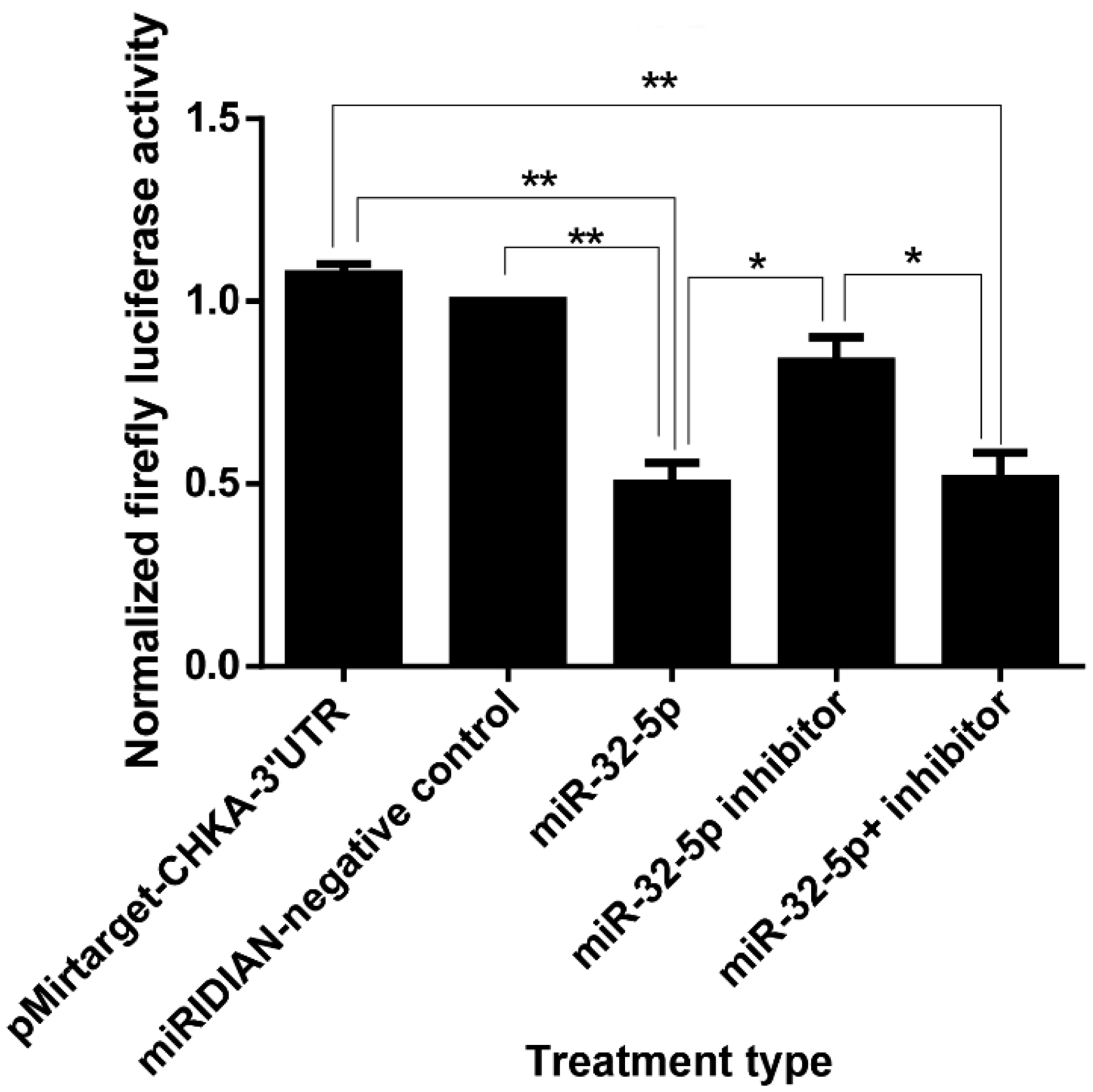
Cervical cancer remains a significant global health challenge, especially in low- and middle-income countries. The elucidation of the molecular pathways underlying the carcinogenesis of cervical cancer is vital to develop targeted therapies. Our study investigates the regulatory effects of miR-32-5p on the choline kinase alpha (chka) gene in HeLa cells, a well-established cervical cancer cell line. Choline kinase alpha is recognized for its role in phosphatidylcholine biosynthesis, which is crucial for cell membrane formation, and is implicated in the oncogenic transformation of cells. Utilizing a combination of in silico prediction, luciferase assays, RT-qPCR, and Western blot analyses, we demonstrated that miR-32-5p directly targets the 3′ untranslated region (3′UTR) of chka mRNA, leading to a significant downregulation of chka expression. Our results demonstrate that miR-32-5p significantly downregulates chka at both the mRNA and protein levels, thus leading to decreased cellular proliferation and increased apoptosis. This was further confirmed by a cell cycle analysis, which showed a notable arrest in the G0/G1 phase. Additionally, scratch assays indicated a reduced migratory capacity in miR-32-5p-transfected cells, suggesting the potential anti-metastatic properties of miR-32-5p. These findings highlight the therapeutic potential of miR-32-5p as a biomarker and a target in cervical cancer treatment strategies. By modulating chka expression, miR-32-5p could serve as a novel approach to curb the progression and spread of cervical cancer, thus offering a promising avenue for future research and clinical applications. This study contributes to the growing understanding of miRNA-mediated gene regulation in cancer biology and underscores the importance of targeted genetic research in the development of cancer therapeutics.
Citation: Sweta Raikundalia, Ling Ling Few, Siti Asma' Hassan, Get Bee Yvonne-Τee, Wei Cun See Too. Choline kinase and miR-32-5p: A crucial interaction promoting apoptosis and delaying wound repair in cervical cancer cells[J]. AIMS Biophysics, 2024, 11(3): 281-295. doi: 10.3934/biophy.2024016
[1] | Angel Rivera-Calzada, Andrés López-Perrote, Roberto Melero, Jasminka Boskovic, Hugo Muñoz-Hernández, Fabrizio Martino, Oscar Llorca . Structure and Assembly of the PI3K-like Protein Kinases (PIKKs) Revealed by Electron Microscopy. AIMS Biophysics, 2015, 2(2): 36-57. doi: 10.3934/biophy.2015.2.36 |
[2] | Emmanuel Reyes-Uribe, Nathalia Serna-Marquez, Eduardo Perez Salazar . DDRs: receptors that mediate adhesion, migration and invasion in breast cancer cells. AIMS Biophysics, 2015, 2(3): 303-317. doi: 10.3934/biophy.2015.3.303 |
[3] | Oleg A. Karpov, Gareth W. Fearnley, Gina A. Smith, Jayakanth Kankanala, Michael J. McPherson, Darren C. Tomlinson, Michael A. Harrison, Sreenivasan Ponnambalam . Receptor tyrosine kinase structure and function in health and disease. AIMS Biophysics, 2015, 2(4): 476-502. doi: 10.3934/biophy.2015.4.476 |
[4] | Michael J. Hamilton, Matthew D. Young, Silvia Sauer, Ernest Martinez . The interplay of long non-coding RNAs and MYC in cancer. AIMS Biophysics, 2015, 2(4): 794-809. doi: 10.3934/biophy.2015.4.794 |
[5] | Mikayel Ginovyan, Svetlana Hovhannisyan, Hayarpi Javrushyan, Gohar Sevoyan, Zaruhi Karabekian, Narine Zakaryan, Naira Sahakyan, Nikolay Avtandilyan . Screening revealed the strong cytotoxic activity of Alchemilla smirnovii and Hypericum alpestre ethanol extracts on different cancer cell lines. AIMS Biophysics, 2023, 10(1): 12-22. doi: 10.3934/biophy.2023002 |
[6] | Qiao Yi Chen, Max Costa, Hong Sun . Structure and function of histone acetyltransferase MOF. AIMS Biophysics, 2015, 2(4): 555-569. doi: 10.3934/biophy.2015.4.555 |
[7] | Mikayel Ginovyan, Agnieszka Bartoszek, Izabela Koss-Mikołajczyk, Barbara Kusznierewicz, Pierre Andreoletti, Mustapha Cherkaoui-Malki, Naira Sahakyan . Growth inhibition of cultured cancer cells by Ribes nigrum leaf extract. AIMS Biophysics, 2022, 9(3): 282-293. doi: 10.3934/biophy.2022024 |
[8] | Derrick Lonsdale, Chandler Marrs . The potential of lipid soluble thiamine in the treatment of cancer. AIMS Biophysics, 2020, 7(1): 17-26. doi: 10.3934/biophy.2020002 |
[9] | Audrey Lejart, Gilles Salbert, Sébastien Huet . Cytosine hydroxymethylation by TET enzymes: From the control of gene expression to the regulation of DNA repair mechanisms, and back. AIMS Biophysics, 2018, 5(3): 182-193. doi: 10.3934/biophy.2018.3.182 |
[10] | Larisa I. Fedoreyeva, Tatiana A. Dilovarova, Boris F. Vanyushin, Inna A. Chaban, Neonila V. Kononenko . Regulation of gene expression in Nicotiana tabacum seedlings by the MKASAA peptide through DNA methylation via the RdDM pathway. AIMS Biophysics, 2022, 9(2): 113-129. doi: 10.3934/biophy.2022011 |
Cervical cancer remains a significant global health challenge, especially in low- and middle-income countries. The elucidation of the molecular pathways underlying the carcinogenesis of cervical cancer is vital to develop targeted therapies. Our study investigates the regulatory effects of miR-32-5p on the choline kinase alpha (chka) gene in HeLa cells, a well-established cervical cancer cell line. Choline kinase alpha is recognized for its role in phosphatidylcholine biosynthesis, which is crucial for cell membrane formation, and is implicated in the oncogenic transformation of cells. Utilizing a combination of in silico prediction, luciferase assays, RT-qPCR, and Western blot analyses, we demonstrated that miR-32-5p directly targets the 3′ untranslated region (3′UTR) of chka mRNA, leading to a significant downregulation of chka expression. Our results demonstrate that miR-32-5p significantly downregulates chka at both the mRNA and protein levels, thus leading to decreased cellular proliferation and increased apoptosis. This was further confirmed by a cell cycle analysis, which showed a notable arrest in the G0/G1 phase. Additionally, scratch assays indicated a reduced migratory capacity in miR-32-5p-transfected cells, suggesting the potential anti-metastatic properties of miR-32-5p. These findings highlight the therapeutic potential of miR-32-5p as a biomarker and a target in cervical cancer treatment strategies. By modulating chka expression, miR-32-5p could serve as a novel approach to curb the progression and spread of cervical cancer, thus offering a promising avenue for future research and clinical applications. This study contributes to the growing understanding of miRNA-mediated gene regulation in cancer biology and underscores the importance of targeted genetic research in the development of cancer therapeutics.
Cancer remains a leading cause of death globally, significantly impeding efforts to enhance life expectancy across various nations. The World Health Organization's 2019 data emphasizes the broad impact of this disease, positioning it as a primary cause of mortality before the age of 70 in over half of the countries worldwide [1]. Notably, transitioning nations experience notably higher death rates from female breast and cervical cancers compared to their more developed counterparts, thus underscoring significant health disparities. Projections indicate a 47% surge in the global cancer burden by 2040, which is primarily attributed to demographic changes and is potentially magnified by globalization and economic development [2]. This alarming trend underscores the urgent need for focused research and efficacious interventions, particularly for cervical cancer, which exhibits significant incidence and mortality rates, especially in less developed regions. It is the fourth most prevalent cancer and the fourth leading cause of cancer deaths among women globally, thus imposing a heavy toll on countries in sub-Saharan Africa, Melanesia, South America, and Southeast Asia [2].
The escalating incidence and mortality rates of cervical cancer underscore the necessity to delve into its molecular basis to further develop more effective therapeutic strategies. Advances in genetic research have identified numerous oncogenes implicated in the cancer's pathogenesis, including choline kinase alpha gene (chka) [3]. Recognized as a potent oncogene, chka plays a vital role in the biosynthesis of phosphocholine (PCho), a key precursor in phospholipid production, which is essential for cell membrane synthesis and proliferation. Its aberrant overexpression is associated with the transformation of non-cancerous cells to cancerous phenotypes, signifying its crucial role in tumorigenesis [4],[5]. Targeting chka through small interfering RNA (siRNA) knockdown has shown promising results, inducing apoptosis in HeLa cells, a type of cervical cancerous cell line, highlighting its therapeutic potential against cervical cancer [6]. The significance of chka in cervical cancer's development and progression positions it as a prime target for research, aiming to unveil targeted anti-cancer strategies that could mitigate the global health challenge posed by this malignancy.
Moreover, the identification of CHKA and phosphocholine as critical biomarkers for cancer diagnoses and treatment efficacy evaluations marks a significant advancement in oncology. The heightened expression of CHKA across various cancer types has pivoted the development of CHKA inhibitors [7],[8], showcasing a novel approach for cancer therapies. Despite these advancements, the regulation of CHKA gene expression, especially through microRNAs (miRNAs), has been relatively underexplored. MiRNAs, which are small non-coding RNAs integral to gene regulation, have been implicated in numerous biological processes and cancer types. Their aberrant expression patterns offer a promising avenue for cancer diagnoses and prognoses, with several miRNAs currently undergoing clinical trials for their potential therapeutic applications [9],[10].
Our research investigates the regulation of choline kinase alpha (chka) gene expression by human miR-32-5p, which is a microRNA cataloged in MiRBase with the accession number MIMAT0000090. This microRNA is derived from the precursor stem-loop structure pre-miR-32, which itself is transcribed from a gene registered under the NCBI accession number NR_029506. The gene is positioned on the long arm of chromosome 9 at the 31.3 locus. The findings from this study not only underscore miR-32-5p's regulatory impact on cancer cell behavior, but also illuminate the complex interplay between miRNAs and oncogene expression, thus offering new insights into potential therapeutic strategies for cervical cancer. This work contributes to the growing body of research which seeks to harness the power of molecular biology in the fight against cancer, highlighting the critical role of targeted genetic research in developing innovative treatments for diseases such as cervical cancer, which continues to challenge global health.
Predictions of microRNAs targeting the 3′ untranslated region of CHKA mRNA were generated using the CHKA gene as the search term in TargetScan, DIANA Tools microT-CDS, microRNA.org, and miRDB, all employing their default settings.
The HeLa cervical cancer cell line (ATCC®CCL2™) was of the American Type Culture Collection and grown in Dulbecco's Modified Eagle's Medium from Thermo Fisher Scientific, Inc., MA, USA. This medium was supplemented with 10% heat-inactivated fetal bovine serum (Gibco; Thermo Fisher Scientific, Inc.), 100 U/ml penicillin, and 100 mg/ml streptomycin (Sigma-Aldrich; Merck KGaA, Darmstadt, Germany). Cultivation occurred at a steady 37 °C in an atmosphere of 5% CO2. For miRNA research, both the miRIDIAN microRNA mimic Housekeeping Positive Control #2 (targeting GAPDH) and the miRIDIAN microRNA mimic Negative Control #1 were procured from GE Healthcare Dharmacon, Inc., CO, USA. Additionally, the hsa-miR-32-5p miRNA mimic, with the sequence 5′-UAUUGCACAUUACUAAGUUGCA-3′, and its specific inhibitor were sourced from Applied Biological Materials in Richmond, Canada. These RNA tools were mixed in 1X siRNA buffer from GE Healthcare Dharmacon, Inc. Furthermore, a firefly luciferase reporter plasmid which contained the 3′-UTR of the chka gene (pMirTarget-chka−3′-UTR) was secured from OriGene Technologies, Inc., MD, USA.
To investigate miRNA regulation, HeLa cancer cells were prepared for transient transfection using Lipofectamine 3000 (Invitrogen; Thermo Fisher Scientific, Inc., MA, USA). The transfection involved a human miR-32-5p mimic, its inhibitor, and a pMirTarget-chka−3′-UTR luciferase reporter. This was conducted in 96-well plates for target validation and 24-well plates for a more extensive analysis. The cells were seeded to reach 70–80% confluency about 16–18 hours before the transfection process.
The transfection mixtures were formulated by diluting the plasmid and miRNA elements to concentrations of 200 ng and 25 nM, respectively, in Opti-MEM™ (Thermo Fisher Scientific, Inc., MA, USA). Then, these were equally mixed with Opti-MEM™ pre-blended with Lipofectamine 3000. After a 20-minute incubation at room temperature, the resulting complexes were ready for use in the transfection process.
After transfecting HeLa cells with the pMirTarget-chka-3′-UTR plasmid that contained the 3′-UTR of the chka gene, and either the miR-32-5p mimic (sequence 5′-UAUUGCACAUUACUAAGUUGCA-3′) or its specific inhibitor (Applied Biological Materials, Richmond, Canada), along with the microRNA Negative Control #1 from GE Healthcare Dharmacon, CO, USA, the following assay protocol was applied: 25 µl of medium was removed from each well of a 96-well plate, replaced with 75 µl of the Dual-Glo luciferase reagent from Promega, WI, USA, and incubated for 10 minutes at room temperature. Then, the firefly luciferase activity was measured in relative light units using a GloMax 20/20 luminometer (Promega, WI, USA). These measurements were conducted in triplicate and the entire procedure was repeated at least twice. Luminescence data from the miR-32-5p mimic and inhibitor were normalized against the baseline from the miRNA Negative Control.
The total RNA was extracted from HeLa cells post-transfection using various agents including the miR-32-5p mimic, its specific inhibitor, their combination, the miRIDIAN negative control, and the miR-GAPDH positive control. This was performed using the Total RNA Isolation Kit (Thermo Fisher Scientific, Inc., MA, USA) following the manufacturer's instructions. Genomic DNA was removed using the RNase-Free DNase Set (Qiagen, Hilden, Germany). RNA concentrations were measured with a NanoDrop ND-1000 spectrophotometer (Thermo Fisher Scientific, Inc., MA, USA). For cDNA synthesis, 1 µg of RNA was reverse transcribed at 42 °C for 1 hour using the RevertAid™ H Minus First Strand cDNA Synthesis Kit in a 20 µl reaction volume.
A quantitative PCR (qPCR) was performed on the ABI Prism 7500 Sequence Detection System using 13 µl reaction volumes which included 6.25 µl of Power SYBR™ Green PCR Master Mix, 0.5 µl of both forward and reverse primers, 1 µl of cDNA, and 4.75 µl of double-distilled water. The PCR cycle consisted of an initial denaturation at 95 °C for 10 minutes, followed by 40 cycles of 95 °C for 10 seconds, and 60 °C for 1 minute. Primers targeted genes including YWHAZ, RPS18, GAPDH, and CHKA. The gene expression levels were normalized against the geometric mean of the Cq values for YWHAZ and RPS18 and calculated using the 2−ΔΔCq method.
Cell lysates were prepared from HeLa cells that were transfected with miRNA for 72 hours using the ProteoJET™ Mammalian Cell Lysis Reagent from Thermo Fisher Scientific, Inc., MA, USA, following the manufacturer's instructions. Protein concentrations of the lysates were determined using the Bradford assay (Bio-Rad, CA, USA). For electrophoresis, 30 µg of the protein samples were loaded onto a 12% SDS-PAGE gel. Then, the proteins were transferred from the gel to a nitrocellulose membrane for a further analysis.
The membrane was blocked with 5% (w/v) non-fat milk in a TBS buffer at room temperature for one hour. Subsequently, the membranes were incubated at 4 °C using primary antibodies against β-actin (1: 5000 dilution; Abcam, Cambridge, UK) and CHKA (1:1000 dilution; Abcam, Cambridge, UK). Then, the membrane was washed at least three times with TBST (0.1% (v/v) Tween-20 in TBS) before a one-hour room temperature incubation with an HRP-conjugated goat anti-rabbit IgG secondary antibody (1: 5,000 dilution; Sigma-Aldrich, Merck KGaA, Darmstadt, Germany). After three additional washes, protein detection was carried out using the SuperSignal™ West Femto Maximum Sensitivity Substrate (Thermo Fisher Scientific, Inc., MA, USA). The protein bands were visualized using the FUSION FX chemiluminescence imaging system (Vilber Lourmat), and the band intensities were quantitatively analyzed using the ImageJ software, version 1.49b (https://imagej.net/ij/).
The Muse™ Annexin V & Dead Cell Assay Kit (Merck Millipore, C) and the Muse™ Cell Analyzer (EMD Millipore) were used to evaluate and quantify apoptosis in the transfected HeLa cells, in accordance with the manufacturer's instructions. Following transfection, the cells were trypsinized, washed with PBS, and resuspended in DMEM enriched with 10% FBS (Gibco; Thermo Fisher Scientific, Inc., MA, USA) to a minimum concentration of 1×106 cells/ml. Then, 100 µl of this cell suspension was mixed with 100 µl of the Muse™ Annexin V & Dead Cell reagent, briefly vortexed, and incubated for 30 minutes at room temperature in the dark, before the stained cells were counted by the Muse™ Cell Analyzer.
The Muse® Cell Cycle Kit (Merck Millipore, Darmstadt, Germany) and the Muse™ Cell Analyzer (EMD Millipore) were used to assess the cell cycle distribution (G0/G1, S, and G2/M phases) of the transfected HeLa cells, following the manufacturer's protocols. The cells were trypsinized after transfection and washed with 1X PBS, before being resuspended in DMEM enriched with 10% FBS (Gibco; Thermo Fisher Scientific, Inc., MA, USA) to achieve a minimal density of 1×106 cells/ml. The cells were then fixed in ice-cold 70% ethanol and stored at −20°C according to the kit's guidelines. For analysis, 5 × 105 fixed cells were washed with PBS, stained with 200 µl of Muse™ Cell Cycle Reagent, briefly vortexed, and incubated for 30 minutes at room temperature in the dark. Then, the Muse™ Cell Analyzer was used to count the stained cells.
To create a scratch wound, a consistent wound was carefully made across the nearly confluent HeLa cell monolayers in 6-well plates using a P20 pipette tip. Following the scratch, the medium and any dislodged cells were removed, and the cells were then rinsed twice with 1X PBS. Each well was subsequently replenished with 2 ml of fresh serum-free medium. Images of the wound were captured at specified intervals using a DINO EYE eyepiece camera (AnMo Electronics Corporation, New Taipei City, Taiwan). The width of the gap between the wound edges was measured using the ImageJ software, version 1.49b (https://imagej.net/ij/).
All experiments were carried out in triplicates. The data were analyzed using either a Student's t-test or a one-way analysis of variance (ANOVA), followed by post hoc comparisons via Tukey's Honestly Significant Difference (HSD) test. A p-value of less than 0.05 was considered to indicate statistical significance. All statistical analyses were performed using the SPSS software, version 22.0. The results are presented as the mean ± standard error of the mean (SEM) based on three independent experiments.
In silico analyses utilizing prediction tools such as TargetScan, DIANA Tools microT-CDS, microRNA.org, and miRDB have demonstrated that miR-32-5p binds to the 3′ untranslated region (3′UTR) of chka mRNA. This interaction between miR-32-5p and chka was further confirmed through a firefly luciferase assay, which provided an empirical validation of the computational predictions.
Normalized firefly luciferase activity was utilized to evaluate the binding efficiency of miR-32-5p to the 3′ untranslated region (3′UTR) of the chka gene within the fusion construct (pMirTarget-chka-3′UTR). Our findings revealed a substantial 50% reduction in the luciferase activity following co-transfection with miR-32-5p as compared to the negative control (Figure 1). This significant decrease indicates that miR-32-5p directly suppresses chka expression. Additionally, transfection with miR-32-5p inhibitor shows a reduction in the luciferase activity compared to the control, though the difference was not significant. When cells were transfected with both miR-32-5p and its inhibitor, the luciferase activity slightly recovered compared to cells solely treated with miR-32-5p. This indicates that the inhibitor was partially effective at blocking the action of miR-32-5p, thus leading to a decrease in its suppressive effect on CHKA expression.
The mimic of miR-32-5p was subsequently transfected into HeLa cells to assess the potential of this miRNA to downregulate the expression of the chka gene.
In HeLa cells, the introduction of miR-32-5p was shown to significantly reduce chka expression by 0.64-fold, thus underscoring the suppressive role on the gene (Figure 2A). However, this downregulation was partially reversed when a miR-32-5p-specific inhibitor was applied, this decreasing chka expression by only 0.27-fold. A more nuanced regulatory effect was observed when miR-32-5p and its specific inhibitor were used together, thus leading to a 0.58-fold reduction in chka at the mRNA level. This combined approach not only mitigated the suppressive effect of miR-32-5p, but also highlighted the complex interplay in regulating gene expression, thus bringing it closer to the baseline levels.
These findings provide compelling evidence of the direct inhibitory action of miR-32-5p on the expression of its target gene. Additionally, the observed effect of the miR-32-5p inhibitor underscores the specificity and potential reversibility of miRNA-mediated gene regulation. This study contributes to our understanding of miRNA function in gene expression regulation, thus offering insights that could guide the development of therapeutic strategies that target miRNA pathways in disease contexts.
In HeLa cells, transfection with miR-32-5p not only reduced chka mRNA expression, but also led to a significant decrease in the CHKA protein levels, as evidenced by the Western blot analysis. Compared to the negative control, CHKA protein expression in cells transfected with miR-32-5p was markedly lower and quantitatively measured at 0.45-fold of the control levels (Figure 2B). These findings are consistent with the regulatory role of miR-32-5p, thus indicating its efficacy in modulating gene expression through targeting and downregulating the chka mRNA transcript. This regulatory effect underscores miR-32-5p's potential in influencing cellular processes by adjusting the expression of specific genes, as reflected in the scaled comparison with the negative control set at 1.0, thus highlighting the miRNA's significant impact on CHKA protein expression.
The effect of the miR-32-5p mimic on cancer cellular apoptosis was investigated in the present study. Based on the results, statistically significant differences were observed in the percentages of live cells and total apoptotic cells between the cells transfected with the negative control miRNA and the miR-32-5p-transfected cells. These results suggested that miR-32-5p induced cell death.
Moreover, the current study evaluated the apoptotic effect of miR-32-5p on HeLa cells using Annexin V staining to distinguish between live, early apoptotic, and late apoptotic/dead cells. The apoptosis profile was meticulously quantified across different treatments: untransfected HeLa cells, miRIDIAN-negative control, and cells treated with miR-32-5p.
Our findings revealed a significant modulation of apoptosis in HeLa cells upon treatment with miR-32-5p. Specifically, the percentage of live cells markedly decreased from 73.64% in the untransfected HeLa cells to 44.65% upon miR-32-5p treatment, thus indicating a potent induction of apoptosis by miR-32-5p. Conversely, the miRIDIAN-negative control exhibited a viability profile similar to that of the untransfected cells, thus underscoring the specificity of miR-32-5p's apoptotic effect (Figure 3).
Moreover, the proportion of cells in early apoptosis slightly increased with the miR-32-5p treatment compared to both the untransfected and the negative control cells. However, a more pronounced effect was observed in the late apoptotic/dead cell population, which surged from 0.08% in the untransfected cells to 7.72% in the cells treated with miR-32-5p, further corroborating the pro-apoptotic role of this microRNA.
These results underscore the critical role of miR-32-5p in regulating cell death pathways in HeLa cells. The ability of miR-32-5p to significantly increase the apoptotic cell population highlights its potential as a therapeutic agent to induce apoptosis in cancer cells. Future studies should focus on unraveling the molecular mechanisms which underlie miR-32-5p-mediated apoptosis, which could pave the way for novel cancer treatments.
Additionally, the study examined the impact of miR-32-5p on the cell cycle distribution of HeLa cells, and particularly focused on transitions through the G0/G1, S, and G2/M phases. The present findings demonstrated that transfection of HeLa cells with miR-32-5p not only suppressed CHKA expression, but also affected their cell cycle distribution (Figure 4). There appeared to be an increase in the percentage of HeLa cells in the G0/G1 phase following transfection with miR-32-5p compared to the untransfected HeLa cells, the miRIDIAN-negative control, and miR-GAPDH. This indicates that miR-32-5p transfection may either induce cell cycle arrest or delay in the G0/G1 phase.
The percentage of cells in the S phase (synthesis phase, where DNA replication occurs) seemed to decrease following miR-32-5p transfection. This reduction suggests that miR-32-5p may interfere with the progression of cells from the G1 phase to the S phase, further supporting the notion of either cell cycle arrest or delay at the G0/G1 phase.
Similar to the S phase, there was a decrease in the percentage of cells in the G2/M phase (where cells prepare for mitosis) following miR-32-5p transfection. This decrease complements the findings for the G0/G1 and S phases, and reinforces the impact of miR-32-5p on cell cycle progression, particularly in either delaying or arresting the cell cycle before cell division.
In conclusion, the transfection of HeLa cells with miR-32-5p has a noticeable impact on the cell cycle, primarily characterized by an increase in cells at the G0/G1 phase and a corresponding decrease in the S and G2/M phases. These effects suggest that miR-32-5p may play a role in cell cycle regulation, potentially by either inducing cell cycle arrest or delaying progression through the cell cycle, particularly at the G1 to S transition.
The current study explores the effect of miR-32-5p on the wound healing capacity of HeLa cells, which is a crucial aspect of cellular behavior that has implications for understanding cancer progression and metastasis. The assay quantitatively assessed the % wound healing in three different conditions: untransfected HeLa cells, cells transfected with miRIDIAN-negative control, and cells transfected with miR-32-5p.
Our results indicated that HeLa cells transfected with miR-32-5p displayed a markedly diminished ability to heal wounds compared to both untransfected cells and those treated with the miRIDIAN-negative control (Figure 5). Specifically, miR-32-5p-transfected cells achieved an approximately 35% scratch closure, while untreated and negative control cells showed complete wound healing within 72 hours. This pronounced decrease underscores the potential of miR-32-5p to inhibit the wound healing process, thus proposing a novel regulatory pathway through which miR-32-5p may impact cancer cell metastasis.
These findings contribute to the growing body of literature on the functional roles of microRNAs in cancer biology. Specifically, the observed decrease in wound healing in miR-32-5p transfected HeLa cells underscores the potential of miR-32-5p as a target for therapeutic strategies aimed at limiting cancer cell migration and metastasis. Further investigation into the molecular pathways mediated by miR-32-5p could provide deeper insights into its role in cancer progression and offer new avenues for the development of anti-metastatic therapies.
The exploration of choline kinase (CHK) and its isoforms illuminates a significant aspect of cancer biology, focusing on its fundamental role in the biosynthesis of phosphatidylcholine (PC), which is a key phospholipid in eukaryotic cell membranes [11],[12]. CHK's activity in phosphorylating choline to phosphocholine underscores the critical early steps in the CDP-choline pathway, and is differentiated by the isoforms CHKA and CHKB, which are produced from chka and chkb genes, respectively.
Increased levels of expression and activity of CHKA have been linked to the progression and heightened aggressiveness of various cancers, such as breast, lung, and colorectal cancers, among others [4],[13]. CHKA's pivotal role in promoting cancer cell aggressiveness and resistance to treatment highlights its significance in the survival, proliferation, and activation of critical Ras signaling pathways, including MAPK and PI3K/AKT [5]. These pathways are integral to a wide range of essential cellular functions, encompassing cell growth and survival, differentiation, apoptosis, cytoskeletal dynamics, and protein trafficking and secretion. CHKA is essential to generate phosphatidic acid, which is crucial for the activation of several Ras signaling pathways, thereby underlining the importance of CHKA's activity in cancer biology [14]. As such, the expression levels of CHKA are increasingly recognized as valuable biomarkers for cancer detection and to assess prognostic outcomes, thereby providing insights into the disease's progression and potential therapeutic targets [11],[15].
In the quest for effective cancer therapies, CHKA inhibitors have emerged as promising agents, with the initial inhibitors demonstrating potent antitumor activities. The first CHKA inhibitor was a dicationic choline-mimetic hemicholinium-3 (HC-3) that was able to inhibit growth factor-induced DNA synthesis in vitro [16]. Inspired by the structure of hemicholinium-3 (HC-3), the development of subsequent inhibitors [7],[8],[17] marks a significant advance in targeting CHKA's oncogenic function. Furthermore, the application of RNA interference (RNAi) techniques for the downregulation of CHKA alpha expression presents an alternative therapeutic strategy, showcasing the potential to induce apoptosis and inhibit cancer cell proliferation and migration without harming normal cells [18],[19]. A study by Falcon et. al [6] showed that an siRNA knockdown of the CHKA protein in HeLa cells resulted in significant cell death through apoptosis.
Our study extends this investigative frontier by examining the regulatory effects of miR-32-5p on chka gene, thus proposing a sophisticated layer of gene expression control mediated by microRNA (miRNA) interactions. Our findings suggest that miR-32-5p targets the 3′ untranslated region of chka, thus offering a novel mechanism to post-transcriptionally suppress chka expression. The introduction of miR-32-5p into cancer cells notably decreased chka expression, thus leading to enhanced apoptosis and reduced wound healing. This underscores the significant therapeutic potential of targeting miR-32-5p in cancers characterized by aberrant CHKA expression, particularly considering CHKA's necessity to activate crucial cell survival and proliferation pathways [5].
However, the function of miR-32-5p in cancer biology is complex and varies depending on the context, thereby acting as an oncogene in some cancers while serving as a tumor suppressor in others. For instance, in oral squamous cell carcinoma, miR-32-5p enhances cell proliferation, migration, invasion, and the transition from epithelial to mesenchymal states, thus underscoring its role in cancer progression [20]. Likewise, it is significantly overexpressed in ovarian cancer, where it boosts cancer cell proliferation and movement [21]. Conversely, in non-small cell lung cancer, miR-32-5p takes on a protective role by curbing migration and invasion. It achieves this by targeting SMAD3, which is a key player in the transforming growth factor-beta signaling pathway that transmits signals from the cell surface to the nucleus, thereby affecting gene activity and cell growth [22]. Furthermore, in cervical cancer, miR-32-5p deters cancerous behaviors by influencing HOXB8, which is a gene regulator crucial for development [23]. This dual nature of miR-32-5p underscores the intricate roles microRNAs play in oncology, thus emphasizing the need for a more profound comprehension of their actions to fine-tune miRNA-based therapeutic approaches.
In conclusion, the oncogenic role of the chka gene and the regulatory potential of miRNAs such as miR-32-5p revealed intricate pathways for potential cancer therapies. By leveraging the detailed interplay between gene expression regulation and oncogenic signaling, our study contributed to the broader effort to develop more effective and targeted treatments for cancer. This research not only highlighted the therapeutic implications of inhibiting CHKA, but also opened avenues to utilize miRNAs as innovative tools in cancer therapy, thus promising advancements in the fight against cancer.
Building on these insights, we recommend the further exploration of miR-32-5p as a potential therapeutic agent for cervical cancer. Its proven effectiveness in downregulating chka expression, which leads to decreased cell proliferation and increased apoptosis, along with its potential anti-metastatic properties, makes miR-32-5p a promising biomarker and therapeutic target. Future research should focus on conducting animal studies and clinical trials to evaluate the efficacy and safety of miR-32-5p-based therapies, with the goal of developing a novel and robust approach to curb the progression and spread of cervical cancer in affected populations.
During the preparation of this work, the authors used ChatGPT in order to improve readability and language. After using this tool, the authors reviewed and edited the content as needed and take full responsibility for the content of the publication.
This work was supported by the Malaysia Ministry of Higher Education Fundamental Research Grant Scheme (FRGS), [FRGS/1/2021/SKK0/USM/02/34 (203/PPSK/6171299)]. We thank the laboratory staff of the School of Health Sciences, Universiti Sains Malaysia (USM) for their technical assistance. We would like to acknowledge the technical support provided by the Central Research Laboratory at the School of Medical Sciences, USM, and the Institute for Research in Molecular Medicine (INFORMM), Health Campus, USM.
[1] | World Health Organization Global Health Estimates 2020: Deaths by Cause, Age, sex, by Country and by Region, 2000−2019, 2023. Available from: https://www.who.int/data/gho/data/themes/mortality-and-global-health-estimates/ghe-leading-causes-of-death |
[2] |
Sung H, Ferlay J, Siegel RL, et al. (2021) Global cancer statistics 2020: GLOBOCAN estimates of incidence and mortality worldwide for 36 cancers in 185 countries. CA Cancer J Clin 71: 209-249. https://doi.org/10.3322/caac.21660 ![]() |
[3] |
Ramírez de Molina A, Gallego-Ortega D, Sarmentero J, et al. (2005) Choline kinase is a novel oncogene that potentiates RhoA-induced carcinogenesis. Cancer Res 65: 5647-5653. https://doi.org/10.1158/0008-5472.CAN-04-4416 ![]() |
[4] |
Lacal Sanjuán JC, Zimmerman T, Campos Rosa JM (2021) Choline kjinase: An unexpected journey for a precision medicine strategy in human diseases. Pharmaceutics 13: 788. https://doi.org/10.3390/pharmaceutics13060788 ![]() |
[5] |
Rizzo A, Satta A, Garrone G, et al. (2021) Choline kinase alpha impairment overcomes TRAIL resistance in ovarian cancer cells. J Exp Clin Cancer Res 40: 5. https://doi.org/10.1186/s13046-020-01794-6 ![]() |
[6] |
Falcon SC, Hudson CS, Huang Y, et al. (2013) A non-catalytic role of choline kinase alpha is important in promoting cancer cell survival. Oncogenesis 2: e38. https://doi.org/10.1038/oncsis.2013.2 ![]() |
[7] |
Trousil S, Kaliszczak M, Schug Z, et al. (2016) The novel choline kinase inhibitor ICL-CCIC-0019 reprograms cellular metabolism and inhibits cancer cell growth. Oncotarget 7: 37103-37120. https://doi.org/10.18632/oncotarget.9466 ![]() |
[8] |
Sola-Leyva A, López-Cara LC, Ríos-Marco P, et al. (2019) Choline kinase inhibitors EB-3D and EB-3P interferes with lipid homeostasis in HepG2 cells. Sci Rep 9: 5109. https://doi.org/10.1038/s41598-019-40885-z ![]() |
[9] |
Shah V, Shah J (2020) Recent trends in targeting miRNAs for cancer therapy. J Pharm Pharmacol 72: 1732-1749. https://doi.org/10.1111/jphp.13351 ![]() |
[10] | Lan H, Lu H, Wang X, et al. (2015) MicroRNAs as potential biomarkers in cancer: opportunities and challenges. Biomed Res Int 2015: 125094. https://doi.org/10.1155/2015/125094 |
[11] |
Arlauckas SP, Popov AV, Delikatny EJ (2016) Choline kinase alpha—Putting the ChoK-hold on tumor metabolism. Prog Lipid Res 63: 28-40. https://doi.org/10.1016/j.plipres.2016.03.005 ![]() |
[12] |
Gruber J, See Too WC, Wong MT, et al. (2012) Balance of human choline kinase isoforms is critical for cell cycle regulation: implications for the development of choline kinase-targeted cancer therapy. FEBS J 279: 1915-1928. https://doi.org/10.1111/j.1742-4658.2012.08573.x ![]() |
[13] |
Ramírez de Molina A, Gutiérrez R, Ramos MA, et al. (2002) Increased choline kinase activity in human breast carcinomas: clinical evidence for a potential novel antitumor strategy. Oncogene 21: 4317-4322. https://doi.org/10.1038/sj.onc.1205556 ![]() |
[14] |
Clem BF, Clem AL, Yalcin A, et al. (2011) A novel small molecule antagonist of choline kinase-α that simultaneously suppresses MAPK and PI3K/AKT signaling. Oncogene 30: 3370-3380. https://doi.org/10.1038/onc.2011.51 ![]() |
[15] |
Gokhale S, Xie P (2021) ChoK-full of potential: Choline kinase in B cell and T cell malignancies. Pharmaceutics 13: 911. https://doi.org/10.3390/pharmaceutics13060911 ![]() |
[16] |
Crilly KS, Tomono M, Kiss Z (1998) The choline kinase inhibitor hemicholinium-3 can inhibit mitogen-induced DNA synthesis independent of its effect on phosphocholine formation. Arch Biochem Biophys 352: 137-143. https://doi.org/10.1006/abbi.1998.0601 ![]() |
[17] |
Schiaffino-Ortega S, Baglioni E, Mariotto E, et al. (2016) Design, synthesis, crystallization and biological evaluation of new symmetrical biscationic compounds as selective inhibitors of human Choline Kinase α1 (ChoKα1). Sci Rep 6: 23793. https://doi.org/10.1038/srep23793 ![]() |
[18] |
Lin XM, Hu L, Gu J, et al. (2017) Choline kinase α mediates interactions between the epidermal growth factor receptor and mechanistic target of rapamycin complex 2 in hepatocellular carcinoma cells to promote drug resistance and xenograft tumor progression. Gastroenterology 152: 1187-1202. https://doi.org/10.1053/j.gastro.2016.12.033 ![]() |
[19] |
Barthe A, Bodaar K, Issaian A, et al. (2022) Choline kinase a is required for alkylating agent resistance in T-cell acute lymphoblastic leukemia. Blood 140: 2258. https://doi.org/10.1182/blood-2022-162508 ![]() |
[20] |
Qin SY, Li B, Chen M, et al. (2022) MiR-32-5p promoted epithelial-to-mesenchymal transition of oral squamous cell carcinoma cells via regulating the KLF2/CXCR4 pathway. Kaohsiung J Med Sci 38: 120-128. https://doi.org/10.1002/kjm2.12450 ![]() |
[21] |
Zeng S, Liu S, Feng J, et al. (2020) MicroRNA-32 promotes ovarian cancer cell proliferation and motility by targeting SMG1. Oncol Lett 20: 733-741. https://doi.org/10.3892/ol.2020.11624 ![]() |
[22] |
Zhang JX, Yang W, Wu JZ, et al. (2021) MicroRNA-32-5p inhibits epithelial-mesenchymal transition and metastasis in lung adenocarcinoma by targeting SMAD family 3. J Cancer 12: 2258-2267. https://doi.org/10.7150/jca.48387 ![]() |
[23] | Liu YJ, Zhou HG, Chen LH, et al. (2019) MiR-32-5p regulates the proliferation and metastasis of cervical cancer cells by targeting HOXB8. Eur Rev Med Pharmacol Sci 23: 87-95. https://doi.org/10.26355/eurrev_201901_16752 |