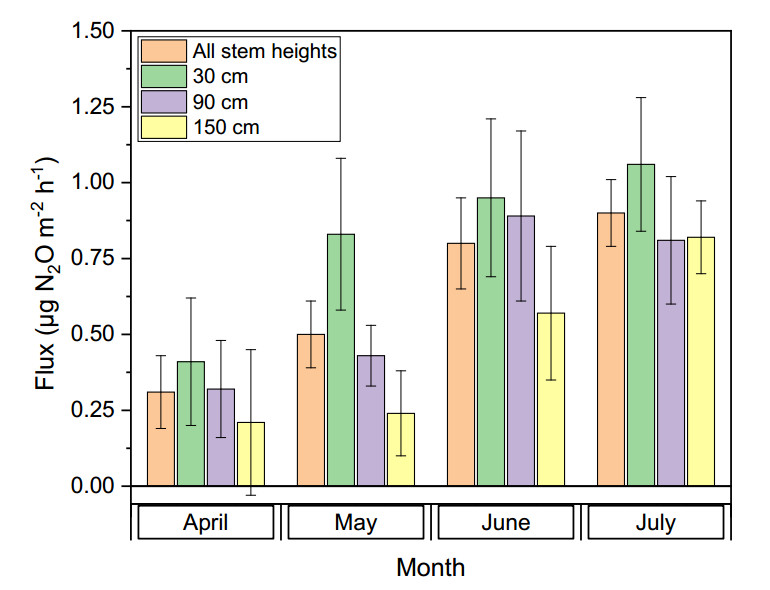
Trees growing in natural and managed environments have the capacity to act as conduits for the transport of greenhouse gases produced belowground to the atmosphere. Nitrous oxide (N2O) emissions have been observed from tree stems in natural ecosystems but have not yet been measured in the context of forested former landfill sites. This research gap was addressed by an investigation quantifying stem and soil N2O emissions from a closed UK landfill and a comparable natural site. Measurements were made by using flux chambers and gas chromatography over a four-month period. Analyses showed that the average N2O stem fluxes from the landfill and non-landfill sites were 0.63 ± 0.06 μg m–2 h–1 and 0.26 ± 0.05 μg m–2 h–1, respectively. The former landfill site showed seasonal patterns in N2O stem emissions and decreasing N2O fluxes with increased stem sampling position above the forest floor. Tree stem emissions accounted for 1% of the total landfill N2O surface flux, which is lower than the contribution of stem fluxes to the total surface flux in dry and flooded boreal forests.
Citation: A. Fraser-McDonald, C. Boardman, T. Gladding, S. Burnley, V. Gauci. Nitrous oxide emissions from trees planted on a closed landfill site[J]. AIMS Environmental Science, 2023, 10(2): 313-324. doi: 10.3934/environsci.2023018
[1] | Chukwuebuka C. Okafor, Juliet C. Ibekwe, Chinelo A. Nzekwe, Charles C. Ajaero, Chiadika M. Ikeotuonye . Estimating emissions from open-burning of uncollected municipal solid waste in Nigeria. AIMS Environmental Science, 2022, 9(2): 140-160. doi: 10.3934/environsci.2022011 |
[2] | Pasquale Avino, Maurizio Manigrasso . Ozone formation in relation with combustion processes in highly populated urban areas. AIMS Environmental Science, 2015, 2(3): 764-781. doi: 10.3934/environsci.2015.3.764 |
[3] | Luigi Falletti, Lino Conte, Andrea Maestri . Upgrading of a wastewater treatment plant with a hybrid moving bed biofilm reactor (MBBR). AIMS Environmental Science, 2014, 1(2): 45-52. doi: 10.3934/environsci.2014.2.45 |
[4] | Anwar Khan, Benjamin Razis, Simon Gillespie, Carl Percival, Dudley Shallcross . Global analysis of carbon disulfide (CS2) using the 3-D chemistry transport model STOCHEM. AIMS Environmental Science, 2017, 4(3): 484-501. doi: 10.3934/environsci.2017.3.484 |
[5] | María E. García, Lara S. Della Ceca, María I. Micheletti, Rubén D. Piacentini, Mariano Ordano, Nora J. F. Reyes, Sebastián Buedo, Juan A. González . Satellite and ground atmospheric particulate matter detection over Tucumán city, Argentina, space-time distribution, climatic and seasonal variability. AIMS Environmental Science, 2018, 5(3): 173-194. doi: 10.3934/environsci.2018.3.173 |
[6] | Patrick Wanjiru, Nancy Karuri, Paul Wanyeki, Paul Kioni, Josephat Tanui . Numerical simulation of the effect of diluents on NOx formation in methane and methyl formate fuels in counter flow diffusion flame. AIMS Environmental Science, 2020, 7(2): 140-152. doi: 10.3934/environsci.2020008 |
[7] | Isabel Pariente María, Martínez Fernando, ÁngelBotas Juan, AntonioMelero Juan . Extrusion of Fe2O3/SBA-15 mesoporous material for application as heterogeneous Fenton-like catalyst. AIMS Environmental Science, 2015, 1(2): 154-168. doi: 10.3934/environsci.2015.2.154 |
[8] | R. Kajaste, P. Oinas . Plastics value chain - Abatement of greenhouse gas emissions. AIMS Environmental Science, 2021, 8(4): 371-392. doi: 10.3934/environsci.2021024 |
[9] | Tiffany L. B. Yelverton, David G. Nash, James E. Brown, Carl F. Singer, Jeffrey V. Ryan, Peter H. Kariher . Dry sorbent injection of trona to control acid gases from a pilot-scale coal-fired combustion facility. AIMS Environmental Science, 2016, 3(1): 45-57. doi: 10.3934/environsci.2016.1.45 |
[10] | Sayali Sandbhor, Sayali Apte, Vaishnavi Dabir, Ketan Kotecha, Rajkumar Balasubramaniyan, Tanupriya Choudhury . AI-based carbon emission forecast and mitigation framework using recycled concrete aggregates: A sustainable approach for the construction industry. AIMS Environmental Science, 2023, 10(6): 894-910. doi: 10.3934/environsci.2023048 |
Trees growing in natural and managed environments have the capacity to act as conduits for the transport of greenhouse gases produced belowground to the atmosphere. Nitrous oxide (N2O) emissions have been observed from tree stems in natural ecosystems but have not yet been measured in the context of forested former landfill sites. This research gap was addressed by an investigation quantifying stem and soil N2O emissions from a closed UK landfill and a comparable natural site. Measurements were made by using flux chambers and gas chromatography over a four-month period. Analyses showed that the average N2O stem fluxes from the landfill and non-landfill sites were 0.63 ± 0.06 μg m–2 h–1 and 0.26 ± 0.05 μg m–2 h–1, respectively. The former landfill site showed seasonal patterns in N2O stem emissions and decreasing N2O fluxes with increased stem sampling position above the forest floor. Tree stem emissions accounted for 1% of the total landfill N2O surface flux, which is lower than the contribution of stem fluxes to the total surface flux in dry and flooded boreal forests.
N2O is a potent greenhouse gas (GHG) with an estimated radiative forcing of 0.17 W m–2 and a global warming potential of 298 over a 100-year timescale [1,2]. N2O is also a contributor to atmospheric NOx; approximately 10% of N2O that reaches the stratosphere is broken down via photolysis to NOx, which then catalytically destroys stratospheric O3 [3,4]. Identifying and quantifying the global sources and sinks of N2O is important for developing climate change mitigation strategies and reducing the rate of stratospheric O3 depletion. N2O is produced in soils and oceans via nitrification and denitrification [5,6]. In anaerobic soils, N2O is produced as an intermediate step during denitrification, where nitrous oxides are reduced to form N2 [7]. In aerobic soils, N2O is produced via nitrification, whereby NH3 and NH4+ are converted to NO3–, and N2O is produced as a by-product [7]. Terrestrial ecosystems account for c.33% of the total (natural and anthropogenic) global N2O emissions [2].
Trees growing in upland and wetland environments can provide a pathway for N2O produced belowground to the atmosphere [6,8,9]. N2O is transported through tree stems via absorption in the root zone and then diffusion through the xylem. N2O is emitted from tree stem surfaces after diffusing across the cambium and meristem to the bark, where it is released via lenticels [6]. Tree-mediated emissions can account for up to 10% of the total N2O ecosystem flux in upland environments, and up to 18% in wetlands [8,9,10]; however, stem N2O emissions have not been quantified in managed environments.
The remediation of landfill sites after closure often includes afforestation because it increases carbon sequestration, reduces water percolation into waste and improves the aesthetic appeal [11]. Trees growing on closed landfill sites emit methane (CH4) and carbon dioxide (CO2) [12,13]); however, their ability to emit N2O has not yet been investigated. N2O emissions from landfill sites can result from nitrification or denitrification, particularly when alternating aerobic and anaerobic zones exist within the waste [14]. N2O can also be formed in aerobic cover soils via methanotrophic nitrification, as methanotrophs co-oxidize CH4 and ammonia [5,15]. Landfill surface fluxes are highly variable (0.05 and 81.9 mg m–2 h–1), with total emissions from landfill and wastewater of c.0.3 Tg N2O yr–1 [2,14,16].
This study aimed to develop an understanding of GHG emissions from trees on closed landfill sites by quantifying N2O fluxes for the first time. A four-month field campaign was designed to investigate spatial and temporal patterns and quantify the contribution of trees to total landfill surface N2O fluxes. The hypotheses for the study were as follows:
1. Trees growing on closed landfill sites will emit more N2O than trees growing in a natural comparable woodland;
2. N2O stem fluxes will be higher in the summer months;
3. N2O emissions decrease with height of the tree trunks (above the ground).
The study sites, sampled trees and their characteristic measurements used in this investigation have previously been described by Fraser-McDonald et al. [12]. Sampling was carried out at a closed landfill and nearby non-landfill area. Waste was accepted at the landfill site from 1964 to 1998 before it was capped (clay of 1-m minimum depth; permeability: 10–7 m s–1) and covered with 2 m of topsoil. Gas and leachate control systems were installed, and a 1.22 ha woodland was created on the site in 2004. Trees were planted on the non-landfill site to create secondary woodland in 2003. The tree species sampled at both sites were Betula pendula, Fraxinus excelsior and Prunus avium. Soils at both sites were loamy clay, with underlying sedimentary bedrock and mudstone, and the mean annual temperature and rainfall were 14.1 ℃ and 565.5 mm, respectively [17]. Tree and soil measurements were made at both sites between April and July 2021. Monthly measurements were obtained from 15 trees and 15 soil locations over a 2-day period each month (between 8:00 and 17:00), with sampling at 30, 90 and 150 cm stem heights (from ground level).
Semi-rigid flux chambers (30 × 15 × 3 cm) constructed from clear polycarbonate and closed-cell silicone foam strips were fastened to trees with ratchet straps and sealed with airtight putty [12]. Soil flux measurements were taken by using rigid cylindrical chambers that were inserted into the soil (rigid polyvinyl chloride cylinders with a radius and depth of 15 cm) [12]. Each chamber was fitted with a lid prior to samples being taken. Tree chambers and soil chamber lids were fitted with rubber septa to allow gas samples to be obtained (Suba-Seal, Sigma-Aldrich). Ten-ml air samples were taken via the septa by using a luer lock syringe and a side port stainless steel needle (to minimize septum coring) (Sigma-Aldrich). Each sample was ejected into a pre-evacuated 5.9 ml flat-bottomed vial (Extetainer, Labco). Vials were over-pressured to minimize the effect of any leakage. Samples were taken from soil and tree chambers immediately after closure, and then again after 20, 40 and 60 minutes.
N2O gas fluxes were calculated by using the ideal gas equation and standardized for temperature and pressure [12]. Three tree and soil locations per site visit were selected prior to the field campaign to test for flux linearity. Each sample (0, 20, 40 and 60 minutes) was analyzed before regression lines were determined, and the associated R2 values were calculated. Once the linearity of fluxes was established, the remaining fluxes for each monthly site visit were calculated by using only the 0- and 60-minute samples.
Samples taken from the stem and soil chambers were analyzed by using gas chromatography. An Agilent 7890A gas chromatograph fitted with a micro-electron capture detector (μECD) and autosampler was used. Full details of this analysis are available in the Supplementary Methods.
Vial leakage tests were carried out to ensure the accuracy of stored samples over time. Vials were filled with a known N2O standard (1 ppm with ± 2% mixture accuracy) and stored for the same period of time as field samples from each month (April, May, June and July; three test vials per month). Regression analysis showed that the N2O concentration decreased over time; therefore, percentage corrections were applied to vial concentrations based on storage time (see Supplementary Methods for details).
Graphs were created in Origin (version 2020) and statistical tests were carried out using R (3.5.1) and SPSS (24). Parametric and non-parametric tests were employed to determine significant differences. Full details of the statistical tests used are detailed in the Tables S2 and S4. The methods described by Fraser-McDonald et al. [12] were used to scale up N2O stem and soil fluxes.
Average N2O tree stem emissions from all measurement heights at the closed landfill site during the measurement period from April to July 2021 were 0.63 ± 0.06 µg m–2 h–1. Mean soil N2O fluxes from the former landfill site were 25.5 ± 3.7 µg m–2 h–1. Average N2O stem (all measurement heights) and soil fluxes from the comparable natural woodland were 0.26 ± 0.05 µg m–2 h–1 and 3.53 ± 0.58 µg m–2 h–1, respectively.
Average monthly N2O fluxes from tree stems on the former landfill site varied significantly (at all stem measurement heights) (P < 0.01). N2O fluxes generally increased from April to July 2021 at all measurement heights, except for the 90 cm measurement height between June and July, where they were statistically the same (P > 0.05) (Figure 1). Average tree stem N2O fluxes were c.2 times greater in the summer months (June and July) than in the spring (April and May), with values of 0.85 ± 0.09 µg m–2 h–1 in the summer and 0.40 ± 0.08 µg m–2 h–1 in the spring (Table S3). The observed trend of increasing tree stem emissions from April through to July at all stem heights concurs with a previous study in which N2O emissions from tree stems in a boreal forest were higher in the summer months [9]. The pattern of increasing stem N2O emissions throughout the measurement period is likely due to the combined effect of variations in air temperature and soil moisture. Variations in stem fluxes at 30 cm correspond with rainfall patterns, as average UK rainfall was low in April 2021, but higher in May and July 2021 (Table S6). Additionally, measurements were taken from the former landfill site within 3 days of rainfall in May and July, but not in April and June 2021, and, on average, soil moisture was higher in May than in other months (Table S6). Relatively high rainfall in May and July would increase the soil water content, likely leading to higher rates of denitrification as O2 becomes more limited [18].
Soil N2O fluxes at the landfill site also varied significantly on a monthly basis (P < 0.01) (Figure 2). Soil N2O emissions in May 2021 were significantly higher than those in April (P < 0.01), June (P < 0.01) and July 2021 (P < 0.01). Soil fluxes in July were also significantly higher than those in June (P < 0.05). Higher soil N2O emissions from the landfill site in months with increased rainfall and soil moisture indicated that rainfall events resulted in higher rates of denitrification. This agrees with results from previous investigations on closed landfills, where N2O fluxes declined with lower soil moisture due to the effect on microbial populations in cover soils [5].
Increased air temperatures in summer were expected to cause a decrease in N2O stem flux rates, as N2O reductase activity increases with higher temperatures. This results in more N2O being converted to N2 during complete denitrification [19]. However, stem N2O emissions in June and July (the warmest months) were higher than in April and May. Because the average air temperatures in June and July were lower than the optimum temperature for denitrification in soils (25–30 ℃), the rate of denitrification may have increased, but not to the extent that complete denitrification to N2 dominated [20]. In contrast, soil N2O fluxes were not higher in months with lower rainfall and increased temperature (June 2021, for example). This suggests that there may be an additional temperature-dependent source of N2O production contributing to emissions from tree stems that is not present in soils. For example, N2O can be formed in plant tissues under anoxic conditions or after exposure to UV light [21]. If tree roots enter anaerobic zones in the soil or the landfill cap, N2O may be produced in mitochondria under anoxic conditions [22]. Measurements taken during this study were constrained by time and sample size; future research should build on this initial quantification of changing N2O emissions over time by evaluating seasonal trends across an entire year.
Average landfill tree stem N2O fluxes (for all months in the measurement period) at 30, 90 and 150 cm measurement heights were 0.81 ± 0.12 µg m–2 h–1, 0.61 ± 0.10 µg m–2 h–1 and 0.46 ± 0.10 µg m–2 h–1, respectively (Figure 3). There was a general trend of decreasing fluxes with increased stem height and a significant difference between N2O emissions at 30 and 150 cm (P < 0.05). Further analysis showed the same pattern of lower stem N2O fluxes with increased measurement height from ground level in each month during the observation period (Figure 1; Table S3). N2O emissions are expected to decrease with increased stem height when emissions are being channeled from an underground source [23]. For example, average N2O emissions from adult trees after soil fertilization in a temperate upland ecosystem decreased linearly with increased stem height [6]. The observed trend at the landfill site of decreasing N2O fluxes with increasing stem height is likely due to the N2O concentration in plant tissues becoming lower with height and, therefore, N2O diffusion being reduced [6]. In addition, higher parts of a tree stem generally have lower rates of transpiration, which could also explain the lower N2O flux rates with increased stem height [24,25]. These results indicate that N2O emitted by the trees growing at the landfill site originated from a belowground source, although it is not certain whether this source is in the waste or the cover soil. Although different sources of N2O were considered, deriving the exact source was beyond the scope of this study; future research should endeavor to identify the source of N2O emissions from tree stems at closed landfill sites. Also, closed landfills with varying ages and management practices should be investigated, as more recently closed sites have been shown to emit more N2O from the soil surface [14].
Spatial variations in stem and soil N2O fluxes from the closed landfill site (including data from all measurement heights and months) are shown in Figure 4. The highest average N2O stem fluxes were largely in the west and northwest, and the lowest average stem fluxes were in the east and southwest areas of the site. The largest N2O soil fluxes were observed on the eastern edge of the site and in relatively small areas in the southwest and northwest. The lowest average soil N2O fluxes were largely in the central area of the site. Unlike previously reported CH4 emissions from the landfill site [12], none of the sampled locations consistently emitted substantially more N2O than others. This indicates that the source of N2O was more uniform across the site than the source of CH4.
Observed average soil and stem fluxes indicated that the closed landfill site and comparable non-landfill area were net sources of N2O throughout the measurement period. Landfill soil N2O measurements ranged from 0.270 to 153.9 µg m–2 h–1, which is comparable to the lower end of the range of N2O fluxes previously measured from landfill surfaces with soil cover (–1.7 to 575 µg m–2 h–1) [16]. The highest soil N2O measurements from this investigation were of the same order of magnitude as a site in Sweden with a cover comprised of ash, bark and sand and no gas extraction (fluxes ranged from –1.7 to 163 µg m–2 h–1) [16]. The relatively low fluxes from the investigated landfill are likely due to the age of the site (closure occurred in 1998), as well as factors such as soil moisture, soil temperature and the availability of mineral nitrogen (the effects of soil temperature and soil moisture are discussed further below) [14]. N2O fluxes from this site were also substantially lower than those from active sites and those with a cover of sewage sludge [14].
N2O fluxes from tree stems were significantly different between the closed landfill site and the comparable non-landfill location (P < 0.01). Average stem N2O emissions from the landfill (0.63 ± 0.06 µg m–2 h–1) were higher than those from the non-landfill site (0.26 ± 0.05 µg m–2 h–1) (Figure 5). There were also significant differences in the stem N2O fluxes between the two sites when fluxes at each measurement height from the ground were considered individually. N2O fluxes from trees at the landfill site were significantly larger than those at the non-landfill site at 30 and 90cm measurement heights (P < 0.05 and P < 0.01, respectively) (Table S7). There was a marginal difference in the stem N2O fluxes between the two sites at the 150 cm measurement height (P = 0.071). Average soil N2O fluxes from the landfill and non-landfill sites during the measurement period were 25.48 ± 3.72 µg m–2 h–1 and 3.53 ± 0.58 µg m–2 h–1, respectively. These differences may be due to factors such as soil moisture, soil temperature or nitrogen availability [14]. The effects of air temperature and soil moisture on tree stem N2O fluxes was discussed above. The average stem N2O flux for the landfill site was within the reported range of fluxes from trees in a non-flooded temperate woodland [9]. The range of stem flux values from the landfill site (–1.43 to 3.39 µg m–2 h–1) was smaller than that from a temperate woodland (–11.87 to 30.28 µg m–2 h–1), where variability was reported to be high [9]. This indicates that trees planted at closed landfill sites do not emit atypical levels of N2O compared with previously published emissions from trees planted in natural woodlands.
Tree stem N2O emissions from this landfill site were substantially lower than previously reported CH4 and CO2 emissions [12]. When the 100-year global warming potentials (GWP100) were considered, the average N2O flux from this closed landfill was equivalent to 166.95 µg CO2e m–2 h–1. This is lower than the average tree stem CH4 flux for the same site (320 µg CO2e m–2 h–1), and substantially lower than reported CO2 stem fluxes (53.3 mg CO2e m–2 h–1) [12]. The upscaled landfill N2O soil surface flux from the landfill site was 2723 g N2O yr–1, and the upscaled stem flux was 26 g N2O yr–1. The stem and soil emissions were equal to 7.7 and 811.5 kg CO2e yr–1, respectively. The tree stem N2O emissions accounted for c.1% of the estimated total landfill surface flux. This agrees with findings from a mesocosm study in which tree stem emissions accounted for 1–3% of the total ecosystem N2O emissions [8]. These results indicate that excluding N2O fluxes from tree stems may result in an underestimation of the amount of N2O emitted from former landfills. However, estimates of the contribution of stem fluxes to the total surface N2O flux in dry and flooded boreal forests were higher, with values of 8% and 18%, respectively [10].
This study has revealed that landfill sites with forested areas have the potential to be a net source of N2O, with tree stem fluxes accounting for 1% of the total landfill surface N2O emissions. Fluxes from the landfill site showed temporal variability, as N2O emissions in the June and July were higher than those in April and May, likely due to variations in temperature and soil moisture. Stem fluxes were lower with increased height from the ground, and this spatial variation indicated a belowground source of N2O. Findings presented here demonstrate that trees planted on a closed landfill site have the capacity to emit more N2O than a comparable non-landfill area. However, the contribution of stem N2O fluxes to the total surface flux on the former landfill was at a similar or lower magnitude than that of fluxes previously reported from natural forested ecosystems. There is a need for further investigation into the environmental factors that influence the magnitude of N2O fluxes from landfills and the source of these N2O emissions. Further measurements should also be taken to identify seasonal trends in N2O emissions over an entire year. This would aid in further explaining the variability in N2O fluxes and mitigating legacy emissions from closed landfill sites. These results indicate that trees growing on closed landfills have the capacity to emit N2O from a belowground source, and that including N2O released via tree stems in GHG budget assessments for former landfills may be beneficial.
The manuscript is based on thesis work which was internally funded by The Open University. We are grateful to Julie Fraser and Ben Sharp for fieldwork assistance, Fotis Sgouridis (University of Bristol) for sample analysis, and to the EGL technicians at the Open University for their support. We would also like to thank the local authorities and trusts responsible for the field sites for providing access to conduct fieldwork.
All authors declare no conflict of interest regarding the publication of this paper.
[1] | Myhre G, Shindell D, Bréon FM, et al. (2013) Anthropogenic and natural radiative forcing. In: Stocker TF, Qin D, Plattner GK, et al. (eds.) Climate Change 2013: The Physical Science Basis. Contribution of Working Group Ⅰ to the Fifth Assessment Report of the Intergovernmental Panel on Climate Change. Cambridge, United Kingdom and New York, NY, USA: Cambridge University Press. |
[2] |
Tian H, Xu R, Canadell J, et al. (2020) A comprehensive quantification of global nitrous oxide sources and sinks. Nature 586: 248–256. https://doi.org/10.1038/s41586-020-2780-0 doi: 10.1038/s41586-020-2780-0
![]() |
[3] | Ravishankara AR, Daniel JS, Portmann RW (2009) Nitrous oxide (N2O): The dominant ozone-depleting substance emitted in the 21st century. Science 326: 123–125. https://www.science.org/doi/10.1126/science.1176985 |
[4] |
Portmann RW, Daniel JS, Ravishankara AR (2012) Stratospheric ozone depletion due to nitrous oxide: Influences of other gases. Philos Trans R Soc B 367: 1256–1264. https://doi.org/10.1098/rstb.2011.0377 doi: 10.1098/rstb.2011.0377
![]() |
[5] |
Mandernack K, Kinney CA, Coleman D, et al.(2000) The biogeochemical controls of N2O production and emission in landfill cover soils: The role of methanotrophs in the nitrogen cycle. Environ Microbiol 2: 298–309. https://doi.org/10.1046/j.1462-2920.2000.00106.x doi: 10.1046/j.1462-2920.2000.00106.x
![]() |
[6] |
Dìaz-Pinés E, Heras P, Gasche R, et al. (2016) Nitrous oxide emissions from stems of ash (Fraxinus angustifolia vahl) and European beech (Fagus sylvatica l.). Plant Soil 398: 35–45. https://doi.org/10.1007/s11104-015-2629-8 doi: 10.1007/s11104-015-2629-8
![]() |
[7] | Smithson P, Addison, K, Atkinson K (2002) Fundamentals of the Physical Environment, London, Routledge. |
[8] |
Machacova, K, Papen, H, Kreuzwieser, J et al. (2013) Inundation strongly stimulates nitrous oxide emissions from stems of the upland tree Fagus sylvatica and the riparian tree Alnus glutinosa. Plant Soil 364: 287–301. https://doi.org/10.1007/s11104-012-1359-4 doi: 10.1007/s11104-012-1359-4
![]() |
[9] |
Moldaschl, E, Kitzler, B, Machacova, K et al. (2021) Stem CH4 and N2O fluxes of Fraxinus excelsior and Populus alba trees along a flooding gradient. Plant Soil 461: 407–420. https://doi.org/10.1007/s11104-020-04818-4 doi: 10.1007/s11104-020-04818-4
![]() |
[10] |
Machacova K, Bäck J, Vanhatalo A, et al. (2016). Pinus sylvestris as a missing source of nitrous oxide and methane in boreal forest. Nature Sci Rep 6: 23410. https://doi.org/10.1038/srep23410 doi: 10.1038/srep23410
![]() |
[11] | EPA (Environmental Protection Agency) (2003) Evapotranspiration landfill cover systems fact sheet. https://clu-in.org/download/remed/epa542f03015.pdf. |
[12] |
Fraser-McDonald A, Boardman C, Gladding T, et al. (2022a) Methane emissions from trees planted on a closed landfill site. Waste Manage Res 40: 1618–1628. https://doi.org/10.1177/0734242X221086955 doi: 10.1177/0734242X221086955
![]() |
[13] |
Fraser-McDonald A, Boardman C, Gladding T, et al. (2022) Methane emissions from forested closed landfill sites: Variations between tree species and landfill management practices. Sci Total Environ 838: 156019. https://doi.org/10.1016/j.scitotenv.2022.156019 doi: 10.1016/j.scitotenv.2022.156019
![]() |
[14] |
Rinne J, Pihlatie M, Lohila A et al. (2005) Nitrous oxide emissions from a municipal landfill. Environ Sci Technol 39: 7790–7793. https://doi.org/10.1021/es048416q doi: 10.1021/es048416q
![]() |
[15] |
Zhang H, He P, Shao L (2009) N2O emissions at municipal solid waste landfill sites: Effects of CH4 emissions and cover soil. Atmos Environ 43: 2623–2631. https://doi.org/10.1016/j.atmosenv.2009.02.011 doi: 10.1016/j.atmosenv.2009.02.011
![]() |
[16] |
Börjesson G, Svensson BH (1997) Nitrous oxide emissions from landfill cover soils in Sweden. Tellus 49B: 357–363. https://doi.org/10.1034/j.1600-0889.49.issue4.2.x = doi: 10.1034/j.1600-0889.49.issue4.2.x=
![]() |
[17] | Lewis E, Quinn N, Blenkinsop S, et al. (2019) Gridded estimates of hourly areal rainfall for Great Britain (1990–2014). Available: https://eip.ceh.ac.uk/data |
[18] |
Bollmann A, Conrad R (1998) Influence of O2 availability on NO and N2O release by nitrification and denitrification in soils. Global Change Bio 4: 387–396. https://doi.org/10.1046/j.1365-2486.1998.00161.x. doi: 10.1046/j.1365-2486.1998.00161.x
![]() |
[19] |
Wang X, Jia M, Lin X, et al. (2017) A comparison of CH4, N2O and CO2 emissions from three different cover types in a municipal solid waste landfill. J Air Waste Manage 67: 507–515. https://doi.org/10.1080/10962247.2016.1268547 doi: 10.1080/10962247.2016.1268547
![]() |
[20] | Skiba U (2008) 'Denitrification', in Jørgensen, S E & Fath, B D (eds.) Encyclopedia of Ecology. Elsevier B.V., 866–871. Available: https://www.sciencedirect.com/science/article/pii/B9780080454054002640?via = ihub |
[21] |
Bruhn D, Albert KR, Mikkelsen TN, et al. (2014). UV-induced N2O emission from plants. Atmos Environ 99: 206–214. https://doi.org/10.1016/j.atmosenv.2014.09.077 doi: 10.1016/j.atmosenv.2014.09.077
![]() |
[22] |
Timilsina A, Zhang C, Pandey B, et al. (2020) Potential pathway of nitrous oxide formation in plants. Front Plant Sci 11: 1177.https://doi.org/10.3389/fpls.2020.01177 doi: 10.3389/fpls.2020.01177
![]() |
[23] | Yamulki S (2017) Tree emissions of CH4 and N2O: Briefing and review of current knowledge Available: https://www.forestresearch.gov.uk/documents/7487/Tree_emission_of_CH4_and_N2O_Sirwan_Yamulki_Oct2017-online.pdf. |
[24] |
Perämäki M, Nikinmaa E, Sevanto S, et al. (2001) Tree stem diameter variations and transpiration in Scots pine: An analysis using a dynamic sap flow model. Tree Physiol 21: 889–897. https://doi.org/10.1093/treephys/21.12-13.889 doi: 10.1093/treephys/21.12-13.889
![]() |
[25] |
Pihlatie M, Ambus P, Rinne J, et al. (2005) Plant-mediated nitrous oxide emissions from beech (Fagus sylvatica) leaves. New Phytol 168: 93–98. https://doi.org/10.1111/j.1469-8137.2005.01542.x doi: 10.1111/j.1469-8137.2005.01542.x
![]() |
![]() |
![]() |