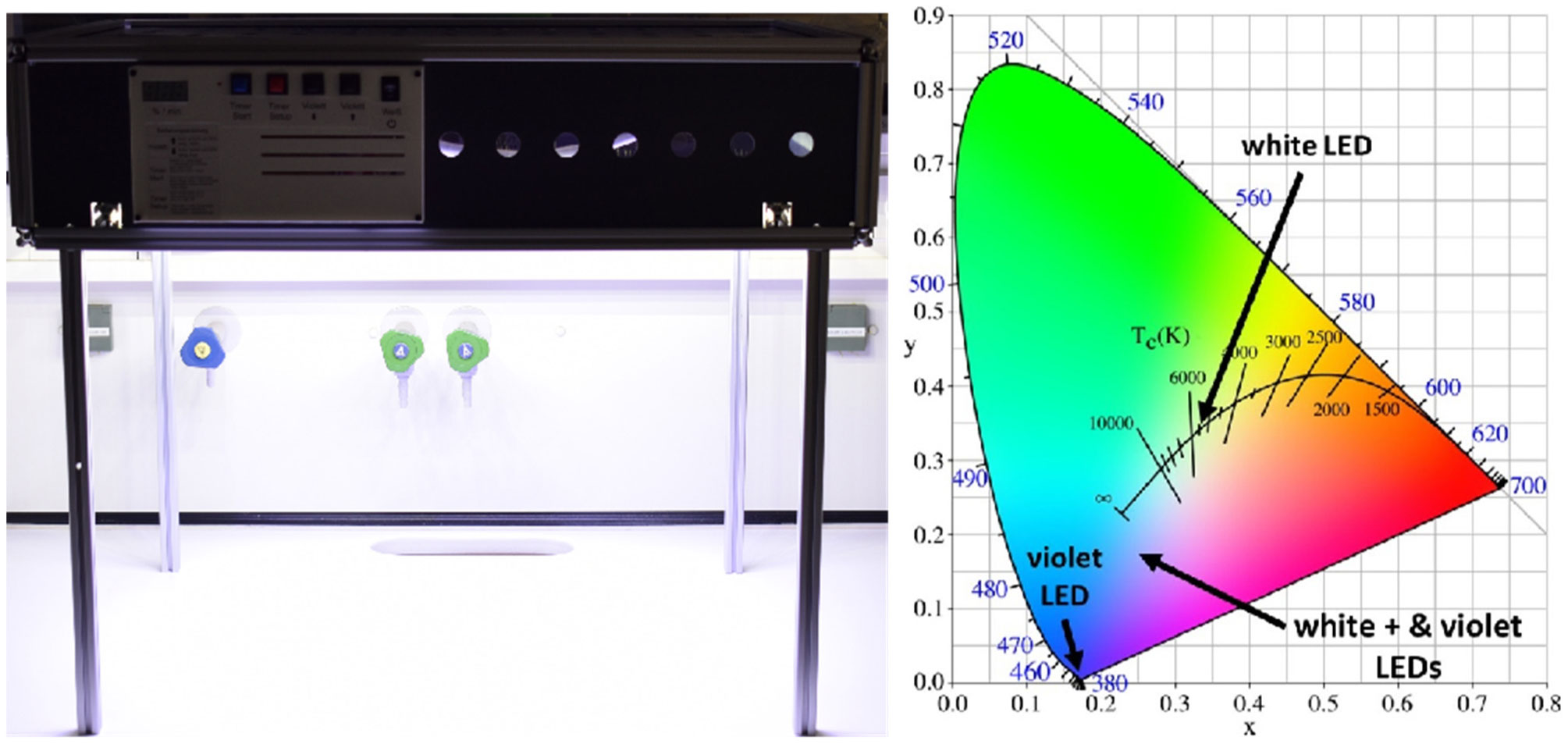
The spread of infections, as in the coronavirus pandemic, leads to the desire to perform disinfection measures even in the presence of humans. UVC radiation is known for its strong antimicrobial effect, but it is also harmful to humans. Visible light, on the other hand, does not affect humans and laboratory experiments have already demonstrated that intense visible violet and blue light has a reducing effect on bacteria and viruses. This raises the question of whether the development of pathogen-reducing illumination is feasible for everyday applications. For this purpose, a lighting device with white and violet LEDs is set up to illuminate a work surface with 2,400 lux of white light and additionally with up to 2.5 mW/cm2 of violet light (405 nm). Staphylococci are evenly distributed on the work surface and the decrease in staphylococci concentration is observed over a period of 46 hours. In fact, the staphylococci concentration decreases, but with the white illumination, a 90% reduction occurs only after 34 hours; with the additional violet illumination the necessary irradiation time is shortened to approx. 3.5 hours. Increasing the violet component probably increases the disinfection effect, but the color impression moves further away from white and the low disinfection durations of UVC radiation can nevertheless not be achieved, even with very high violet emissions.
Citation: Martin Hessling, Tobias Meurle, Katharina Hoenes. Surface disinfection with white-violet illumination device[J]. AIMS Bioengineering, 2022, 9(2): 93-101. doi: 10.3934/bioeng.2022008
[1] | Nikolaos Giormezis, Konstantinos Papakonstantinou, Fevronia Kolonitsiou, Eleanna Drougka, Antigoni Foka, Styliani Sarrou, Evangelos D. Anastassiou, Efthimia Petinaki, Iris Spiliopoulou . Biofilm synthesis and its relationship with genetic characteristics in clinical methicillin-resistant staphylococci. AIMS Bioengineering, 2015, 2(4): 375-386. doi: 10.3934/bioeng.2015.4.375 |
[2] | Simge Çelebi, Mert Burkay Çöteli . Red and white blood cell classification using Artificial Neural Networks. AIMS Bioengineering, 2018, 5(3): 179-191. doi: 10.3934/bioeng.2018.3.179 |
[3] | Letizia Fracchia, Jareer J. Banat, Massimo Cavallo, Chiara Ceresa, Ibrahim M. Banat . Potential therapeutic applications of microbial surface-active compounds. AIMS Bioengineering, 2015, 2(3): 144-162. doi: 10.3934/bioeng.2015.3.144 |
[4] | Jéssica de Oliveira Rossi, Gabriel Tognon Rossi, Maria Eduarda Côrtes Camargo, Rogerio Leone Buchaim, Daniela Vieira Buchaim . Effects of the association between hydroxyapatite and photobiomodulation on bone regeneration. AIMS Bioengineering, 2023, 10(4): 466-490. doi: 10.3934/bioeng.2023027 |
[5] | Mohamed Neifar, Rim Chatter, Habib Chouchane, Raya Genouiz, Atef Jaouani, Ahmed Slaheddine Masmoudi, Ameur Cherif . Optimization of enzymatic saccharification of Chaetomorpha linum biomass for the production of macroalgae-based third generation bioethanol. AIMS Bioengineering, 2016, 3(3): 400-411. doi: 10.3934/bioeng.2016.3.400 |
[6] | Frank B Dazzo, Youssef G Yanni, Ashley Jones, Abdelgawad Y Elsadany . CMEIAS bioimage informatics that define the landscape ecology of immature microbial biofilms developed on plant rhizoplane surfaces. AIMS Bioengineering, 2015, 2(4): 469-486. doi: 10.3934/bioeng.2015.4.469 |
[7] | Satoshi Migita, Kunitaka Araki . Effect of nanometer scale surface roughness of titanium for osteoblast function. AIMS Bioengineering, 2017, 4(1): 162-170. doi: 10.3934/bioeng.2017.1.162 |
[8] | N. Supraja, T.N.V.K.V. Prasad, M. Soundariya, R. Babujanarthanam . Synthesis, characterization and dose dependent antimicrobial and anti-cancerous activity of phycogenic silver nanoparticles against human hepatic carcinoma (HepG2) cell line. AIMS Bioengineering, 2016, 3(4): 425-440. doi: 10.3934/bioeng.2016.4.425 |
[9] | Natalia Fullana, Victoria Braña, Juan José Marizcurrena, Danilo Morales, Jean-Michel Betton, Mónica Marín, Susana Castro-Sowinski . Identification, recombinant production and partial biochemical characterization of an extracellular cold-active serine-metalloprotease from an Antarctic Pseudomonas isolate. AIMS Bioengineering, 2017, 4(3): 386-401. doi: 10.3934/bioeng.2017.3.386 |
[10] | Sven Sölmann, Anke Rattenholl, Hannah Blattner, Guido Ehrmann, Frank Gudermann, Dirk Lütkemeyer, Andrea Ehrmann . Mammalian cell adhesion on different 3D printed polymers with varying sterilization methods and acidic treatment. AIMS Bioengineering, 2021, 8(1): 25-35. doi: 10.3934/bioeng.2021004 |
The spread of infections, as in the coronavirus pandemic, leads to the desire to perform disinfection measures even in the presence of humans. UVC radiation is known for its strong antimicrobial effect, but it is also harmful to humans. Visible light, on the other hand, does not affect humans and laboratory experiments have already demonstrated that intense visible violet and blue light has a reducing effect on bacteria and viruses. This raises the question of whether the development of pathogen-reducing illumination is feasible for everyday applications. For this purpose, a lighting device with white and violet LEDs is set up to illuminate a work surface with 2,400 lux of white light and additionally with up to 2.5 mW/cm2 of violet light (405 nm). Staphylococci are evenly distributed on the work surface and the decrease in staphylococci concentration is observed over a period of 46 hours. In fact, the staphylococci concentration decreases, but with the white illumination, a 90% reduction occurs only after 34 hours; with the additional violet illumination the necessary irradiation time is shortened to approx. 3.5 hours. Increasing the violet component probably increases the disinfection effect, but the color impression moves further away from white and the low disinfection durations of UVC radiation can nevertheless not be achieved, even with very high violet emissions.
For over 2 years, the coronavirus pandemic with its 6 million fatalities so far [1], has dominated global events, sometimes creating the impression that it is the only relevant infectious disease. However, in 2019 alone, there were also almost 5 million deaths related to antibiotic-resistant bacteria [2]. This emphasizes the importance of infectious diseases and that this threat will not disappear even if the current pandemic ends.
In addition to medications, vaccinations and personal protective equipment, disinfection measures are an important element in the fight against all infections. Irradiation with ultraviolet radiation in the UVC spectral range of 200–280 nm is one of the oldest and most effective disinfection techniques [3], which can quickly reduce pathogens by several orders of magnitude, even at very low irradiation doses [4],[5]. The effect is based on the destruction of DNA and RNA of bacteria, fungi and viruses [3]–[5] including coronaviruses like SARS-CoV-2 [4],[6]–[8]. Unfortunately, radiation of these wavelengths also cause harm to human DNA, which is why UVC radiation should not be applied in the presence of humans.
However, the development of LED technology in recent years, has made another almost unknown disinfection approach possible: irradiation with visible violet or blue light (instead of ultraviolet radiation). This visible light is absorbed by naturally present endogenous photosensitizers such as porphyrins or flavins, which have their absorption maxima in the violet and blue spectral range, respectively. During this absorption the photosensitizer molecule is transferred from its singlet ground state to an excited singlet state. This high energy state can decay be immediate emission of a fluorescence photon back to its ground state or it can reach a high energy triplet state. In the presence of oxygen two different types of chemical reactions that generate reactive oxygen species (ROS) are possible. Type I reactions lead to the formation of OH*, O2*− or H2O2 by electron transfer and type II reactions generate 1O2 by energy transfer. All of these radicals damage cell structures such as DNA, proteins or membranes [9]–[15] and can thus lead to cell death. If the irradiation dose is high enough, visible light appears to be effective against bacteria, fungi and even viruses [16]–[18] without endangering humans or human cells [19],[20].
White light also exhibits an antimicrobial activity, as it has blue emission components [21]–[24]. However, the effect is weak and a reduction of 90% takes at least many hours or even days. It is difficult to achieve a significant microbial reduction in a short time, with a white illumination. In the past we have already been able to demonstrate successful bacterial reduction with white illumination [24]. However the setup was performed in a laboratory environment with a very high illuminance of about 200,000 lux, which is way above typical indoor illumination levels. To reach more realistic use case scenarios, the antimicrobial effect of white illumination can be amplified by adding violet emissions [21],[24].
Therefore, this study will investigate to what extent bacterial reduction is possible under more realistic lighting conditions, by applying both white and violet radiation simultaneously, and how much this affects the color temperature of the illumination. The experiments will be conducted on Staphylococcus carnosus, as this non-pathogenic staphylococcal strain is similarly photosensitive as the pathogenic Staphylococcus aureus [25]. In addition, staphylococci also appear to be roughly similar to coronaviruses concerning light sensitivity [26]. Some manufacturers of white-violet LED lights already claim that their products can reduce coronaviruses by several orders of magnitude [27], which is why scientifically verifying this statement is of interest.
To illuminate an area of 60 × 40 cm2, 6 × 4 white LEDs type PM2E-1LWE of Prolight Opto (Taoyuan City, Taiwan) and 6 × 4 violet 405 nm LEDs type PK2B-3JLE-GNVL of Prolight Opto were installed on a copper board at a height of approximately 50 cm above a work surface to be disinfected. The white and violet irradiation could be switched on independently. Dimming was also possible, but in all experiments presented here, both types of LED were operated at maximum or switched off. In the setting demonstrated on the left side of Figure 1, both LED types were operated at their maximum intensities.
The illuminance achieved with the white LEDs was 2,400 lux at a color temperature of 5600 K and a color rendering index of 74. The violet LEDs provided an irradiance of 2.5 mW/cm2 @405 nm. The CIE (Commission Internationale de l'Éclairage) color coordinates of the combined white and violet lighting are illustrated in Figure 1 (right). Illuminance and irradiance below the luminaire were homogeneous with a deviation of +/− 10 % of the average values.
The test organism in this study was Staphylococcus carnosus (DSM 20501), which was provided by Deutsche Sammlung von Mikroorganismen und Zellkulturen (Brauschweig, Germany). The application of this bacterium facilitated the investigation as it is non-pathogenic, but exhibits a photosensitivity comparable to that of S. aureus [25], which is one of the most important and deadliest bacterial pathogens [2]. S. carnosus was cultivated in M92 medium up to an optical density of about 0.2. This corresponded to a concentration of approximately 3 × 107 colony forming units (cfu)/ml. The bacterial culture was then diluted 1 : 1000 in phosphate buffered saline (PBS).
Before applying this bacterial solution, the work surface, glazed stoneware of WALDNER Laboreinrichtungen (Wangen, Germany), was disinfected by a 70% ethanol solution and UVC irradiation. To obtain a homogenous distribution of bacteria on the work surface, the following procedure was performed: Two industrial paper towels of Glaeser, (Ulm, Germany) were placed side by side on the work surface and a Pasteur pipette was used to spread approximately 7 ml of bacterial solution evenly on each towel before the towels were manually flattened with sterile gloves. After about 1 hour the towel was dry and could be removed.
Bacterial concentration sampling was performed at predefined time intervals of 0 h, 12 h, 24 h, 36 h and 46 h and at predefined positions with caso contact agar plates of VWR (Darmstadt, Germany) under different illumination conditions (dark, white, white + violet). For each illumination situation 5 runs were performed, typically using 3 contact plates for each sampling, resulting in a total of about 250 analyzed contact agar plates.
The plates were cultivated at 37 °C in an incubator for about 24 h, before the number of staphylococcal colonies were counted. Colonies that seemed to be caused by other microorganisms, due to differences in shape, size or color, were ignored.
The time course of the bacterial reduction is given in Figure 2. Even without illumination, the staphylococcal concentration decreases. Extrapolation of the trend line leads to an expected decrease of 90% (1 log level) after 86 hours. For white illumination with 2,400 lux and a color temperature of 5600 K, the bacterial reduction progresses more quickly. After 34 hours, only 10% of the bacteria are still left. The combination of white and violet lighting is notably faster in reduction of bacterial load. A 90% staphylococcal reduction is achieved here after less than 3.5 hours. However, as can already be perceived in Figure 1, the illumination no longer appears properly white and the violet portion is recognizable.
It turns out that even the (pure) white illumination causes a noticeable reduction of bacteria. Staphylococci are inactivated significantly faster than in the dark. However, the effect is rather small under the relatively bright lighting of 2,400 lux. Many workplaces are less brightly lit. The international standard EN 12464-1:2021 (“Light and lighting-Lighting of workplaces-Part 1: Indoor workplaces”) specifies an illuminance of only 500 lux for many office workplaces, but also for lecture halls and classrooms. Even for medical examination rooms the imposed illuminance is only 1,000 lux [29].
The situation is different for operating theatres. Illuminance levels of 10,000 to 100,000 lux are demanded here [29]. This is more than four times brighter than the test lighting studied. Therefore, a 90% bacterial reduction might be achieved within 1 to 10 hours of pure white lighting with illuminance levels between 100,000 and 10,000 lux, respectively.
In combination with the violet LEDs, the antimicrobial effect of the presented test illumination is about 10 times stronger than with pure white light (2,400 lux) alone, but 3.5 hours of irradiation are still required for a 90% bacterial reduction. This is still very long compared to typical UVC disinfection durations of minutes to seconds [5]–[8],[30]–[39]. However, for less time-critical applications, such as the disinfection of work areas or rooms overnight, such irradiation with white-violet light is conceivable and in contrast to UVC emitters, without any relevant risk to humans.
A question is whether the violet emission can be raised even further to increase the disinfection effect. This is possible in principle, but even with a tenfold increase in the violet radiation, a 90% reduction of bacteria would probably still take about 20 minutes. At the same time, the color impression of the illumination would move even further away from white, with the risk that this would be unpleasant for people. A potential blue light hazard would also have to be discussed.
In the introduction it was already mentioned that there exist white-violet luminaires, which are advertised by manufacturers with an antiviral effect [27]. In fact, scientific studies show that visible violet light even inactivates coronaviruses [18]. Many of the investigations appear to have been performed (unintentionally) with additional external photosensitizers, representing conditions differing from everyday settings, but not all of them [18]. In our own study without external photosensitizers, we achieved a 90% reduction of (bovine) coronaviruses with a 405 nm irradiation dose of 57.5 J/cm2 [26]. This dose would be reached after 6.4 hours of violet irradiation with the illumination setup presented above. Thus, similar to bacteria, reduction by visible light is also possible for (corona-) viruses, but on a time scale of hours that depends strongly on the intensity of white and violet illumination.
The light of white LEDs exhibits a weak antimicrobial effect due to its blue content. The brighter the blue emissions are, the stronger is the antimicrobial effect. Warm white LEDs emit a large proportion of green, yellow and red light, to which the human eye is very sensitive. However, this light is not absorbed by the relevant microbial photosensitizers and hardly contributes to photoinactivation of microorganisms. The weak blue emissions of warm-white LEDs therefore lead to only a modest antimicrobial effect. Cold-white LEDs have a strong blue component, but the eye is relatively insensitive in this spectral range, which is why cold-white LEDs appear darker than warm-white LEDs at the same total radiant power. Therefore, at the same brightness perception of a user, the blue component absorbable by microbial photosensitizers and thus the antimicrobial effect is much stronger with cold-white LEDs.
Combining white light with visible violet light can significantly increase the antimicrobial effect compared to a pure white illumination, but under realistic conditions it is impossible to achieve disinfection times equivalent to those of UVC irradiation.
The test organism investigated here was only a single staphylococcal strain, but firstly, staphylococcal strains such as S. aureus are among the most important bacterial pathogens and secondly, they have a rather average photosensitivity for violet light [16], so that similar results can be expected for other bacteria. Coronaviruses require similar or even higher doses for reduction [18]. Therefore, white or white-violet lighting should not be expected to work miracles in terms of very rapid reduction of bacteria or (corona-) viruses.
[1] | Coronavirus Resource Center, COVID-19 Dashboard: (Global Map), 2022. Available from: https://coronavirus.jhu.edu/map.html |
[2] |
Murray CJL, Ikuta KS, Sharara F, et al. (2022) Global burden of bacterial antimicrobial resistance in 2019: a systematic analysis. The Lancet 399: 629-655. https://doi.org/10.1016/S0140-6736(21)02724-0 ![]() |
[3] |
Jagger J (1968) Introduction to research in ultraviolet photobiology. Photochem Photobiol 7: 413. ![]() |
[4] |
Masjoudi M, Mohseni M, Bolton JR (2021) Sensitivity of bacteria, protozoa, viruses, and other microorganisms to ultraviolet radiation. J Res Natl Inst Stan 126: 1-77. https://doi.org/10.6028/jres.126.021 ![]() |
[5] |
Kowalski W (2009) Ultraviolet Germicidal Irradiation Handbook. Berlin: Springer. ![]() |
[6] |
Liu S, Luo W, Li D, et al. (2021) Sec-eliminating the SARS-CoV-2 by AlGaN based high power deep ultraviolet light source. Adv Funct Mater 31: 2008452. https://doi.org/10.1002/adfm.202008452 ![]() |
[7] |
Rathnasinghe R, Karlicek RF, Schotsaert M, et al. (2021) Scalable, effective, and rapid decontamination of SARS-CoV-2 contaminated N95 respirators using germicidal ultraviolet C (UVC) irradiation device. Sci Rep 11: 1-10. https://doi.org/10.1038/s41598-021-99431-5 ![]() |
[8] |
Ruetalo N, Businger R, Schindler M (2021) Rapid, dose-dependent and efficient inactivation of surface dried SARS-CoV-2 by 254 nm UV-C irradiation. Eurosurveillance 26: 2001718. https://doi.org/10.2807/1560-7917.ES.2021.26.42.2001718 ![]() |
[9] |
Ashkenazi H, Malik Z, Harth Y, et al. (2003) Eradication of Propionibacterium acnes by its endogenic porphyrins after illumination with high intensity blue light. FEMS Immunol Med Microbiol 35: 17-24. https://doi.org/10.1111/j.1574-695X.2003.tb00644.x ![]() |
[10] |
Guffey JS, Wilborn J (2006) In vitro bactericidal effects of 405-nm and 470-nm blue light. Photomed Laser Ther 24: 684-688. https://doi.org/10.1089/pho.2006.24.684 ![]() |
[11] |
Maclean M, MacGregor SJ, Anderson JG, et al. (2008) High-intensity narrow-spectrum light inactivation and wavelength sensitivity of Staphylococcus aureus. FEM Microbiol Lett 285: 227-232. https://doi.org/10.1111/j.1574-6968.2008.01233.x ![]() |
[12] |
Feuerstein O, Ginsburg I, Dayan E, et al. (2005) Mechanism of visible light phototoxicity on Porphyromonas gingivalis and Fusobacterium nucleatum. Photochem Photobiol 81: 1186-1189. https://doi.org/10.1562/2005-04-06-RA-477 ![]() |
[13] |
Amin RM, Bhayana B, Hamblin MR, et al. (2016) Antimicrobial blue light inactivation of Pseudomonas aeruginosa by photo-excitation of endogenous porphyrins: in vitro and in vivo studies. Laser Surg Med 48: 562-568. https://doi.org/10.1002/lsm.22474 ![]() |
[14] |
Plavskii VY, Mikulich AV, Tretyakova AI, et al. (2018) Porphyrins and flavins as endogenous acceptors of optical radiation of blue spectral region determining photoinactivation of microbial cells. J Photochem Photobiol B 183: 172-183. https://doi.org/10.1016/j.jphotobiol.2018.04.021 ![]() |
[15] |
Cieplik F, Späth A, Leibl C, et al. (2014) Blue light kills aggregatibacter actinomycetemcomitans due to its endogenous photosensitizers. Clin Oral Invest 18: 1763-1769. https://doi.org/10.1007/s00784-013-1151-8 ![]() |
[16] |
Hessling M, Spellerberg B, Hoenes K (2017) Photoinactivation of bacteria by endogenous photosensitizers and exposure to visible light of different wavelengths–a review on existing data. FEM Microbiol Lett 364: fnw270. https://doi.org/10.1093/femsle/fnw270 ![]() |
[17] |
Tomb RM, White TA, Coia JE, et al. (2018) Review of the comparative susceptibility of microbial species to photoinactivation using 380–480 nm violet-blue light. Photochem Photobiol 94: 445-458. https://doi.org/10.1111/php.12883 ![]() |
[18] |
Hessling M, Lau B, Vatter P (2022) Review of virus inactivation by visible light. Photonics 9: 113. https://doi.org/10.3390/photonics9020113 ![]() |
[19] |
Kleinpenning MM, Smits T, Frunt MHA, et al. (2010) Clinical and histological effects of blue light on normal skin. Photodermatol Photoimmunol Photome 26: 16-21. https://doi.org/10.1111/j.1600-0781.2009.00474.x ![]() |
[20] |
McDonald R, Gupta S, MacLean M, et al. (2013) 405 nm light exposure of osteoblasts and inactivation of bacterial isolates from arthroplasty patients: potential for new disinfection applications?. Eur Cells Mater 25: 204-214. https://doi.org/10.22203/eCM.v025a15 ![]() |
[21] |
Gillespie JB, Maclean M, Wilson MP, et al. (2017) Development of an antimicrobial blended white LED system containing pulsed 405nm LEDs for decontamination applications. Proc SPIE 10056: 100560Y. https://doi.org/10.1117/12.2250539 ![]() |
[22] |
Rohan A, Khan I, Yin D, et al. (2019) Passive ceiling light disinfection system to reduce bioburden in an intensive care unit. J Pediatr Intensive 8: 138-143. https://doi.org/10.1055/s-0038-1676655 ![]() |
[23] |
Rutala WA, Kanamori H, Gergen MF, et al. (2018) Antimicrobial activity of a continuous visible light disinfection system. Infect Control Hosp Epidemiol 39: 1250-1253. https://doi.org/10.1017/ice.2018.200 ![]() |
[24] |
Buehler J, Sommerfeld F, Meurle T, et al. (2021) Disinfection properties of conventional white LED illumination and their potential increase by violet LEDs for applications in medical and domestic environments. Adv Sci Technol Res J 15: 169-175. https://doi.org/10.12913/22998624/134641 ![]() |
[25] |
Hoenes K, Bauer R, Meurle T, et al. (2021) Inactivation effect of violet and blue light on ESKAPE pathogens and closely related non-pathogenic bacterial species–a promising tool against antibiotic-sensitive and antibiotic-resistant microorganisms. Front Microbiol 11: 612367. https://doi.org/10.3389/fmicb.2020.612367 ![]() |
[26] |
Lau B, Becher D, Hessling M (2021) High intensity violet light (405 nm) inactivates coronaviruses in phosphate buffered saline (PBS) and on surfaces. Photonics 8: 414. https://doi.org/10.3390/photonics8100414 ![]() |
[27] | Amerlux LLC, Active Clean: Nonstop Antimicrobial Action Against Viruses and Bacteria, 2021. Available from: https://bestlight.amerlux.com/active-clean/ |
[28] | User Par, Planckian Locus, 2012. Available from: https://commons.wikimedia.org/wiki/File:PlanckianLocus.png |
[29] | DIN EN 12464-1:2021-11, Light and lighting-Lighting of work places-Part 1: Indoor work places, Beuth Verlag GmbH, Berlin. Available from: https://standards.globalspec.com/std/14462596/EN%2012464-1 |
[30] |
Michelini Z, Mazzei C, Magurano F, et al. (2021) UltraViolet SANitizing system for sterilization of ambulances fleets and for real-time monitoring of their sterilization level. Int J Environ Res Public Health 19: 331. https://doi.org/10.3390/ijerph19010331 ![]() |
[31] |
Yang JH, Wu UI, Tai HM, et al. (2019) Effectiveness of an ultraviolet-C disinfection system for reduction of healthcare-associated pathogens. J Microbiol Immunol Infect 52: 487-493. https://doi.org/10.1016/j.jmii.2017.08.017 ![]() |
[32] |
Rutala WA, Gergen MF, Tande BM, et al. (2013) Rapid hospital room decontamination using ultraviolet (UV) light with a nanostructured UV-reflective wall coating. Infect Control Hosp Epidemiol 34: 527-529. https://doi.org/10.1086/670211 ![]() |
[33] |
Bormann M, Alt M, Schipper L, et al. (2021) Disinfection of SARS-CoV-2 contaminated surfaces of personal items with UVC-LED disinfection boxes. Viruses 13: 598. https://doi.org/10.3390/v13040598 ![]() |
[34] |
Rutala WA, Gergen MF, Tande BM, et al. (2014) Room decontamination using an ultraviolet-C device with short ultraviolet exposure time. Infect Control Hosp Epidemiol 35: 1070-1072. https://doi.org/10.1086/677149 ![]() |
[35] |
Rutala WA, Gergen MF, Weber DJ (2010) Room decontamination with UV radiation. Infect Control Hosp Epidemiol 31: 1025-1029. https://doi.org/10.1086/656244 ![]() |
[36] |
McDevitt JJ, Milton DK, Rudnick SN, et al. (2008) Inactivation of poxviruses by upper-room UVC light in a simulated hospital room environment. PLoS One 3: e3186. https://doi.org/10.1371/journal.pone.0003186 ![]() |
[37] |
Bedell K, Buchaklian AH, Perlman S (2016) Efficacy of an automated multiple emitter whole-room ultraviolet-C disinfection system against coronaviruses MHV and MERS-CoV. Infect Control Hosp Epidemiol 37: 598-599. https://doi.org/10.1017/ice.2015.348 ![]() |
[38] |
Nunayon SS, Zhang H, Lai ACK (2020) Comparison of disinfection performance of UVC-LED and conventional upper-room UVGI systems. Indoor Air 30: 180-191. https://doi.org/10.1111/ina.12619 ![]() |
[39] |
Szeto W, Yam WC, Huang H, et al. (2020) The efficacy of vacuum-ultraviolet light disinfection of some common environmental pathogens. BMC Infect Dis 20: 1-9. https://doi.org/10.1186/s12879-020-4847-9 ![]() |
1. | Katja Zeller, Stephan Mühleisen, Pranavi Shanmugarajah, Nicole Fehler, Robin Haag, Martin Hessling, Influence of Visible Violet, Blue and Red Light on the Development of Cataract in Porcine Lenses, 2022, 58, 1648-9144, 721, 10.3390/medicina58060721 | |
2. | Valentina Lucarelli, Davide Amodeo, Isa de Palma, Nicola Nante, Gabriele Cevenini, Gabriele Messina, The potential role of violet-blue light to preventing hospital acquired infections: a systematic review, 2024, 12, 2296-2565, 10.3389/fpubh.2024.1474295 |