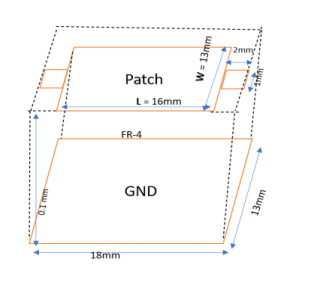
A bandwidth enhanced multilayer Electromagnetic Band Gap (EBG) structure to reduce the simultaneous switching noise (SSN) in high frequency operating circuits, which useful for the satellite communication application, is presented in this paper. A proposed stack structure is mathematically analyzed by the dispersion method and transmission matrix method. Simulation results show good mitigation of SSN in scattering parameters and signal integrity in terms of eye diagrams. We have also checked for power integrity analysis using self-impedance. The proposed structure gives a good SSN suppression at -30 dB from 817 MHz to 26.32 GHz, around 25.50 GHz bandwidth and also reduces the cavity mode resonance within the stopband range. The proposed multilayer structure is compared with planar EBG plane and reference board. It is also compared with published results.
Citation: Manisha R. Bansode, Surendra S. Rathod. A bandwidth enhanced multilayer electromagnetic bandgap structure to reduce the simultaneous switching noise[J]. AIMS Electronics and Electrical Engineering, 2023, 7(4): 406-420. doi: 10.3934/electreng.2023021
[1] | Vasudevan Karuppiah, UmaMaheswari Gurusamy . Compact EBG structure for ground bounce noise suppression in high-speed digital systems. AIMS Electronics and Electrical Engineering, 2022, 6(2): 124-143. doi: 10.3934/electreng.2022008 |
[2] | José M. Campos-Salazar, Roya Rafiezadeh, Juan L. Aguayo-Lazcano, Constanza Márquez . Reduction of harmonic distortion in electromagnetic torque of a single-phase reluctance motor using a multilevel neutral-point-clamped DC-AC converter. AIMS Electronics and Electrical Engineering, 2025, 9(2): 215-242. doi: 10.3934/electreng.2025011 |
[3] | Shimin V V, Varsha A Shah, Makarand M Lokhande . An advanced semiconductor material selection for switching devices in electric vehicles using three multiple attribute decision making methods. AIMS Electronics and Electrical Engineering, 2020, 4(4): 369-388. doi: 10.3934/ElectrEng.2020.4.369 |
[4] | Ch Priyanka, D Venkata Ratnam, Sai Krishna Santosh G . A Review on design of low noise amplifiers for global navigational satellite system. AIMS Electronics and Electrical Engineering, 2021, 5(3): 206-228. doi: 10.3934/electreng.2021012 |
[5] | Shaswat Chirantan, Bibhuti Bhusan Pati . Torque and d-q axis current dynamics of an inverter fed induction motor drive that leverages computational intelligent techniques. AIMS Electronics and Electrical Engineering, 2024, 8(1): 28-52. doi: 10.3934/electreng.2024002 |
[6] | Tarun Naruka, Debasis Tripathy, Prangya Mohanty . Power quality enhancement by a solar photovoltaic-based distribution static compensator. AIMS Electronics and Electrical Engineering, 2025, 9(2): 192-214. doi: 10.3934/electreng.2025010 |
[7] | Fadhil A. Hasan, Lina J. Rashad . Combining fractional-order PI controller with field-oriented control based on maximum torque per ampere technique considering iron loss of induction motor. AIMS Electronics and Electrical Engineering, 2024, 8(3): 380-403. doi: 10.3934/electreng.2024018 |
[8] | Mulualem T. Yeshalem, Baseem Khan, Om Prakash Mahela . Conducted electromagnetic emissions of compact fluorescent lamps and electronic ballast modeling. AIMS Electronics and Electrical Engineering, 2022, 6(2): 178-187. doi: 10.3934/electreng.2022011 |
[9] | Rasool M. Imran, Kadhim Hamzah Chalok, Siraj A. M. Nasrallah . Innovative two-stage thermal control of DC-DC converter for hybrid PV-battery system. AIMS Electronics and Electrical Engineering, 2025, 9(1): 26-45. doi: 10.3934/electreng.2025002 |
[10] | Masoud Kiani, Jafar Adabi, Firuz Zare . Increasing LTCC inductance density by using inverse coupling technique and multi-permeability structure. AIMS Electronics and Electrical Engineering, 2018, 2(3): 85-102. doi: 10.3934/ElectrEng.2018.3.85 |
A bandwidth enhanced multilayer Electromagnetic Band Gap (EBG) structure to reduce the simultaneous switching noise (SSN) in high frequency operating circuits, which useful for the satellite communication application, is presented in this paper. A proposed stack structure is mathematically analyzed by the dispersion method and transmission matrix method. Simulation results show good mitigation of SSN in scattering parameters and signal integrity in terms of eye diagrams. We have also checked for power integrity analysis using self-impedance. The proposed structure gives a good SSN suppression at -30 dB from 817 MHz to 26.32 GHz, around 25.50 GHz bandwidth and also reduces the cavity mode resonance within the stopband range. The proposed multilayer structure is compared with planar EBG plane and reference board. It is also compared with published results.
In the most recent technological advancements, the creation of high frequency operating multilayer printed circuit boards have received considerable attention. It offers a dependable and economical manufacturing technique that efficiently realizes passive planar components, power buses and high-speed interconnects. Structures are employed in a variety of electronic applications, such as high-speed digital, analogue and microwave circuits. A sizable amount of multilayer PCB technology has been developed for high-speed circuit design for power processing [1]. Additionally, it was developed for mixed-signal devices that can fit onto a small space on PCBs, which often exchange metal traces and planes, as opposed to high-speed PCBs that require numerous layers. However, the signal and power integrity issues continue to be more difficult despite the design advancements in the usage of numerous layers and device placement.
Present-day mixed-signal systems are particularly susceptible to simultaneous switching noise (SSN) on the signal and ground planes because they combine high-speed CPUs, RF circuits and analogue components onto just one multilayer printed circuit board. The ground and signal layers are affected by the SSN that digital switching components emit. It greatly reduces system performance, which lowers the noise margin and lowers sensitivity. As a result of recent advancements in digital devices' operational frequencies with different data rates, SSN spectral contents now cover a wide frequency range for mixed mode applications [1,2]. Due to continuous increases in data rate and clock frequency, a significant problem of SSN, is becoming more of an issue for higher frequency applications. In high-speed printed circuit boards, a voltage fluctuation known as simultaneous switching noise is an effect of resonance in the cavity mode in signal planes [2,3]. Power integrity and signal integrity are hampered by resonance modes on the ground and power planes. High-speed circuits and PCBs also frequently experience issues with electromagnetic interference. According to studies, signal and power integrity issues have effects on both the temporal and voltage domains. Therefore, it is essential for electronics systems to ensure signal and power integrity. It may reduce the effect of noise [4‒6] by adjusting the time of the system.
Simultaneous switching noise is reduced by High Impedance Surfaces (HIS), such as Electromagnetic Band Gap (EBG). EBG has been discovered to be useful in many applications, because of its special wave attenuation properties. Periodic patches in the form of mushrooms are present in EBG designs, and the patches are connected to the ground using vias [7]. Wide band gaps for high-speed circuits have been proposed using hybrid EBG structures, which integrate multiple designs [8,9,10,11]. The use of mixed combination EBG structures, which combine two or more structures, have been proposed for high-speed circuits [11,12,13,14]. Electromagnetic bandgap structures are widely used because of their miniature size, which is advantageous for bandgap spectrum enhancement by mitigating their power noise effect at GHz range frequencies. Many planar EBG designs [15,16,17,18,19,20] are used to reduce the effect of electromagnetic compatibility from printed circuit boards. The attenuation reduction in fast speeds design is accomplished via a succession of patches fastened to several types of meander lines and bridges [21,22,23,24,25]. From the published review mentioned above, it is clear that SSN attenuation and band expansion are receiving increased attention.
The majority of planar EBG structure research that focuses on enlarging the bandgap or the EBG layer is always worked as an outside multilayer by some operation. The ordinary filtering function of the planar EBG used as an internal layer cannot be assured when particular design changes fail to occur. When buried in the stack-up, the EBG layer is frequently sandwiched between two ground plates. The requisite bandgap is not provided by the top cavity or the lower cavity associated with used configuration. The modes of the two solid plates may be observed inside the appropriate bandgap as a result of noise coupling that takes place between the two cavities through the EBG layer gaps. By preventing the resonances of the two solid planes cavities, the filtering capabilities connected to the top and lower cavities can be recovered. A band gap effect can be produced by connecting the two solid ground planes using vias. In order to create a ring that is circular in shape around the vias, the EBG layer separates the patches from the vias [16,17].
Even though EBG structures are currently in use, they are not appropriate for power noise reduction at the lower frequency limits due to their lower frequency flow, which often uses more from higher frequency ranges. The flow of present EBG structures must be reduced without impacting the suppression in order to bandwidth enhancement encompassing the lower frequency limits. In multilayer PCBs, broad reduction of power/ground noise has been achieved using EBG configurations but not with lower limit of flow. Stepped impedance (SI) structures are the foundation of one of the basic configurations of EBG structures [17,18,19,20]. Stepped impedance EBG structures have been the focus of numerous investigations due to its simple design and broad stopband with significant suppression level. The goal is to decrease flow in lower frequency limits with proper EBG unit cells. The objective of using appropriate EBG cells is to reduce flow in lower frequency limitations.
In this paper, a rectangular EBG unit cell is designed to broaden the reduction of SSN linking with low flow. It is suggested a stepped impedance (SI) multilayer EBG structure, low pass filters with multiple lines of exceptionally high and low specific impedance will be used by SI. These filters are called stepped-impedance filters, High-Impedance (H-I) filters or Low-Impedance (L-I) filters and are used because they are simpler to design and need less space. The topmost signal layer and internal signal layers of the signal planes in the structure are connected upright by the metal patches to produce a maximum impedance called H-I patch and a minimum impedance is called L-I patch in the suggested multilayer design. The H-I and L-I patches with the SI design are connected through via upright from other layers, the suggested design offers substantial branch inductance in contrast to the planar two-dimensional EBG structure and reference plane. The SSN reduction bandwidth was then improved using rectangular patch design EBG cell size, which resulted in a decrease in flow. Our main idea of this paper is to design a multilayer structure using a step impedance technique, which has advantages of broad stopband, high suppression level and simplified broad connections. If one of the two surfaces is etched appropriately, a series of rectangular patches connected by thin bridges/vias can be produced, altering the resonance behavior of the mentioned perfect cavity. Due to the forced conduction of current through the bridges/vias, the changing geometry has an impact on the electromagnetic field inside the cavity at the resonant frequencies. This effect affects the downshifted resonant frequencies, shown as the operation principle for a suggested design. It is observed that the flow value starts at 817 MHz and that the considerable stopband addition occurs between 817 MHz and 26.32 MHz. This frequency range is suitable for microwave applications including microwave ovens, digital television broadcasting, satellite systems and radar transmitters.
The following subsections describe design and full wave simulation of the proposed multilayer EBG structure utilizing a wide stopband for SSN mitigation.
EBG structures have a greater bandgap and demonstrate permeability-negative behavior at frequencies above their resonant frequencies. It is supposed that a parallel plate waveguide has a thinner dielectric layer compared to its width and length. Helmholtz equations are used to calculate resonance frequencies [21,22].
fTMpq=c2π√μr.ϵr√(pπ)A2+(qπ)B2 | (2.1) |
where 'p' is length of assumed structure, 'q' is width of assumed structure and 'c', 𝜖𝑟 and 𝜇𝑟 are permittivity velocities. In general patch and substrate provide minimum impedance whereas substrate and branch provide maximum impedance. It is possible to determine characteristics impedance by [23],
Z0=ηdW√LC | (2.2) |
where, 'W' is patch width, 'd' is the dielectric thickness, 'L' is a patch and other plate inductance. 'C' is a branch and other plate capacitance and '𝜂' is FR4 internal impedance. This establishes a stopband in the desired frequency range.
flow=12πc√μr.ϵrZ0L∗W | (2.3) |
Initial frequency (flow) and higher frequency limit (fhigh) determination is done by the equation (2.3) and (2.4) for mode 1,
fhigh=12c√μr.ϵrZ0W | (2.4) |
When the starting low frequency of the bandgap has a mode value of (zero, one), the patch dimensions are calculated [24] using Eq (2.4). The impacts of length and breadth variations, as well as the likelihood of reaching a greater bandgap, are used to determine the branch dimensions.
The reference plane (without EBG) is more susceptible to being affected by simultaneous switching noise. It is also designed without an EBG reference plane with the same dimensions, for correct comparison of suggested design. Proposed multilayer structure is designed with a stepped impedance method and with a rectangular shape patch. The suggested EBG structure is made of six periodic power plane layers with metal patches connected upright with the other layer patch and there is an alternate complete ground layer. Rectangular shaped designed unit cell with length of the patch is 16 mm and width of patch is 13 mm. Each patch is connected with branch connections at the left and right side, branch length is 2 mm and the branch width is 1 mm in dimensions. The distance between H-I and L-I patches is 0.1 mm and for both H-I patches is 0.4 mm as depicted in Figure 1.
As shown in Figure 2, the H-I and L-I patches in the various layers are linked to one another by an upright connection of via with a branch made up of small structure on both the sides on the top and inner layers. Additionally, H-I patches are internally connected through via with different distances and layers. An FR-4 epoxy with a loss tangent (𝛿) of 0.02 and a relative permittivity (𝜖𝑟) of 4.4 is used as a dielectric material. Ports are created as shown in Figure 3. The complete suggested multilayer structure with all the layers is in sink as shown in Figure 3. Ground vias are also present in original design but not mentioned in Figure 3 for the clear visualization of design. A common limit is set up to reduce simultaneous switching noise. High-frequency structure simulator (HFSS) by an Analysis System (ANSYS) performs the input-impedance and scattering characteristics as a frequency response [26].
Planar structure is designed for a better comparison with multilayer structure. For one voltage level, the structure in Figure 3 has a continuous layer, while the EBG designed plane is at another voltage level in two-dimension form. As seen in Figure 11 a, a top view of a 2 X 6 structure is depicted, with the top layer being a periodic structure, or EBG, and the base layer, being a continuous ground plane. The plane of ground and the EBG designed power plane are separated by a dielectric layer. FR-4 epoxy used as a dielectric material. Specially created ports make it simpler to check insertion loss. There are six rectangular patches that are linked together by branches, in a similar manner of proposed multilayer design as shown in Figure 3. The branches add more inductive impact, whereas patches add capacitive impact to the design, causing the stopband to broaden in the scattering parameters. To simulate the scattering parameter, the ANSYS High Frequency Structure Simulator (HFSS) is utilized [26]. The suggested multilayer design transmission coefficient (S21) is shown in Figure 4 and it provides a satisfactory stopband from 817 MHz to 26.32 GHz frequency. It is also checked for comparison of reflection coefficient S11 and S22 of suggested design as in Figure 5. It also shows good agreement of both reflection coefficients.
In these sections, a unit cell simulation in the ANSYS HFSS eigenmode solution is shown for the purpose of conducting a dispersion diagram. The equivalent circuit for 1-D structure is designed for the calculations of dispersion equation with the help of ABCD parameters using the transfer matrix method [27].
Extraction of the model of an equivalent circuit for the multilayer EBG design is an important step [25]. As depicted in Figure 6, an equivalent circuit is extracted to design a one-dimensional structure. The upright branch can be represented as an inductance via 'Vib' and branch 'Lbr' by combining the inductance of vias, as well as the branch inductance on the topmost and inner layers. The capacitance 'Cr' serves as a representation for the H-I and the associated ground plane. According to Figure 6, the capacitance 'Cr' represents the L-I patch and the ground plane capacitance. The patch has patch inductance 'Lr', divided in two parts with capacitance.
Dispersion analysis of the proposed structure using eigenmode and transmission matrix has been used in this work as given in [23]. The one-dimensional periodic single cell, illustrated in Figure 1, is considered for the analysis. A single cell is connected on both sides with branches and vias. The ground vias connections also exist in the work, but are not mention in the calculations. It is assumed a transmission line segment for the mathematical calculations.
Tunit cell=[1Zvi01][1Zbr01][coskTjZosinkTjYosinkTcoskT][1Zbr01][1Zvi01] | (3.1) |
where, Zvi=jwL, Impedance of branch and via, kT is transmission line segment of phase delay,
k=2πf√μϵ | (3.2) |
where µ is permeability and 𝜖 permittivity of dielectric FR4. Zo is impedance and Yo is admittance of assumed segment, w=2πf, where f is frequency.
The transmission matrix method uses ABCD parameters as in Figure 7, consider finite length transmission line, with input and output terminals in the form of voltage and current. Hence, the relation between input and output terminal and current and voltage vector is given below,
S21=2A+BZo+C∗Zo+D | (3.3) |
Equation 3.3 is shown scattering parameters in the form of ABCD matrix.
[VvIv]=Tunitcell[Vv+1Iv+1] | (3.4) |
After some calculations obtained equations are,
[VvIv]=[ABCD][Vv+1Iv+1] | (3.5) |
In this case, γ is equal to α+jβ as given in [30],
[VvIv]=eγt[Vv+1Iv+1] | (3.6) |
The final expression is obtained for the dispersion analysis, shown in Figure 8. A dispersion diagram for the equation is almost similar to the original dispersion diagram obtained from eigenmode simulation in the finite element software. The blue dotted plot indicates equation representation and the red solid graph shows the unit cell eigen plot.
coskT=coskT−2πflowZosinkT | (3.7) |
System performance can be analyzed using input impedance parameters and S-parameters. The input impedance (Z11) and scattering parameters (S21) in electromagnetic compatibility are necessary components for determining low impedance [28].
The suggested multilayer EBG plane does pretty well in decreasing SSN at higher frequencies. A lower-frequency threshold, reduction depth and reduction bandwidth are requirements for SSN mitigation. It is demonstrated that noise reduction works well in the MHz to 30 GHz frequency band when utilizing the full wave simulator for scattering parameters. The ground and power plane H-I and L-I patch and via connections of the proposed EBG plane behave like a parallel plate waveguide.
Comparative simulation results are represented as without EBG solid plane, two-dimensional planar structure and suggested multilayer EBG design. The results of the simulations demonstrate that, as compared to a solid plane and planar structure with a proposed multilayer design (blue solid plot), the bandgap range of 817 MHz to 26.32 GHz is around the 25.50 GHz bandwidth, as shown in Figure 9, and the proposed structure minimizes wideband noise by a -30 dB isolation level. It is observed that, without an EBG reference plane (red color dotted plot) showing a wide stopband in simulation, it only gives a bandgap at lower frequencies and fails to get wide bandgap at higher values. Moreover, planar structure (green dashed plot) shows a 13.56 GHz to 30 GHz bandgap at higher frequencies but it fails to achieve good stopband range at lower frequencies. It is demonstrated that the proposed structure gives a wide band mitigation of SSN about 25.50 GHz bandwidth.
In order to see how well the planned structure suppresses SSN, the design is essential to look at its signal propagation capabilities [28]. Impact of noise on system performance is depicted in an eye diagram. Signal quality in high-speed designs is visually represented by an eye diagram. MATLAB software [29] is used in this work to model eye diagrams. An eye diagram is used to conduct a signal integrity analysis of the solid board, planar board and proposed multilayer EBG structure. For the procedure for the eye diagram plotting, a touchstone file is imported from the simulator software HFSS. The number of poles is utilized to calculate the transfer function and rotational function for imported data. The spinning object frequency response has been compared to the original data. Finally, the rotational function that applies to the object to a randomly selected 100 Mbps pulse was plotted on an eye diagram using all measurements like time, amplitude and jitter.
Figure 10, Figure 11 and Figure 12 show the structure and eye diagram of a reference board, planar board and proposed multilayer board respectively. Characteristics of the eye diagrams are obtained in terms of two parameters like time, amplitude. The crucial part for evaluating the quality of an eye pattern is maximum eye opening (MEO), which exhibits noise voltage, and maximum eye width (MEW), which exhibits temporal jitter. The MEO and MEW values for the suggested structure are 1.94 V and 19.61 ns, respectively, as seen in Figure 12b, while the values for the reference plane are 1.03 V and 5.73 ns and for the planar plane are 1.44 V and 9.09 ns, as shown in Figure 10b and Figure 11b. With the suggested structure for MEO, MEW has a concerning high signal to noise ratio (SNR) of 26.99 with comparison without an EBG board. Comparison Table 1 provides improved results for the suggested design.
Eye Measurement and Parameters | Reference structure | 2-D planar structure | Proposed Multilayer EBG |
In terms of amplitude -Eye Height | 1.03 V | 1.44 V | 1.94 V |
In terms of amplitude -Vertical Opening | 1.03 V | 1.44 V | 1.94 V |
In terms of amplitude-SNR | 3.84 | 6.09 | 26.99 |
In terms of time-Eye Width | 4.53 ns | 8.31 ns | 19.61 ns |
In terms of time-Horizontal Opening | 5.73 ns | 9.09 ns | 19.61 ns |
Any hardware design effectiveness can be examined using reflection coefficient and the input impedance parameter. The Z parameter self-impedance (Z11) is used to assess low impedance. The target impedance ought to be lower than a particular impedance for a system to maintain power integrity [25,28,30]. It is necessary to maintain a system impedance below the desired impedance because of resonance modes brought on by cavity effects in the signal plane. In the MHz range, decoupling capacitors are utilized, but at higher frequencies, they are unable to maintain adequate power integrity. In order to control impedance over a greater frequency range by reducing cavity modes, EBG structures are used at higher frequencies. Figure 13 show the input impedance of the solid plane, 2-D planar plane and proposed multilayer structure to explore the effects of cavity mode damping in the proposed EBG design. It is discovered that more cavity modes are suppressed for a larger range of frequencies from lower value GHz to 30 GHz, in the proposed multilayer EBG plane compared to the solid plane and planar plane. The self-impedance is larger in the reference board and planar board because of signal bus resonance, but it is less in the suggested multilayer structure because of a reduction in power bus resonance due to the arrangement and via connections as shown in Figure 13.
There is comparison of published results with the proposed structure. Table 2 compares the proposed multilayer structure to the results that have been published. In Ref. [1], 12.7 mm of unit cell with 19.35 GHz bandgap was employed. Ref. [31] has a 3.9 GHz bandgap and a 15 mm by 15 mm of unit cell. Ref [32] provides 4.3 GHz bandwidth. With a 25.50 GHz bandwidth and a 13 mm X 16 mm patch size, the proposed multilayer structure exhibits good bandwidth enhancement and noise suppression between 817 GHz and 26.32 GHz.
EBG Structure | Suppression Depth = -30dB | Suppression Depth = -30dB | Suppression Depth = -30dB |
Ref. and Patch size in mm | Lower frequency in GHz | Higher frequency in GHz | Bandwidth in GHz |
Ref [1] 12.7 X 12.7 | 0.65 | 20 | 19.35 |
Ref [31] 15 X 15 | 2.2 | 6.1 | 3.9 |
Ref [32] 15 X 15 | 2.1 | 6.4 | 4.3 |
Proposed structure 13 X 16 | 0.817 | 26.32 | 25.50 |
This research suggests a proposed multilayer structure to reduce simultaneous switching noise (SSN). It offers adequate wide band SSN suppression from 817 MHz to 26.32 GHz and a suppression bandwidth of 25.50 GHz with the special design technique. Compared to reference plane and two-dimensional planar plane, the proposed design minimizes SSN effectively for the wide range of frequencies, also compared with published research.
The proposed structure is verified by dispersion analysis using eigenmode and transmission matrix method, which provides good agreement of specified bandgap. The simulation findings demonstrate strong signal and power integrity. Analysis and HFSS simulations were used to design the suggested structure. The results show that specific branches have more inductance which increase system bandwidth. However, by adding EBG structure onto multilayer PCB, signal integrity issues can be reduced.
The authors declare that they have not used Artificial Intelligence (AI) tools in the creation of this article.
The authors declare that there are no conflicts of interest in this paper.
[1] |
Kim M, Koo K, Shim Y, Hwang C, Pak JS, Ahn S, et al. (2012) Vertical Stepped Impedance EBG (VSI-EBG) Structure for Wideband Suppression of Simultaneous Switching Noise in Multilayer PCBs. IEEE T Electromagn Compatibility 55: 307–314. https://doi.org/10.1109/TEMC.2012.2216883 doi: 10.1109/TEMC.2012.2216883
![]() |
[2] |
Huh SL, Swaminathan M (2012) design technique for embedded electromagnetic band gap structure in load board applications. IEEE T Electromagn Compatibility 54: 443–456. https://doi.org/10.1109/TEMC.2011.2162337 doi: 10.1109/TEMC.2011.2162337
![]() |
[3] |
Shi LF, Jiang HF (2013) Vertical Cascaded Planar EBG Structure for SSN Suppression. Progress In Electromagnetics Research 142: 423–435. https://doi.org/10.2528/PIER13080107 doi: 10.2528/PIER13080107
![]() |
[4] | Zhu HR, Mao JF, Li JJ (2013) Signal and power integrity analysis for the novel power plane of EBG structure in high-speed mixed signal systems. IEEE International Wireless Symposium (IWS), 1-4. https://doi.org/10.1109/IEEE-IWS.2013.6616701 |
[5] |
de Paulis F, Raimondo L, Orlandi (2010) IR-drop analysis and thermal assessment of planar electromagnetic bandgap structures for power integrity applications. IEEE T Adv Packaging 33: 617–622. https://doi.org/10.1109/TADVP.2009.2033572 doi: 10.1109/TADVP.2009.2033572
![]() |
[6] |
Zhu HR, Sun YF, Huang ZX, Wu XL (2019) A Compact EBG Structure with Etching Spiral Slots for Ultra-Wideband Simultaneous Switching Noise Mitigation in Mixed Signal Systems. IEEE T Comp Pack Man 9: 1559–1567. https://doi.org/10.1109/TCPMT.2018.2888512 doi: 10.1109/TCPMT.2018.2888512
![]() |
[7] |
Sievenpiper D, Zhang L, Broas RF, Alexopolous NG, Yablonovitch E (1999) High impedance electromagnetic surfaces with a forbidden frequency band. IEEE T Mircow Theory 47: 2059–2073. https://doi.org/10.1109/22.798001 doi: 10.1109/22.798001
![]() |
[8] |
Kim KH, Schutt-Ainé JE (2008) Analysis and modeling of hybrid planar type electromagnetic-bandgap structures and feasibility study on power distribution network applications. IEEE T Microw Theory 56: 178–186. https://doi.org/10.1109/TMTT.2007.912199 doi: 10.1109/TMTT.2007.912199
![]() |
[9] |
Wu TL, Wang CC, Lin YH, Wang TK, Chang G (2005) A novel power plane with super-wideband elimination of ground bounce noise on high-speed circuits. IEEE Microwave and Wireless Components Letters 15: 174–176. https://doi.org/10.1109/LMWC.2005.844216 doi: 10.1109/LMWC.2005.844216
![]() |
[10] |
Karuppiah V, Gurusamy U (2022) Compact EBG structure for ground bounce noise suppression in high-speed digital systems. AIMS Electronics and Electrical Engineering 6: 124–143. https://doi.org/10.3934/electreng.2022008 doi: 10.3934/electreng.2022008
![]() |
[11] |
Shi LF, Sun ZM, Liu GX, Chen S (2018) Hybrid-Embedded EBG Structure for Ultrawideband Suppression of SSN. IEEE T Electromagn Compatibility 60: 747–753. https://doi.org/10.1109/TEMC.2017.2743039 doi: 10.1109/TEMC.2017.2743039
![]() |
[12] |
Shi LF, Li KJ, Hu HQ, Chen S (2016) Novel L-EBG Embedded structure for the Suppression of SSN. IEEE T Electromagn Compatibility 58: 241–248. https://doi.org/10.1109/TEMC.2015.2505736 doi: 10.1109/TEMC.2015.2505736
![]() |
[13] | Paulis FD, Orlandi A, Raimondo L, Antonini G (2009) Fundamental mechanisms of coupling between planar electromagnetic bandgap structures and interconnects in high-speed digital circuits. Part I – Microstrip lines. Proc. EMC European Workshop, 1-4. https://doi.org/10.1109/EMCEUROPE.2009.5189701 |
[14] |
Shahparnia S, Ramahi OM (2005) Miniaturized electromagnetic bandgap structures for broadband switching noise suppression in PCBs. Electron Lett 41: 519–520. https://doi.org/10.1049/el:20050445 doi: 10.1049/el:20050445
![]() |
[15] |
Bansode MR, Rathod SS (2005) Compact Planar Electromagnetic Bandgap Structure for Signal and Power Integrity Improvement in High-speed Circuits. Progress In Electromagnetics Research M 114: 233–243. http://dx.doi.org/10.2528/PIERM22102205 doi: 10.2528/PIERM22102205
![]() |
[16] |
Fan J, Ye X, Kim J, Archambeault B, Orlandi A (2010) Signal Integrity Design for High-Speed Digital Circuits: Progress and Directions. IEEE T Electromagn Compatibility 52: 392–400. https://doi.org/10.1109/TEMC.2010.2045381 doi: 10.1109/TEMC.2010.2045381
![]() |
[17] |
Wu TL, Fan J, de Paulis F, Wang CD, Scogna AC, Orlandi A (2010) Mitigation of Noise Coupling in Multilayer High-Speed PCB: State of the Art Modeling Methodology and EBG Technology. IEEE T Commun 93: 1678–1689. https://doi.org/10.1587/transcom.E93.B.1678 doi: 10.1587/transcom.E93.B.1678
![]() |
[18] |
Toyota Y, Engin AE, Kim TH, Swaminathan M (2006) Stopband Analysis Using Dispersion Diagram for Two-Dimensional Electromagnetic Bandgap Structures in Printed Circuit Boards. IEEE Microw Wireless Comp Lett 16: 645–647. https://doi.org/10.1109/LMWC.2006.885587 doi: 10.1109/LMWC.2006.885587
![]() |
[19] |
Kim M, Kim S, Bae B, Cho J, Kim J, Kim J, et al. (2013) Application of VSI-EBG Structure to High-Speed Differential Signals for Wideband Suppression of Common Mode Noise. ETRI J 35: 827–637. http://dx.doi.org/10.4218/etrij.13.0112.0875 doi: 10.4218/etrij.13.0112.0875
![]() |
[20] |
Keshwani VR, Bhavarthe PP, Rathod SS (2021) Eight shape Electromagnetic Band Gap structure for bandwidth improvement of wearable antenna. Progress In Electromagnetics Research C 116: 37–49. http://dx.doi.org/10.2528/PIERC21070603 doi: 10.2528/PIERC21070603
![]() |
[21] |
de Paulis F, Orlandi A (2012) Accurate and efficient analysis of planar electromagnetic band-gap structures for power bus noise mitigation in the GHz band. Progress In Electromagnetics Research B 37: 59–80. http://dx.doi.org/10.2528/PIERB11100402 doi: 10.2528/PIERB11100402
![]() |
[22] | de Paulis F, Orlandi A (2006) A novel synthesis method for designing electromagnetic bandgap (EBG) structures in packaged mixed signal systems. Proc 56th Electron Compon Technol Conf 1645–1651. https://doi.org/10.1109/ECTC.2006.1645878 |
[23] | Choi J, Govind V, Swaminathan M (2004) A Novel Electromagnetic Bandgap (EBG) Structure for Mixed-Signal System Applications. IEEE Radio and Wireless Conference 243-246. https://doi.org/10.1109/RAWCON.2004.1389120 |
[24] |
Lu H, Zhao J, Yu Z (2012) Design and Analysis of a Novel Electromagnetic Bandgap Structure For Suppressing Simultaneous Switching Noise. Progress In Electromagnetics Research C 30: 81–91. https://doi.org/10.2528/PIERC12042709 doi: 10.2528/PIERC12042709
![]() |
[25] | Bansode MR, Dahatonde R, Rathod SS (2021) Simultaneous Switching Noise Reduction in High-Speed Circuits. IEEE International Conference on Communication information and Computing Technology (ICCICT) 1–4. https://doi.org/10.1109/ICCICT50803.2021.9510097 |
[26] | High Frequency Structure Simulator. Available from: http://www.ansoft.com. |
[27] | Pozar M (2013) Microwave Engineering, 3rd ed. New York, NY, USA: Wiley. |
[28] | Orlandi A, Archambeault B, de Paulis F, Connor S (2017) Impact of Planar EBGs on Signal Integrity in High-Speed Digital Boards. https://doi.org/10.1002/9781119281559.ch3 |
[29] | The MathWorks, Inc. (2022) MATLAB version: 9.13.0 (R2022b). Accessed: January 01, 2023. Available from: https://www.mathworks.com. |
[30] | Bansode MR, Rathod SS (2023) Enhanced Signal and Power Integrity using Novel Planar EBG design. 2023 Joint Asia-Pacific International Symposium on Electromagnetic Compatibility and International Conference on Electro Magnetic Interference Compatibility (APEMC/INCEMIC). https://doi.org/10.1109/APEMC57782.2023.10217404 |
[31] |
Kim M, Ahn S (2013) A Compact and Multi-Stack Electromagnetic Bandgap Structure for Gigahertz Noise Suppression in Multilayer Printed Circuit Boards. Appl Sci 7: 804. https://doi.org/10.3390/app7080804 doi: 10.3390/app7080804
![]() |
[32] | Kim M (2016) Multi-stack Technique for a Compact and Wideband EBG Structure in High-Speed Multilayer Printed Circuit Boards. ETRI Journals 38: 903-910. |
Eye Measurement and Parameters | Reference structure | 2-D planar structure | Proposed Multilayer EBG |
In terms of amplitude -Eye Height | 1.03 V | 1.44 V | 1.94 V |
In terms of amplitude -Vertical Opening | 1.03 V | 1.44 V | 1.94 V |
In terms of amplitude-SNR | 3.84 | 6.09 | 26.99 |
In terms of time-Eye Width | 4.53 ns | 8.31 ns | 19.61 ns |
In terms of time-Horizontal Opening | 5.73 ns | 9.09 ns | 19.61 ns |
EBG Structure | Suppression Depth = -30dB | Suppression Depth = -30dB | Suppression Depth = -30dB |
Ref. and Patch size in mm | Lower frequency in GHz | Higher frequency in GHz | Bandwidth in GHz |
Ref [1] 12.7 X 12.7 | 0.65 | 20 | 19.35 |
Ref [31] 15 X 15 | 2.2 | 6.1 | 3.9 |
Ref [32] 15 X 15 | 2.1 | 6.4 | 4.3 |
Proposed structure 13 X 16 | 0.817 | 26.32 | 25.50 |
Eye Measurement and Parameters | Reference structure | 2-D planar structure | Proposed Multilayer EBG |
In terms of amplitude -Eye Height | 1.03 V | 1.44 V | 1.94 V |
In terms of amplitude -Vertical Opening | 1.03 V | 1.44 V | 1.94 V |
In terms of amplitude-SNR | 3.84 | 6.09 | 26.99 |
In terms of time-Eye Width | 4.53 ns | 8.31 ns | 19.61 ns |
In terms of time-Horizontal Opening | 5.73 ns | 9.09 ns | 19.61 ns |
EBG Structure | Suppression Depth = -30dB | Suppression Depth = -30dB | Suppression Depth = -30dB |
Ref. and Patch size in mm | Lower frequency in GHz | Higher frequency in GHz | Bandwidth in GHz |
Ref [1] 12.7 X 12.7 | 0.65 | 20 | 19.35 |
Ref [31] 15 X 15 | 2.2 | 6.1 | 3.9 |
Ref [32] 15 X 15 | 2.1 | 6.4 | 4.3 |
Proposed structure 13 X 16 | 0.817 | 26.32 | 25.50 |