bioimpedance of a given fruit or vegetable is not a factor of variability. Thus, our process of measurement can directly be applied in assessing quality (or condition) of fruits or vegetables. In addition to this, our method can be applied to monitor the ripening of fruits similar to citrus fruit like orange [8].
Single dispersion Cole bioimpedance model is a widely accepted model is extensively used for various kinds of biological tissue
where,
Separation of Z into real and imaginary parts is not straight forward due to the non-integer value of α (0 < α ≤1), which turns the
Thus, the modulus of Z becomes
Experimentally, Eq. (4) |Z| is obtained as a function of frequency f (= ω/2π). The experimental data is then fitted (non-linear curve fitting) and values of R0, R∞, C, α are obtained. From these parameters, the relaxation time τ is calculated using Eq. (2).
In general, the physiological parameters are non-repetitive and standard deviation is large. For example, if at identical condition, the cell size is measured for a given tissue, the variation of more than 20% is not uncommon while the grain size of a metallic conductor is measured, the result is found to be within the tolerance level (about 5% or so). The variation in cell size would therefore lead to a large variation in the tissue related parameters. Thus, it is extremely important to specify the tissue by means of Cole model parameters as well as relaxation time. On the other hand, it is essential to keep the distance (or gap) between the electrode-pair invariant. Intuitively, this is due to the fact that the Cole parameters (except α) are highly dependent on the gap between the electrodes. Hence, to discriminate tissues from one another, it is essential to obtain the invariability of impedance irrespective of measuring position (of tissue) of fruits or vegetable.
An electrode-pair is designed and fabricated for the purpose of bioimpedance measurement of fruit or vegetable. A schematic of the fabricated electrode-pair is shown in Figure 2. The depth of penetration and diameter of the electrodes are 1.5 cm and 0.28 mm respectively and these are maintained throughout this work unless otherwise stated. The electrodes are separated by plastic material (of negligibly low leakage current). The plastic holder of the electrode-pair is made by cutting a disposable syringe. The gap between the electrode is kept at 3 mm. For electrode material, stain-less steel is chosen for its inertness of reacting with any other materials and retain sufficient strength at low wire diameter.
Sample | Species/Description | Designation of Measurement |
Apple-1 | Apple-1 and Apple-2 are of same species (Green Granny Smith) and both are purchased from same local shop. Apple-1 and Apple-2 can be considered to be biologically identical. | A11, A12, A13, A14, A15 |
Apple-2 | A21, A22, A23, A24, A25 | |
Apple-3 | Apple-3 (Red Washington) is of different species that of Apple-1 and Apple-2. Apple-3 is biologically different from Apple-1 and Apple-2. | A31, A32, A33, A34, A35 |
Potato-1 | Potato-1 and Potato-2 are of same species (Kufri Chandramukhi) and both are purchased from same local shop. Potato-1 and Potato-2 can be considered to be biologically identical. | P11, P12, P13, P14, P15 |
Potato-2 | P21, P22, P23, P24, P25 | |
Potato-3 | Potato-3 (Kufri Jyoti) is of different species that of Potato-1 and Potato-2. Potato-3 is biologically different from Potato-1 and Potato-2. | P31, P32, P33, P34, P35 |
Remarks: In this table, Amn (or Pmn) represents impedance measurement of Apple-m (or Potato-m) at position n.
The sample matrix is shown Table 1. In order to remove any parasitic effect that may arise from electrodes and probes, the LCR meter (Model: 8101G, Make: Gw Instek) is calibrated before taking any measurement data. Each time electrodes are cleaned by medicated sprit and dried properly before inserting into fruit or vegetable. By inserting the electrode-pair at desired position of the sample, impedance is measured in the frequency range of 1 Hz to 1 MHz with amplitude of 1 V (peak to peak). All measurements are conducted at identical condition. In order to avoid any physical damage in the tissue due to high electric field, small signal is chosen for measurement. The impedance is found to decrease with the increase in frequency and becomes sufficiently flat around the frequency of 1 MHz and thus restricts the choice of upper limit on the frequency of interest. For each sample, five measurements are done at five different positions and each measurement contains four hundred data points. Typical measurement data are plotted as a function of frequency and is shown in Figure 3.
Experimentally |Z| is obtained as a function of frequency for different samples and for different position of a given sample as mentioned in Table 1. The non-linear curve fitting is performed by commercial MS Excel 2016 package. As a common practice, in the curve fitting process, the value of R0 and R∞ is considered to be the measured values R0 and R∞. Hence in this work, the measured impedances at 1 Hz and 1 MHz are considered to be R0 and R∞ respectively. The value of α and C are estimated by fitting the experimental curve with the Eq. (4). The characteristic time constant τ is then estimated using Eq. (2) for each set of data.
From the experimental plots of |Z| as a function of frequency (see Figure 3) it is not possible to distinguish the species of a given fruit or vegetable and this is because the plots of different species almost overlap on each other when plotted simultaneously in a single graph. On the other hand, the important parameters related to equivalent circuit of these plots may differ significantly.
The fitted Cole plots are shown in Figure 3(b) and Figure 3(d) for apple and potato respectively. Since, the sample Apple-1 and Apple-2 are from same species, the Cole plots of these do not differ and follow one another for the entire span of frequency range. While the Cole plot for the sample Apple-3 slightly differ from the other two, especially at the low frequency range. This is probably due to the fact that the species of sample Apple-3 is different from that of sample Apple-1 or Apple-2. Exactly similar observation is found for potato samples (Figure 3. (d)). From the fitted Cole plots, it is noticed that the distinguishable capability of bioimpedance method is better at the low frequency range for apple as well for potato. This fact suggests that the bioimpedance study of apple and potato can be limited to 3 kHz. By reducing the frequency range while keeping the number data point same, we can obtain better experimental results.
In this study, all ANOVA are performed using commercial MS Excel 2016 package. The Cole parameters for apple samples are shown in Table 2. ANOVA on τ is performed considering position and sample as two factors. The first factor is considered to understand whether position of measurement is a factor or not. On the other hand, the second factor is considered to understand the discriminating capability of the bioimpedance measurement.
To test these hypotheses, two apples of same species (Green Granny Smith in this case) are taken from a local shop and thus the biological differences between the apples are minimized. Then for each apple, impedance is measured at five different positions. The measured and calculated data is shown in Table 2. Result of ANOVA for tissue of apple is shown in Table 3. Interestingly it is found that none of the factors are significant (since, Fcritical > F-value) at the confidence level as high as 99% when ANOVA is performed among the sample Apple-1 and Apple-2. This means that one the Cole parameters i.e. the relaxation time are indistinguishable as far position is concerned. The two samples (Apple-1/Apple-2) are also found to be indistinguishable. Or in other words, the tissues of Apple-1 and Apple-2 are statistically same. Thus, with respect to our electrode system, the relaxation time is invariable and it is does depend on the position of measurement and remains same for biologically same samples.
On the other hand, though the position is found be indistinguishable in Apple-1 and Apple-3 (or Apple-2 and Apple-3), they are found to be disguisable (Fcritical < F-value) when ANOVA is performed for these two pair of samples. Thus, again as before, the position of measurement is not found to be a factor. However, in the present two cases, the sample is found to be a factor and is discriminated by respective relaxation time. This means the tissues of Apple-1 and Apple-3 are different. This fact proves that the relaxation time of apple tissue can be used to discriminate different species of apple.
Sample | R0 (kΩ) |
R∞ (kΩ) |
α | C (nF secα−1) |
τ (µsec) |
Regression coefficient |
12.93 | 0.64 | 0.719 | 76.65 | 63 | 0.9989 | |
14.21 | 0.74 | 0.703 | 88.30 | 70 | 0.9987 | |
Apple-1 | 13.58 | 0.77 | 0.691 | 99.99 | 65 | 0.9983 |
13.75 | 0.65 | 0.705 | 91.55 | 72 | 0.9989 | |
12.12 | 0.63 | 0.692 | 103.83 | 60 | 0.9983 | |
12.83 | 0.61 | 0.694 | 98.25 | 63 | 0.9980 | |
13.67 | 0.70 | 0.708 | 86.55 | 69 | 0.9985 | |
Apple-2 | 13.90 | 0.72 | 0.696 | 103.47 | 76 | 0.9985 |
12.47 | 0.67 | 0.693 | 112.05 | 70 | 0.9983 | |
10.97 | 0.70 | 0.694 | 113.46 | 59 | 0.9984 | |
11.85 | 0.83 | 0.745 | 62.35 | 57 | 0.9993 | |
10.53 | 0.77 | 0.747 | 64.20 | 52 | 0.9991 | |
Apple-3 | 11.35 | 0.84 | 0.754 | 55.15 | 51 | 0.9994 |
13.75 | 0.84 | 0.767 | 47.47 | 65 | 0.9997 | |
11.74 | 0.84 | 0.751 | 56.65 | 53 | 0.9993 |
Remarks: Apple-1&Apple-2 are of same species and are taken from same lot. While Apple-3 is different from the other two.
Sample | Factor | P-value | F-value | Fcritical | Distinguishability | Level of Confidence |
Apple-1 & Apple-2 | Position | 0.119 | 3.627 | 15.977 | No | 99% |
Sample | 0.594 | 0.334 | 21.197 | No | ||
Apple-1 & Apple-3 | Position | 0.146 | 3.153 | 10.899 | No | 98% |
Sample | 0.017 | 15.384 | 14.039 | Yes |
Sample | R0 (kΩ) |
R∞ (kΩ) |
α | C (nF secα−1) |
τ (µsec) | Regression coefficient |
5.17 | 0.14 | 0.6400 | 341 | 48 | 0.9888 | |
5.48 | 0.16 | 0.7000 | 204 | 41 | 0.9956 | |
Potato-1 | 5.58 | 0.13 | 0.6638 | 246 | 47 | 0.9920 |
5.65 | 0.12 | 0.6505 | 325 | 60 | 0.9922 | |
5.09 | 0.11 | 0.6797 | 243 | 51 | 0.9951 | |
5.14 | 0.12 | 0.6275 | 445 | 60 | 0.9897 | |
5.43 | 0.13 | 0.6532 | 286 | 48 | 0.9918 | |
Potato-2 | 6.66 | 0.16 | 0.6077 | 450 | 68 | 0.9890 |
4.70 | 0.12 | 0.6415 | 363 | 47 | 0.9891 | |
5.67 | 0.12 | 0.5979 | 580 | 68 | 0.9865 | |
5.52 | 0.12 | 0.6360 | 420 | 70 | 0.9934 | |
5.57 | 0.15 | 0.6099 | 610 | 68 | 0.9906 | |
Potato-3 | 6.70 | 0.14 | 0.6202 | 432 | 78 | 0.9912 |
5.86 | 0.11 | 0.6362 | 393 | 69 | 0.9916 | |
5.46 | 0.13 | 0.6436 | 349 | 57 | 0.9924 |
Remarks: Potato -1 & Potato -2 are of same species and are taken from same lot, while Potato -3 is different from the other two.
Sample | Factor | P-value | F-value | Fcritical | Distinguishability | Level of Confidence |
Potato-1 & Potato-2 | Position | 0.6046 | 0.7537 | 15.977 | No | 99% |
Sample | 0.2124 | 2.1975 | 21.198 | No | ||
Potato-1& Potato-3 | Position | 0.6201 | 0.7218 | 10.899 | No | 98% |
Sample | 0.0182 | 14.856 | 14.040 | Yes |
The various Cole parameters for potato samples are tabulated in Table 4. Similar to apple samples, ANOVA is performed on τ for potato samples and the result of the ANOVA is shown in Table 5. Based on the ANOVA, we have obtained similar conclusion as in apples. That is position of measurement is not a factor and different tissue of potato samples can be distinguished based on the relaxation time.
In order to gain confidence on our discrimination method described above, the entire experiment is repeated by taking another two specimens of each type using the same electrode-pair. When data is analyzed similar outcome is resulted. Thus, our method is found to be repetitive and robust.
It is intuitive from the Figure 1(a), that the values of the Cole parameters are highly dependent on (a) cell size, (b) porosity, (c) constituents etc. In order to assess the cell size, extensive analysis is performed on the cellular images obtained using a confocal microscope (Olympus CX41). The average cell size of apple and potato (of typical species used in bioimpedance measurement) are estimated to be (10–12) × 103 µm2 and (18–20) × 103 µm2 respectively. The measured cell areas agree well with the similar measurement presented in published references [23],[24]. Thus, the cell size of potato is about 1.8 times larger than that of apple.
On the other hand, the porosity of the apple and potato are in the range of 0.15–0.22 and 0.03–0.08 respectively [25]. Hence, apple tissue contains much more extracellular space than that of the potato. The estimated cell size and porosity in the tissues under investigation can be used to explain the capacitance obtained experimentally. The capacitance of potato tissue is found larger than that of apple tissue (see Table II and Table IV). In the cellular structure, two nearby cells and extracellular space between cells behave like the plates and dielectric material respectively of a parallel plate capacitor. As the capacitance of a parallel plate capacitor is proportional to the plate area and is inversely proportional to the separation between the plates (the extracellular space), the capacitance (C) is found larger in potato tissue as its cell size is larger and extracellular space (porosity) is smaller.
The dc resistance on the other hand depends on the length the dc current path. Longer the dc current path, larger is the dc resistance R0. Typical dc current paths are drawn manually (based on the principle that dc current flows through the extracellular space) and are shown in Figure 4. It is clear from this figure that the detouring of dc current path of apple tissue is greater than that of potato tissue and as a result apple shows larger R0 which is indeed found experimentally (see Table 2 and Table 4). Thus, the orientation of cells in the tissue highly affects the dc resistance. More the misorientation among the cells, longer is the dc current path and yields larger dc resistance. Another factor that may affect the dc resistance is the resistivity of the constituents of the extracellular space in tissues and such investigation is beyond the scope of this work. Therefore, the comparison of Cole parameters of the tissues of Apple and Potato from the biophysical point of view is tentative.
An experimental method is established here to distinguish different species of fruits or vegetable by means of measuring Cole parameters. Cole parameters are extracted for apple and potato tissue by specially designed electrode-pair using direct measurement technique. Among the various Cole parameters, the relaxation time τ is found to be most promising tissue characterizing parameter, since it contains all other Cole parameters of a given tissue. With statistical relevance, it is shown that tissues of different species of apple as well as potato can be distinguished by our experimental method. Our method can have wide application to distinguish unhealthy (or treated) fruits or vegetables from healthy one. The method is not costly and therefore this method has potential application in accessing plant tissue.
[1] | Zaghib K, Mauger A, Julien CM (2015) Rechargeable lithium batteries for energy storage in smart grids, In: Rechargeable Lithium Batteries, Woodhead Publishing Series in Energy number 81, Elsevier. |
[2] |
Kasavajjula U, Wang C, Appleby AJ (2007) Nano- and bulk-silicon-based insertion anodes for lithium-ion secondary cells. J Power Sources 163: 1003–1039. doi: 10.1016/j.jpowsour.2006.09.084
![]() |
[3] | Julien CM, Mauger A, Vijh A, et al. (2016) Lithium Batteries: Science and Technology, Springer, New York, 323–429. |
[4] | Zhao K, Pharr M, Hartle L, et al. (2012) Fracture and debonding in lithium-ion batteries with electrodes of hollow core-shell nanostructures. J Power Sources 218: 6–14. |
[5] | Ma ZS, Xie ZC, Wang Y, et al. (2015) Failure modes of hollow core-shell structural active materials during the lithiation-delithiation process. J Power Sources 290: 114–122. |
[6] |
Wang C, Ma Z, Wang Y, et al. (2016) Failure prediction of high-capacity electrode materials in lithium-ion batteries. J Electrochem Soc 163: A1157–A1163. doi: 10.1149/2.0251607jes
![]() |
[7] |
Jiang W, Li T, Ma Z, et al. (2015) Optimal design of hollow core-shell structural active materials for lithium ion batteries. Results in Phys 5: 250–252. doi: 10.1016/j.rinp.2015.08.010
![]() |
[8] |
Hu Y, Zhao X, Suo Z (2010) Averting cracks caused by insertion reaction in lithium-ion batteries. J Mater Res 25: 1007–1010. doi: 10.1557/JMR.2010.0142
![]() |
[9] | Wang Y, Ma Z, Lei W, et al. (2016) Double effect of electrochemical reaction and substrate on hardness in electrodes of lithium-ion batteries. Acta Mechan 1–6. |
[10] |
Ma Z, Li T, Huang YL, et al. (2013) Critical silicon-anode size for averting lithiation-induced mechanical failure of lithium-ion batteries. RSC Adv 3: 7398–7402. doi: 10.1039/c3ra41052h
![]() |
[11] |
Haftbaradaran H, Gao H (2012) Ratcheting of silicon island electrodes on substrate due to cyclic intercalation. Appl Phys Lett 100: 121907. doi: 10.1063/1.3696298
![]() |
[12] |
Xie Z, Ma Z, Wang Y, et al. (2016) A kinetic model for diffusion and chemical reaction of silicon anode lithiation in lithium ion batteries. RSC Adv 6: 22383–22388. doi: 10.1039/C5RA27817A
![]() |
[13] |
Zhang P, Ma Z, Wang Y, et al. (2015) A first principles study of the mechanical properties of Li-Sn alloys. RSC Adv 5: 36022–36029. doi: 10.1039/C5RA04685H
![]() |
[14] |
Zhang P, Ma Z, Jiang W, et al. (2016) Mechanical properties of Li-Sn alloys for Li-ion battery anodes: A first-principles perspective. AIP Adv 6: 015107. doi: 10.1063/1.4940131
![]() |
[15] |
Gao X, Ma Z, Jiang W, et al. (2016) Stress-strain relationships of LixSn alloys for lithium ion batteries. J Power Sources 311: 21–28. doi: 10.1016/j.jpowsour.2016.02.024
![]() |
[16] | Sony press news (2005) Available from: www.Sony.net/SonyInfo/News/Press/200502/05-006 E/index.html. |
[17] |
Agubra VA, Zuniga L, Flores D, et al. (2016) Composite nano-fibers as advanced materials for Li-ion, Li-O2 and Li-S batteries. Electrochim Acta 192: 529–550. doi: 10.1016/j.electacta.2016.02.012
![]() |
[18] |
Aravindan V, Sundaramurthy J, Kumar PS, et al. (2015) Electrospun nanofibers: A prospective electro-active material for constructing high performance Li-ion batteries. Chem Commun 51: 2225–2234. doi: 10.1039/C4CC07824A
![]() |
[19] | Jung JW, Lee CL, Yu S, et al. (2016) Electrospun nanofibers as a platform for advanced secondary batteries: a comprehensive review. J Mater Chem A 4: 703–750. |
[20] |
Goodenough JB, KimY (2010) Challenges for rechargeable Li batteries. Chem Mater 22: 587–603. doi: 10.1021/cm901452z
![]() |
[21] | Liu Y, Yang Y (2016) Recent progress of TiO2-based anodes for Li ion batteries. J Nanomater 2016: 1–15. |
[22] | Zhang Y, Tang Y, Li W, et al. (2016) Nanostructured TiO2-based anode materials for high-performance rechargeable lithium-ion batteries. ChemNanoMat. |
[23] |
Rahman A, Wong Y, Song G, et al. (2015) A review on porous negative electrodes for high performance lithium-ion batteries. J Porous Mater 22: 1313–1343. doi: 10.1007/s10934-015-0010-1
![]() |
[24] | Niu J, Zhang S, Niu Y, et al. (2015) Silicon-based anode materials for lithium-ion batteries. Prog Chem 27: 1275–1290. |
[25] |
Roy P, Srivastava K (2015) Nanostructured anode materials for lithium ion batteries. J Mater Chem A 3: 2454–2484. doi: 10.1039/C4TA04980B
![]() |
[26] | Ji L, Lin Z, Alcoutlabi M, et al. (2011) Recent developments in nanostructured anode materials for rechargeable lithium-ion batteries. Energy Environ Sci 4: 22682–2699. |
[27] |
Zhang WJ (2011) A Review of the electrochemical performance of alloy anodes for lithium-ion batteries. J Power Sources 196: 13–24. doi: 10.1016/j.jpowsour.2010.07.020
![]() |
[28] | Lou S, Cheng X, Ma Y, et al. (2015) Nb-based oxides as anode materials for lithium ion batteries. Prog Chem 27: 297–309. |
[29] |
Lahiri I, Oh SM, Hwang JY, et al. (2011) Ultrathin alumina-coated carbon nanotubes as an anode for high-capacity Li-ion batteries. J Mater Chem 21: 13621–13626. doi: 10.1039/c1jm11474c
![]() |
[30] |
Leung K, Qi Y, Zavadil KR, et al. (2011) Using atomic layer deposition to hinder solvent decomposition in lithium-ion batteries: first principles modeling and experimental studies. J Am Chem Soc 133: 14741–14754. doi: 10.1021/ja205119g
![]() |
[31] |
De Las Cazas C, Li WZ (2012) A review of application of carbon nanotubes for lithium-ion battery anode material. J Power Sources 208: 74–85. doi: 10.1016/j.jpowsour.2012.02.013
![]() |
[32] | Fan S, Sun T, Rui X, et al. (2012) Cooperative enhancement of capacities in nanostructured SnSb/carbon nanotube network nanocomposite as anode for lithium ion batteries. J Power Sources 201: 288–293. |
[33] |
Wu Y, Wei Y, Wang J, et al. (2013) Conformal Fe3O4 sheath on aligned carbon nanotube scaffolds as high-performance anodes for lithium-ion batteries. Nano Lett 13: 818–823. doi: 10.1021/nl3046409
![]() |
[34] |
Ban C, Wu Z, Gillaspie DT, et al. (2010) Nanostructured Fe3O4/SWNT electrode: binder-free and high-rate Li-ion anode. Adv Energy Mater 22: E145–E149. doi: 10.1002/adma.200904285
![]() |
[35] |
Bindumadhavan K, Srivastava SK, Mahanty S (2013) MoS2-MWCNT hybrids as a superior anode in lithium-ion batteries. Chem Commun 49: 1823–1825. doi: 10.1039/c3cc38598a
![]() |
[36] |
Wang ZL, Xu D, Wang HG, et al. (2013) In-situ fabrication of porous graphene electrodes for high performance energy storage. ACS Nano 7: 2422–2430. doi: 10.1021/nn3057388
![]() |
[37] | Lavoie N, Courtel F, Malenfant PRL, et al. (2013) Graphene-based composite anodes for lithium-ion batteries, In: Nanotechnology for Lithium-Ion Batteries, ed. by Y. Abdu-Lebdeh, I. Davidson, Springer, New York. |
[38] |
Fu YS, Zhu JW, Hu C, et al. (2014) Covalently coupled hybrid of graphitic carbon nitride with reduced graphene oxide as a superior performance lithium-ion battery anode. Nanoscale 6: 12555–12564. doi: 10.1039/C4NR03145H
![]() |
[39] | Takamura T, Ohara S, Uehara M, et al. (2004) A vacuum deposited Si film having a Li extraction capacity over 2000 mAh g?1 with a long cycle life. J Power Sources 129: 96–100. |
[40] | Ohara S, Suzuki J, Sekine K, et al. (2003) Li insertion/extraction reaction at a Si film evaporated on a Ni foil. J Power Sources 119–121: 591–596. |
[41] |
Chen LB, Xie JY, Yu HC, et al. (2009) An amorphous thin film Si anode with high capacity and long cycling life for lithium ion batteries. J Appl Electrochem 39: 1157–1162. doi: 10.1007/s10800-008-9774-1
![]() |
[42] |
Ohara S, Suzuki J, Sekine K, et al. (2004) A thin film silicon anode for Li-ion batteries having a very large specific capacity and long cycle life. J Power Sources 136: 303–306. doi: 10.1016/j.jpowsour.2004.03.014
![]() |
[43] |
Kim JB, Lee, HY, Lee KS, et al. (2003) Fe/Si multi-layer thin film anodes for lithium rechargeable thin film batteries. Electrochem Commun 5: 544–548. doi: 10.1016/S1388-2481(03)00120-6
![]() |
[44] |
Lee HY, Lee SM (2002) Graphite-FeSi alloy composites as anode materials for rechargeable lithium ion batteries. J Power Sources 112: 649–654. doi: 10.1016/S0378-7753(02)00461-5
![]() |
[45] |
Yu C, Li X, Ma T, et al. (2012) Silicon thin films as anodes for high-performance lithium-ion batteries with effective stress relaxation. Adv Energy Mater 2: 68–73. doi: 10.1002/aenm.201100634
![]() |
[46] |
Liu Y, Hudak NS, Huber DL, et al. (2011) In-situ transmission electron microscopy observation of pulverization of aluminum nanowires and evolution of the thin surface Al2O3 layers during lithiation-delithiation cycles. Nano Lett 11: 4188–4194. doi: 10.1021/nl202088h
![]() |
[47] |
Xiao X, Lu P, Dahn J (2011) Ultrathin multifunctional oxide coatings for lithium ion batteries. Adv Mater 23: 3911–3915. doi: 10.1002/adma.201101915
![]() |
[48] |
He Y, Yu X, Wang Y, et al. (2011) Alumina-coated patterned amorphous silicon as the anode for a lithium ion battery with high coulombic efficiency. Adv Mater 23: 4938–4941. doi: 10.1002/adma.201102568
![]() |
[49] |
Ge M, Rong J, Fang X, et al. (2012) Porous doped silicon nanowires for lithium ion battery anode with long cycle life. Nano Lett 12: 2318–2323. doi: 10.1021/nl300206e
![]() |
[50] |
Kim H, Cho J (2008) Superior lithium electroactive mesoporous Si@carbon core-shell nanowires for lithium battery anode material. Nano Lett 8: 3688–3691. doi: 10.1021/nl801853x
![]() |
[51] | Lytle JC (2013) Inverse opal nanoarchitecture as lithium ion anode materials. In: Nanotechnology for Lithium-Ion Batteries, ed. by Y. Abdu-Lebdeh, I. Davidson, Springer, New York. |
[52] |
Chen HX, Dong ZX, Fu YP, et al. (2010) Silicon nanowires with and without carbon coating as anode materials for lithium ion batteries. J Solid State Electrochem 14: 1829–1834. doi: 10.1007/s10008-009-1001-4
![]() |
[53] |
Sethuraman VA, Kowolik K, Sirinavan V (2011) Increased cycling efficiency and rate capability of copper-coated silicon anode materials for lithium-ion batteries. J Power Sources 196: 6657–6662. doi: 10.1016/j.jpowsour.2010.12.075
![]() |
[54] |
Yao Y, Liu N, McDowell MT, et al. (2012) Improving the cycling stability of silicon nanowire anodes with conducting polymer coatings. Energy Environ Sci 5: 7927–7930. doi: 10.1039/c2ee21437g
![]() |
[55] |
Memarzadeh EL, Kalisvaart WP, Kohandehghan A, et al. (2012) Silicon nanowire core aluminium shell coaxial nanocomposites for lithium ion anodes grown with and without a TiN interlayer. J Mater Chem 22: 6655–6668. doi: 10.1039/c2jm16167b
![]() |
[56] |
Ruy I, Choi JW, Cui Y, et al. (2011) Size dependent fracture of Si nanowire battery anodes. J Mechan Phys Solids 59: 1717–1730. doi: 10.1016/j.jmps.2011.06.003
![]() |
[57] |
Liu XH, Zheng H, Zhong L, et al. (2011) Anisotropic swelling and fracture of silicon nanowires during lithiation. Nano Lett 11: 3312–3318. doi: 10.1021/nl201684d
![]() |
[58] |
Nguyen HT, Zamfir MR, Duong LD, et al. (2012) Alumina-coated silicon-based nanowire arrays for high quality Li-ion battery anodes. J Mater Chem 22: 24618–24626. doi: 10.1039/c2jm35125k
![]() |
[59] | Wu H, Chan G, Choi JW, et al. (2012) Stable cycling for double-walled silicon nanotubes battery anodes through solid-electrolyte interphase control. Nano Technol 7: 310–315. |
[60] |
Song T, Cheng HY, Choi H, et al. (2012) Si/Ge double-layered nanotube array as a lithium ion battery anode. ACS Nano 6: 303–309. doi: 10.1021/nn203572n
![]() |
[61] |
Rong J, Fang X, Ge M, et al. (2013) Coaxial Si/anodic titanium oxide/Si nanotube arrays for lithium-ion battery anodes. Nano Res 6: 182–190. doi: 10.1007/s12274-013-0294-x
![]() |
[62] | Choi NS, Yao Y, Cui Y, et al. (2011) One-dimensional Si/Sn-based nanowires and nanotubes for lithium-ion energy storage materials. J Mater Chem 21:9 825–9840. |
[63] |
Evanoff K, Kahn J, Balandin AA, et al. (2012) Towards ultra-thick battery electrodes: aligned carbon nanotube, enabled architecture. Adv Mater 24: 533–537. doi: 10.1002/adma.201103044
![]() |
[64] |
Krishnan R, Lu TM, Koratkar N (2011) Functionally strain-graded nanoscoops for High Power Li-Ion Battery Anodes. Nano Lett 11: 377–384. doi: 10.1021/nl102981d
![]() |
[65] |
Kim H, Han B, Choo J, et al. (2008) Three-dimensional porous silicon particles for uses in high-performance lithium secondary batteries. Angew Chem 47: 10151–10154. doi: 10.1002/anie.200804355
![]() |
[66] |
Son IH, Park JH, Kwon S, et al. (2015) Silicon carbide-free graphene growth on silicon for lithium-ion battery with high volumetric energy density. Nature Commun 6: 7393. doi: 10.1038/ncomms8393
![]() |
[67] |
Wu H, Zheng G, Liu N, et al. (2012) Engineering empty space between Si nanoparticles for lithium-ion battery anodes. Nano Lett 12: 904–909. doi: 10.1021/nl203967r
![]() |
[68] |
Liu N, Wu H, McDowell MT, et al. (2012) A yolk-shell design for stabilized and scalable li-ion battery alloy anodes. Nano Lett 12: 3315–3325. doi: 10.1021/nl3014814
![]() |
[69] |
Liu N, Lu Z, Zhao J, et al. (2014) A pomegranate-inspired nanoscale design for large-volume-change lithium battery anodes. Nat Nanotechnol 9: 187–190. doi: 10.1038/nnano.2014.6
![]() |
[70] |
Chou SL, Wang JZ, Choucair M, et al. (2010) Enhanced reversible lithium storage in a nanosize silicon/graphene composite. Electrochem Commun 12: 303–306. doi: 10.1016/j.elecom.2009.12.024
![]() |
[71] |
Wang JZ, Zhong C, Chou SL, et al. (2010) Flexible free-standing graphene-silicon composite film for lithium-ion batteries. Electrochem Commun 12: 1467–1470. doi: 10.1016/j.elecom.2010.08.008
![]() |
[72] |
Lee JK, Smith KB, Hayner CM, et al. (2010) Silicon nanoparticles-graphene paper composites for Li ion battery anodes. Chem Commun 46: 2025–2027. doi: 10.1039/b919738a
![]() |
[73] |
Chen S, Bao P, Huang X, et al. (2014) Hierarchical 3D mesoporous silicon@graphene nanoarchitectures for lithium ion batteries with superior performance. Nano Res 7: 85–94. doi: 10.1007/s12274-013-0374-y
![]() |
[74] |
Zhao X, Hayner CM, Kung MC, et al. (2011) In-plane vacancy-enabled high-power Si-graphene composite electrode for lithium-ion batteries. Adv Energy Mater 1: 1079–1084. doi: 10.1002/aenm.201100426
![]() |
[75] |
Zhao X, Li M, Chang KH, et al. (2014) Composites of graphene and encapsulated silicon for practically viable high-performance lithium-ion batteries. Nano Res 7: 1429–1438. doi: 10.1007/s12274-014-0463-6
![]() |
[76] |
Zamfir MR, Nguyen HT, Moyen E, et al. (2013) Silicon nanowires for Li-based battery anodes: a review. J Mat Chem A 1: 9566–9586. doi: 10.1039/c3ta11714f
![]() |
[77] |
Wen Z, Lu G, Mao S, et al. (2013) Silicon nanotube anode for lithium-ion batteries. Electrochem Commun 29: 67–70. doi: 10.1016/j.elecom.2013.01.015
![]() |
[78] |
Baggetto L, Notten PHN (2009) Lithium ion (de)insertion reaction of germanium thin-film electrodes and electrochemical and in-situ XRD study batteries and energy storage. J Electrochem Soc 156: A169–A175. doi: 10.1149/1.3055984
![]() |
[79] |
Liu XH, Huang S, Picraux ST, et al. (2011) Reversible nanopore formation in Ge nanowires during lithiation-delithiation cycling: an in-situ transmission electron microscopy study. Nano Lett 11: 3991–3997. doi: 10.1021/nl2024118
![]() |
[80] |
Liang W, Yang H, Fan F, et al. (2013) Tough germanium nanoparticles under electrochemical cycling. ACS Nano 7: 3427–3433. doi: 10.1021/nn400330h
![]() |
[81] |
Graetz J, Ahn CC, Yazami R, et al. (2004) Nano crystalline and thin film germanium electrodes with high lithium capacity and high rate capabilities. J Electrochem Soc 151: A698–A702 doi: 10.1149/1.1697412
![]() |
[82] |
Ren JG, Wu Q-H, Tang H, et al. (2013) Germanium-graphene composite anode for high-energy lithium batteries with long cycle life. J Mater Chem A 1: 1821–1826. doi: 10.1039/C2TA01286C
![]() |
[83] |
Fang S, Shan L, Zheng H, et al. (2015) Ge-graphene-carbon nanotube composite anode for high performance lithium-ion batteries. J Mater Chem A 3: 1498–1503. doi: 10.1039/C4TA04350B
![]() |
[84] |
DiLeo RA, Frisco S, Ganter MJ, et al. (2011) Hybris germanium nanoparticle—single wall carbon nanotube free-standing anodes for lithium batteries. J Phys Chem C 115: 22609–22614. doi: 10.1021/jp205992w
![]() |
[85] |
Ashuri M, He Q, Shaw LL (2016) Silicon as a potential anode material for Li-ion batteries: where size, geometry and structure matter. Nanoscale 8: 74–103. doi: 10.1039/C5NR05116A
![]() |
[86] | Kamali AR, Fray DJ (2011) Tin-based as advanced anode materials for lithium-ion batteries: a review. Rev Adv Mater Sci 27: 14–24. |
[87] |
Youn DH, Heller A, Mullins CB (2016) Simple synthesis of nanostructured Sn/nitrogen-doped carbon composite using nitrilotriacetic acid as lithium ion Battery Anode. Chem Mater 28: 1343–1347. doi: 10.1021/acs.chemmater.5b04282
![]() |
[88] |
Yu Y, Gu L, Wang C, et al. (2009) Encapsulation of Sn-carbon nanoparticles in bamboo-like hollow carbon nanofibers as an anode material in lithium-based batteries. Angew Chem Int Ed 48: 6485–6489. doi: 10.1002/anie.200901723
![]() |
[89] |
Wang Y, Ma Z, Lu C (2016) A twins-structural Sn@C core-shell composite as anode materials for lithium-ion batteries. Compos Interface 23: 273–280. doi: 10.1080/09276440.2016.1136523
![]() |
[90] |
Liang S, Zhu X, Lian P, et al. (2011) Superior cycle performance of Sn@C/graphene nanocomposite as an anode material for lithium-ion batteries. J Solid Stat Chem 184: 1400–1404. doi: 10.1016/j.jssc.2011.03.052
![]() |
[91] |
Agubra VA, Zuniga L, De La Garza D, et al. (2016) Forcespinning: A new method for the mass production of Sn/C composite nano fiber anodes for lithium ion batteries. Solid State Ionics 286: 72–82. doi: 10.1016/j.ssi.2015.12.020
![]() |
[92] |
Beaulieu LY, Dahn JR (2000) The reaction of lithium with Sn-Mn-C intermetallics prepared by mechanical alloying. J Electrochem Soc 147: 3237–3241. doi: 10.1149/1.1393889
![]() |
[93] | Mao O, Dunlap RA, Dahn JR (1999) Mechanically alloyed Sn-Fe-C powders as anode materials for Li-ion batteries. I. The Sn2Fe-C system. J Electrochem Soc 146: 405–413. |
[94] | Mao O, Dahn JR (1999) Mechanically alloyed Sn-Fe-C powders as anode materials for Li-ion batteries. II. The Sn-Fe system. J Electrochem Soc 146: 414–422. |
[95] | Mao O, Dahn J (1999) Mechanically alloyed Sn-Fe-C powders as anode materials for Li-ion batteries. III. Sn2Fe: SnFe3C active/inactive composites. J Electrochem Soc 146: 423–427. |
[96] | Dahn JR, Mar RE, Abouzeid A (2006) Combinatorial study of Sn1-xCox (0 < x < 0.6) and [Sn0.55Co0.45]1-yCy (0 < y < 0.5) alloy negative electrode materials for Li-ion batteries. J Electrochem Soc 153: A361–A365. |
[97] |
Hassoun J, Ochal P, Panero S, et al. (2008) The effect of CoSn/CoSn2 phase ratio on the electrochemical behavior of Sn40Co40C20 ternary alloy electrodes in Li cells. J Power Sources 180: 568–575. doi: 10.1016/j.jpowsour.2008.01.059
![]() |
[98] |
Hassoun J, Mulas G, Panero S, et al. (2007) Ternary Sn-Co-C Li-ion battery electrode material prepared by high energy ball milling. Electrochem Commun 9: 2075–2081. doi: 10.1016/j.elecom.2007.05.033
![]() |
[99] |
Mukaibo H, Sumi T, Yokoshima T, et al. (2003) Electrodeposited Sn-Ni alloy film as a high capacity anode material for lithium-ion secondary batteries. Electrochem Solid-State Lett 6: A218–A220. doi: 10.1149/1.1602331
![]() |
[100] |
Wolfenstine J, Campos S, Foster D, et al. (2002) Nano-scale Cu6Sn5 anodes. J Power Sources 109: 230–233. doi: 10.1016/S0378-7753(02)00061-7
![]() |
[101] |
Ferguson PP, Todd ADW, Dahn JR (2008) Comparison of mechanically alloyed and suttered tin-cobalt-carbon as an anode material for lithium-ion batteries. Electrochem Commun 10: 25–31. doi: 10.1016/j.elecom.2007.10.025
![]() |
[102] |
Hassoun J, Panero S, Mulas G, et al. (2007) An electrochemical investigation of a Sn-Co-C ternary alloy as a negative electrode in Li-ion batteries. J Power Sources 171: 928–931. doi: 10.1016/j.jpowsour.2007.06.067
![]() |
[103] | Sony corporation (2011) Sony the market for notebook PC; development of a tin-based amorphous anode for high-capacity rechargeable lithium-ion battery 3.5 Ah: the “Nexelion”. Available from: http:www.sony.co.jp/SonyInfo/News/Press/201107/11-078/. |
[104] |
Lei WX, Pan Y, Zhou YC, et al. (2014) CNTs-Cu composite layer enhanced Sn-Cu alloy as high performance anode materials for lithium-ion batteries. RSC Adv 4: 3233–3237. doi: 10.1039/C3RA44431G
![]() |
[105] |
Meschini I, Nobili F, Mancini M, et al. (2013) High performance Sn@carbon nanocomposite anode for lithium batteries. J Power Sources 226: 241–248. doi: 10.1016/j.jpowsour.2012.11.004
![]() |
[106] |
Jiang W, Zeng W, Ma Z, et al. (2014) Advanced amorphous nanoporous stannous oxide composite with carbon nanotubes as anode materials for lithium-ion batteries. RSC Adv 4: 41281–41286. doi: 10.1039/C4RA06968D
![]() |
[107] |
Zhu XJ, Guo ZP, Zhang P, et al. (2009) Highly porous reticular tin-cobalt oxide composite thin film anodes for lithium ion batteries. J Mater Chem 19: 8360–8365. doi: 10.1039/b913993a
![]() |
[108] | Zhu XJ, Guo ZP, Zhang P, et al. (2010) Three-dimensional reticulartin-manganese oxide composite anode materials for lithium ion batteries. Electrochim Acta 55: 4982–4986. |
[109] | Chen JS, Cheah YL, Chen YT, et al. (2009) SnO2 nanoparticles with controlled nano-coating as high-capacity anode for lithium-ion batteries. J Phys Chem 113: 20504–20508. |
[110] |
He M, Yuan L, Zhang W, et al. (2013) A SnO2-carbon nanocluster anode material with superior cyclablity and rate capability for lithium batteries. Nanoscale 5: 3298–3305. doi: 10.1039/c3nr34133j
![]() |
[111] |
Hassan MF, Rahman MM, Guo Z, et al. (2010) SnO2-NiO-C nanocomposite as a high capacity anode material for lithium-ion batteries. J Mater Chem 20: 9707–9712. doi: 10.1039/c0jm01341b
![]() |
[112] |
Yesibolati N, Shahid M, Chen W, et al. (2014) SnO2 anode surface passivation by atomic layer deposited HfO2 improves Li-ion battery performance. Small 10: 2849–2858. doi: 10.1002/smll.201303898
![]() |
[113] |
Du Z, Zhang S, Jiang T, et al. (2012) Facile synthesis of SnO2 nanocrystals coated conductive polymer nanowires for enhanced lithium storage. J Power Sources 219: 199–203. doi: 10.1016/j.jpowsour.2012.07.052
![]() |
[114] |
Lou XW, Li CM, Archer LA (2009) Design synthesis of coaxial SnO2@carbon hollow nanospheres for highly reversible lithium storage. Adv Mater 21: 2536–2539. doi: 10.1002/adma.200803439
![]() |
[115] | Wang Z, Fierke MA, Stein A (2008) Porous carbon/tin oxide monoliths as anodes for lithium-ion batteries. J Electrochem Soc 155: A658–A663. |
[116] |
Zhang L, Wu HB, Liu B, et al. (2014) Formation of porous SnO2 microboxes via selective leaching for highly reversible lithium storage. Energy Environ Sci 7: 1013–1017. doi: 10.1039/c3ee43305f
![]() |
[117] |
Du G, Zhong C, Zhang P, et al. (2010) Tin dioxyde/carbon nanotube composites with high uniform SnO2 loading as anode materials for lithium ion batteries. Electrochim Acta 55: 2582–2586. doi: 10.1016/j.electacta.2009.12.031
![]() |
[118] |
Ren JR, Yang J, Abouimrane A, et al. (2011) SnO2 nanocrystals deposited on multiwalled carbon nanotubes with superior stability as anode material for Li-ion batteries. J Power Sources 196: 8701–8705. doi: 10.1016/j.jpowsour.2011.06.036
![]() |
[119] |
Zhu CL, Zhang ML, Qiao YJ, et al. (2010) High capacity and good cycling stability of multi-walled carbon nanotube/SnO2 core-shell structures as anode materials of lithium-ion batteries. Mater Res Bull 45: 437–441. doi: 10.1016/j.materresbull.2009.11.011
![]() |
[120] |
Wang Y, Zeng HC, Lee JY (2006) Highly reversible lithium storage in porous SnO2 nanotubes with coaxially grown carbon nanotubes overlayers. Adv Mater 18: 645–649. doi: 10.1002/adma.200501883
![]() |
[121] | Zhang J, Ma Z, Jiang W, et al. (2016) Sandwich-like CNTs@SnO2/SnO/Sn anodes on three-dimensional Ni foam substrate for lithium ion batteries. J Electroanal Chem 767: 49–55. |
[122] | Li YM, Lv XJ, Lu J, et al. (2010) Preparation of SnO2-nanocrystal/graphene-nanosheets composites and their lithium storage ability. J Phys Chem C 114: 21770–21774. |
[123] |
Zhao B, Zhang GH, Song JS, et al. (2011) Bivalent tin ion assisted reduction for preparing graphene/SnO2 composite with good cyclic performance and lithium storage capacity. Electrochim Acta 56: 7340–7346. doi: 10.1016/j.electacta.2011.06.037
![]() |
[124] |
Zhong C, Wang JZ, Chen ZX, et al. (2011) Photoinduced optical transparency in dye-sensitized solar cells containing graphene nanoribbons. J Phys Chem C 115: 25115–25131. doi: 10.1021/jp2061128
![]() |
[125] |
Lian PC, Zhu XF, Liang SZ, et al. (2011) High reversible capacity of SnO2/graphene nanocomposite as an anode material for lithium-ion batteries. Electrochim Acta 56: 4532–4539. doi: 10.1016/j.electacta.2011.01.126
![]() |
[126] |
Huang XD, Zhou XF, Zhou LA, et al. (2011) A facile one-step solvothermal synthesis of SnO2/graphene nanocomposite and its application as an anode material for lithium-ion batteries. ChemPhysChem 12: 278–281. doi: 10.1002/cphc.201000376
![]() |
[127] | Xie J, Liu SY, Chen XF, et al. (2011) Nanocrystal-SnO2-loaded graphene with improved Li-storage properties prepared by a facile one-pot hydrothermal route. Int J Electrochem Sci 6: 5539–5549. |
[128] |
Baek S, Yu SH, Park SK, et al. (2011) A one-pot microwave-assisted non-aqueous sol-gel approach to metal oxide/graphene nanocomposites for Li-ion batteries. RSC Adv 1: 1687–1690. doi: 10.1039/c1ra00797a
![]() |
[129] |
Wang XY, Zhou XF, Yao K, et al. (2011) A SnO2/graphene composite as a high stability electrode for lithium ion batteries. Carbon 49: 133–139. doi: 10.1016/j.carbon.2010.08.052
![]() |
[130] |
Xu CH, Sun J, Gao L (2012) Direct growth of monodisperse SnO2 nanorods on graphene as high capacity anode materials for lithium ion batteries. J Mater Chem 22: 975–979. doi: 10.1039/C1JM14099J
![]() |
[131] |
Lin J, Peng Z, Xiang C, et al. (2013) Graphene nanoribbon and nanostructured SnO2 composite anodes for lithium ion batteries. ACS Nano 7: 6001–6007. doi: 10.1021/nn4016899
![]() |
[132] |
Zhou X, Wan LJ, Guo YG (2013) Binding SnO2 nanocrystals in nitrogen-doped graphene sheets as anode materials for lithium-ion batteries. Adv Mater 25: 2152–2157. doi: 10.1002/adma.201300071
![]() |
[133] | Botas C, Carriazo D, Singh G, et al. (2015) Sn- and SnO2-graphene flexible foams suitable as binder-free anodes for lithium ion batteries. J Mater Chem A 25: 13402–13410. |
[134] |
Jiang W, Zhao X, Ma Z, et al. (2016) SnO2/reduced graphene oxide nanocomposite as anode material for lithium-ion batteries with enhanced cyclability. J Nanosci Nanotech 16: 4136–4140. doi: 10.1166/jnn.2016.12541
![]() |
[135] |
Sun J, Xiao L, Jiang S, et al. (2015) Fluorine-doped SnO2@graphene porous composite for high capacity lithium-ion batteries. Chem Mater 27: 4594–4603. doi: 10.1021/acs.chemmater.5b00885
![]() |
[136] |
Liu L, An M, Yang P, et al. (2015) Superior cycle performance and high reversible capacity of SnO2/graphene composite as an anode material for lithium-ion batteries. Sci Rep 5: 9055. doi: 10.1038/srep09055
![]() |
[137] |
Deng Y, Fang C, Chen G (2016) The developments of SnO2/graphene nanocomposites as anode materials for high performance lithium ion batteries: a review. J Power Sources 304: 81–101. doi: 10.1016/j.jpowsour.2015.11.017
![]() |
[138] |
Liu S, Wang R, Liu M, et al. (2014) Fe2O3-SnO2 nanoparticle decorated graphene flexible films as high-performance anode materials for lithium-ion batteries. J Mater Chem A 2: 4598–4604. doi: 10.1039/c3ta14897a
![]() |
[139] |
Bhaskar A, Deepa M, Ramakrishna M, et al. (2014) Poly(3,4-ethylenedioxythiophene) sheath over a SnO2 hollow spheres/graphene oxide hybrid for a durable anode in Li-ion batteries. J Phys Chem C 118: 7296–7306. doi: 10.1021/jp412038y
![]() |
[140] | Ren H, Yu RB, Wang JY, et al. (2014) Multishelled TiO2 hollow microspheres as anodes with superior reversible capacity for lithium ion batteries. Nano Lett 14: 6679–6684. |
[141] |
Wang HY, Chen JZ, Hy S, et al. (2014) High-surface-area mesoporous TiO2 microspheres via one-step nanoparticle self-assembly for enhanced lithium-ion storage. Nanoscale 6: 14926–14931. doi: 10.1039/C4NR04729J
![]() |
[142] |
Xia T, Zhang W, Wang Z, et al. (2014) Amorphous carbon-coated TiO2 nanocrystals for improved lithium-ion battery and photocatalytic performance. Nano Energy 6: 109–118. doi: 10.1016/j.nanoen.2014.03.012
![]() |
[143] |
Acevedo-Peña P, Haro M, Rincón ME, et al. (2014) Facile kinetics of Li-ion intake causes superior rate capability in multiwalled carbon nanotube@TiO2 nanocomposite battery anodes. J Power Sources 268: 397–403. doi: 10.1016/j.jpowsour.2014.06.058
![]() |
[144] | Jiang X, Yang X, Zhu Y, et al. (2014) 3D nitrogen-doped graphene foams embedded with ultrafine TiO2 nanoparticles for high-performance lithium-ion batteries. J Mater Chem A 2: 11124–11133. |
[145] | Fu Y, Ming H, Zhou Q, et al. (2014) Nitrogen-doped carbon coating inside porous TiO2 using small nitrogen-containing molecules for improving performance of lithium-ion batteries. Electrochim Acta 134: 478–485. |
[146] |
Chen WN, Jiang H, Hu YJ, et al. (2014) Mesoporous single crystals Li4Ti5O12 grown on rGO as high-rate anode materials for lithium-ion batteries. Chem Commun 50: 8856–8859. doi: 10.1039/C4CC02886D
![]() |
[147] |
Xin X, Zhou XF, Wu JH, et al. (2012) Scalable synthesis of TiO2/graphene nanostructured composite with high-rate performance for lithium ion batteries. ACS Nano 6: 11035–11043. doi: 10.1021/nn304725m
![]() |
[148] |
Mo RW, Lei ZY, Sun KN, et al. (2014) Facile synthesis of anatase TiO2 quantum-dot/graphene-nanosheet composites with enhanced electrochemical performance for lithium-ion batteries. Adv Mater 26: 2084–2088. doi: 10.1002/adma.201304338
![]() |
[149] |
Wang J, Zhou Y, Xiong B, et al. (2013) Fast lithium-ion insertion of TiO2 nanotube and graphene composites. Electrochim Acta 88: 847–857. doi: 10.1016/j.electacta.2012.10.010
![]() |
[150] |
Zhang X, Zhang J, Liu Y, et al. (2015) Improving the anode performances of TiO2-carbon-rGO composites in lithium ion batteries by UV irradiation. New J Chem 39: 9345–9350. doi: 10.1039/C5NJ01855B
![]() |
[151] |
Zha CY, He DF, Zou JW, et al. (2014) A minky-dot-fabric-shaped composite of porous TiO2 microsphere/reduced graphene oxide for lithium ion batteries. J Mater Chem A 2: 16931–16938. doi: 10.1039/C4TA03675A
![]() |
[152] |
Fang Y, Lv Y, Che R, et al. (2013) Two-dimensional mesoporous carbon nanosheets and their derived graphene nanosheets: synthesis and efficient lithium ion storage. J Am Chem Soc 135: 1524–1530. doi: 10.1021/ja310849c
![]() |
[153] |
Lan T, Kiu H, Xie F, et al. (2015) Rutile TiO2mesocrystals/reduced graphene oxide with high-rate and long-term performance for lithium-ion batteries. Sci Rep 5: 8498. doi: 10.1038/srep08498
![]() |
[154] |
Liu H, Bi Z, Sun XG, et al. (2011) Mesoporous TiO2-B microspheres with superior rate performance for lithium ion batteries. Adv Mater 23: 3450–3454. doi: 10.1002/adma.201100599
![]() |
[155] |
Yang Z, Du G, Guo Z, et al. (2011) TiO2(B)-carbon composite nanowires as anode for lithium ion batteries with enhanced reversible capacity and cyclic performance. J Mater Chem 21: 8591–8596. doi: 10.1039/c0jm03873c
![]() |
[156] |
Yan X, Li Y, Li M, et al. (2015) Ultrafast lithium storage in TiO2-bronze nanowires/N-doped graphene nanocomposites. J Mater Chem A 3: 4180–4187. doi: 10.1039/C4TA06361A
![]() |
[157] |
Etacheri V, Yourey JE, Bartlett BM (2014) Chemically bonded TiO2-Bronze nanosheet/reduced graphene oxide hybrid for high-power lithium ion batteries. ACS Nano 8: 1491–1499. doi: 10.1021/nn405534r
![]() |
[158] |
Li MS, Li XF, Li WH, et al. (2015) Atomic layer deposition derived amorphous TiO2 thin film decorating graphene nanosheets with superior rate capability. Electrochem Commun 57: 43–47. doi: 10.1016/j.elecom.2015.05.005
![]() |
[159] |
Luo J, Xia X, Luo Y, et al. (2013) Rationally designed hierarchical TiO2/Fe2O3 hollow nanostructures for improved lithium ion storage. Adv Energy Mater 3: 737–743. doi: 10.1002/aenm.201200953
![]() |
[160] |
Liao JY, Higgins D, Lui G, et al. (2013) Multifunctional TiO2-C/MnO2 core-double-shell nanowire arrays as high-performance 3D electrodes for lithium ion batteries. Nano Lett 13: 5467–5473. doi: 10.1021/nl4030159
![]() |
[161] |
Jeun JH, Park KY, Kim DH, et al. (2013) SnO2@TiO2 double-shell nanotubes for a lithium ion battery anode with excellent high rate cyclability. Nanoscale 5: 8480–8483. doi: 10.1039/c3nr01964k
![]() |
[162] |
Jung HG, Myung ST, Yoon CS, et al. (2011) Microscale spherical carbon-coated Li4Ti5O12 as ultra high power anode material for lithium batteries. Energy Environ Sci 4: 1345–1351. doi: 10.1039/c0ee00620c
![]() |
[163] |
Zaghib K, Dontigny M, Guerfi A, et al. (2012) An improved high-power battery with increased thermal operating range: C-LiFePO4/C-Li4Ti5O12. J Power Sources 216: 192–200. doi: 10.1016/j.jpowsour.2012.05.025
![]() |
[164] |
Li B, Han C, He YB, et al. (2012) Facile synthesis of Li4Ti5O12/C composite with super rate performance. Energy Environ Sci 5: 9595–9602. doi: 10.1039/c2ee22591c
![]() |
[165] |
Zhao L, Hu YS, Li H, et al. (2011) Porous Li4Ti5O12 coated with N-doped carbon from ionic liquids for Li-ion batteries. Adv Mater 23: 1385–1388. doi: 10.1002/adma.201003294
![]() |
[166] | Fan L, Tan X, Yu T, et al. (2016) Li4Ti5O12/hollow graphitized nano-carbon composites as anode materials for lithium ion battery. RCS Adv 6: 26406–26411. |
[167] |
Shen L, Yuan C, Luo H, et al. (2011) Novel template-free solvothermal synthesis of mesoporous Li4Ti5O12-C microspheres for high power lithium ion batteries. J Mater Chem 21: 14414–14416. doi: 10.1039/c1jm12324f
![]() |
[168] | Lin C, Fan X, Yin Y, et al. (2014) Mono-dispersed mesoporous Li4Ti5O12 submicrospheres as anode materials for lithium-ion batteries: morphology and electrochemical performances. Nanoscale 21: 6651–6650. |
[169] |
Jhan YR, Duh JG (2012) Synthesis of entanglement structure in nanosized Li4Ti5O12/multi-walled carbon nanotubes composite anode material for Li-ion batteries by ball-milling-assisted solid-state reaction. J Power Sources 198: 294–297. doi: 10.1016/j.jpowsour.2011.09.063
![]() |
[170] |
Shi Y, Wen L, Li F, et al. (2011) Nanosized Li4Ti5O12/graphene hybrid materials with low polarization for high rate lithium ion batteries. J Power Sources 196: 8610–8617. doi: 10.1016/j.jpowsour.2011.06.002
![]() |
[171] |
Li X, Qu M, Huai Y, et al. (2010) Preparation and electrochemical performance of Li4Ti5O12/carbon/carbon nanotubes for lithium ion battery. Electrochim Acta 55: 2978–2982. doi: 10.1016/j.electacta.2010.01.015
![]() |
[172] |
Jhan YR, Duh JG (2012) Synthesis of entanglement structure in nanosized Li4Ti5O12/multi-walled carbon nanotubes composite anode material for Li-ion batteries by ball-milling-assisted solid-state reaction. J Power Sources 198: 294–297. doi: 10.1016/j.jpowsour.2011.09.063
![]() |
[173] |
Ni H, Fan LZ (2012) Nano-Li4Ti5O12 anchored on carbon nanotubes by liquid phase deposition as anode material for high rate lithium-ion batteries. J Power Sources 214: 195–199. doi: 10.1016/j.jpowsour.2012.04.074
![]() |
[174] | Shen L, Yuan C, Luo H, et al. (2012) In situ growth of Li4Ti5O12 on multi-walled carbon nanotubes: novel coaxial nanocables for high rate lithium ion batteries. J Mater Chem 21: 761–767. |
[175] |
Zhu N, Liu W, Xue MQ, et al. (2010) Graphene as a conductive additive to enhance the high-rate capabilities of electrospun Li4Ti5O12 for lithium-ion batteries. Electrochim Acta 55: 5813–5818. doi: 10.1016/j.electacta.2010.05.029
![]() |
[176] |
Ge H, Hao T, Osgood H, et al. (2016) Advanced mesoporous spinel Li4Ti5O12/rGO composites with increased surface lithium storage capability for high-power lithium-ion batteries. ACS Appl Mater Inter 8: 9162–9169. doi: 10.1021/acsami.6b01644
![]() |
[177] |
Chen C, Agrawal R, Wang C (2015) High performance Li4Ti5O12/Si composite anodes for Li-ion batteries. Nanomaterials 5: 1469–1480. doi: 10.3390/nano5031469
![]() |
[178] | Li N, Mei T, Zhu Y, et al. (2012) Hydrothermal synthesis of layered Li1.81H0.19Ti2O5·xH2O nanosheets and their transformation to single-crystalline Li4Ti5O12 nanosheets as the anode materials for Li-ion batteries. CrystEngComm 14: 6435–6440. |
[179] |
Liu GY, Wang HY, Liu GQ, et al. (2013) Synthesis and electrochemical performance of high-rate dual-phase Li4Ti5O12-TiO2 nanocrystallines for Li-ion batteries. Electrochim Acta 87: 218–223. doi: 10.1016/j.electacta.2012.09.010
![]() |
[180] |
Xu C, Xue L, Zhang W, et al. (2014) Hydrothermal synthesis of Li4Ti5O12/TiO2 nano-composite as high performance anode material for Li-ion batteries. Electrochim Acta 147: 506–512. doi: 10.1016/j.electacta.2014.09.060
![]() |
[181] |
Zhong H, Yang G, Song H, et al. (2012) Vertically aligned graphene-like SnS2 ultrathin nanosheet arrays: excellent energy storage, catalysis, photoconduction, and field-emitting performances. J Phys Chem C 116: 9319–9326. doi: 10.1021/jp301024d
![]() |
[182] |
Du Y, Yin Z, Rui X, et al. (2013) A facile, relative green, and inexpensive synthetic approach toward large-scale production of SnS2 nanoplates for high-performance lithium-ion batteries. Nanoscale 5: 1456–1459. doi: 10.1039/c2nr33458e
![]() |
[183] |
He M, Yuan LX, Huang YH (2013) Acetylene black incorporated three-dimensional porous SnS2 nanoflowers with high performance for lithium storage. RSC Adv 3: 3374–3383. doi: 10.1039/c2ra22764a
![]() |
[184] |
Li J, Wu P, Lou F, et al. (2013) Mesoporous carbon anchored with SnS2 nanosheets as an advanced anode for lithium-ion batteries. Electrochim Acta 111: 862–868. doi: 10.1016/j.electacta.2013.08.104
![]() |
[185] |
Sun H, Ahmad M, Luo J, et al. (2014) SnS2 nanoflakes decorated multiwalled carbon nanotubes as high performance anode materials for lithium-ion batteries. Mater Res Bull 49: 319–324. doi: 10.1016/j.materresbull.2013.09.005
![]() |
[186] | Balogun MS, Qiu W, Jian J, et al. (2015) Vanadium nitride nanowire supported SnS2 nanosheets with high Reversible capacity as anode material for lithium ion batteries. ACS Appl Mater Inter 7: 23205–23215. |
[187] |
Wang Q, Huang Y, Miao J, et al. (2013) Graphene-supported Ce-SnS2 nanocomposite as anode material for lithium-ion batteries. J Am Ceram Soc 96: 2190–2196. doi: 10.1111/jace.12302
![]() |
[188] |
Chen P, Su Y, Liu H, et al. (2013) Interconnected tin disulfide nanosheets grown on graphene for Li-ion storage and photocatalytic applications. ACS Appl Mater Inter 5: 12073–12082. doi: 10.1021/am403905x
![]() |
[189] |
Chang K, Wang Z, Huang G, et al. (2012) Few-layer SnS2/graphene hybrid with exceptional electrochemical performance as lithium-ion battery anode. J Power Sources 201: 259–266. doi: 10.1016/j.jpowsour.2011.10.132
![]() |
[190] |
Zhuo L, Wu Y, Wang L, et al. (2012) One-step hydrothermal synthesis of SnS2/graphene composites as anode material for highly efficient rechargeable lithium ion batteries. RSC Adv 2: 5084–5087. doi: 10.1039/c2ra00002d
![]() |
[191] |
Yin J, Cao H, Zhou Z, et al. (2012) SnS2@reduced graphene oxide nanocomposites as anode materials with high capacity for rechargeable lithium ion batteries. J Mater Chem 22: 23963–23970. doi: 10.1039/c2jm35137d
![]() |
[192] |
Wang Q, Nie YX, He B, et al. (2014) SnS2/graphene nanocomposites as anodes of lithium-ion batteries. Solid State Sci 31: 81–84. doi: 10.1016/j.solidstatesciences.2014.03.001
![]() |
[193] |
Liu S, Lu X, Xie J, et al. (2013) Preferential c-axis orientation of ultrathin SnS2 nanoplates on graphene as high-performance anode for Li-ion batteries. ACS Appl Mater Inter 5: 1588–1595. doi: 10.1021/am302124f
![]() |
[194] |
Zhang Q, Li R, Zhang M, et al. (2014) SnS2/reduced graphene oxide nanocomposites with superior lithium storage performance. Electrochim Acta 115: 425–433. doi: 10.1016/j.electacta.2013.10.193
![]() |
[195] |
Mei L, Xu C, Yang T, et al. (2013) Superior electrochemical performance of ultra-small SnS2 nanocrystals decorated on flexible RGO in lithium-ion batteries. J Mater Chem A 1: 8658–8664. doi: 10.1039/c3ta11269a
![]() |
[196] |
Luo B, Fang Y, Wang B, et al. (2012) Two dimensional grapheme-SnS2 hybrids with superior rate capability for lithium ion storage. Energy Environ Sci 5: 5226–5230. doi: 10.1039/C1EE02800F
![]() |
[197] |
Qin J, He C, Zhao N, et al. (2014) Graphene networks anchored with Sn@graphene as lithium ion battery anode. ACS Nano 8: 1728–1738. doi: 10.1021/nn406105n
![]() |
[198] |
Jiang X, Yang X, Zhu Y, et al. (2013) In situ assembly of graphene sheets-supported SnS2 nanoplates into 3D macroporous aerogels for high-performance lithium ion batteries. J Power Sources 237: 178–186. doi: 10.1016/j.jpowsour.2013.03.048
![]() |
[199] | Zhao Y, Li X, Yan B, et al. (2015) Significant impact of 2D graphene nanosheets on large volume change tin-based anodes in lithium-ion batteries: A review. J Power Sources 274: 869–884. |
[200] |
Lei WX, Pan Y, Zhou YC, et al. (2014) CNTs-Cu composite layer enhanced Sn-Cu alloy as high performance anode materials for lithium-ion batteries. RSC Adv 4: 3233–3237. doi: 10.1039/C3RA44431G
![]() |
[201] | Chen SY, Wang ZX, Fang XP, et al. (2011) characterization of TiS2 as an anode material for lithium ion batteries. Acta Phys Chim Sin 27: 97–102. |
[202] |
Kartick B, Srivastava SK, Mahanty S (2013) TiS2-MWCNT hybrid as highperformance anode in lithium-ion battery. J Nanopart Res 15: 1950–1962. doi: 10.1007/s11051-013-1950-5
![]() |
[203] |
Deng D (2015) Li-ion batteries: basics, progress, and challenges. Energy Sci Eng 3: 385–418. doi: 10.1002/ese3.95
![]() |
[204] |
Rui X, Tan H, Yan Q (2014) Nanostructured metal sulfides for energy storage. Nanoscale 6: 9889–9924. doi: 10.1039/C4NR03057E
![]() |
[205] |
Son MY, Choi JH, Kang YC (2014) Electrochemical properties of bare nickel sulfide and nickel sulfide-carbon composites prepared by one-pot spray pyrolysis as anode materials for lithium secondary batteries. J Power Sources 251: 480–487. doi: 10.1016/j.jpowsour.2013.10.093
![]() |
[206] |
Aso K, Sakuda A, Hayashi A, et al. (2013) All-solid-state lithium secondary batteries using NiS-carbon fiber composite electrodes coated with Li2S-P2S5 solid electrolytes by pulsed laser deposition. ACS Appl Mater Inter 5: 686–690. doi: 10.1021/am302164e
![]() |
[207] |
Aso K, Hayashi A, Tatsusago M (2012) Synthesis of NiS-carbon fiber composites in high-boiling solvent to improve electrochemical performance in all-solid-state lithium secondary batteries. Electrochim Acta 83: 448–453. doi: 10.1016/j.electacta.2012.07.088
![]() |
[208] | Sebastian ST, Jagan RS, Rajagoplan R, et al. (2014) Lithium-ion storage performance of camphoric carbon wrapped NiS nano/micro-hybrids RSC Adv 4: 11673–11679. |
[209] |
Geng H, Kong SF, Wang Y (2014) NiS nanorod-assembled nanoflowers grown on graphene: morphology evolution and Li-ion storage applications. J Mater Chem A 2: 15152–15158. doi: 10.1039/C4TA03440F
![]() |
[210] |
Pan Q, Xie J, Liu S, et al. (2013) Facile one-pot synthesis of ultrathin NiS nanosheets anchored on graphene and the improved electrochemical Li-storage properties. RSC Adv 3: 3899–3906. doi: 10.1039/c2ra22410k
![]() |
[211] | Zhou W, Zheng JL, Yue YH, et al. (2015) Highly stable rGO-wrapped Ni3S2 nanobowls: Structure fabrication and superior long-life electrochemical performance in LIBs. Nano Energy 11: 428-435. |
[212] | Gao Z, Song N, Zhang Y, et al. (2015) Cotton-textile-enabled, flexible lithium-ion batteries with enhanced capacity and extended lifespan. Nano Lett 15: 8194-8203. |
[213] |
Chen Q, Chen W, Ye JB, et al. (2015) L-Cysteine-assisted hydrothermal synthesis of nickel disulfide/graphene composite with enhanced electrochemical performance for reversible lithium storage. J Power Sources 294: 51–58. doi: 10.1016/j.jpowsour.2015.06.071
![]() |
[214] | Mahmoud N, Zhang C, Hou Y (2013) Nickel sul?de/nitrogen-doped graphene composites: phase-controlled synthesis and high performance a increased the grafting of node materials for lithium ion batteries. Small 9: 1321–1328. |
[215] |
Reddy ALM, Srivastava A, Gowda SR, et al. (2010) Synthesis of nitrogen-doped graphene films for lithium battery application. ACS Nano 4: 6337–6342. doi: 10.1021/nn101926g
![]() |
[216] | Zhou Y, Yan D, Xu H, et al. (2015) Hollow nanospheres of mesoporous Co9S8 as a high-capacity and long-life anode for advanced lithium ion batteries. Nano Energy 12: 528-537. |
[217] | Shi W, Zhu J, Rui X, et al. (2012) Controlled synthesis of carbon-coated cobalt sulfide nanostructures in oil phase with Enhanced Li Storage Performances. ACS Appl Mater Inter 4: 2999-3006. |
[218] |
Gu Y, Wang Y (2013) Graphene-Wrapped CoS Nanoparticles for high-capacity lithium-ion storage. ACS Appl Mater Inter 5: 801–806. doi: 10.1021/am3023652
![]() |
[219] |
Zhang D, Mai YJ, Xiang JY, et al. (2012) FeS2/C composite as an anode for lithium ion batteries with enhanced reversible capacity. J Power Sources 217: 229–235. doi: 10.1016/j.jpowsour.2012.05.112
![]() |
[220] |
Gan Y, Xu F, Luo J, et al. (2016) One-pot biotemplate synthesis of FeS decorated sulfur-doped carbon fiber as high capacity anode for lithium-ion batteries. Electrochim Acta 209: 201–209. doi: 10.1016/j.electacta.2016.05.076
![]() |
[221] |
Wen X, Wei X, Yang L, et al. (2015) Self-assembled FeS2 cubes anchored on reduced graphene oxide as an anode material for lithium ion batteries. J Mater Chem A 3: 2090–2096. doi: 10.1039/C4TA05575F
![]() |
[222] |
Zhang SS, Tran DT (2015) Electrochemical verification of the redox mechanism of FeS2 in a rechargeable lithium battery. Electrochim Acta 176: 784–789. doi: 10.1016/j.electacta.2015.07.096
![]() |
[223] |
Cao F, Pan GX, Chen J, et al. (2016) Synthesis of pyrite/carbon shells on cobalt nanowires forming core/branch arrays as high-performance cathode for lithium ion batteries. J Power Sources 303: 35–40. doi: 10.1016/j.jpowsour.2015.10.096
![]() |
[224] |
Cui H, Zhu G, Liu X, et al. (2015) Niobium nitride Nb4N5 as a new high-performance electrode material for supercapacitors. Adv Sci 2: 1500126. doi: 10.1002/advs.201500126
![]() |
[225] |
Fu ZW, Wang Y, Yue XL, et al. (2004) Electrochemical reactions of lithium with transition metal nitride electrodes. J Phys Chem B 108: 2236–2244. doi: 10.1021/jp030530s
![]() |
[226] |
Balogun MS, Qiu W, Wang W, et al. (2015) Recent advances in metal nitrides as high-performance electrode materials for energy storage devices. J Mater Chem A 3: 1364–1387. doi: 10.1039/C4TA05565A
![]() |
[227] |
Miller TS, Jorge AB, Sella A, et al. (2015) The use of graphitic carbon nitride based composite anodes for lithium-ion battery applications. Electroanal 27: 2614–2619. doi: 10.1002/elan.201500205
![]() |
[228] |
Tan G, Wu F, Yuan Y, et al. (2016) Free standing three-dimensional core-shell nanoarrays for lithium-ion battery anodes. Nature Commun 7: 11774. doi: 10.1038/ncomms11774
![]() |
[229] | Wang Y, Ding X, Huang L, et al. (2015) Critical influence of carbon nitride self-assembly coating on the electrochemical performance of SnO2-TiO2 nanocomposite anode material for lithium ion battery. Int J Electrochem Sci 11: 2461–2472. |
[230] | Ulvestad A, Maehlen JP, Kirkengen M (2015) Silicon nitride coated silicon thin films as anodes for Li-ion batteries. ECS Trans 64: 107–111. |
[231] | Wang R, Lang J, Zghang P, et al. (2015) Fast and large lithium storage in 3D porous VN nanowires-graphene composite as a superior anode toward high-performance hybrid supercapacitors. Adv Func Mater 25: 2270–2278. |
[232] |
Zhang K, Wang H, He X, et al. (2011) A hybrid material of vanadium nitride and nitrogen-doped graphene for lithium storage. J Mater Chem 21: 11916–11922. doi: 10.1039/c1jm11710f
![]() |
[233] |
Lei D, Yang T, Qu B, et al. (2014) Synthesis of TiN@C nanocomposites for enhanced electrochemical properties. Sustain Ener 2: 1–4. doi: 10.7569/JSEE.2014.629502
![]() |
[234] |
Zheng H, Fang S, Tong Z, et al. (2015) Stabilized titanium nitride nanowire supported silicon core-shell nanorods as high capacity lithium-ion anodes. J Mater Chem A 3: 12476–12481. doi: 10.1039/C5TA02259B
![]() |
[235] |
Zhang J, Zhang J, Cai W, et al. (2012) Improving electrochemical properties of spinel lithium titanate by incorporation of titanium nitride via high-energy ball-milling. J Power Sources 211: 133–139. doi: 10.1016/j.jpowsour.2012.03.088
![]() |
[236] |
Yue Y, Han P, He X, et al. (2012) In situ synthesis of a graphene/titanium nitride hybrid material with highly improved performance for lithium storage. J Mater Chem 22: 4938–4943. doi: 10.1039/c2jm16128a
![]() |
[237] |
Liu M, Zhang L, Han P, et al. (2015) Controllable formation of niobium nitride/nitrogen-doped graphene nanocomposites as anode materials for lithium-ion capacitors. Part Part Syst Charact 32: 1006–1011. doi: 10.1002/ppsc.201500095
![]() |
[238] |
Li Y, Yan Y, Ming H, et al. (2014) One-step synthesis Fe3N surface-modified Fe3O4 nanoparticles with excellent lithium storage ability. Appl Surf Sci 305: 683–688. doi: 10.1016/j.apsusc.2014.03.169
![]() |
[239] |
Liu J, Tang S, Lu Y, et al. (2013) Synthesis of MoN nanolayer coated MoO2 hollow nanostructures as high-performance anode materials for lithium-ion batteries. Energy Environ Sci 6: 2691–2697. doi: 10.1039/c3ee41006d
![]() |
[240] |
Sun Q, Fu ZW (2012) Mn3N2 as a novel negative electrode material for rechargeable lithium batteries. Appl Surf Sci 258: 3197–3201. doi: 10.1016/j.apsusc.2011.11.063
![]() |
[241] |
Wu Y, Liu M, Feng H, et al. (2014) Carbon coated MnO@Mn3N2 core-shell composites for high performance lithium ion battery anodes. Nanoscale 6: 14697–14701. doi: 10.1039/C4NR05043F
![]() |
[242] |
Zhang L, Hu P, Zhao X, et al. (2011) Controllable synthesis of core-shell Co-CoO nanocomposites with a superior performance as an anode material for lithium-ion batteries. J Mater Chem 21: 18279–18283. doi: 10.1039/c1jm12990b
![]() |
[243] | Xiong S, Chen JS, Lou XW, et al. (2012) Mesoporous Co3O4 and CoO-C topotactically transformed from chrysanthemum-like Co(CO3)0.5(OH)·0.11H2O and their lithium-storage properties. Adv Funct Mater 22: 861–871. |
[244] |
Qi Y, Du N, Zhang H, et al. (2012) CoO/NiSix core-shell nanowire arrays as lithium-ion anodes with high rate capabilities. Nanoscale 4: 991–996. doi: 10.1039/C2NR11545J
![]() |
[245] |
Qi Y, Du N, Zhang H, et al. (2012) Nanostructured hybrid cobalt oxide/copper electrodes of lithium-ion batteries with reversible high-rate capabilities. J Alloy Compd 521: 83–89. doi: 10.1016/j.jallcom.2012.01.046
![]() |
[246] |
Zhao W, Du N, Zhang H, et al. (2016) A novel Co-Li2O@Si core-shell nanowire array composite as a high-performance lithium-ion battery anode material. Nanoscale 8: 4511-4519. doi: 10.1039/C5NR06120B
![]() |
[247] |
Nethravathi C, Rajamathi CR, Rajamathi M, et al. (2013) N-doped graphene-VO2(B) nanosheet-built 3D flower hybrid for lithium ion battery. ACS Appl Mater Inter 5: 2708–2714. doi: 10.1021/am400202v
![]() |
[248] |
Zhang L, Wang Z, Yang K, et al. (2016) Preparation and electrochemical performances of CoO/3D graphene composite as anode for lithium-ion batteries. J Alloy Compd 656: 278–283. doi: 10.1016/j.jallcom.2015.09.208
![]() |
[249] |
Qi Y, Zhang H, Du N, et al. (2013) Highly loaded CoO/graphene nanocomposites as lithium-ion anodes with superior reversible capacity. J Mat Chem A 1: 2337–2342. doi: 10.1039/c2ta00929c
![]() |
[250] |
Zhu J, Zhu T, Zhou X, et al. (2011) Facile synthesis of metal oxide/reduced graphene oxide hybrids with high lithium storage capacity and stable cyclability. Nanoscale 3: 1084–1089. doi: 10.1039/C0NR00744G
![]() |
[251] |
Peng C, Chen B, Qin Y, et al. (2012) Facile ultrasonic synthesis of CoO quantum dot/graphene nanosheet composites with high lithium storage capacity. ACS Nano 6: 1074–1081. doi: 10.1021/nn202888d
![]() |
[252] |
Sun Y, Hu X, Luo W, et al. (2012) Ultrathin CoO/graphene hybrid nanosheets: a highly stable anode material for lithium-ion batteries. J Phys Chem C 116: 20794–20799. doi: 10.1021/jp3070147
![]() |
[253] |
Verrelli R, Hassoun J (2015) High-capacity NiO-(mesocarbon microbeads) conversion anode for lithium-ion battery. ChemElectroChem 2: 988–994. doi: 10.1002/celc.201500069
![]() |
[254] | Sun X, Yan C, Chen Y, et al. (2014) Three-dimensionally curved NiO nanomembranes as ultrahigh rate capability anodes for Li-ion batteries with long cycle lifetimes. Adv Energy Mater 4. |
[255] |
Chen X, Zhang N, Sun K (2012) Facile ammonia-induced fabrication of nanoporous NiO films with enhanced lithium-storage properties. Electrochem Commun 20: 137–140. doi: 10.1016/j.elecom.2012.04.009
![]() |
[256] |
Huang XH, Yuan YF, Wang Z, et al. (2011) Electrochemical properties of NiO/Co-P nanocomposite as anode materials for lithium ion batteries. J Alloy Compd 509: 3425–3429. doi: 10.1016/j.jallcom.2010.12.117
![]() |
[257] |
Zou Y, Wang Y (2011) NiO nanosheets grown on graphene nanosheets as superior anode materials for Li-ion batteries. Nanoscale 3: 2615–2620. doi: 10.1039/c1nr10070j
![]() |
[258] |
Huang Y, Huang XL, Lian JS, et al. (2012) Self-assembly of ultrathin porous NiO nanosheets/graphene hierarchical structure for high-capacity and high-rate lithium storage. J Mater Chem 22: 2844–2847. doi: 10.1039/c2jm15865e
![]() |
[259] |
Zhu XJ, Hu J, Dai HL, et al. (2012) Reduced graphene oxide and nanosheet-based nickel oxide microsphere composite as an anode material for lithium ion battery. Electrochim Acta 64: 23–28. doi: 10.1016/j.electacta.2011.12.040
![]() |
[260] |
Choi SH, Ko YN, Lee JK, et al. (2014) Rapid continuous synthesis of spherical reduced graphene ball-nickel oxide composite for lithium ion batteries. Sci Rep 4: 5786. doi: 10.1038/srep05786
![]() |
[261] | Zhou F, Chen Y, Liu K, et al. (2016) Metal organic frameworks derived hierarchical hollow NiO/Ni/graphene composites for lithium and sodium storage. ACS Nano 10: 377-386. |
[262] |
Zhong KF, Xia X, Zhang B, et al. (2010) MnO powder as anode active materials for lithium ion batteries. J Power Sources 195: 3300–3308. doi: 10.1016/j.jpowsour.2009.11.133
![]() |
[263] |
Li SR, Sun Y, Ge SY, et al. (2012) A facile route to synthesize nano-MnO/C composites and their application in lithium ion batteries. Chem Eng J 192: 226–231. doi: 10.1016/j.cej.2012.04.009
![]() |
[264] |
Zhong K, Zhang B, Luo S, et al. (2011) Investigation on porous MnO microsphere anode for lithium ion batteries. J Power Sources 196: 6802–6808. doi: 10.1016/j.jpowsour.2010.10.031
![]() |
[265] |
Tang X, Sui G, Cai Q, et al. (2016) Novel MnO/carbon composite anode material with multi-modal pore structure for high performance lithium-ion batteries. J Mater Chem A 4: 2082–2088. doi: 10.1039/C5TA10073A
![]() |
[266] |
Xia Y, Xiao Z, Dou X, et al. (2013) Green and facile fabrication of hollow porous MnO/C microspheres from microalgaes for lithium-ion batteries. ACS Nano 7: 7083–7092. doi: 10.1021/nn4023894
![]() |
[267] |
Yue J, Gu X, Wang N, et al. (2014) General synthesis of hollow MnO2, Mn3O4 and MnO nanospheres as superior anode materials for lithium ion batteries. J Mater Chem A 2: 17421–17426. doi: 10.1039/C4TA03924F
![]() |
[268] | Liu B, Hu X, Xu H, et al. (2014) Encapsulation of MnO nanocrystals in electrospun carbon nanofibers as high-performance anode materials for lithium-ion batteries. Sci Rep 4: 4229. |
[269] |
Sun Y, Hu X, Luo W, et al. (2013) Reconstruction of conformal nanoscale MnO on graphene as a high-capacity and long-life anode material for lithium ion batteries. Adv Func Mater 23: 2436–2443. doi: 10.1002/adfm.201202623
![]() |
[270] |
Jiang H, Hu YJ, Guo SJ, et al. (2014) Rational design of MnO/carbon nanopeapods with internal void space for high-rate and long-life Li-ion batteries. ACS Nano 8: 6038–6046. doi: 10.1021/nn501310n
![]() |
[271] |
Wang Y, Han ZJ, Yu SF, et al. (2013) Core-leaf onion-like carbon/MnO2 hybrid nano-urchins for rechargeable lithium-ion batteries. Carbon 64: 230–236. doi: 10.1016/j.carbon.2013.07.057
![]() |
[272] |
Lai H, Li JX, Chen ZG, et al. (2012). Carbon nanohorns as a high-performance carrier for MnO2 anode in lithium-ion batteries. ACS Appl Mater Inter 4: 2325–2328. doi: 10.1021/am300378w
![]() |
[273] |
Xia H, Lai M, Lu L (2010) Nanoflaky MnO2/carbon nanotube nanocomposites as anode materials for lithium-ion batteries. J Mater Chem 20: 6896–6902. doi: 10.1039/c0jm00759e
![]() |
[274] |
Yun YS, Kim JM, Park HH, et al. (2013) Free-standing heterogeneous hybrid papers based on mesoporous γ-MnO2 particles and carbon nanotubes for lithium-ion battery anodes. J Power Sources 244: 747–751. doi: 10.1016/j.jpowsour.2012.11.056
![]() |
[275] |
Yu AP, Park HW, Davies A, et al. (2011) Free-standing layer-by-layer hybrid thin film of graphene-MnO2 nanotube as anode for lithium ion batteries. J Phys Chem Lett 2: 1855–1860. doi: 10.1021/jz200836h
![]() |
[276] |
Li L, Raji ARO, Tour JM (2013) Graphene-wrapped MnO2-graphene nanoribbons as anode materials for high-performance lithium ion batteries. Adv Mater 25: 6298–6302. doi: 10.1002/adma.201302915
![]() |
[277] |
Li YY, Zhang QW, Zhu JL, et al. (2014) An extremely stable MnO2 anode incorporated with 3D porous graphene-like networks for lithium-ion batteries. J Mater Chem A 2: 3163–3168. doi: 10.1039/c3ta14372d
![]() |
[278] |
Luo W, Hu X, Sun Y, et al. (2011) Electrospinning of carbon-coated Mo2 nanofibers with enhanced lithium-storage properties. Phys Chem Chem Phys 13: 16735–16740. doi: 10.1039/c1cp22184a
![]() |
[279] |
Liu B, Zhao X, Tian Y, et al. (2013) A simple reduction process to synthesize MoO2/C composites with cage-like structure for high-performance lithium-ion batteries. Phys Chem Chem Phys 15: 8831–8837. doi: 10.1039/c3cp44707c
![]() |
[280] | Gao Q, Yang L, Lu X, et al. (2010) Synthesis, characterization and lithium-storage performance of MoO2/carbon hybrid nanowires. J Mater Chem 20: 2807–2812. |
[281] |
Zeng L, Zheng C, Deng C, et al. (2013) MoO2-ordered mesoporous carbon nanocomposite as an anode material for lithium-ion batteries. ACS Appl Mater Inter 5: 2182–2187. doi: 10.1021/am303286n
![]() |
[282] |
Ihsan M, Wang H, Majid SR, et al. (2016) MoO2/Mo2C/C spheres as anode materials for lithium ion batteries. Carbon 96: 1200–1207. doi: 10.1016/j.carbon.2015.10.076
![]() |
[283] | Xu Y, Yi R, Yuan B, et al. (2012) High capacity MoO2/graphite oxide composite anode for lithium-ion batteries. J Phys Chem Lett 3: 309–314. |
[284] |
Sun Y, Hu X, Luo W, et al. (2011) Self-assembled hierarchical MoO2/graphene nanoarchitectures and their application as a high-performance anode material for lithium-ion batteries. ACS Nano 5: 7100–7107. doi: 10.1021/nn201802c
![]() |
[285] |
Tang Q, Shan Z, Wang L, et al. (2012) MoO2-graphene nanocomposite as anode material for lithium-ion batteries. Electrochim Acta 79: 148–153. doi: 10.1016/j.electacta.2012.06.093
![]() |
[286] |
Hou Y, Li JY, Wen ZH, et al. (2014) N-doped graphene/porous g-C3N4 nanosheets supported layered-MoS2 hybrid as robust anode materials for lithium-ion batteries. Nano Energy 8: 157–164. doi: 10.1016/j.nanoen.2014.06.003
![]() |
[287] |
Kumar V, Lee PS (2015) Redox active polyaniline-h-MoO3 hollow nanorods for improved pseudocapacitive performance. J Phys Chem C 119: 9041–9049. doi: 10.1021/acs.jpcc.5b00153
![]() |
[288] |
Brezesinski T, Wang J, Tolbert SH, et al. (2010) Ordered mesoporous α-MoO3 with iso-oriented nanocrystalline walls for thin-film pseudocapacitors. Nat Mater 9: 146–151. doi: 10.1038/nmat2612
![]() |
[289] |
Zhou K, Zhou W, Liu X, et al. (2015) Ultrathin MoO3 nanocrystals self-assembled on graphene nanosheets via oxygen bonding as supercapacitor electrodes of high capacitance and long cycle life. Nano Energy 12: 510–520. doi: 10.1016/j.nanoen.2015.01.017
![]() |
[290] | Han B, Lee KH, Lee YW, et al. (2015) MoO3 nanostructured electrodes prepared via hydrothermal process for lithium ion batteries. Int J Electrochem Sci 10: 4232–4240.. |
[291] | Wang Q, Sun J, Wang Q, et al. (2015) Electrochemical performance of α-MoO3-In2O3 core-shell nanorods as anode materials for lithium-ion batteries. J Mater Chem A 3: 5083–5091. |
[292] |
Hamed B, Shahid M, Nagae-raju DH, et al. (2015) Surface passivation of MoO3 nanorods by atomic layer deposition toward high rate durable Li ion battery anodes. ACS Appl Mater Inter 7: 13154–13163. doi: 10.1021/acsami.5b03395
![]() |
[293] | Zhao G, Li C, Zhang L, et al. (2015) “Sea cucumber”-like Ti@MoO3 nanorod arrays as self-supported lithium ion battery anodes with enhanced rate capability and durability. J Mater Chem A 3: 22547–22551. |
[294] |
Ma F, Yuan A, Xu J, et al. (2015) Porous α-MoO3/MWCNT nanocomposite synthesized via a surfactant-assisted solvothermal route as a lithium-ion-battery high-capacity anode material with excellent rate capability and cyclability. ACS Appl Mater Inter 7: 15531–15541. doi: 10.1021/acsami.5b03953
![]() |
[295] | Tauseef A, Li W, Li J, et al. (2016) Lithium storage study on MoO3-grafted TiO2 nanotube arrays. Appl Nanoscience [in press]. |
[296] |
Xue XY, Chen ZH, Xing LL, et al. (2011) SnO2/MoO3 core-shell nanobelts and their extraordinarily high reversible capacity as lithium-ion battery anodes. Chem Commun 47: 5205–5207. doi: 10.1039/c1cc00076d
![]() |
[297] | Hashem AM, Abbas SB, Abdel-Ghany AE, et al. (2016) Blend formed by oxygen deficient MoO3?δ oxides as lithium-insertion compounds. J Alloy Compd 686: 744–752. |
[298] |
Bruck A, Cama CA, Gannett CN, et al. (2016) Nanocrystalline iron oxide based electroactive materials in lithium ion batteries: the critical role of crystallite size, morphology, and electrode heterostructure on battery relevant electrochemistry. Inorg Chem Front 3: 26–40. doi: 10.1039/C5QI00247H
![]() |
[299] |
Zhang WM, Wu XL, Hu JS, et al. (2008) Carbon-coated Fe3O4 nanospindles as a superior anode material for lithium-ion batteries. Adv Funct Mater 18: 3941–3946. doi: 10.1002/adfm.200801386
![]() |
[300] |
Zhu T, Chen JS, Lou XW (2011) Glucose-assisted one-pot synthesis of FeOOH nanorods and their transformation to Fe3O4@carbon nanorods for application in lithium ion batteries. J Phys Chem C 115: 9814–9820. doi: 10.1021/jp2013754
![]() |
[301] |
Chen JS, Zhang YM, Lou XW (2011) One-pot synthesis of uniform Fe3O4 nanospheres. ACS Appl Mater Inter 3: 3276–3279. doi: 10.1021/am201079z
![]() |
[302] |
Wang ZY, Luan DY, Madhavi S, et al. (2012) Assembling carbon-coated γ-Fe2O3 hollow nanohorns on the CNT backbone for superior lithium storage capability. Energ Environ Sci 5: 5252–5256. doi: 10.1039/C1EE02831F
![]() |
[303] |
Ji L, Toprakci O, Alcoutlabi M, et al. (2012) α-Fe2O3 nanoparticle-loaded carbon nanofibers as stable and high-capacity anodes for rechargeable lithium-ion batteries. ACS Appl Mater Inter 4: 2672–2679. doi: 10.1021/am300333s
![]() |
[304] |
Sun Z, Madej E, Genç A, et al. (2016) Demonstrating the steady performance of iron oxide composites over 2000 cycles at fast charge-rates for Li-ion batteries. Chem Commun 52: 7348–7351. doi: 10.1039/C6CC00168H
![]() |
[305] |
Jang B, Park M, Chae OB, et al. (2012) Direct synthesis of self-assembled ferrite/carbon hybrid nanosheets for high performance lithium-ion battery anodes. J Am Chem Soc 134: 15010–15015. doi: 10.1021/ja305539r
![]() |
[306] |
He CN, Wu S, Zhao NQ, et al. (2013) Carbon-encapsulated Fe3O4 nanoparticles as a high-rate lithium-ion battery anode material. ACS Nano 7: 4459–4469. doi: 10.1021/nn401059h
![]() |
[307] |
Zhu X, Zhu Y, Murali S, et al. (2011) Nanostructured reduced graphene oxide/Fe2O3 composite as a high-performance anode material for lithium ion batteries. ACS Nano 5: 3333–3338. doi: 10.1021/nn200493r
![]() |
[308] |
Wang G, Liu T, Luo Y, et al. (2011) Preparation of Fe2O3/graphene composite and its electrochemical performance as an anode material for lithium ion batteries. J Alloy Compd 509: L216–L220. doi: 10.1016/j.jallcom.2011.03.151
![]() |
[309] | Shi M, Wu T, Song X, et al. (2016) Active Fe2O3 nanoparticles encapsulated in porous g-C3N4/graphene sandwich-type nanosheets as superior anode for high-performance lithium-ion batteries. J Mater Chem A [in press]. |
[310] |
Wang R, Xu C, Sun J, et al. (2013) Flexible free-standing hollow Fe3O4/graphene hybrid films for lithium-ion batteries. J Mater Chem A 1: 1794–1800. doi: 10.1039/C2TA00753C
![]() |
[311] |
Zhuo L, Wu Y, Wang L, et al. (2013) CO2-expanded ethanol chemical synthesis of a Fe3O4/graphene composite and its good electrochemical properties as anode material for lithium-ion batteries. J Mater Chem A 1: 3954–3960. doi: 10.1039/c3ta01388j
![]() |
[312] |
Pan L, Zhu XD, Xie XM, et al. (2015) Smart hybridization of anatase TiO2 nanorods and Fe3O4 nanoparticles with pristine graphene nanosheets: hierarchically nanoengineered ternary heterostructures for high-rate lithium storage. Adv Func Mater 25: 3341–3350. doi: 10.1002/adfm.201404348
![]() |
[313] |
Mo R, Lei Z, Sun K, et al. (2014) Facile synthesis of anatase TiO2 quantum-dot/graphene-nanosheet composites with enhanced electrochemical performance for lithium-ion batteries. Adv Mater 26: 2084–2088. doi: 10.1002/adma.201304338
![]() |
[314] |
Ding S, Chen JS, Luan D, et al. (2011) Graphene-supported anatase TiO2 nanosheets for fast lithium storage. Chem Commun 47: 5780–5782. doi: 10.1039/c1cc10687b
![]() |
[315] |
Qiu B, Xing M, Zhang J (2014) Mesoporous TiO2 nanocrystals grown in situ on graphene aerogels for high photocatalysis and lithium-ion batteries. J Am Chem Soc 136: 5852–5855. doi: 10.1021/ja500873u
![]() |
[316] |
Li W, Wang F, Feng S, et al. (2013) Sol-gel design strategy for ultradispersed TiO2 nanoparticles on graphene for high-performance lithium ion batteries. J Am Chem Soc 135: 18300–18303. doi: 10.1021/ja4100723
![]() |
[317] |
Gao L, Hu H, Li G, et al. (2014) Hierarchical 3D TiO2@Fe2O3 nano-framework arrays as high-performance anode materials. Nanoscale 6: 6463–6467. doi: 10.1039/c4nr00387j
![]() |
[318] | Yan C, Chen G, Sun J, et al. (2016) Novel anode of C&N co-doped Co3O4 hollow nanofibres with excellent performance for lithium-ion batteries. Phys Chem Chem Phys [in press]. |
[319] | Yang H, Wang Y, Nie Y, et al. (2016) Co3O4/porous carbon nanofibers composite as anode for high-performance lithium ion batteries with improved cycle performance and lithium storage capacity. J Compos Mater [in press]. |
[320] |
Zhu X, Ning G, Ma X, et al. (2013) High density Co3O4 nanoparticles confined in a porous graphene nanomesh network driven by an electrochemical process: ultra-high capacity and rate performance for lithium ion batteries. J Mater Chem A 1: 14023–14030. doi: 10.1039/c3ta12824e
![]() |
[321] |
Li BJ, Cao HQ, Shao J, et al. (2011) Co3O4@graphene composites as anode materials for high-performance lithium ion batteries. Inorg Chem 50: 1628–1632. doi: 10.1021/ic1023086
![]() |
[322] |
Kim H, Seo DH, Kim SW, et al. (2011) Highly reversible Co3O4/graphene hybrid anode for lithium rechargeable batteries. Carbon 49: 326–332. doi: 10.1016/j.carbon.2010.09.033
![]() |
[323] |
Sun H, Liu Y, Yu Y, et al. (2014) Mesoporous Co3O4 nanosheets-3D graphene networks hybrid materials for high-performance lithium ion batteries. Electrochim Acta 118: 1–9. doi: 10.1016/j.electacta.2013.11.181
![]() |
[324] |
Li P, Cui M, Zhang M, et al. (2016) Facile fabrication of Co3O4/nitrogen-doped graphene hybrid materials as high performance anode materials for lithium ion batteries. CrystEngComm 18: 3383–3388. doi: 10.1039/C6CE00462H
![]() |
[325] | Dou Y, Xu J, Ruan B, et al. (2016) Atomic layer-by-layer Co3O4/graphene composite for high performance lithium-ion batteries. Adv Energy Mater 6. |
[326] | Wang HG, Ma DL, Huang Y, et al. (2012) General and controllable synthesis strategy of metal oxide/TiO2 hierarchical heterostructures with improved lithium-ion battery performance. Sci Rep 2: 136–136. |
[327] | Weng BC, Xu FH, Lozano K (2014) Mass production of carbon nanotube-reinforced polyacrylonitrile fine composite fibers. J Appl Polym Sci 131: 2928–2935. |
1. | Arijit Roy, Somnath Bhattacharjee, Soumyajit Podder, Advaita Ghosh, Measurement of bioimpedance and application of Cole model to study the effect of moisturizing cream on human skin, 2020, 7, 2377-9098, 362, 10.3934/biophy.2020025 | |
2. | Amalia C. Nur’aidha, Dhananjaya Y.H Kumarajati, Experimental Study of Electrode Design and Configuration for Bioimpedance Measurement, 2021, 1823, 1742-6588, 012009, 10.1088/1742-6596/1823/1/012009 | |
3. | Rhea Patel, Madhuri Vinchurkar, Rajul Patkar, Gopal Pranjale, Maryam Shojaei Baghini, 2021, Impedance Based Biosensor for Agricultural Pathogen Detection, 978-1-6654-4156-8, 385, 10.1109/NANO51122.2021.9514277 | |
4. | Valerii Kryvonosov, Nataliia Prudnikova, Lilia Martyniuk, Justification of the electrical scheme of biological tissue replacementunder the action of DC voltage, 2022, 13, 26631342, 10.31548/machenergy.13(4).2022.60-71 | |
5. | Jirat Chimnoy, Khunanon Karawanich, Pipat Prommee, 2024, Low-Cost Technique for Extracting the Cole-Cole Model Parameters, 979-8-3503-8155-9, 1, 10.1109/ECTI-CON60892.2024.10594987 | |
6. | Patricia Vazquez-Guerrero, Rohisha Tuladhar, Costas Psychalinos, Ahmed Elwakil, Maurice J. Chacron, Fidel Santamaria, Fractional order memcapacitive neuromorphic elements reproduce and predict neuronal function, 2024, 14, 2045-2322, 10.1038/s41598-024-55784-1 | |
7. | Md. Tushar Abdullah, Chitra Roy, Md. Ibrahim Al Imran, 2024, Exploring Age-Related Changes in Bioimpedance across Diverse Biological Samples, 979-8-3503-5549-9, 1, 10.1109/ICISET62123.2024.10939557 |
Sample | Species/Description | Designation of Measurement |
Apple-1 | Apple-1 and Apple-2 are of same species (Green Granny Smith) and both are purchased from same local shop. Apple-1 and Apple-2 can be considered to be biologically identical. | A11, A12, A13, A14, A15 |
Apple-2 | A21, A22, A23, A24, A25 | |
Apple-3 | Apple-3 (Red Washington) is of different species that of Apple-1 and Apple-2. Apple-3 is biologically different from Apple-1 and Apple-2. | A31, A32, A33, A34, A35 |
Potato-1 | Potato-1 and Potato-2 are of same species (Kufri Chandramukhi) and both are purchased from same local shop. Potato-1 and Potato-2 can be considered to be biologically identical. | P11, P12, P13, P14, P15 |
Potato-2 | P21, P22, P23, P24, P25 | |
Potato-3 | Potato-3 (Kufri Jyoti) is of different species that of Potato-1 and Potato-2. Potato-3 is biologically different from Potato-1 and Potato-2. | P31, P32, P33, P34, P35 |
Remarks: In this table, Amn (or Pmn) represents impedance measurement of Apple-m (or Potato-m) at position n.
Sample | R0 (kΩ) |
R∞ (kΩ) |
α | C (nF secα−1) |
τ (µsec) |
Regression coefficient |
12.93 | 0.64 | 0.719 | 76.65 | 63 | 0.9989 | |
14.21 | 0.74 | 0.703 | 88.30 | 70 | 0.9987 | |
Apple-1 | 13.58 | 0.77 | 0.691 | 99.99 | 65 | 0.9983 |
13.75 | 0.65 | 0.705 | 91.55 | 72 | 0.9989 | |
12.12 | 0.63 | 0.692 | 103.83 | 60 | 0.9983 | |
12.83 | 0.61 | 0.694 | 98.25 | 63 | 0.9980 | |
13.67 | 0.70 | 0.708 | 86.55 | 69 | 0.9985 | |
Apple-2 | 13.90 | 0.72 | 0.696 | 103.47 | 76 | 0.9985 |
12.47 | 0.67 | 0.693 | 112.05 | 70 | 0.9983 | |
10.97 | 0.70 | 0.694 | 113.46 | 59 | 0.9984 | |
11.85 | 0.83 | 0.745 | 62.35 | 57 | 0.9993 | |
10.53 | 0.77 | 0.747 | 64.20 | 52 | 0.9991 | |
Apple-3 | 11.35 | 0.84 | 0.754 | 55.15 | 51 | 0.9994 |
13.75 | 0.84 | 0.767 | 47.47 | 65 | 0.9997 | |
11.74 | 0.84 | 0.751 | 56.65 | 53 | 0.9993 |
Remarks: Apple-1&Apple-2 are of same species and are taken from same lot. While Apple-3 is different from the other two.
Sample | Factor | P-value | F-value | Fcritical | Distinguishability | Level of Confidence |
Apple-1 & Apple-2 | Position | 0.119 | 3.627 | 15.977 | No | 99% |
Sample | 0.594 | 0.334 | 21.197 | No | ||
Apple-1 & Apple-3 | Position | 0.146 | 3.153 | 10.899 | No | 98% |
Sample | 0.017 | 15.384 | 14.039 | Yes |
Sample | R0 (kΩ) |
R∞ (kΩ) |
α | C (nF secα−1) |
τ (µsec) | Regression coefficient |
5.17 | 0.14 | 0.6400 | 341 | 48 | 0.9888 | |
5.48 | 0.16 | 0.7000 | 204 | 41 | 0.9956 | |
Potato-1 | 5.58 | 0.13 | 0.6638 | 246 | 47 | 0.9920 |
5.65 | 0.12 | 0.6505 | 325 | 60 | 0.9922 | |
5.09 | 0.11 | 0.6797 | 243 | 51 | 0.9951 | |
5.14 | 0.12 | 0.6275 | 445 | 60 | 0.9897 | |
5.43 | 0.13 | 0.6532 | 286 | 48 | 0.9918 | |
Potato-2 | 6.66 | 0.16 | 0.6077 | 450 | 68 | 0.9890 |
4.70 | 0.12 | 0.6415 | 363 | 47 | 0.9891 | |
5.67 | 0.12 | 0.5979 | 580 | 68 | 0.9865 | |
5.52 | 0.12 | 0.6360 | 420 | 70 | 0.9934 | |
5.57 | 0.15 | 0.6099 | 610 | 68 | 0.9906 | |
Potato-3 | 6.70 | 0.14 | 0.6202 | 432 | 78 | 0.9912 |
5.86 | 0.11 | 0.6362 | 393 | 69 | 0.9916 | |
5.46 | 0.13 | 0.6436 | 349 | 57 | 0.9924 |
Remarks: Potato -1 & Potato -2 are of same species and are taken from same lot, while Potato -3 is different from the other two.
Sample | Factor | P-value | F-value | Fcritical | Distinguishability | Level of Confidence |
Potato-1 & Potato-2 | Position | 0.6046 | 0.7537 | 15.977 | No | 99% |
Sample | 0.2124 | 2.1975 | 21.198 | No | ||
Potato-1& Potato-3 | Position | 0.6201 | 0.7218 | 10.899 | No | 98% |
Sample | 0.0182 | 14.856 | 14.040 | Yes |
Sample | Species/Description | Designation of Measurement |
Apple-1 | Apple-1 and Apple-2 are of same species (Green Granny Smith) and both are purchased from same local shop. Apple-1 and Apple-2 can be considered to be biologically identical. | A11, A12, A13, A14, A15 |
Apple-2 | A21, A22, A23, A24, A25 | |
Apple-3 | Apple-3 (Red Washington) is of different species that of Apple-1 and Apple-2. Apple-3 is biologically different from Apple-1 and Apple-2. | A31, A32, A33, A34, A35 |
Potato-1 | Potato-1 and Potato-2 are of same species (Kufri Chandramukhi) and both are purchased from same local shop. Potato-1 and Potato-2 can be considered to be biologically identical. | P11, P12, P13, P14, P15 |
Potato-2 | P21, P22, P23, P24, P25 | |
Potato-3 | Potato-3 (Kufri Jyoti) is of different species that of Potato-1 and Potato-2. Potato-3 is biologically different from Potato-1 and Potato-2. | P31, P32, P33, P34, P35 |
Sample | R0 (kΩ) |
R∞ (kΩ) |
α | C (nF secα−1) |
τ (µsec) |
Regression coefficient |
12.93 | 0.64 | 0.719 | 76.65 | 63 | 0.9989 | |
14.21 | 0.74 | 0.703 | 88.30 | 70 | 0.9987 | |
Apple-1 | 13.58 | 0.77 | 0.691 | 99.99 | 65 | 0.9983 |
13.75 | 0.65 | 0.705 | 91.55 | 72 | 0.9989 | |
12.12 | 0.63 | 0.692 | 103.83 | 60 | 0.9983 | |
12.83 | 0.61 | 0.694 | 98.25 | 63 | 0.9980 | |
13.67 | 0.70 | 0.708 | 86.55 | 69 | 0.9985 | |
Apple-2 | 13.90 | 0.72 | 0.696 | 103.47 | 76 | 0.9985 |
12.47 | 0.67 | 0.693 | 112.05 | 70 | 0.9983 | |
10.97 | 0.70 | 0.694 | 113.46 | 59 | 0.9984 | |
11.85 | 0.83 | 0.745 | 62.35 | 57 | 0.9993 | |
10.53 | 0.77 | 0.747 | 64.20 | 52 | 0.9991 | |
Apple-3 | 11.35 | 0.84 | 0.754 | 55.15 | 51 | 0.9994 |
13.75 | 0.84 | 0.767 | 47.47 | 65 | 0.9997 | |
11.74 | 0.84 | 0.751 | 56.65 | 53 | 0.9993 |
Sample | Factor | P-value | F-value | Fcritical | Distinguishability | Level of Confidence |
Apple-1 & Apple-2 | Position | 0.119 | 3.627 | 15.977 | No | 99% |
Sample | 0.594 | 0.334 | 21.197 | No | ||
Apple-1 & Apple-3 | Position | 0.146 | 3.153 | 10.899 | No | 98% |
Sample | 0.017 | 15.384 | 14.039 | Yes |
Sample | R0 (kΩ) |
R∞ (kΩ) |
α | C (nF secα−1) |
τ (µsec) | Regression coefficient |
5.17 | 0.14 | 0.6400 | 341 | 48 | 0.9888 | |
5.48 | 0.16 | 0.7000 | 204 | 41 | 0.9956 | |
Potato-1 | 5.58 | 0.13 | 0.6638 | 246 | 47 | 0.9920 |
5.65 | 0.12 | 0.6505 | 325 | 60 | 0.9922 | |
5.09 | 0.11 | 0.6797 | 243 | 51 | 0.9951 | |
5.14 | 0.12 | 0.6275 | 445 | 60 | 0.9897 | |
5.43 | 0.13 | 0.6532 | 286 | 48 | 0.9918 | |
Potato-2 | 6.66 | 0.16 | 0.6077 | 450 | 68 | 0.9890 |
4.70 | 0.12 | 0.6415 | 363 | 47 | 0.9891 | |
5.67 | 0.12 | 0.5979 | 580 | 68 | 0.9865 | |
5.52 | 0.12 | 0.6360 | 420 | 70 | 0.9934 | |
5.57 | 0.15 | 0.6099 | 610 | 68 | 0.9906 | |
Potato-3 | 6.70 | 0.14 | 0.6202 | 432 | 78 | 0.9912 |
5.86 | 0.11 | 0.6362 | 393 | 69 | 0.9916 | |
5.46 | 0.13 | 0.6436 | 349 | 57 | 0.9924 |
Sample | Factor | P-value | F-value | Fcritical | Distinguishability | Level of Confidence |
Potato-1 & Potato-2 | Position | 0.6046 | 0.7537 | 15.977 | No | 99% |
Sample | 0.2124 | 2.1975 | 21.198 | No | ||
Potato-1& Potato-3 | Position | 0.6201 | 0.7218 | 10.899 | No | 98% |
Sample | 0.0182 | 14.856 | 14.040 | Yes |