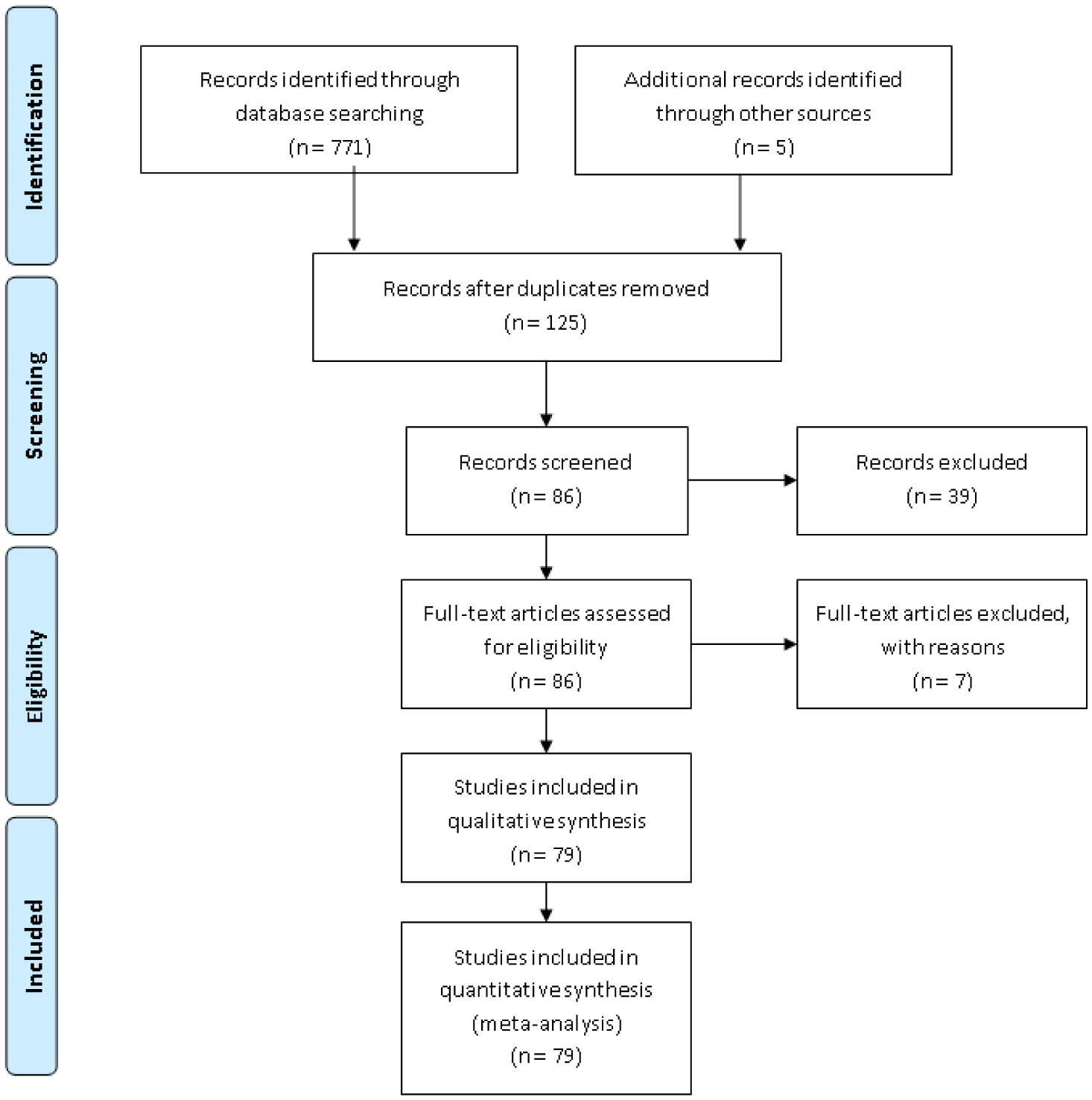
At present, radiotherapy (RT) is widely used in cancer treatment, but traditional RT methods using ionizing radiation cannot avoid damage to normal tissues. Therefore, the development of a more precise RT is an important research direction for relevant researchers. Concurrently, research on radiosensitizers (RSs) using nanotechnology is developing rapidly, and RSs that are selective for cancerous tissues or cancer cells may become an important part of future precision RT.
Using RSs and RT as keywords, the relevant papers in the PubMed database from 2013 to 2022 were summarized. Articles on RS with selectivity to cancer tissue were collected. Among the selected articles, RSs were classified into “active selectivity”, “passive selectivity” and “others” according to the different selectivity principles of RSs.
A total of 771 articles were retrieved from PubMed. After screening, the research content of the remaining 79 articles was found to be related to the selectivity of RSs to cancer tissues. Among them, 28 articles were classified as “active selectivity”, and most of the sensitizers in this category could target specific targets in cancer tissues. There were 30 papers classified as “passive selectivity” and the selectivity principles were mainly the enhanced permeability and retention (EPR) effect, aggregation caused by pH sensitivity, and aggregation in anoxic environments. There were 21 papers classified as “others”. The sensitizers in these studies showed selectivity for cancer tissue, but the mechanism was not clear. This review attempts to summarize studies on RSs that are selective for cancer tissues.
We reviewed nearly ten years of literature on selective RSs and classified the selectivity of different RSs into active and passive selectivities.
Citation: Hengmao Zhang, Haobo Zhao, Ming Chi, Kaizhen Yang, Yukang Chen, Jiahui Mao, Peilin Li, Zukang Wang, Faqiao Song, Wenxuan Guo, Miyu Sakai, Junko Takahashi. A systematic review on the development of radiosensitizers, with cancer selectivity, for radiotherapy using ionizing radiation[J]. AIMS Bioengineering, 2023, 10(2): 89-110. doi: 10.3934/bioeng.2023008
[1] | Dewmini Mututantri-Bastiyange, James C. L. Chow . Imaging dose of cone-beam computed tomography in nanoparticle-enhanced image-guided radiotherapy: A Monte Carlo phantom study. AIMS Bioengineering, 2020, 7(1): 1-11. doi: 10.3934/bioeng.2020001 |
[2] | Mujibullah Sheikh, Pranita S. Jirvankar . Harnessing artificial intelligence for enhanced nanoparticle design in precision oncology. AIMS Bioengineering, 2024, 11(4): 574-597. doi: 10.3934/bioeng.2024026 |
[3] | Meiling Sun, Changlei Cui . Transforming lung cancer care: Synergizing artificial intelligence and clinical expertise for precision diagnosis and treatment. AIMS Bioengineering, 2023, 10(3): 331-361. doi: 10.3934/bioeng.2023020 |
[4] | Chun He, James C.L. Chow . Gold nanoparticle DNA damage in radiotherapy: A Monte Carlo study. AIMS Bioengineering, 2016, 3(3): 352-361. doi: 10.3934/bioeng.2016.3.352 |
[5] | Praveen Kumar, Sakshi V. Izankar, Induni N. Weerarathna, David Raymond, Prateek Verma . The evolving landscape: Role of artificial intelligence in cancer detection. AIMS Bioengineering, 2024, 11(2): 147-172. doi: 10.3934/bioeng.2024009 |
[6] | P. Mora-Raimundo, M. Manzano, M. Vallet-Regí . Nanoparticles for the treatment of osteoporosis. AIMS Bioengineering, 2017, 4(2): 259-274. doi: 10.3934/bioeng.2017.2.259 |
[7] | Segun Akinola, Leelakrishna Reddy . Nanoscale antenna systems: Transforming wireless communications and biomedical applications. AIMS Bioengineering, 2023, 10(3): 300-330. doi: 10.3934/bioeng.2023019 |
[8] | Gerald Pawlish, Kyle Spivack, Adam Gabriel, Zuyi Huang, Noelle Comolli . Chemotherapeutic loading via tailoring of drug-carrier interactions in poly (sialic acid) micelles. AIMS Bioengineering, 2018, 5(2): 106-132. doi: 10.3934/bioeng.2018.2.106 |
[9] | Kristen K. Comfort . The rise of nanotoxicology: A successful collaboration between engineering and biology. AIMS Bioengineering, 2016, 3(3): 230-244. doi: 10.3934/bioeng.2016.3.230 |
[10] | Amin Shakiba, Oussama Zenasni, Maria D. Marquez, T. Randall Lee . Advanced drug delivery via self-assembled monolayer-coated nanoparticles. AIMS Bioengineering, 2017, 4(2): 275-299. doi: 10.3934/bioeng.2017.2.275 |
At present, radiotherapy (RT) is widely used in cancer treatment, but traditional RT methods using ionizing radiation cannot avoid damage to normal tissues. Therefore, the development of a more precise RT is an important research direction for relevant researchers. Concurrently, research on radiosensitizers (RSs) using nanotechnology is developing rapidly, and RSs that are selective for cancerous tissues or cancer cells may become an important part of future precision RT.
Using RSs and RT as keywords, the relevant papers in the PubMed database from 2013 to 2022 were summarized. Articles on RS with selectivity to cancer tissue were collected. Among the selected articles, RSs were classified into “active selectivity”, “passive selectivity” and “others” according to the different selectivity principles of RSs.
A total of 771 articles were retrieved from PubMed. After screening, the research content of the remaining 79 articles was found to be related to the selectivity of RSs to cancer tissues. Among them, 28 articles were classified as “active selectivity”, and most of the sensitizers in this category could target specific targets in cancer tissues. There were 30 papers classified as “passive selectivity” and the selectivity principles were mainly the enhanced permeability and retention (EPR) effect, aggregation caused by pH sensitivity, and aggregation in anoxic environments. There were 21 papers classified as “others”. The sensitizers in these studies showed selectivity for cancer tissue, but the mechanism was not clear. This review attempts to summarize studies on RSs that are selective for cancer tissues.
We reviewed nearly ten years of literature on selective RSs and classified the selectivity of different RSs into active and passive selectivities.
Currently, 60–70% of cancer patients in Europe and the United States receive radiotherapy (RT) during treatment, although the percentage varies by country [1]. RT is by far the most widely used and effective antitumor therapy. However, although irradiation can destroy cancer cells, it cannot avoid damaging healthy cells and tissues near the treatment area. Therefore, to reduce the damage to normal tissues, precision RT has become an important trend in the development of RT technology.
In the development of precision RT, the use of radiosensitizers (RSs) is becoming an important part in the treatment of precise RT using ionizing radiation. Radiosensitizers are drugs that are used to make tumor cells more sensitive to radiation, make radiation more effective in killing tumor cells and improve the control and cure rates. The main mechanisms involved are direct and indirect. Direct radiation damages cancer cells by generating reactive oxygen species (ROS) through the physicochemical reaction between ionizing radiation and RSs. The indirect mechanisms include: (I) inhibiting the repair of radiation-induced DNA damage, aggravating the degree of DNA damage; (II) disrupting the cell cycle and organelle function to increase cytotoxicity; and (III) inhibiting the expression of radiation resistance genes or promoting the expression of radiation-sensitive genes [2].
Currently, research on RSs using nanotechnology is developing rapidly, and RSs that are selective for cancerous tissues or cancer cells may become an important part of precision RT in the future. The key to the study of such RSs is to explore their selectivity for cancer tissues. Therefore, we used the PubMed database to search for papers related to RSs published from 2013 to 2022. RSs with selectivity for cancer tissues or cancer cells were classified and summarized.
Medline (via PubMed) was searched using the term “(Radiotherapy) AND (Radiosensitizer)”. Only studies conducted between 2013 and 2022 were included in the meta-analysis. A total of 771 articles were obtained, followed by screening for the selectivity of the RSs in the study, and a total of 79 articles where the RSs were selective were mentioned in the abstract were finally included. This was followed by classification according to the principle of action of RS selectivity into active selection (specific receptors on tumor cells, etc.) and passive selection (enhanced permeability and retention (EPR) effects, etc.), yielding a total of 28 articles for “active” selection, 30 articles for “passive” selection, and 21 articles for “others”.
Because the drugs studied by different investigators varied depending on the cancers they focused on in their articles, we categorized them according to the RS-related cancers they studied. In addition, since these RSs have various selectivity principles for cancer tissue or cancer cells, we divided them into “active selectivity” and “passive selectivity” according to their selectivity principles.
Active selectivity means that an RS can target a certain receptor in cancer cells or cancer tissue and only has a radiosensitizing effect on the cancer tissue. The “receptor” may be a specific protein overexpressed on the cancer cell membrane or specific enzymes or other substances in the tumor microenvironment.
Passive selectivity refers to the use of the characteristics of the tumor microenvironment to aggregate drug particles at the tumor location. For example, the EPR effect uses the special complex vascular environment of tumor tissue to ensure the aggregation effect of specific size particles; some pH-sensitive substances are present in the microenvironment and specific reactions occur in acidic environments; and certain drugs specifically target the hypoxic environment inside cancer tissues.
However, there are still some studies on new sensitizers that are only at the initial stage, and researchers have not yet identified their specific mechanism, so we classify these sensitizers as “others”.
In addition, when we summarized the articles of these researchers, we found that those sensitizers with passive selectivity are almost all targeted at the special environment of tumors, and do not have an effect specific to a single type of cancer. Furthermore, among sensitizers with active selectivity, some sensitizers are selective for certain types of cancer-specific targets, here named “cancer-specific”, while sensitizers that only target overexpressed proteins are the same as those with passive selectivity, here named “non-cancer-specific”.
Active selection of RSs for tumor cells is achieved primarily with specific receptors that are only overexpressed on tumor cells, usually by surface modification of the RS in the form of nanoparticles (NPs) to make it tumor-cell selective [3]–[30]. Some typical examples are listed in Table 1.
Selectivity Mechanism | RS | Condition | Published Date | Ref |
Folic acid (FA) receptor | a biodegradable nanocomposite BiPt-folic acid-modified amphiphilic polyethylene glycol (BiPt-PFA) | breast cancer | 2020 | [3] |
FA receptor | a dual functional mesoporous silica nanoparticle (MSN) formulation of the valproic acid (VPA) | glioblastoma | 2017 | [4] |
FA receptor | FA-BSANP | cervical cancer | 2014 | [5] |
FA receptor | Au@Fe2O3 NPs modified with FA | KB cells(papilloma) | 2019 | [6] |
FA receptor | albumin coated bismuth sulfide NPs | breast cancer | 2019 | [7] |
Photosensitizer (BPD) | benzoporphyrin derivative (BPD) | head and neck squamous cell carcinoma (HNSCC) | 2021 | [8] |
αvβ3 integrin | ultrasmall iridium nanocrystals (Ir-RGD-TAT (Ir-R/T) NCs) | breast cancer | 2019 | [9] |
αvβ3 integrin | GNP-PEG-cRGDfK | glioma | 2018 | [10] |
Photosensitizer (Ce6) | hyaluronic acid (HA), polyaniline (PANI), WS2 nanodots (WS2), and chlorin e6 (Ce6) into a single nanoplatform (HA-WS2@PANI/Ce6) | breast cancer | 2017 | [11] |
Photosensitizer (TCPP) | Hafnium (Hf4+) and tetrakis (4-carboxyphenyl) porphyrin (TCPP) | breast cancer | 2016 | [12] |
Photosensitizer (GaPcCl) | gallium phthalocyanine chloride (GaPcCl) | breast cancer | 2020 | [13] |
Photosensitizer (MTX) | Mitoxantrone (MTX) | melanoma | 2013 | [14] |
Photosensitizer (5-ALA) | 5-aminolevulinic acid (5-ALA) | prostate cancer | 2019 | [15] |
PARA | Talazoparib | small cell lung cancer (SCLC) | 2018 | [17] |
αvβ3 integrin | nucleus-targeting MTiO2 NPs (MTiO2(SN-38)-TAT-RGD) | breast cancer | 2019 | [21] |
αvβ3 integrin | antiEGFRiRGD | gastric cancer | 2018 | [22] |
αvβ3 integrin | RGD peptide has been covalently conjugated to selenadiazole derivative (RGD-SeD) | liver cancer | 2018 | [23] |
αvβ3 integrin | Gold NP | prostate cancer | 2020 | [24] |
EGFR | lapatinib | breast cancer | 2016 | [25] |
EGFR | silibinin | prostate cancer | 2015 | [26] |
EGFR | C-siPLK1 NPs | non-small cell lung cancer (NSCLC) | 2019 | [27] |
PSMA | PSMA-targeted DTX-loaded Au NPs | prostate cancer(Pca) | 2021 | [28] |
PSMA | AuNPs | prostate cancer | 2020 | [29] |
PSMA | gold-based radiation sensitizer | prostate cancer | 2019 | [30] |
Among the widely used RSs with active selectivity, there are some without tumor-specific properties. These RSs exploit the typical characteristics of tumor cells, thus achieving selectivity for active cancer cells. Among these agents, the folate receptor and ανβ3 integrin, which are overexpressed in many cancer cells, are the most commonly used. The realization of selectivity for these two receptors will be the focus of this review.
Folic acid (FA) is a non-immunogenic small molecule, and many cancer cells overexpress FA receptors that can bind specifically to FA. Therefore, folate receptors allow FA-functionalized NPs to realize specific recognition of cancer cells, which provides a way for the active selectivity of RSs. Several studies have been conducted on FA receptors, and Zhang et al. proposed biodegradable nanocomplexes of bi-folate-modified amphiphilic polyethylene glycols (BiPt-PFA NPs) as the RSs. BiPt-PFA is an FA receptor overexpressed on the surface of a variety of cancer cells, such as breast cancer cells, and is, thus. ideal for tumor targeting. BiPt-PFA NPs can act as enzymes to catalyze the breakdown of hydrogen peroxide in the tumor environment, generating oxygen to improve the hypoxic environment, and can absorb near-infrared light energy, converting it into heat, thus producing a thermal effect and enhancing the therapeutic effect of radiation [3]. To target folate receptors that are specifically overexpressed in glioblastoma cells, Zhang et al. synthesized mesoporous silica nanoparticle (MSNs) surfaces modified by a folate ligand that selectively binds to folate receptors. The expression of FA receptors in cancer cells represents an attractive therapeutic target for novel tumor-specific agents. Based on pH-responsive nanocarriers, highly efficient targeted delivery of sensitizers to tumors through the expression of tumor cell microenvironment, using pH and irradiation to activate the effect of sensitizers, is a novel and efficient method that can be applied to other cancers [4]. In addition to the studies described above, Huang et al. chose FA as the targeting ligand to create formulations of bovine serum albumin nanoparticles (BSANPs) targeting cancer cells as a drug delivery system for organoselenium compounds (PSeD) [5]. Mirrahimi et al. used folic acid to modify Au@Fe2O3 NPs to make them selective for tumor cells [6], and Nosrati et al. used albumin coated bismuth sulfide NPs as a selective RS [7].
In addition to the use of folic acid receptors, the application of ανβ3 integrin, which is overexpressed on the surface of tumor cells, is also an important way to achieve tumor selectivity. Many studies have reported that malignant tumors, such as breast, malignant melanoma, ovarian, and lung tumors, characteristically overexpress αvβ3 integrins in the cell membrane compared to normal cells. This feature presents an attractive basis for the design of targeting peptides for active tumor cell vectorization, and some related studies have been published. One of the hot spots of current research is the unique targeting effect of arginylglycylaspartic acid (RGD) peptide, a tumor-targeting peptide, which is a new target for the treatment of tumors. ανβ3 integrin is overexpressed in various cancer cells. Due to its selective binding to νβ3 integrin, the RGD peptide is regarded as an ideal targeting molecule for modifying anticancer/antitumor drugs and NP delivery systems. To further improve the selectivity of selenazole derivatives for cancer cells in RT, a new selenazole derivative (SeD) was modified with a cyclic RGD peptide. After modification, both in vivo and in vitro experiments proved that RGD-SeD significantly improved the selectivity compared with SeD alone, and enhanced the anticancer effect in HepG2 hepatoma cells overexpressing αVβ3 integrin, but it had no toxicity in normal hepatocytes. Similarly, the nonspecific restriction of cell-penetrating peptides (CPP) can be solved by combining it with the RGD peptide [8]. Wang et al. used ultra-small iridium nanocrystals (Ir-RGD-TAT (Ir-R/T) NCs) as RSs, which may confer significant tumor targeting by attaching both RGD and TAT peptides to the surface of the designed nanosensitizers [9]. In this way, RSs may be made to have attractive tumor cell nuclear targeting properties, facilitating sensitization for tumor RT in vitro and in vivo. It can completely eradicate mouse mammary 4T1 tumors, offering the potential for highly efficacious and bio-safe multimodal tumor therapy. In addition, Enferadi et al. found that integrin αvβ3 is a transmembrane glycoprotein α/β receptor that participates in inflammation, tumor metastasis and angiogenesis, and can therefore be considered as a notable target for the delivery of therapeutic agents to cancer cells. The cyclic RGD peptide, as a functionalized NP, targets ανβ3 and causes vascular damage specifically to tumor tissues. In addition, ultra-small gold nanoparticles (GNPs) are less likely to accumulate in the blood and ensure lower GNP uptake in the liver and spleen with a higher dose enhancement [10]. Receptor proteins exist in tumor tissues and can directly act on tumor tissue and cause vascular damage.
A selective photosensitizer is a substance that selectively accumulates in cancer cells and reacts with electromagnetic wave energy. Radiation sensitizers using such photosensitizers have been studied, and the mechanism of tumor selectivity differs depending on the type of photosensitizer used. The mechanisms by which Chlorin e6 (Ce6), benzoporphyrin derivative (BPD), tetrakis (4-carboxyphenyl) porphyrin (TCPP) and other porphyrin-derivative photosensitizers work are not yet fully elucidated, but are thought to be mediated by the LDL receptor, and are being investigated as RSs using this as a tumor-selective carrier. Hyaluronic acid (HA), polyaniline (PANI), WS2 nanodots (WS2) and chlorin e6 (Ce6) were synthesized as single nanoRSs (HA-WS2@PANI/Ce6) to exploit the tumor selectivity of Ce6, because the cell-damaging effect of light irradiation enhances the therapeutic effect of X-rays [11]. The administration of a BPD followed by light irradiation enhances the subsequent radiation effects [8]. Nanoscale metal organic frameworks composed of hafnium (Hf4+) and (TCPP) are RSs designed with TCPP as a carrier. Moreover, hafnium has a sensitizing effect upon exposure to radiation [12]. In contrast, gallium phthalocyanine chloride (GaPcCl) induced apoptosis by X-ray irradiation alone, and the effect of its combined use with light irradiation has been verified [13]. Anticancer drug mitoxantrone (MTX) is known to have a photosensitizing ability. Sazgarnia et al. showed that MTX alone functions as a radiation sensitizer and has further cell-damaging effects when exposed to X-rays and light [14]. The compound 5-aminolevulinic acid (5-ALA) utilizes the metabolic mechanism of cancer cells to accumulate protoporphyrin IX (PpIX) in a cancer cell-specific manner. It has already been used clinically as an agent for photodynamic diagnosis and therapy, and data on the selective accumulation of PpIX in various tumors have been accumulated. In addition, 5-ALA has been tested for its efficacy as an RS in various types of cancers. Miyake et al. examined the radioprotective ability against normal tissues, as well as the ability to radiosensitize tumor cells [15]. Moreover, the radiosensitizing action of PpIX has been verified to generate ROS upon exposure to radiation [16].
In addition to the selective RSs described above, which are universally applicable to most cancer cells, other studies have focused on exploiting the unique qualities of certain tumor cells to achieve specific selectivity of RSs for a particular tumor cell, which is in line with the need for targeted therapy.
In small cell lung cancer (SCLC), poly ADP-ribose polymerase 1 (PARP1) is a promising molecular target, and clinical trials of its inhibitors are currently underway. A study by Laird et al. suggested that PARP trapping might be particularly important in SCLC, as PARP is only expressed at high levels in SCLC and is captured by the PARP1 enzyme [17]. However, the specific clinical applications require further study. With regard to U87 glioma cells, Li et al. recently developed several aptamers which can bind tightly to U87 glioma cells. Among these aptamers, GMT8 has the highest affinity for U87 cells; therefore, it can be used as a good targeting ligand to deliver NPs to glioma cells [18]. Metal NPs modified by the GMT8 aptamer and with a poly(ethylene glycol) (PEG) surface enable passive accumulation of PEGylated NPs at tumor sites through the EPR effect, in addition to their targeting properties.
Although the use of the tumor microenvironment can also enhance the effect of RS, Jiao et al. found that low-density lipoprotein receptor-related protein-1 (LRP1) is overexpressed in brain capillary endothelial cells and glioma cells, and Angiopep-2 can target LRP1 [19]. Since matrix metalloproteinase 2 (MMP-2) is highly expressed in the tumor microenvironment, angiopep-2 was conjugated using an MMP-2-responsive peptide as an enzymatically cleavable linker. Angiopep-2 penetrates the blood-brain barrier and accumulates at tumor sites, where the linker is cleaved by MMP-2, eliminating its shielding effect. The exposed CPPs then internalize the delivery system into tumor cells. FOXO3a is an important member of the transcription factor FOX protein family and is considered a tumor suppressor gene. However, some studies have shown that FOXO3a can protect tumor cells from oxidative stress and hypoxia. Therefore, FOXO3a may play different roles in different conditions and environments. A study confirmed that the short-chain fatty acid, butyrate, significantly enhanced radiation-induced cell death and therapeutic effects, and showed high specificity and selective anticancer effects against Patient-derived organoids from colorectal cancer patients (CRC-PDOs), which originated from colorectal cancer patients [20]. Specifically, butyrate increases the radiosensitivity of CRC-PDO via the Warburg effect. It induces cell cycle arrest regulated by p21, p57, and GADD45 by increasing FOXO3A transcription. At the same time, it does not increase cell death induced by radiation in normal organs. However, owing to the high heterogeneity of tumors, it is not an RS that can act effectively on most cancer cells.
The tumor microenvironment (TME) consists of tumor tissue and normal cells (blood vessels, immune cells, fibroblasts, signaling molecules, extracellular matrix (ECM), etc.) and influences tumor progression. Tumors influence the TME by promoting tumor angiogenesis and inducing peripheral immune tolerance through extracellular signals. Some RSs can achieve the effects of targeted therapy by changing the tumor microenvironment [31]–[60]. Typical examples of this type of RS are listed in Table 2.
Selectivity Mechanism | RS | Condition | Publish Date | Ref |
EPR Effect | multistage-responsive Cs–Au-ICG NPs | breast cancer | 2021 | [32] |
EPR Effect | gold nanoparticles (AuNP-PEG-HER2ab) | human ovarian cancer | 2019 | [33] |
EPR Effect | hyaluronic acid modified Au nanocages (AuNCs-HA) | breast cancer | 2019 | [34] |
EPR Effect | dumbbell-like Au-TiO2 nanoparticles (DATs) | breast cancer | 2018 | [35] |
EPR Effect | sub-2 nm gold nanoclusters | cervical cancer | 2014 | [36] |
EPR Effect | Bi2Se3 | cervical cancer | 2014 | [37] |
EPR Effect | iridium nanocrystals (IrNCs) | breast cancer | 2018 | [38] |
EPR Effect | DM1-NO PLGA NPs | NSCLC | 2020 | [40] |
EPR Effect | cisdiamminedichloroplatinum(Ⅱ) (cisplatin, CDDP) | NSCLC | 2015 | [41] |
Hypoxic condition toxic | a hypoxic RS-prodrug liposome (MLP) | glioma | 2017 | [42] |
PH-Value Sensitive | stimuli-responsive core-shell NP system | lung cancer | 2017 | [43] |
PH-Value Sensitive | Mn clusters | breast cancer | 2020 | [45] |
Hypoxic condition toxic | nitroimidazole alkylsulfonamides (2-nitroimidazole analogues,7 and 19 are effective) | colorectal carcinoma | 2018 | [46] |
EPR Effect | bacteria: 1. Escherichia coli strain K-12, Salmonella typhimurium ΔppGpp (S.t ΔppGpp); 2. lipid mutants Salmonella strains (Salmonella YS1456 and YS1646) Nanoparticles:gold (Au), gadolinium (Gd), iron (Fe), bismote (Bi), titanium (Ti), and hafnium (Hf) |
colon cancer | 2018 | [47] |
EPR Effect | Au-Pt NPs | HUEVC; breast cancer | 2021 | [48] |
EPR Effect | BSA-Au-MnO2 composite NPs | breast cancer | 2017 | [49] |
EPR Effect | Gd@C-dots | NSCLC | 2021 | [50] |
EPR Effect | cetuximab-functionalized gold NPs | HNSCC | 2018 | [51] |
Hypoxic condition toxic | UCHM (upconversion nanoparticle (UCNP) core located within the hollow core of an outer mesoporous silica (HMs) shells) | hela cells | 2018 | [51] |
EPR Effect | folic acid and BSA decorated gold nanoclusters | intracranial glioma tumors | 2019 | [52] |
EPR Effect | gold nanoparticles (GNPs) | brain tumor | 2013 | [53] |
EPR Effect | ThermoDox or doxorubicin (DOX) | human fibrosarcoma | 2018 | [54] |
EPR Effect | 2DG-grafted GQDs | Osteosarcoma (OS) | 2020 | [55] |
EPR Effect | MnO2-HA@H2PtCl6 (MHP) | colon cancer | 2020 | [56] |
PH-Value Sensitive | pharmacological ascorbate | glioblastoma, lung cancer, pancreatic cancer | 2021 | [57] |
Hypoxic condition toxic | integrated nanosystem (Bac@BNP) | breast cancer | 2022 | [58] |
Hypoxic condition toxic | CAC4A+AQ4N | breast cancer | 2022 | [59] |
Hypoxic condition toxic | CuII and ZnII Complexes | lung cancer | 2020 | [60] |
A key concern when using radiation sensitizers for RT is how to achieve radiation sensitization by accumulating radiation sensitizing NPs in large numbers near the tumor tissue. Compared with normal tissues, tumor-selective or tumor-specific properties, including EPR effects, are still poorly understood. Most solid tumors exhibit a unique pathology called the EPR effect, which includes extensive angiogenesis, resulting in hyper-vasculature, defective vasculature, impaired lymphatic drainage/recovery systems and increased production of permeable mediators [31]. The EPR effect has been used by many drugs or drug delivery methods, and is an important mechanism for the delivery of anticancer drugs.
There are many metallic nanoparticle RSs that use the EPR effect to accumulate in tumor tissue. Hua et al. developed tunable size photothermal therapy (PTT)/RT/polyamide (PA)/near-infrared fluorescence (NIRF) reagent Cs-Au-ICG NPs by combining indocyanine green (ICG) with carboxymethyl chitosan (CS)-modified size-tunable Au NCs system (Cs-Au NCs). The combination of the EPR effect, which allows for the accumulation in large numbers around the tumor, with photothermal treatment achieves the desired therapeutic effect [32]. Hatoyama et al. conducted a quantitative study on gold NPs. Polyethylene glycol-modified gold NPs were used to enhance the EPR effect. The production of ROS around gold NPs aggregated in tumor tissue is accelerated by the photoelectric and/or Compton effects, which enhances the RT effect [33]. Xu et al. found that nanoplatform based on HA modified Au nanocages (AuNCs-HA) can be used as a RS for RT, photosensitizer for photodynamic therapy, and therapeutic agent for PTT. HA is a natural polymer material that aggregates near tumors owing to the EPR effect and has shown the ability to target cd44-overexpressed breast cancers [34]. Cheng et al. investigated a hybrid anisotropic nanostructure of gold (Au) and titanium dioxide (TiO2) as an RS for the RT of triple-negative breast cancer. Owing to the EPR effect, the radiodensity effect of dumbbell-like Au-TiO2 nanoparticles (DATs) on tumor tissues is significantly enhanced, even at lower concentrations [35]. Bismuth is a high-atomic-number element that effectively enhances radiosensitization, while selenium is a non-metallic element with anticancer activity. Zhang et al. creatively combined Bi2Se3, a compound of the two, with 54-nm-wide nanoplates to synthesize metabolizable radiosensitizing nanoplates [36], the principle of which is to enhance damage to cancer cell tissue by enhancing permeability and retention; after 90 days of treatment, the nanoplates achieved a 93% clearance rate. Finally, it is noteworthy that bismuth has a higher photoelectric absorption coefficient than Au and Pt; therefore, Bi2Se3 nanoplates can also be used as X-ray contrast agents. Interestingly, the Sub-2 nm gold nanoclusters developed by Zhang et al. are also a typical example of the EPR effect [37]. The developed gold nanoclusters are made up of Au cores, which have a strong radiosensitizing effect, and glutathione shells, which have good biocompatibility. To make more effective use of the EPR effect and improve the efficiency of nanogold accumulation and effective post-treatment renal clearance, while minimizing the toxic side effects, the authors chose ultra-small sub-2 nm nanogold particles, which could improve the enhancement of cancer RT through better penetration and retention.
Some metallic nanoparticle RSs not only have an EPR effect, but can also break down hydrogen peroxide in the tumor environment into oxygen, improving the effect of the hypoxic environment on RT. Feng et al. were the first to successfully synthesize very large iridium nanocrystals (IrNCs) with a uniform particle size distribution and encapsulated them in invisible liposomal carriers to obtain catalytically active Ir@liposomes [38]. Hua et al. proposed the fabrication of smart multifunctional NPs using biocompatible and biodegradable components for RT. These particles gradually degrade into small 10 nm particles once inside the tumor, resulting in good intra-tumor penetration. Simple Au4-IO NP-cRGD assemblies based on aggregation-induced emission (AIE) enhance the Fenton reaction at RT [39]. These NPs not only aggregate around tumors due to the EPR effect but also catalyze the breakdown of oxygen by hydrogen peroxide in the tumor surroundings, further enhancing the effectiveness of RT.
In addition, some non-metallic NPs can be used as RS and accumulate in cancer tissues owing to the EPR effect. Gao et al. presented their study on Nanoparticles Encapsulating Nitrosylated Maytansine and reported that once delivered to tumors, external irradiation cleaves the NP S–N bond within the tumor, releasing DM1 as an antimitotic agent [40]. Jung et al. investigated cisplatin-incorporated liposomes targeting the epidermal growth factor receptor and showed that they enhanced the efficacy of RT without nephrotoxicity [41]. Size-controlled liposomes deliver drugs to tumors via the EPR effect and protect them from metabolic processes that clear them from the body too early [41].
Doxorubicin (DOX), which is often used as a chemotherapeutic agent, can also act as a RS to cure human fibrosarcoma [42]. Currently, to reduce its toxicity and increase its efficacy, a thermosensitive liposome containing DOX (ThermoDox) is used for tumor delivery by liposomes, allowing it to act as a nanomedicine [42]. Thus, DOX selectively targets fibrosarcoma cells. However, ThermoDox at 37 °C did not induce radiosensitization. For radio sensitization to be realized, the temperature should be increased to 43 °C, which requires local hyperthermia [42].
In addition to environmental factors of vascular structure, the pH value can also be exploited for the development of RSs with cancer tissue selectivity due to the different pH values of the tumor tissue. Menon et al. developed a combination of chemotherapy and RT using core-shell nano particles for lung cancer treatment [43]. This NP system controls the release of RS (NU7441) and the chemotherapeutic drug (gemcitabine hydrochloride) by a poly(lactic-co-glycolic acid) (PLGA) core in response to the acidic tumor environment. Another strategy employed was a “double-key” response strategy, which was used to construct a transglutaminase (TGase)/pH-responsive RS (Au@TAcoGal). When it reaches the tumor site, it can be incorporated into hepatoma cells via phenylboronic acid (PBA) and can aggregate based on cell acidity levels and overexpression of TGase [44].
The Mn clusters developed by Lv et al. also effectively utilize the pH value of the tumor tissue [45]. The Mn clusters not only utilize the pH of the tumor microenvironment to enhance radiation damage to cancer cells, but also show reducibility and free radical scavenging ability in a neutral environment, which protects normal tissue cells from radiation damage. Mn clusters increase radiation-induced production of ROS through catalytic oxidation in acidic environments. In contrast, in a neutral environment, Mn clusters have a strong ability to remove ROS and relieve and repair radiation damage to the hematopoietic system. Mn clusters can be rapidly excreted from the body without causing long-term toxic effects.
Furthermore, targeting the hypoxic tumor environment is also one of the means to improve tumor therapy. In experiments on colorectal cancer cells, the characteristics of hypoxia selectivity and radiosensitization were tested in vitro. The results showed that 2-nitroimidazole with neutral and basic side chains showed considerable hypoxia selectivity (HCR 40–96), while the example with acidic side chains showed lower hypoxia selectivity. Simultaneously, two new RSs, 7(N-(2-Hydroxyethyl) (2-nitro-1H-imidazol-1-yl) methane sulfonamide) and 19(N-(2-Hydroxyethyl) (5-nitro-1H-imidazol-1-yl) methane sulfonamide), were identified. When delivered as phosphate precursor drugs, 39(2-(((2-Nitro-1H-imidazol-1-yl) methyl) sulfonamide) ethyl Dihydrogen Phosphate) and 41(2-(((5-Nitro-1H-imidazol-1-yl) methyl) sulfonamide) ethyl Dihydrogen Phosphate), the peak concentration of tumor drugs increased due to the improvement of water solubility and drug delivery efficiency. Moreover, 2-nitroimidazole 7, with high electron affinity, also showed hypoxia-selective cytotoxicity. Therefore, they are more suitable for SBRT environments [46]. At present, many bacteria have been used for cancer treatment, such as Escherichia coli strain K-12, Salmonella typhimurium ΔppGpp (S.t ΔppGpp), and lipid mutant Salmonella strains (Salmonella YS1456 and YS1646), which have been applied to in vivo experiments to verify their therapeutic effects on colon cancer and melanoma cancer. Anaerobic bacteria that grow selectively in the hypoxic region of the tumor can destroy cancer cells by releasing proteases, lipases, hydrolases, or protein toxins, such as Pseudomonas exotoxin, diphtheria toxin and ricin [47].
There were 21 papers where the RS were classified as “other” [61]–[81]. As it was difficult to describe them systematically, we selected anticancer drugs, microRNAs, and natural substances as topics. In addition, there is a special example of RS from Park et al. which we classified as “others”, because the RS they investigated shows the characteristics of both active and passive RSs, which belongs to neither “active” nor “passive”. Table 3 shows examples of “others”.
One of the categories available is that of anticancer agents. Minea et al. based their previous research on temozolomide (TMZ), followed by chemical derivatization of TMZ at other positions (other than the alkyl group), and generated NEO212 by derivatizing TMZ with the natural monoterpene perillyl alcohol. NEO212 crosses the blood-brain barrier more efficiently than TMZ, and its selective accumulation in tumors has been verified using mouse models. Furthermore, NEO212 was shown to possess potent cytotoxic and RS activities in an in vitro evaluation using a glioblastoma cell line containing a TMZ-resistant isogenic variant [32],[61]. In addition, DOX is widely used in chemotherapy and acts on DNA. Chastagner et al. attempted to reduce toxicity and increase permeability using a liposomal encapsulated form. Liposome encapsulation of pegylated forms has been shown to significantly improve penetration across the blood-tumor barrier and preferential deposition in tumoral tissue compared to free DOX in animal tumor models. These studies demonstrated that nanocarriers targeting brain tumors are effective in combination with anthracyclines and RT [62].
MicroRNAs (miRNAs) are 21–25 base-long single-stranded RNA molecules involved in the post-transcriptional regulation of gene expression in eukaryotes. Several attempts have been made to use miRNAs as RSs. MiR-29a is downregulated in radioresistant nasopharyngeal carcinoma (NPC) cells and tissues. Introduction of miR-29a can suppress COL1A1 gene expression and render NPC cells radiosensitive. miR-29a is a determinant of the radiation response in NPC patients, and its induction is considered a therapeutic option to enhance radiosensitivity [63]. MiR-365 has been suggested to be associated with the malignancy of non-small cell lung cancer (NSCLC), and high expression of miR-365 suppresses the development of NSCLC. MiR-365 was confirmed to be positively correlated with the radiosensitivity of NSCLC cells and to target CDC25A to enhance radiosensitivity in vitro and in vivo. This suggests that miR-365 can be used as a radiosensitizer for NSCLC treatment [64]. Jab1/CSN5 functions as an oncoprotein in human cancers and miR-24 inhibits its translation. Analysis of NPC tissue from a patient with NPC showed that miR-24 levels were significantly reduced in recurrent NPCs, and levels of the target Jab1/CSN5 were higher than those of primary NPCs. In addition, miR-24 enhances radiosensitivity and may be useful for treating radioresistant NPCs [65].
Certain natural products have antioxidant properties and different effects on normal and tumor cells where the radiosensitivity of tumor cells and radiotolerance of normal cells may be amplified. Since the RS mechanism of a natural product with low toxicity and antioxidant effect is of interest, we conducted a survey and included a paper; the radiosensitizing ability of natural substances was reported in this survey [66],[67]. These natural products mainly include polyphenols (curcumin, quercetin, kaempferol, ellagic acid, etc.), polysaccharides (Ganoderma lucidum polysaccharides, Wyer, brown algae sulfated dextran, ginseng polysaccharides, etc.), alkaloids (berberine, (−)-Agelamide D, Hamala alkaloids, etc.) and other plant-derived extracts (ginsenoside and emodin) [68]. Radiation toxicity in cancer cells can be selectively enhanced by the regulation of various molecular mechanisms. The direct mechanism of action is that radiation causes an increase in ROS levels in tumors and induces cancer cell death [69]–[71]. Higher endogenous ROS levels in cancer cells make them more vulnerable to ROS induction therapy. Simultaneously, these natural products can also achieve antioxidant function and reduce ROS levels in normal cells, thus achieving radiation protection [66],[72]. The indirect mechanisms mainly include (a) delaying DNA damage repair and inhibiting tumor cell proliferation [73]; (b) regulating non-coding RNA, including miRNA and LNC RNA [66]; (c) regulating the expression of proteins related to radiation resistance, such as anti-apoptotic protein Bcl-2 and pre-apoptotic protein Bax [73]; (d) effects on apoptotic pathways, such as PI3K/Akt, STAT3, p38/ROS/caspase[74]–[76], and selective regulation of the NF-κB pathway and induction of autophagy death [77],[78]; and (e) regulation of key tumor suppressor genes and cytokines [79],[80]. At present, research has proven that these natural products can help overcome drug resistance, increase the therapeutic index, and reduce side effects. However, considering that humans seldom absorb natural products completely, there are still few clinical applications of RT. Further exploration is needed to provide a basis for clinical trials of other combined therapies.
Selectivity description | Material | Condition | Published Date | Ref |
anticancer drug | NE0212 (temozolomide derivative) | glioblastoma | 2020 | [61] |
anticancer drug | doxorubicin (DOX) | brain tumor | 2015 | [62] |
RNA target | miR-29a | nasopharyngeal carcinoma (NPC) | 2019 | [63] |
RNA target | miR-365 | NSCLC | 2019 | [64] |
RNA target | miR-24 | NPC | 2016 | [65] |
natural products | bromelain (BL) | Ehrlich ascites carcinoma (EAC) cell | 2020 | [66] |
natural products | Ellagic Acid (EA) | breast cancer | 2017 | [67] |
natural products | dimethoxycurcumin (curcumin) | lung cancer | 2016 | [69] |
natural products | resveratrol | lung cancer | 2013 | [70] |
natural products | genistein | NSCLC | 2016 | [72] |
natural products | quercetin | keloid | 2018 | [74] |
natural products | luteolin | NSCLC | 2015 | [76] |
natural products | epigallocatechin-3-gallate(EGCG) | breast cancer | 2012 | [78] |
pH value sensitive and folate receptor targeting | CD44 and folate receptor-targeting multi-functional dual drug-loaded NPs | breast cancer | 2021 | [81] |
Through the classification of RSs in the literature, we identified some features of RS selectivity. We classified these sensitizers into active and passive selectivities, according to the principle of selective action. Most passive selective sensitizers take advantage of the fact that particles of a certain size range are easily retained in the tumor tissue structure, through the EPR effect. Active, selective sensitizers achieve selectivity for cancer cells by targeting different proteins or RNAs that exist in the tumor environment and are specifically expressed by the tumor cells.
For RSs with passive selectivity, their selectivity to tumors mainly depends on the pH of the tumor environment, the excessively formed vascular structure, and the hypoxic environment in the tumor center. Through the studies of Liu et al., and Menon et al., we noticed that the slightly acidic character of the tumor environment is mainly used to control the release of functional drugs [42],[43]. RS in hypoxic environments uses a different approach [42],[51],[58]–[60]. Hou et al. studied a supramolecular RT strategy that combined hypoxia-responsive macrocyclic drugs with small-molecule RSs [59]. The selectivity of sensitizers relies on the selective reduction of azo functional groups in hypoxic microenvironments to achieve high intertumoral accumulation, while Nandy et al. used 5-nitroimidazole to exhibit activity in hypoxic environments, as hypoxic cytotoxins are considered to target cancer cells in hypoxic environments. Finally, almost all metal NP composites exploit the characteristics of overformed microvascular structures to achieve selective RS. These metal NPs are sized at the time of synthesis and depend on enhanced permeability to maintain excellent retention in tumor tissue caused by excessive vascularization to achieve tumor tissue selectivity.
RSs with active selectivity mainly target microparticles, such as proteins that are specifically expressed or overexpressed in cancer cells or in the tumor environment, to achieve selectivity to cancer tissue. In the literature we searched, there are many kinds of targets introduced in articles involving targeting receptors [3]–[30]; for example, the nanomaterials studied by Zhang et al., Huang et al., and Mirrahimi et al. all target an FA receptor that is overexpressed on the surface of a majority of cancer cells, such as breast cancer cells. In addition, overexpression of αvβ3 integrin can act as a target in the tumor cell membrane. Wang et al., Pan et al., and Enferadi et al. studied different drugs that target αvβ3 integrin. The RSs of αvβ3 integrins have been proven to have satisfactory cancer-destruction properties and have certain application prospects [21]–[24]. For cancer cells with heterogeneous EGFR levels, such as NSCLC, drugs that target EGFR can significantly reduce the cellular activity of such cancer cells. In addition, for cancers with high expression of PARP enzymes, such as SCLC, drugs targeting PARP enzymes can increase the radiosensitivity of cancer cells. For prostate cancer, prostate-specific membrane antigen (PSMA) is an important target [28]–[30], and RSs that selectively target this receptor can effectively arrest the cancer cell cycle in the G2/M phase, which increases the radioactivity of prostate cancer cells. There is one special case that belongs to both “active” and “passive” at the same time and has been classified as other [81].
With selective RSs, the treatment effect of RT can be enhanced more effectively, and the effect of RSs on normal tissue can be reduced. The main direct advantages that they can bring, whether they are RSs with active or passive selectivity that rely on factors, such as EPR, are as follows:
The immediate advantage is that selective RSs can effectively increase the accumulation of RSs in tumors, and achieve higher retention rates in tumors, thereby increasing the efficiency of RT. For example, Ma et al. investigated the cumulative effect of 64Cu-DOTA-pPD-Gd@C-dots, where the tumor uptake of this sensitizer showed minimal clearance within 24 h of injection and negligible uptake in otherwise normal tissues, demonstrating that this sensitizer could be more suitable for RT.
For active RSs, selective RSs allow for more accurate delivery of RSs to cancer cells and less to normal tissues, thus reducing the impact on normal cells. One of the most popular applications is the use of FA receptors, which are poorly expressed in normal cells but significantly expressed in tumor cells, to achieve the active selectivity of RSs for tumor cells. For example, Huang et al. chose FA as the targeting ligand to create a formulation of cancer-targeting BSANPs as a drug delivery system for PSeD, thus allowing NPs to target FA receptors and precisely select cancer cells. In addition, the most important advantage of active RSs is their cancer-specific properties: RSs, such as the PARP1 enzyme, can target the receptor on cancer cells, which provides a possible way for precise treatment of cancer.
For passive RSs, versatility is the biggest advantage of this type of RS. Since this kind of RS does not target any receptor, and the selectivity of the RS is related to the condition of the tumor tissue, these RSs can be used for the treatment of several types of cancers. In addition, most passive RSs are NPs that can be combined with other drugs. This provides an opportunity to combine different drugs with NPs, which provides great flexibility in treatment plan design.
Although selective RSs have several advantages and may be very useful for cancer treatment, there are some disadvantages that cannot be overlooked. For both active and passive RSs, some are toxic to normal cells, which requires researchers to balance treatment efficacy and damage to normal cells.
For active RSs, in addition to FA receptors, which are common to many cancer cells, drugs that target specific receptors on certain cancer cells have a relatively limited treatment. For example, adotrastuzumab emtansine (T-DM1), in combination with IR, prolongs tumor control in HER2 + target-expressing tumors, but not in target-negative tumors.
For passive RSs, the distribution of RS may affect the treatment efficacy, and this distribution relies on the condition of the tumor tissue. Uneven distribution of RSs may lead to poor treatment efficacy. In addition, the generation of some multi-functionalized NPs can be very complex, which leads to high cost and low production.
At present, the selectivity of RSs is mainly achieved by drug characteristics, external control and corresponding targets.
Due to the increasing research on tumors and the tumor microenvironment in recent years, there has been an increasing number of RSs that achieve selectivity through tumor characteristics. However, these sensitizers are usually metal particles, which are expensive and can remain in the body after treatment. Therefore, RSs that can be controlled externally or on a target are of increasing interest.
RSs that release drugs through external control can deliver other drugs while sensitizing or performing image inspection and other operation, and are suitable for the combined treatment using multiple methods. The main research direction of this kind of selective sensitizer in the future lies in the selection of both the shell and the combined treatment methods.
Radiosensitizing drugs targeting tumor-specific genes are still in their infancy. Since there are not enough studies on the specific effects of target genes, the effects and side effects of the corresponding inhibitory drugs have not been clearly studied. The development direction of targeted drugs includes the principle of action, the study of signaling pathways and the combination of different target genes.
No sophisticated radiological device has a cancer selection system at the cellular level. RSs with tumor selectivity at the cellular level are desirable.
In conclusion, we reviewed nearly ten years of literature on selective RSs and classified the selectivity of different RSs into active and passive selectivities. Among them, sensitizers with active selectivity are characterized by targeting specific or overexpressed receptors in the environment of cancer cells or cancer tissues, whereas sensitizers with passive selectivity use the unique pH environment of cancer tissues, hypoxic environment and overgrown vascular structures to accumulate in cancer tissue. Active selectivity mainly depends on the presence of specific receptors in cancer cells or the tumor microenvironment, whereas passive selectivity mainly depends on factors, such as pH sensitivity and molecular size of the drug itself. Such selective RSs have good prospects in the current development of precision cancer therapy, but there is currently a lack of clinical trials, and their specific practical value needs to be further studied by researchers.
[1] |
Baumann M, Krause M, Overgaard J, et al. (2016) Radiation oncology in the era of precision medicine. Nat Rev Cancer 16: 234-249. https://doi.org/10.1038/nrc.2016.18 ![]() |
[2] |
Komorowska D, Radzik T, Kalenik S, et al. (2022) Natural radiosensitizers in radiotherapy: cancer treatment by combining ionizing radiation with resveratrol. Int J Mol Sci 23: 10627. https://doi.org/10.3390/ijms231810627 ![]() |
[3] |
Zhang J, Liu Y, Wang X, et al. (2020) Nanozyme-incorporated biodegradable bismuth mesoporous radiosensitizer for tumor microenvironment-modulated hypoxic tumor thermoradiotherapy. ACS Appl Mater Interfaces 12: 57768-57781. https://doi.org/10.1021/acsami.0c18853 ![]() |
[4] |
Zhang H, Zhang W, Zhou Y, et al. (2017) Dual functional mesoporous silicon nanoparticles enhance the radiosensitivity of VPA in glioblastoma. Transl Oncol 10: 229-240. https://doi.org/10.1016/j.tranon.2016.12.011 ![]() |
[5] |
Huang Y, Luo Y, Zheng W, et al. (2014) Rational design of cancer-targeted BSA protein nanoparticles as radiosensitizer to overcome cancer radioresistance. ACS Appl Mater Interfaces 6: 19217-19228. https://doi.org/10.1021/am505246w ![]() |
[6] |
Mirrahimi M, Hosseini V, Shakeri-Zadeh A, et al. (2019) Modulation of cancer cells' radiation response in the presence of folate conjugated Au@Fe2O3 nanocomplex as a targeted radiosensitizer. Clin Transl Oncol 21: 479-488. https://doi.org/10.1007/s12094-018-1947-8 ![]() |
[7] |
Nosrati H, Charmi J, Salehiabar M, et al. (2019) Tumor targeted albumin coated bismuth sulfide nanoparticles (Bi2S3) as radiosensitizers and carriers of curcumin for enhanced chemoradiation therapy. ACS Biomater Sci Eng 5: 4416-4424. https://doi.org/10.1021/acsbiomaterials.9b00489 ![]() |
[8] |
Cho WJ, Kessel D, Rakowski J, et al. (2021) Photodynamic therapy as a potent radiosensitizer in head and neck squamous cell carcinoma. Cancers 13: 1193. https://doi.org/10.3390/cancers13061193 ![]() |
[9] |
Wang L, Zhang T, Huo M, et al. (2019) Construction of nucleus-targeting iridium nanocrystals for photonic hyperthermia-synergized cancer radiotherapy. Small 15: e1903254. https://doi.org/10.1002/smll.201903254 ![]() |
[10] |
Enferadi M, Fu SY, Hong JH, et al. (2018) Radiosensitization of ultrasmall GNP-PEG-cRGDfK in ALTS1C1 exposed to therapeutic protons and kilovoltage and megavoltage photons. Int J Radiat Biol 94: 124-136. https://doi.org/10.1080/09553002.2018.1407462 ![]() |
[11] |
Wang J, Pang X, Tan X, et al. (2017) A triple-synergistic strategy for combinational photo/radiotherapy and multi-modality imaging based on hyaluronic acid-hybridized polyaniline-coated WS2 nanodots. Nanoscale 9: 5551-5564. https://doi.org/10.1039/c6nr09219e ![]() |
[12] |
Liu J, Yang Y, Zhu W, et al. (2016) Nanoscale metal-organic frameworks for combined photodynamic & radiation therapy in cancer treatment. Biomaterials 97: 1-9. https://doi.org/10.1016/j.biomaterials.2016.04.034 ![]() |
[13] |
Mayahi S, Neshasteh-Riz A, Pornour M, et al. (2020) Investigation of combined photodynamic and radiotherapy effects of gallium phthalocyanine chloride on MCF-7 breast cancer cells. J Biol Inorg Chem 25: 39-48. https://doi.org/10.1007/s00775-019-01730-w ![]() |
[14] |
Sazgarnia A, Montazerabadi AR, Bahreyni-Toosi MH, et al. (2013) In vitro survival of MCF-7 breast cancer cells following combined treatment with ionizing radiation and mitoxantrone-mediated photodynamic therapy. Photodiagnosis Photodyn Ther 10: 72-78. https://doi.org/10.1016/j.pdpdt.2012.06.001 ![]() |
[15] |
Miyake M, Tanaka N, Hori S, et al. (2019) Dual benefit of supplementary oral 5-aminolevulinic acid to pelvic radiotherapy in a syngenic prostate cancer model. Prostate 79: 340-351. https://doi.org/10.1002/pros.23740 ![]() |
[16] |
Takahashi J, Misawa M, Iwahashi H (2016) Combined treatment with X-ray irradiation and 5-aminolevulinic acid elicits better transcriptomic response of cell cycle-related factors than X-ray irradiation alone. Int J Radiat Biol 92: 774-789. https://doi.org/10.1080/09553002.2016.1230240 ![]() |
[17] |
Laird JH, Lok BH, Ma J, et al. (2018) Talazoparib is a potent radiosensitizer in small cell lung cancer cell lines and xenografts. Clin Cancer Res 24: 5143-5152. https://doi.org/10.1158/1078-0432.CCR-18-0401 ![]() |
[18] |
Li D, Zhao J, Ma J, et al. (2022) GMT8 aptamer conjugated PEGylated Ag@Au core-shell nanoparticles as a novel radiosensitizer for targeted radiotherapy of glioma. Colloids Surf B Biointerfaces 211: 112330. https://doi.org/10.1016/j.colsurfb.2022.112330 ![]() |
[19] |
Jiao X, Yu Y, Meng J, et al. (2019) Dual-targeting and microenvironment-responsive micelles as a gene delivery system to improve the sensitivity of glioma to radiotherapy. Acta Pharm Sin B 9: 381-396. https://doi.org/10.1016/j.apsb.2018.12.001 ![]() |
[20] |
Park M, Kwon J, Shin HJ, et al. (2020) Butyrate enhances the efficacy of radiotherapy via FOXO3A in colorectal cancer patient‑derived organoids. Int J Oncol 57: 1307-1318. https://doi.org/10.3892/ijo.2020.5132 ![]() |
[21] |
Pan W, Gong S, Wang J, et al. (2019) A nuclear-targeted titanium dioxide radiosensitizer for cell cycle regulation and enhanced radiotherapy. Chem Commun 55: 8182-8185. https://doi.org/10.1039/c9cc01651a ![]() |
[22] | Ji F, Sha H, Meng F, et al. (2018) Tumor‑penetrating peptide fused EGFR single‑domain antibody enhances radiation responses following EGFR inhibition in gastric cancer. Oncol Rep 40: 1583-1591. https://doi.org/10.3892/or.2018.6532 |
[23] |
Zeng D, Deng S, Sang C, et al. (2018) Rational design of cancer-targeted selenadiazole derivative as efficient radiosensitizer for precise cancer therapy. Bioconjug Chem 29: 2039-2049. https://doi.org/10.1021/acs.bioconjchem.8b00247 ![]() |
[24] |
Bromma K, Chithrani DB (2020) Advances in gold nanoparticle-based combined cancer therapy. Nanomaterials 10: 1671. https://doi.org/10.3390/nano10091671 ![]() |
[25] |
Koo T, Kim IA (2016) Brain metastasis in human epidermal growth factor receptor 2-positive breast cancer: from biology to treatment. Radiat Oncol J 34: 1-9. https://doi.org/10.3857/roj.2016.34.1.1 ![]() |
[26] |
Nambiar DK, Rajamani P, Deep G, et al. (2015) Silibinin preferentially radiosensitizes prostate cancer by inhibiting DNA repair signaling. Mol Cancer Ther 14: 2722-2734. https://doi.org/10.1158/1535-7163.MCT-15-0348 ![]() |
[27] |
Reda M, Ngamcherdtrakul W, Gu S, et al. (2019) PLK1 and EGFR targeted nanoparticle as a radiation sensitizer for non-small cell lung cancer. Cancer Lett 467: 9-18. https://doi.org/10.1016/j.canlet.2019.09.014 ![]() |
[28] |
Guo XX, Guo ZH, Lu JS, et al. (2021) All-purpose nanostrategy based on dose deposition enhancement, cell cycle arrest, DNA damage, and ROS production as prostate cancer radiosensitizer for potential clinical translation. Nanoscale 13: 14525-14537. https://doi.org/10.1039/d1nr03869a ![]() |
[29] |
Luo D, Johnson A, Wang X, et al. (2020) Targeted radiosensitizers for MR-guided radiation therapy of prostate cancer. Nano Lett 20: 7159-7167. https://doi.org/10.1021/acs.nanolett.0c02487 ![]() |
[30] |
Luo D, Wang X, Zeng S, et al. (2019) Targeted gold nanocluster-enhanced radiotherapy of prostate cancer. Small 15: e1900968. https://doi.org/10.1002/smll.201900968 ![]() |
[31] |
Maeda H, Wu J, Sawa T, et al. (2000) Tumor vascular permeability and the EPR effect in macromolecular therapeutics: a review. J Control Release 65: 271-284. https://doi.org/10.1016/s0168-3659(99)00248-5 ![]() |
[32] |
Hua S, He J, Zhang F, et al. (2021) Multistage-responsive clustered nanosystem to improve tumor accumulation and penetration for photothermal/enhanced radiation synergistic therapy. Biomaterials 268: 120590. https://doi.org/10.1016/j.biomaterials.2020.120590 ![]() |
[33] |
Hatoyama K, Kitamura N, Takano-Kasuya M, et al. (2019) Quantitative analyses of amount and localization of radiosensitizer gold nanoparticles interacting with cancer cells to optimize radiation therapy. Biochem Biophys Res Commun 508: 1093-1100. https://doi.org/10.1016/j.bbrc.2018.12.016 ![]() |
[34] |
Xu X, Chong Y, Liu X, et al. (2019) Multifunctional nanotheranostic gold nanocages for photoacoustic imaging guided radio/photodynamic/photothermal synergistic therapy. Acta Biomater 84: 328-338. https://doi.org/10.1016/j.actbio.2018.11.043 ![]() |
[35] |
Cheng K, Sano M, Jenkins CH, et al. (2018) Synergistically enhancing the therapeutic effect of radiation therapy with radiation activatable and reactive oxygen species-releasing nanostructures. ACS Nano 12: 4946-4958. https://doi.org/10.1021/acsnano.8b02038 ![]() |
[36] |
Zhang XD, Chen J, Min Y, et al. (2014) Metabolizable Bi2Se3 nanoplates: biodistribution, toxicity, and uses for cancer radiation therapy and imaging. Adv Funct Mater 24: 1718-1729. https://doi.org/10.1002/adfm.201302312 ![]() |
[37] |
Zhang XD, Chen J, Luo Z, et al. (2014) Enhanced tumor accumulation of sub-2 nm gold nanoclusters for cancer radiation therapy. Adv Healthc Mater 3: 133-141. https://doi.org/10.1002/adhm.201300189 ![]() |
[38] |
Feng L, Dong Z, Liang C, et al. (2018) Iridium nanocrystals encapsulated liposomes as near-infrared light controllable nanozymes for enhanced cancer radiotherapy. Biomaterials 181: 81-91. https://doi.org/10.1016/j.biomaterials.2018.07.049 ![]() |
[39] |
Hua Y, Wang Y, Kang X, et al. (2021) A multifunctional AIE gold cluster-based theranostic system: tumor-targeted imaging and Fenton reaction-assisted enhanced radiotherapy. J Nanobiotechnol 19: 438. https://doi.org/10.1186/s12951-021-01191-x ![]() |
[40] |
Gao S, Zhang W, Wang R, et al. (2020) Nanoparticles encapsulating nitrosylated maytansine to enhance radiation therapy. ACS Nano 14: 1468-1481. https://doi.org/10.1021/acsnano.9b05976 ![]() |
[41] |
Jung J, Jeong SY, Park SS, et al. (2015) A cisplatin‑incorporated liposome that targets the epidermal growth factor receptor enhances radiotherapeutic efficacy without nephrotoxicity. Int J Oncol 46: 1268-1274. https://doi.org/10.3892/ijo.2014.2806 ![]() |
[42] |
Liu H, Xie Y, Zhang Y, et al. (2017) Development of a hypoxia-triggered and hypoxic radiosensitized liposome as a doxorubicin carrier to promote synergetic chemo-/radiotherapy for glioma. Biomaterials 121: 130-143. https://doi.org/10.1016/j.biomaterials.2017.01.001 ![]() |
[43] |
Menon JU, Kuriakose A, Iyer R, et al. (2017) Dual-drug containing core-shell nanoparticles for lung cancer therapy. Sci Rep 7: 13249. https://doi.org/10.1038/s41598-017-13320-4 ![]() |
[44] |
Zhang Z, Niu X, Feng X, et al. (2021) Construction of a pH/TGase “dual key”-responsive gold nano-radiosensitizer with liver tumor-targeting ability. ACS Biomater Sci Eng 7: 3434-3445. https://doi.org/10.1021/acsbiomaterials.1c00428 ![]() |
[45] |
Lv S, Long W, Chen J, et al. (2020) Dual pH-triggered catalytic selective Mn clusters for cancer radiosensitization and radioprotection. Nanoscale 12: 548-557. https://doi.org/10.1039/c9nr08192e ![]() |
[46] |
Bonnet M, Hong CR, Wong WW, et al. (2018) Next-generation hypoxic cell radiosensitizers: nitroimidazole alkylsulfonamides. J Med Chem 61: 1241-1254. https://doi.org/10.1021/acs.jmedchem.7b01678 ![]() |
[47] |
Kouhsari E, Ghadimi-Daresajini A, Abdollahi H, et al. (2018) The potential roles of bacteria to improve radiation treatment outcome. Clin Transl Oncol 20: 127-139. https://doi.org/10.1007/s12094-017-1701-7 ![]() |
[48] |
Yang S, Han G, Chen Q, et al. (2021) Au-Pt nanoparticle formulation as a radiosensitizer for radiotherapy with dual effects. Int J Nanomedicine 16: 239-248. https://doi.org/10.2147/IJN.S287523 ![]() |
[49] |
Chen J, Chen Q, Liang C, et al. (2017) Albumin-templated biomineralizing growth of composite nanoparticles as smart nano-theranostics for enhanced radiotherapy of tumors. Nanoscale 9: 14826-14835. https://doi.org/10.1039/c7nr05316a ![]() |
[50] |
Ma X, Lee C, Zhang T, et al. (2021) Image-guided selection of Gd@C-dots as sensitizers to improve radiotherapy of non-small cell lung cancer. J Nanobiotechnol 19: 284. https://doi.org/10.1186/s12951-021-01018-9 ![]() |
[51] |
Wang H, Mu X, He H, Zhang XD (2018) Cancer Radiosensitizers. Trends Pharmacol Sci 39: 24-48. https://doi.org/10.1016/j.tips.2017.11.003 ![]() |
[52] |
Kefayat A, Ghahremani F, Motaghi H, et al. (2019) Ultra-small but ultra-effective: Folic acid-targeted gold nanoclusters for enhancement of intracranial glioma tumors' radiation therapy efficacy. Nanomedicine 16: 173-184. https://doi.org/10.1016/j.nano.2018.12.007 ![]() |
[53] | Dorsey JF, Sun L, Joh DY, et al. (2013) Gold nanoparticles in radiation research: potential applications for imaging and radiosensitization. Transl Cancer Res 2: 280-291. https://doi.org/10.3978/j.issn.2218-676X.2013.08.09 |
[54] |
Besse HC, Bos C, Zandvliet MMJM, et al. (2018) Triggered radiosensitizer delivery using thermosensitive liposomes and hyperthermia improves efficacy of radiotherapy: An in vitro proof of concept study. PLoS One 13: e0204063. https://doi.org/10.1371/journal.pone.0204063 ![]() |
[55] |
Tung FI, Zheng LJ, Hou KT, et al. (2020) One-stop radiotherapeutic targeting of primary and distant osteosarcoma to inhibit cancer progression and metastasis using 2DG-grafted graphene quantum dots. Nanoscale 12: 8809-8818. https://doi.org/10.1039/c9nr10823h ![]() |
[56] |
Zhao L, Qiu G, Wang K, et al. (2020) A nano-integrated diagnostic and therapeutic platform with oxidation-reduction reactions in tumor microenvironments. Nanoscale Adv 2: 2192-2202. https://doi.org/10.1039/c9na00786e ![]() |
[57] |
Mehdi Z, Petronek MS, Stolwijk JM, et al. (2021) Utilization of pharmacological ascorbate to enhance hydrogen peroxide-mediated radiosensitivity in cancer therapy. Int J Mol Sci 22: 10880. https://doi.org/10.3390/ijms221910880 ![]() |
[58] |
Pan P, Dong X, Chen Y, et al. (2022) Engineered bacteria for enhanced radiotherapy against breast carcinoma. ACS Nano 16: 801-812. https://doi.org/10.1021/acsnano.1c08350 ![]() |
[59] |
Hou X, Chang YX, Yue YX, et al. (2022) Supramolecular radiosensitizer based on hypoxia-responsive macrocycle. Adv Sci 9: e2104349. https://doi.org/10.1002/advs.202104349 ![]() |
[60] |
Nandy P, Mukherjee A, Pradhan C, et al. (2020) Radio-sensitizing effects of CuII and ZnII complexes of ornidazole: role of nitro radical anion. ACS Omega 5: 25668-25676. https://doi.org/10.1021/acsomega.0c02811 ![]() |
[61] |
Minea RO, Duc TC, Swenson SD, et al. (2020) Developing a clinically relevant radiosensitizer for temozolomide-resistant gliomas. PLoS One 15: e0238238. https://doi.org/10.1371/journal.pone.0238238 ![]() |
[62] |
Chastagner P, Sudour H, Mriouah J, et al. (2015) Preclinical studies of pegylated- and non-pegylated liposomal forms of doxorubicin as radiosensitizer on orthotopic high-grade glioma xenografts. Pharm Res 32: 158-166. https://doi.org/10.1007/s11095-014-1452-x ![]() |
[63] |
Guo Y, Zhai J, Zhang J, et al. (2019) Improved radiotherapy sensitivity of nasopharyngeal carcinoma cells by miR-29-3p targeting COL1A1 3′-UTR. Med Sci Monit 25: 3161-3169. https://doi.org/10.12659/MSM.915624 ![]() |
[64] |
Li H, Jiang M, Cui M, et al. (2019) MiR-365 enhances the radiosensitivity of non-small cell lung cancer cells through targeting CDC25A. Biochem Biophys Res Commun 512: 392-398. https://doi.org/10.1016/j.bbrc.2019.03.082 ![]() |
[65] |
Wang S, Pan Y, Zhang R, et al. (2016) Hsa-miR-24-3p increases nasopharyngeal carcinoma radiosensitivity by targeting both the 3′UTR and 5′UTR of Jab1/CSN5. Oncogene 35: 6096-6108. https://doi.org/10.1038/onc.2016.147 ![]() |
[66] |
Mekkawy MH, Fahmy HA, Nada AS, et al. (2020) Study of the radiosensitizing and radioprotective efficacy of bromelain (a pineapple extract): in vitro and in vivo. Integr Cancer Ther 19: 1534735420950468. https://doi.org/10.1177/1534735420950468 ![]() |
[67] |
Ahire V, Kumar A, Mishra KP, et al. (2017) Ellagic acid enhances apoptotic sensitivity of breast cancer cells to γ-Radiation. Nutr Cancer 69: 904-910. https://doi.org/10.1080/01635581.2017.1339811 ![]() |
[68] |
Yi J, Zhu J, Zhao C, et al. (2021) Potential of natural products as radioprotectors and radiosensitizers: opportunities and challenges. Food Funct 12: 5204-5218. https://doi.org/10.1039/d1fo00525a ![]() |
[69] |
J Jayakumar S, Patwardhan RS, Pal D, et al. (2016) Dimethoxycurcumin, a metabolically stable analogue of curcumin enhances the radiosensitivity of cancer cells: Possible involvement of ROS and thioredoxin reductase. Biochem Biophys Res Commun 478: 446-454. https://doi.org/10.1016/j.bbrc.2016.06.144 ![]() |
[70] |
Luo H, Wang L, Schulte BA, et al. (2013) Resveratrol enhances ionizing radiation-induced premature senescence in lung cancer cells. Int J Oncol 43: 1999-2006. https://doi.org/10.3892/ijo.2013.2141 ![]() |
[71] |
Meza-Menchaca T, Poblete-Naredo I, Albores-Medina A, et al. (2020) Ergosterol peroxide isolated from oyster medicinal mushroom, pleurotus ostreatus (agaricomycetes), potentially induces radiosensitivity in cervical cancer. Int J Med Mushrooms 22: 1109-1119. https://doi.org/10.1615/IntJMedMushrooms.2020036673 ![]() |
[72] |
Liu X, Sun C, Liu B, et al. (2016) Genistein mediates the selective radiosensitizing effect in NSCLC A549 cells via inhibiting methylation of the keap1 gene promoter region. Oncotarget 7: 27267-27279. https://doi.org/10.18632/oncotarget.8403 ![]() |
[73] |
Lin C, Yu Y, Zhao HG, et al. (2012) Combination of quercetin with radiotherapy enhances tumor radiosensitivity in vitro and in vivo. Radiother Oncol 104: 395-400. https://doi.org/10.1016/j.radonc.2011.10.023 ![]() |
[74] | Si LB, Zhang MZ, Han Q, et al. (2018) Sensitization of keloid fibroblasts by quercetin through the PI3K/Akt pathway is dependent on regulation of HIF-1α. Am J Transl Res 10: 4223-4234. https://doi.org/10.4172/2155-9554.1000409 |
[75] |
Yang YP, Chang YL, Huang PI, et al. (2012) Resveratrol suppresses tumorigenicity and enhances radiosensitivity in primary glioblastoma tumor initiating cells by inhibiting the STAT3 axis. J Cell Physiol 227: 976-993. https://doi.org/10.1002/jcp.22806 ![]() |
[76] |
Cho HJ, Ahn KC, Choi JY, et al. (2015) Luteolin acts as a radiosensitizer in non-small cell lung cancer cells by enhancing apoptotic cell death through activation of a p38/ROS/caspase cascade. Int J Oncol 46: 1149-1158. https://doi.org/10.3892/ijo.2015.2831 ![]() |
[77] |
Veeraraghavan J, Natarajan M, Lagisetty P, et al. (2011) Impact of curcumin, raspberry extract, and neem leaf extract on rel protein-regulated cell death/radiosensitization in pancreatic cancer cells. Pancreas 40: 1107-1119. https://doi.org/10.1097/MPA.0b013e31821f677d ![]() |
[78] |
Zhang G, Wang Y, Zhang Y, et al. (2012) Anti-cancer activities of tea epigallocatechin-3-gallate in breast cancer patients under radiotherapy. Curr Mol Med 12: 163-176. https://doi.org/10.2174/156652412798889063 ![]() |
[79] |
Choi C, Cho Y, Son A, et al. (2020) Therapeutic potential of (-)-agelamide d, a diterpene alkaloid from the marine sponge Agelas sp., as a natural radiosensitizer in hepatocellular carcinoma models. Mar Drugs 18: 500. https://doi.org/10.3390/md18100500 ![]() |
[80] |
Kang Q, Zhang X, Cao N, et al. (2019) EGCG enhances cancer cells sensitivity under 60Coγ radiation based on miR-34a/Sirt1/p53. Food Chem Toxicol 133: 110807. https://doi.org/10.1016/j.fct.2019.110807 ![]() |
[81] |
Askar MA, Thabet NM, El-Sayyad GS, et al. (2021) Dual hyaluronic acid and folic acid targeting pH-sensitive multifunctional 2DG@DCA@MgO-nano-core-shell-radiosensitizer for breast cancer therapy. Cancers 13: 5571. https://doi.org/10.3390/cancers13215571 ![]() |
1. | Das Salini, Thakur Debanjan, Sengupta Debomita, Mukherjee Sutapa, Restoration of Radiosensitivity by Soya Isoflavone Genistein is Accomplished by Facilitating DNA Damage Response in Radioresistant Cervical Cancer in vitro, 2024, 2588-9273, 10.4103/jrcr.jrcr_68_23 |
Selectivity Mechanism | RS | Condition | Published Date | Ref |
Folic acid (FA) receptor | a biodegradable nanocomposite BiPt-folic acid-modified amphiphilic polyethylene glycol (BiPt-PFA) | breast cancer | 2020 | [3] |
FA receptor | a dual functional mesoporous silica nanoparticle (MSN) formulation of the valproic acid (VPA) | glioblastoma | 2017 | [4] |
FA receptor | FA-BSANP | cervical cancer | 2014 | [5] |
FA receptor | Au@Fe2O3 NPs modified with FA | KB cells(papilloma) | 2019 | [6] |
FA receptor | albumin coated bismuth sulfide NPs | breast cancer | 2019 | [7] |
Photosensitizer (BPD) | benzoporphyrin derivative (BPD) | head and neck squamous cell carcinoma (HNSCC) | 2021 | [8] |
αvβ3 integrin | ultrasmall iridium nanocrystals (Ir-RGD-TAT (Ir-R/T) NCs) | breast cancer | 2019 | [9] |
αvβ3 integrin | GNP-PEG-cRGDfK | glioma | 2018 | [10] |
Photosensitizer (Ce6) | hyaluronic acid (HA), polyaniline (PANI), WS2 nanodots (WS2), and chlorin e6 (Ce6) into a single nanoplatform (HA-WS2@PANI/Ce6) | breast cancer | 2017 | [11] |
Photosensitizer (TCPP) | Hafnium (Hf4+) and tetrakis (4-carboxyphenyl) porphyrin (TCPP) | breast cancer | 2016 | [12] |
Photosensitizer (GaPcCl) | gallium phthalocyanine chloride (GaPcCl) | breast cancer | 2020 | [13] |
Photosensitizer (MTX) | Mitoxantrone (MTX) | melanoma | 2013 | [14] |
Photosensitizer (5-ALA) | 5-aminolevulinic acid (5-ALA) | prostate cancer | 2019 | [15] |
PARA | Talazoparib | small cell lung cancer (SCLC) | 2018 | [17] |
αvβ3 integrin | nucleus-targeting MTiO2 NPs (MTiO2(SN-38)-TAT-RGD) | breast cancer | 2019 | [21] |
αvβ3 integrin | antiEGFRiRGD | gastric cancer | 2018 | [22] |
αvβ3 integrin | RGD peptide has been covalently conjugated to selenadiazole derivative (RGD-SeD) | liver cancer | 2018 | [23] |
αvβ3 integrin | Gold NP | prostate cancer | 2020 | [24] |
EGFR | lapatinib | breast cancer | 2016 | [25] |
EGFR | silibinin | prostate cancer | 2015 | [26] |
EGFR | C-siPLK1 NPs | non-small cell lung cancer (NSCLC) | 2019 | [27] |
PSMA | PSMA-targeted DTX-loaded Au NPs | prostate cancer(Pca) | 2021 | [28] |
PSMA | AuNPs | prostate cancer | 2020 | [29] |
PSMA | gold-based radiation sensitizer | prostate cancer | 2019 | [30] |
Selectivity Mechanism | RS | Condition | Publish Date | Ref |
EPR Effect | multistage-responsive Cs–Au-ICG NPs | breast cancer | 2021 | [32] |
EPR Effect | gold nanoparticles (AuNP-PEG-HER2ab) | human ovarian cancer | 2019 | [33] |
EPR Effect | hyaluronic acid modified Au nanocages (AuNCs-HA) | breast cancer | 2019 | [34] |
EPR Effect | dumbbell-like Au-TiO2 nanoparticles (DATs) | breast cancer | 2018 | [35] |
EPR Effect | sub-2 nm gold nanoclusters | cervical cancer | 2014 | [36] |
EPR Effect | Bi2Se3 | cervical cancer | 2014 | [37] |
EPR Effect | iridium nanocrystals (IrNCs) | breast cancer | 2018 | [38] |
EPR Effect | DM1-NO PLGA NPs | NSCLC | 2020 | [40] |
EPR Effect | cisdiamminedichloroplatinum(Ⅱ) (cisplatin, CDDP) | NSCLC | 2015 | [41] |
Hypoxic condition toxic | a hypoxic RS-prodrug liposome (MLP) | glioma | 2017 | [42] |
PH-Value Sensitive | stimuli-responsive core-shell NP system | lung cancer | 2017 | [43] |
PH-Value Sensitive | Mn clusters | breast cancer | 2020 | [45] |
Hypoxic condition toxic | nitroimidazole alkylsulfonamides (2-nitroimidazole analogues,7 and 19 are effective) | colorectal carcinoma | 2018 | [46] |
EPR Effect | bacteria: 1. Escherichia coli strain K-12, Salmonella typhimurium ΔppGpp (S.t ΔppGpp); 2. lipid mutants Salmonella strains (Salmonella YS1456 and YS1646) Nanoparticles:gold (Au), gadolinium (Gd), iron (Fe), bismote (Bi), titanium (Ti), and hafnium (Hf) |
colon cancer | 2018 | [47] |
EPR Effect | Au-Pt NPs | HUEVC; breast cancer | 2021 | [48] |
EPR Effect | BSA-Au-MnO2 composite NPs | breast cancer | 2017 | [49] |
EPR Effect | Gd@C-dots | NSCLC | 2021 | [50] |
EPR Effect | cetuximab-functionalized gold NPs | HNSCC | 2018 | [51] |
Hypoxic condition toxic | UCHM (upconversion nanoparticle (UCNP) core located within the hollow core of an outer mesoporous silica (HMs) shells) | hela cells | 2018 | [51] |
EPR Effect | folic acid and BSA decorated gold nanoclusters | intracranial glioma tumors | 2019 | [52] |
EPR Effect | gold nanoparticles (GNPs) | brain tumor | 2013 | [53] |
EPR Effect | ThermoDox or doxorubicin (DOX) | human fibrosarcoma | 2018 | [54] |
EPR Effect | 2DG-grafted GQDs | Osteosarcoma (OS) | 2020 | [55] |
EPR Effect | MnO2-HA@H2PtCl6 (MHP) | colon cancer | 2020 | [56] |
PH-Value Sensitive | pharmacological ascorbate | glioblastoma, lung cancer, pancreatic cancer | 2021 | [57] |
Hypoxic condition toxic | integrated nanosystem (Bac@BNP) | breast cancer | 2022 | [58] |
Hypoxic condition toxic | CAC4A+AQ4N | breast cancer | 2022 | [59] |
Hypoxic condition toxic | CuII and ZnII Complexes | lung cancer | 2020 | [60] |
Selectivity description | Material | Condition | Published Date | Ref |
anticancer drug | NE0212 (temozolomide derivative) | glioblastoma | 2020 | [61] |
anticancer drug | doxorubicin (DOX) | brain tumor | 2015 | [62] |
RNA target | miR-29a | nasopharyngeal carcinoma (NPC) | 2019 | [63] |
RNA target | miR-365 | NSCLC | 2019 | [64] |
RNA target | miR-24 | NPC | 2016 | [65] |
natural products | bromelain (BL) | Ehrlich ascites carcinoma (EAC) cell | 2020 | [66] |
natural products | Ellagic Acid (EA) | breast cancer | 2017 | [67] |
natural products | dimethoxycurcumin (curcumin) | lung cancer | 2016 | [69] |
natural products | resveratrol | lung cancer | 2013 | [70] |
natural products | genistein | NSCLC | 2016 | [72] |
natural products | quercetin | keloid | 2018 | [74] |
natural products | luteolin | NSCLC | 2015 | [76] |
natural products | epigallocatechin-3-gallate(EGCG) | breast cancer | 2012 | [78] |
pH value sensitive and folate receptor targeting | CD44 and folate receptor-targeting multi-functional dual drug-loaded NPs | breast cancer | 2021 | [81] |
Selectivity Mechanism | RS | Condition | Published Date | Ref |
Folic acid (FA) receptor | a biodegradable nanocomposite BiPt-folic acid-modified amphiphilic polyethylene glycol (BiPt-PFA) | breast cancer | 2020 | [3] |
FA receptor | a dual functional mesoporous silica nanoparticle (MSN) formulation of the valproic acid (VPA) | glioblastoma | 2017 | [4] |
FA receptor | FA-BSANP | cervical cancer | 2014 | [5] |
FA receptor | Au@Fe2O3 NPs modified with FA | KB cells(papilloma) | 2019 | [6] |
FA receptor | albumin coated bismuth sulfide NPs | breast cancer | 2019 | [7] |
Photosensitizer (BPD) | benzoporphyrin derivative (BPD) | head and neck squamous cell carcinoma (HNSCC) | 2021 | [8] |
αvβ3 integrin | ultrasmall iridium nanocrystals (Ir-RGD-TAT (Ir-R/T) NCs) | breast cancer | 2019 | [9] |
αvβ3 integrin | GNP-PEG-cRGDfK | glioma | 2018 | [10] |
Photosensitizer (Ce6) | hyaluronic acid (HA), polyaniline (PANI), WS2 nanodots (WS2), and chlorin e6 (Ce6) into a single nanoplatform (HA-WS2@PANI/Ce6) | breast cancer | 2017 | [11] |
Photosensitizer (TCPP) | Hafnium (Hf4+) and tetrakis (4-carboxyphenyl) porphyrin (TCPP) | breast cancer | 2016 | [12] |
Photosensitizer (GaPcCl) | gallium phthalocyanine chloride (GaPcCl) | breast cancer | 2020 | [13] |
Photosensitizer (MTX) | Mitoxantrone (MTX) | melanoma | 2013 | [14] |
Photosensitizer (5-ALA) | 5-aminolevulinic acid (5-ALA) | prostate cancer | 2019 | [15] |
PARA | Talazoparib | small cell lung cancer (SCLC) | 2018 | [17] |
αvβ3 integrin | nucleus-targeting MTiO2 NPs (MTiO2(SN-38)-TAT-RGD) | breast cancer | 2019 | [21] |
αvβ3 integrin | antiEGFRiRGD | gastric cancer | 2018 | [22] |
αvβ3 integrin | RGD peptide has been covalently conjugated to selenadiazole derivative (RGD-SeD) | liver cancer | 2018 | [23] |
αvβ3 integrin | Gold NP | prostate cancer | 2020 | [24] |
EGFR | lapatinib | breast cancer | 2016 | [25] |
EGFR | silibinin | prostate cancer | 2015 | [26] |
EGFR | C-siPLK1 NPs | non-small cell lung cancer (NSCLC) | 2019 | [27] |
PSMA | PSMA-targeted DTX-loaded Au NPs | prostate cancer(Pca) | 2021 | [28] |
PSMA | AuNPs | prostate cancer | 2020 | [29] |
PSMA | gold-based radiation sensitizer | prostate cancer | 2019 | [30] |
Selectivity Mechanism | RS | Condition | Publish Date | Ref |
EPR Effect | multistage-responsive Cs–Au-ICG NPs | breast cancer | 2021 | [32] |
EPR Effect | gold nanoparticles (AuNP-PEG-HER2ab) | human ovarian cancer | 2019 | [33] |
EPR Effect | hyaluronic acid modified Au nanocages (AuNCs-HA) | breast cancer | 2019 | [34] |
EPR Effect | dumbbell-like Au-TiO2 nanoparticles (DATs) | breast cancer | 2018 | [35] |
EPR Effect | sub-2 nm gold nanoclusters | cervical cancer | 2014 | [36] |
EPR Effect | Bi2Se3 | cervical cancer | 2014 | [37] |
EPR Effect | iridium nanocrystals (IrNCs) | breast cancer | 2018 | [38] |
EPR Effect | DM1-NO PLGA NPs | NSCLC | 2020 | [40] |
EPR Effect | cisdiamminedichloroplatinum(Ⅱ) (cisplatin, CDDP) | NSCLC | 2015 | [41] |
Hypoxic condition toxic | a hypoxic RS-prodrug liposome (MLP) | glioma | 2017 | [42] |
PH-Value Sensitive | stimuli-responsive core-shell NP system | lung cancer | 2017 | [43] |
PH-Value Sensitive | Mn clusters | breast cancer | 2020 | [45] |
Hypoxic condition toxic | nitroimidazole alkylsulfonamides (2-nitroimidazole analogues,7 and 19 are effective) | colorectal carcinoma | 2018 | [46] |
EPR Effect | bacteria: 1. Escherichia coli strain K-12, Salmonella typhimurium ΔppGpp (S.t ΔppGpp); 2. lipid mutants Salmonella strains (Salmonella YS1456 and YS1646) Nanoparticles:gold (Au), gadolinium (Gd), iron (Fe), bismote (Bi), titanium (Ti), and hafnium (Hf) |
colon cancer | 2018 | [47] |
EPR Effect | Au-Pt NPs | HUEVC; breast cancer | 2021 | [48] |
EPR Effect | BSA-Au-MnO2 composite NPs | breast cancer | 2017 | [49] |
EPR Effect | Gd@C-dots | NSCLC | 2021 | [50] |
EPR Effect | cetuximab-functionalized gold NPs | HNSCC | 2018 | [51] |
Hypoxic condition toxic | UCHM (upconversion nanoparticle (UCNP) core located within the hollow core of an outer mesoporous silica (HMs) shells) | hela cells | 2018 | [51] |
EPR Effect | folic acid and BSA decorated gold nanoclusters | intracranial glioma tumors | 2019 | [52] |
EPR Effect | gold nanoparticles (GNPs) | brain tumor | 2013 | [53] |
EPR Effect | ThermoDox or doxorubicin (DOX) | human fibrosarcoma | 2018 | [54] |
EPR Effect | 2DG-grafted GQDs | Osteosarcoma (OS) | 2020 | [55] |
EPR Effect | MnO2-HA@H2PtCl6 (MHP) | colon cancer | 2020 | [56] |
PH-Value Sensitive | pharmacological ascorbate | glioblastoma, lung cancer, pancreatic cancer | 2021 | [57] |
Hypoxic condition toxic | integrated nanosystem (Bac@BNP) | breast cancer | 2022 | [58] |
Hypoxic condition toxic | CAC4A+AQ4N | breast cancer | 2022 | [59] |
Hypoxic condition toxic | CuII and ZnII Complexes | lung cancer | 2020 | [60] |
Selectivity description | Material | Condition | Published Date | Ref |
anticancer drug | NE0212 (temozolomide derivative) | glioblastoma | 2020 | [61] |
anticancer drug | doxorubicin (DOX) | brain tumor | 2015 | [62] |
RNA target | miR-29a | nasopharyngeal carcinoma (NPC) | 2019 | [63] |
RNA target | miR-365 | NSCLC | 2019 | [64] |
RNA target | miR-24 | NPC | 2016 | [65] |
natural products | bromelain (BL) | Ehrlich ascites carcinoma (EAC) cell | 2020 | [66] |
natural products | Ellagic Acid (EA) | breast cancer | 2017 | [67] |
natural products | dimethoxycurcumin (curcumin) | lung cancer | 2016 | [69] |
natural products | resveratrol | lung cancer | 2013 | [70] |
natural products | genistein | NSCLC | 2016 | [72] |
natural products | quercetin | keloid | 2018 | [74] |
natural products | luteolin | NSCLC | 2015 | [76] |
natural products | epigallocatechin-3-gallate(EGCG) | breast cancer | 2012 | [78] |
pH value sensitive and folate receptor targeting | CD44 and folate receptor-targeting multi-functional dual drug-loaded NPs | breast cancer | 2021 | [81] |