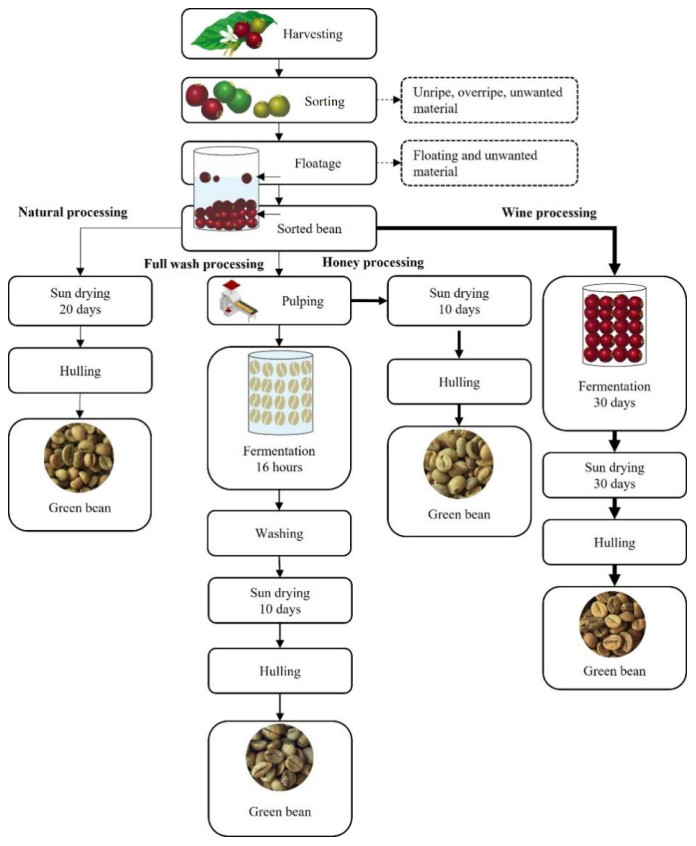
In Bogor, the farmers employed several methods for robusta post-harvest processing, including natural, honey, full wash, and wine processing. This research was conducted to examine the influence of the different post-harvest processing methods on volatile compounds and to identify volatile markers that can authenticate coffee roasted under second-crack roasting and characterize its dry aroma. The study identified and semiquantified 140 compounds. Post-harvest processing affected carboxylic acids, esters, alcohols, hydrocarbons, phenolics, thiophenes, and total volatile compounds. Principal component analysis (PCA) modeling showed that natural and honey processes had similar volatile compound compositions, while full wash and wine processes tended to differ. Based on the variable importance in projection (VIP) values from orthogonal partial least square discriminant analysis (OPLS-DA) modeling and percent contribution, two compounds (i.e., ethyl salicylate and 2-Methyl-5-methoxy-4H-pyran-4-one) were identified as potential markers for natural and wine processing. Ethyl acetate and 5-amino-2-methylbenzothiazole were identified as potential markers for wine processing. Honey and full wash processing did not have any distinct volatile marker. Natural processing exhibited a dry aroma of caramelly, roasted peanut, and chocolate, while honey processing had caramelly, nutty, and earthy aromas. Full wash processing had sweet nut, earthy, and herbal aromas with a hint of potato, and wine processing had fermented, winey, molasses, and chocolaty aromas. This research demonstrated that post-harvest processing influenced volatile compounds in second-crack roasted coffee. Identifying potential markers provides valuable information for authenticating second-crack roasted coffee and differentiating it based on post-harvest processing and dry aroma.
Citation: Nur Fajriani Suaib, Didah Nur Faridah, Dede Robiatul Adawiyah, Nuri Andarwulan. Semiquantification of volatile compounds and identification of potential volatile markers and dry aroma from robusta second-crack roasted coffee processed from several post-harvest processing[J]. AIMS Agriculture and Food, 2025, 10(1): 74-96. doi: 10.3934/agrfood.2025005
[1] | Cíntia Sorane Good Kitzberger, David Pot, Pierre Marraccini, Luiz Filipe Protasio Pereira, Maria Brígida dos Santos Scholz . Flavor precursors and sensory attributes of coffee submitted to different post-harvest processing. AIMS Agriculture and Food, 2020, 5(4): 700-714. doi: 10.3934/agrfood.2020.4.700 |
[2] | Ioannis K. Karabagias, Chara Papastephanou, Vassilios K. Karabagias . Geographical differentiation of Cypriot multifloral honeys through specific volatile compounds and the use of DFA. AIMS Agriculture and Food, 2019, 4(1): 149-162. doi: 10.3934/agrfood.2019.1.149 |
[3] | Cíntia Sorane Good Kitzberger, Maria Brígida dos Santos Scholz, João Batista Gonçalves Dias da Silva, Marta de Toledo Benassi, Luiz Filipe Protasio Pereira . Free choice profiling sensory analysis to discriminate coffees. AIMS Agriculture and Food, 2016, 1(4): 455-469. doi: 10.3934/agrfood.2016.4.455 |
[4] | Sapta Raharja, Yogi Purna Rahardjo, Samsudin, Khaswar Syamsu . Aroma precursor enhancing in dried cocoa beans fermentation using enzyme and heat addition. AIMS Agriculture and Food, 2023, 8(2): 674-686. doi: 10.3934/agrfood.2023037 |
[5] | Dody Dwi Handoko, Anisa Maharani Kaseh, Laras Cempaka, Wahyudi David, Bram Kusbiantoro, Afifah Zahra Agista, Yusuke Ohsaki, Hitoshi Shirakawa, Ardiansyah . Effects of household-scale cooking on volatile compounds, sensory profile, and hypotensive effect of Kenikir (Cosmos caudatus). AIMS Agriculture and Food, 2023, 8(1): 198-213. doi: 10.3934/agrfood.2023011 |
[6] | Ardiansyah, Fauziyyah Ariffa, Rizki Maryam Astuti, Wahyudi David, Dody Dwi Handoko, Slamet Budijanto, Hitoshi Shirakawa . Non-volatile compounds and blood pressure-lowering activity of Inpari 30 and Cempo Ireng fermented and non-fermented rice bran. AIMS Agriculture and Food, 2021, 6(1): 337-359. doi: 10.3934/agrfood.2021021 |
[7] | Siska Septiana, Nancy Dewi Yuliana, Boy Muchlis Bachtiar, Christofora Hanny Wijaya . Aroma-active compounds of Melaleuca cajuputi essential oil, a potent flavor on Cajuputs Candy. AIMS Agriculture and Food, 2020, 5(2): 292-306. doi: 10.3934/agrfood.2020.2.292 |
[8] | Esteban Largo-Avila, Carlos Hernán Suarez-Rodríguez, Jorge Latorre Montero, Madison Strong, Osorio-Arias Juan . The influence of hot-air mechanical drying on the sensory quality of specialty Colombian coffee. AIMS Agriculture and Food, 2023, 8(3): 789-803. doi: 10.3934/agrfood.2023042 |
[9] | Eleni C. Pappa, Efthymia Kondyli, Anna-Maria Vlachou, Athanasia Kakouri, Evdokia Malamou, John Samelis . Semi industrial production of Tsalafouti dairy product. AIMS Agriculture and Food, 2022, 7(2): 444-460. doi: 10.3934/agrfood.2022028 |
[10] | Cíntia Sorane Good Kitzberger, Maria Brígida dos Santos Scholz, Luiz Filipe Protasio Pereira, João Batista Gonçalves Dias da Silva, Marta de Toledo Benassi . Profile of the diterpenes, lipid and protein content of different coffee cultivars of three consecutive harvests. AIMS Agriculture and Food, 2016, 1(3): 254-264. doi: 10.3934/agrfood.2016.3.254 |
In Bogor, the farmers employed several methods for robusta post-harvest processing, including natural, honey, full wash, and wine processing. This research was conducted to examine the influence of the different post-harvest processing methods on volatile compounds and to identify volatile markers that can authenticate coffee roasted under second-crack roasting and characterize its dry aroma. The study identified and semiquantified 140 compounds. Post-harvest processing affected carboxylic acids, esters, alcohols, hydrocarbons, phenolics, thiophenes, and total volatile compounds. Principal component analysis (PCA) modeling showed that natural and honey processes had similar volatile compound compositions, while full wash and wine processes tended to differ. Based on the variable importance in projection (VIP) values from orthogonal partial least square discriminant analysis (OPLS-DA) modeling and percent contribution, two compounds (i.e., ethyl salicylate and 2-Methyl-5-methoxy-4H-pyran-4-one) were identified as potential markers for natural and wine processing. Ethyl acetate and 5-amino-2-methylbenzothiazole were identified as potential markers for wine processing. Honey and full wash processing did not have any distinct volatile marker. Natural processing exhibited a dry aroma of caramelly, roasted peanut, and chocolate, while honey processing had caramelly, nutty, and earthy aromas. Full wash processing had sweet nut, earthy, and herbal aromas with a hint of potato, and wine processing had fermented, winey, molasses, and chocolaty aromas. This research demonstrated that post-harvest processing influenced volatile compounds in second-crack roasted coffee. Identifying potential markers provides valuable information for authenticating second-crack roasted coffee and differentiating it based on post-harvest processing and dry aroma.
Bogor Regency is one of the areas in Indonesia that produces robusta coffee, and the processing methods used by farmers have undergone rapid development. Robusta coffee farmers commonly employ several post-harvest processing methods including natural, honey, full wash, and wine. The differences between these processes lie in the treatment of the coffee beans before processing, the fermentation methods used during processing, the presence of a washing stage, and the duration of the drying process [1]. In coffee beans, the composition of metabolites is influenced by genetic factors; agricultural practices, such as harvesting techniques and processing methods; and environmental factors, such as the climate where the coffee plants grow [2,3,4]. Previous research has shown variations in volatile compounds from robusta coffee beans processed using the natural, honey, and full wash methods. This study continues that research by focusing on coffee that has undergone the roasting process.
Roasting is a critical process in coffee processing because it is during roasting that volatile compounds and flavors are formed, which can become the distinctive characteristics of coffee brew [2,5,6]. During roasting, these compounds undergo significant changes due to the Maillard reaction, acid degradation, protein degradation and denaturation, caramelization, pyrolysis, and other reactions [6,7]. Temperature and roasting time are typically controlled during the roasting process, and parameters, such as color, aroma, flavor, pH, cracking sound, and mass loss, are often used by roasters to determine the degree of roasting in real time [8,9,10]. While color parameters have long been used to measure roasting degree, known as roast level, they are considered inadequate for providing a clear picture of the roasting process [11]. Cracking is a phenomenon that occurs in coffee beans during roasting, characterized by a popping sound audible to the human ear. It is caused by the accumulation of CO2, which leads to steam pressure buildup. Two cracks occur during the roasting, the first crack, which produces a softer popping sound, and the second crack, which is characterized by louder and more frequent pops [8]. Based on the physical quality of the coffee beans produced using the four processing methods, the wine-processed coffee beans showed the most distinctive color characteristics, brownish hues. Due to this color difference, the roast color parameter was deemed less suitable; therefore, the second crack phase parameter was chosen for analysis in this study.
Variations in post-harvest coffee processing can lead to discrepancies in the aroma of roasted coffee, while the roasting procedure itself may affect the extraction of volatile compounds [12,13,14]. These volatile compounds contribute to the distinctive aroma of coffee. The profile of volatile compounds of Bogor robusta processed by natural, honey, full wash, and wine methods and roasted to the second crack phase has not been reported before. This study was designed to investigate whether the natural, honey, full wash, and wine coffee processing methods influence the concentration of volatile compounds produced. The study also aims to identify potential marker compounds of second-crack roasted coffee that can differentiate between post-harvest processing methods. Additionally, it seeks to determine the aroma characteristics of second-crack roasted coffee produced from these four processing methods.
Robusta (Coffea canephora) beans were obtained from farmers in the Sukamakmur District of Bogor Regency, Indonesia. The coffee is grown at 900–1000 meters above sea level. Only ripe red coffee cherries were selected for post-harvest processing. The farmers used traditional methods for processing as shown in Figure 1. A total of 20 kg of coffee beans were collected per processing batch, then sorted according to the fine robusta standards and protocols established by the Uganda Coffee Development Authority and The Coffee Institute [15]. The coffee beans were manually sorted at each refining step to produce fine-grade robusta green coffee beans. The beans were then passed through sieves with 8 mm, 7 mm, and 6 mm diameters. Coffee beans that passed through the 8 mm sieve but could not pass the 7 mm sieve were collected and used in this study.
A total of 500 g of coffee beans from each processing method were roasted using a Super Roaster roasting machine (Indonesia) with a capacity of 2.5 kg, featuring a gas burner heater and electric drive. The sound of cracking was carefully monitored during the roasting process. The endpoint of the roasting process was determined once the second crack sound was heard. Upon hearing the second crack, the heat source was immediately lowered to prevent a further increase in the final temperature. The roasted coffee was removed from the roasting machine and cooled for 3 minutes. The entire roasting process up until the second crack was recorded using Artisan 2.0.0 software (GPLv3.0 License, Germany) connected to a laptop. An experienced roaster carried out the roasting process to ensure accurate results. For volatile compound analysis, the roasted coffee was ground using a coffee grinder (Gemilai crm 9053, China).
The volatile compounds were analyzed using GC (Agilent 7890A) and MS (Agilent 5975C XL EI/CI). The analysis of volatile compounds using HS-SPME-GCMS is based on the method referenced in [16], with minor modifications. A 22 mL vial containing 2 g of roasted coffee powder was filled with 5 µL of 0.01 concentration of the internal standard (3-heptanone). To achieve equilibrium, the vial was heated to 70 ℃ for 10 minutes. Subsequently, a 2 cm DVB/CAR/PDMS (Divinylbenzene/Carboxen/Polydimethylsiloxane) fiber was inserted into the headspace of the vial and incubated for 20 minutes at 70 ℃. The volatile compounds were then desorbed for 5 minutes in the GC. Compound separation was carried out using a DB-wax column (30 m x 250 µm x 0.25 µm). The GC injector was operated in spitless mode at 250 ℃, with helium as the carrier gas at a flow rate of 0.8 mL/min. The oven temperature was initially set to 50 ℃ for 5 minutes, then increased to 240 ℃ at a rate of 5 ℃/min, where it was maintained for 10 minutes. The ion source (MS source was set to 230 ℃, MS Quad was set to 150 ℃), and the interface was set to 250 ℃. Mass spectra were scanned in the range of m/z 29–550 amu. Volatile compounds were identified by comparing their mass spectra and retention indices with the NIST-14 database. The retention times of C9-C32 alkanes were used to calculate the retention index.
Compounds were semiquantified based on the ratio of their peak area to the peak area of the 3-heptanone internal standard, which was used to calculate the relative amount of each compound in µg/g. The percent contribution of each compound was determined by dividing the peak area of that compound by the total peak area of all identified compounds. All measurements were conducted in duplicate.
Dry aroma evaluation was conducted by six trained panelists, including one Q-grader, three baristas, and two roasters. Following the method outlined in [15], 8.75 g of ground coffee was placed in a porcelain container with a lid. The panelists were asked to smell the coffee and identify the type of dry aroma and then rate it on a scale from 1 to 6.
This research has received ethical clearance for sensory evaluation from the Research Ethics Commission of IPB University, under approval number 613/IT3.KEPMSM-IPB/SK/2022.
An analysis of variance (ANOVA) and Duncan's multiple range test were used to assess the impact of post-harvest processing on relative amounts of volatile compounds. SPSS version 27 (SPSS Inc., Chicago, IL, USA) was employed to perform the ANOVA and Duncan tests. Multivariate analysis of the percent contribution data of compounds from GC-MS was conducted using principal component analysis (PCA) and orthogonal partial least square discriminant analysis (OPLS-DA) modeling to illustrate the grouping of compounds based on their post-harvest processing and to identify potential discriminant marker compounds using variable importance in projection (VIP) values. Compounds with a VIP value > 0, 8 were considered to have the highest potential for discrimination [17]. Compounds with VIP values > 0, 8 and percent contribution values > 0, 1 were identified as potential markers. PCA and OPLS-DA analysis were performed using SIMCA software version 14.1 (Umetrics, Umea, Sweden).
Based on the roasting process conducted (Figure 2), naturally processed coffee tends to reach the second crack earlier, followed by honey, full wash, and wine-processed coffee, with the latter experiencing the second crack last. The temperature at which the second crack occurred for each processing method was generally similar, ranging from 223 ℃ to 228 ℃.
Cracks in roasted coffee occur in two phases: the first crack and the second crack. The first crack typically occurs when the bean temperature reaches around 200 ℃ and is characterized by a loud, low-frequency popping sound lasting about two minutes. When the beans reach a temperature of approximately 230 ℃, the second crack occurs, characterized by a more frequent popping sound [18]. It has been reported that the roast profile is closely related to factors, such as coffee variety, temperature, time, airflow, and the roasting method, all of which influence the characteristics the roasted coffee produced [19].
During the first crack phase, treatments had no significant temperature or first crack duration differences. Differences between treatments became apparent during the second crack phase. Naturally processed coffee entered the second crack phase more quickly than the other treatments, which resulted in a shorter roasting duration for natural processed coffee. This phenomenon was previously reported by [20], coffee processed using the natural method exhibited a denser bean structure, causing it to lose mass more rapidly during roasting than full wash processed coffee. In contrast, honey and wine processed coffees did not differ significantly from full wash processed coffee. The results of the second-crack roasting process (Figure 3) revealed the presence of oil on the surface of the coffee. Based on the physical characteristics of the coffee and the temperature, which ranged from 223 ℃ to 228 ℃, the second-crack roasted coffee produced by all processing was classified as a medium-to-dark roast level [21,22].
HS-SPME GC-MS was used to extract volatile compounds from the second crack-roasted coffee, resulting in the identification and quantification of up to 140 compounds, which accounted for a total contribution of over 87%. Analysis with PCA (Figure 4), based on the percent contribution value of volatile compounds, produced a model with a total variance of 70.10%, with PC1 contributing 49.90% and PC2 contributing 20.20%.
PCA analysis was used to examine the dominant volatile compounds and the grouping of processing methods based on the similarity of their volatile compounds. Figure 4 demonstrates a clear separation between the processing methods. Natural and honey processed coffee were located on the same plane, corresponding to positive PC1. Full wash processed coffee was positioned in the negative PC1 plane, while wine-processed coffee appeared in the farthest plane, negative PC2. The proximity of natural and honey-processed coffee on the same plane suggests that the volatile compound profiles of these two processes were similar. In contrast, full wash and wine processing differed significantly from the others. These results confirmed previous research [1], which reported that natural and honey-processed coffee beans shared similar volatile compounds, while full wash and wine-processed coffee beans were distinct. The influence of processing on the volatile compound profile remained consistent even after the second-crack roasting process. As reported by [4], coffee processing involving anaerobic fermentation affects the chemical components of the coffee fruit, leading to flavors that differ from those of other processing methods.
The semiquantification of volatile compounds in roasted coffee is presented in Table 1. The analysis revealed that the dominant compounds in roasted coffee furan, pyrazine, phenolic, and carboxylic acid. The furan group, which was the most abundant compound group in second-crack roasted coffee, is formed during the roasting process due to interaction between high temperatures and sugar, amino acids, ascorbic acid oxidation, unsaturated fatty acids, and carotenoids [23,24]. The dominant compound within the furan group included 2-furanmethanol, furfuryl acetate, 5-methyl-furfural, and furfural. Among these, 2-furanmethanol was the predominant compound in Bogor robusta coffee, a finding consistent with results reported by [4,25,26]. However, [27] reported that methylbutyrate, 2,3-dimethylpyrazine, and 3-hexanone were the dominant compounds in the robusta samples observed. This suggests that the compounds in roasted robusta coffee are influenced by various factors, including the treatment applied to the coffee beans. Furfuryl acetate, the second-highest compound, has been reported to be more prevalent in robusta coffee than in arabica coffee [28]. The analysis showed that the post-harvest processing did not affect the furan group compounds (Table 1). The concentration of furan in roasted coffee was reported to be more influenced by the coffee species. The degree of roasting, where robusta coffee tends to contain higher furan compounds compared to arabica, and coffee with a dark roast level contains more furan compared to the light level, dark roast level contains 5697 µg/kg of furan in robusta coffee. In comparison, arabica coffee contains 3809 µg/kg [29,30].
Compound | RT (min) | RI | Relative amount | |||
Natural | Honey | Full wash | Wine | |||
Aldehyde | ||||||
acetaldehyde | 1.59 | - | 0.23 ± 0.04 | 0.31 ± 0.00 | 0.29 ± 0.01 | 0.23 ± 0.03 |
2-methylbutanal | 2.57 | 912 | 0.49 ± 0.05 | 0.34 ± 0.01 | 0.39 ± 0.04 | 0.75 ± 0.10 |
3-methylbutanal | 2.60 | 914 | 0.39 ± 0.02 | 0.28 ± 0.01 | 0.34 ± 0.03 | 0.50 ± 0.07 |
1-methyl-1H-pyrrole-2-carboxaldehyde | 20.93 | 1619 | 0.69 ± 0.06 | 0.72 ± 0.02 | 0.95 ± 0.03 | 0.99 ± 0.13 |
2-phenyl-2-butenal | 27.88 | 1931 | 0.30 ± 0.01 | 0.26 ± 0.02 | 0.41 ± 0.00 | 0.37 ± 0.08 |
Total Aldehydes | 2.11 ± 0.06ab | 1.90 ± 0.05a | 2.38 ± 0.03ab | 2.83 ± 0.40b | ||
Furan | ||||||
furan | 1.85 | - | 0.10 ± 0.01 | 0.09 ± 0.01 | 0.12 ± 0.01 | 0.11 ± 0.01 |
2-methylfuran | 2.23 | - | 0.27 ± 0.01 | 0.22 ± 0.01 | 0.33 ± 0.01 | 0.22 ± 0.04 |
2.5-dimethylfuran | 2.99 | 946 | 0.12 ± 0.01 | 0.10 ± 0.00 | 0.15 ± 0.01 | 0.14 ± 0.02 |
2-pentylfuran | 10.41 | 1238 | 0.14 ± 0.01 | 0.15 ± 0.01 | 0.17 ± 0.00 | 0.15 ± 0.01 |
dihydro-2-methyl-3-furanone | 11.15 | 1262 | 0.51 ± 0.05 | 0.52 ± 0.01 | 0.51 ± 0.02 | 0.32 ± 0.02 |
2-methyl tetrahydrofuran | 13.93 | 1354 | 0.14 ± 0.04 | 0.15 ± 0.02 | 0.15 ± 0.00 | 0.17 ± 0.01 |
furfural | 16.92 | 1461 | 3.23 ± 0.21 | 2.53 ± 0.13 | 2.91 ± 0.24 | 2.41 ± 0.29 |
2-furfuryl methyl sulfide | 17.56 | 1485 | 0.17 ± 0.00 | 0.20 ± 0.01 | 0.24 ± 0.00 | 0.25 ± 0.02 |
2-acetylfuran | 18.02 | 1502 | 1.00 ± 0.01 | 1.08 ± 0.03 | 1.29 ± 0.01 | 1.02 ± 0.10 |
2-furfuryl-acetate | 18.92 | 1538 | 5.84 ± 0.15 | 6.53 ± 0.55 | 6.69 ± 0.10 | 8.76 ± 0.04 |
2-furanmethanamine | 19.54 | 1563 | 0.19 ± 0.02 | 0.19 ± 0.01 | 0.28 ± 0.01 | 0.30 ± 0.06 |
5-methylfurfural | 19.76 | 1571 | 4.65 ± 0.14 | 3.70 ± 0.08 | 4.43 ± 0.21 | 3.73 ± 0.47 |
2-ethyl-5-methylfuran | 19.84 | 1575 | 0.21 ± 0.00 | 0.23 ± 0.01 | 0.28 ± 0.01 | 0.16 ± 0.03 |
2.2-methylenebisfuran | 20.71 | 1610 | 0.74 ± 0.05 | 0.75 ± 0.03 | 0.93 ± 0.01 | 0.73 ± 0.08 |
2-acetyl-5-methylfuran | 20.80 | 1613 | 0.20 ± 0.01 | 0.21 ± 0.02 | 0.25 ± 0.01 | 0.19 ± 0.01 |
2-furanmethanol | 22.02 | 1666 | 12.70 ± 0.43 | 11.87 ± 0.54 | 15.15 ± 0.65 | 14.90 ± 1.93 |
2-furfuryl-5-methylfuran | 22.40 | 1681 | 0.38 ± 0.01 | 0.45 ± 0.02 | 0.68 ± 0.01 | 0.36 ± 0.05 |
2-hexyl furan | 23.12 | 1712 | 0.19 ± 0.02 | 0.12 ± 0.01 | 0.21 ± 0.01 | 0.23 ± 0.03 |
3.4-dimethylfuran-2.5-dione | 23.46 | 1727 | 0.39 ± 0.02 | 0.36 ± 0.02 | 0.47 ± 0.02 | 0.44 ± 0.03 |
3-phenyl furan | 26.24 | 1853 | 0.53 ± 0.00 | 0.44 ± 0.01 | 0.65 ± 0.03 | 0.72 ± 0.08 |
2-methyl-3-(2-furyl)propenal | 26.71 | 1875 | 0.18 ± 0.00 | 0.17 ± 0.01 | 0.25 ± 0.01 | 0.18 ± 0.05 |
5-methyl-2-furanmethanethiol | 26.93 | 1885 | 0.18 ± 0.01 | 0.17 ± 0.02 | 0.26 ± 0.01 | 0.29 ± 0.04 |
5-ethylfuran-2-carboxylic acid | 27.02 | 1890 | 0.08 ± 0.01 | 0.08 ± 0.01 | 0.10 ± 0.03 | 0.13 ± 0.04 |
difurfuryl disulfide | 27.25 | 1900 | 0.15 ± 0.01 | 0.17 ± 0.03 | 0.28 ± 0.02 | 0.20 ± 0.06 |
2-propyl furan | 27.29 | 1902 | 0.13 ± 0.02 | 0.14 ± 0.02 | 0.18 ± 0.02 | 0.12 ± 0.02 |
furfural acetone | 27.35 | 1905 | 0.15 ± 0.01 | 0.15 ± 0.00 | 0.23 ± 0.01 | 0.24 ± 0.06 |
furfuryl ether | 29.00 | 1985 | 0.47 ± 0.02 | 0.53 ± 0.04 | 0.79 ± 0.00 | 0.53 ± 0.07 |
furaneol | 30.06 | 2038 | 0.12 ± 0.00 | 0.10 ± 0.00 | 0.16 ± 0.0- | 0.11 ± 0.03 |
2-n-butyl furan | 34.03 | 2245 | 0.14 ± 0.02 | 0.16 ± 0.02 | 0.29 ± 0.02 | 0.23 ± 0.04 |
2-ethylfuran | 34.43 | 2267 | 0.10 ± 0.03 | 0.13 ± 0.02 | 0.16 ± 0.03 | 0.14 ± 0.03 |
Total Furans | 33.39 ± 0.08a | 31.71 ± 1.56a | 38.60 ± 0.16a | 37.64 ± 3.82a | ||
Ketone | ||||||
acetone | 1.94 | - | 0.43 ± 0.04 | 0.31 ± 0.01 | 0.44 ± 0.03 | 0.52 ± 0.06 |
2.3-butanedione | 3.28 | 970 | 0.16 ± 0.00 | 0.12 ± 0.01 | 0.16 ± 0.00 | 0.13 ± 0.01 |
2.3-pentanedione | 4.91 | 970 | 0.27 ± 0.02 | 0.20 ± 0.01 | 0.21 ± 0.01 | 0.18 ± 0.02 |
3-penten-2-one | 6.82 | 1123 | 0.05 ± 0.00 | 0.04 ± 0.00 | 0.05 ± 0.00 | 0.29 ± 0.04 |
hydroxyacetone | 12.22 | 1296 | 0.62 ± 0.02 | 0.51 ± 0.02 | 0.56 ± 0.01 | 0.52 ± 0.05 |
1-acetoxy-2-butanone | 18.82 | 1534 | 0.32 ± 0.01 | 0.28 ± 0.00 | 0.32 ± 0.01 | 0.27 ± 0.01 |
1-acetylcyclohexene | 19.32 | 1554 | 0.07 ± 0.01 | 0.06 ± 0.01 | 0.08 ± 0.00 | 0.15 ± 0.01 |
2-acetylpyridine | 20.49 | 1600 | 0.18 ± 0.00 | 0.18 ± 0.01 | 0.21 ± 0.02 | 0.20 ± 0.01 |
2-acetyl-4-methylpyridine | 21.15 | 1628 | 0.09 ± 0.01 | 0.12 ± 0.00 | 0.14 ± 0.01 | 0.11 ± 0.03 |
3-ethyl-2-hydroxy-2-cyclopentenone | 22.44 | 1682 | 0.82 ± 0.03 | 0.80 ± 0.00 | 0.99 ± 0.03 | 0.85 ± 0.09 |
1-(6-methyl-2-pyrazinyl)-1-ethanone | 22.66 | 1692 | 0.63 ± 0.03 | 0.56 ± 0.01 | 0.87 ± 0.04 | 0.87 ± 0.10 |
(+)-2-bornanone | 23.18 | 1714 | 0.14 ± 0.03 | 0.12 ± 0.01 | 0.19 ± 0.03 | 0.21 ± 0.03 |
Total Ketones | 3.78 ± 0.08ab | 3.30 ± 0.03a | 4.21 ± 0.12ab | 4.29 ± 0.45b | ||
Carboxylic acid | ||||||
acetic acid | 16.62 | 1450 | 3.59 ± 0.15 | 3.09 ± 0.03 | 4.21 ± 0.08 | 6.37 ± 0.34 |
4-hydroxybutyric acid | 21.05 | 1624 | 1.46 ± 0.15 | 1.31 ± 0.08 | 1.73 ± 0.14 | 2.63 ± 0.33 |
2-methyl-2-vinylmaleimide | 21.32 | 1635 | 0.20 ± 0.01 | 0.18 ± 0.00 | 0.25 ± 0.01 | 0.27 ± 0.02 |
isovaleric acid | 22.22 | 1673 | 1.26 ± 0.10 | 1.20 ± 0.06 | 1.70 ± 0.04 | 1.34 ± 0.15 |
3-methylcrotonic acid | 25.08 | 1799 | 0.53 ± 0.03 | 0.55 ± 0.03 | 0.75 ± 0.02 | 0.67 ± 0.08 |
2-furanpropionic acid | 25.24 | 1806 | 0.24 ± 0.01 | 0.25 ± 0.03 | 0.36 ± 0.00 | 0.62 ± 0.09 |
Total Carboxylic acid* | 7.27 ± 0.45a | 6.58 ± 0.23a | 9.00 ± 0.26b | 11.89 ± 0.32c | ||
Esther | ||||||
methyl acetate | 1.99 | - | 0.12 ± 0.00 | 0.11 ± 0.01 | 0.18 ± 0.01 | 0.22 ± 0.04 |
ethyl acetate | 2.34 | - | nd | nd | nd | 0.36 ± 0.05 |
ethyl formate | 2.50 | 906 | 0.12 ± 0.01 | 0.34 ± 0.00 | 0.17 ± 0.00 | 0.24 ± 0.01 |
furfuryl propionate | 20.45 | 1599 | 0.47 ± 0.02 | 0.49 ± 0.02 | 0.52 ± 0.05 | 0.38 ± 0.03 |
furfuryl isovalerate | 22.75 | 1695 | 0.33 ± 0.02 | 0.40 ± 0.02 | 0.57 ± 0.00 | 0.34 ± 0.03 |
methyl salicylate | 24.53 | 1774 | 0.47 ± 0.02 | 0.47 ± 0.03 | 0.59 ± 0.01 | 0.57 ± 0.05 |
methyl nicotinate | 24.62 | 1778 | 0.27 ± 0.02 | 0.26 ± 0.02 | 0.36 ± 0.01 | 0.28 ± 0.04 |
ethyl salicylate | 25.31 | 1810 | 0.17 ± 0.04 | nd | nd | 1.24 ± 0.15 |
methyl 3-methylfuroate | 26.52 | 1866 | 0.47 ± 0.04 | 0.45 ± 0.03 | 0.56 ± 0.02 | 0.43 ± 0.07 |
methyl palmitate | 33.50 | 2217 | 0.10 ± 0.00 | 0.10 ± 0.01 | 0.16 ± 0.00 | 0.09 ± 0.00 |
Total Esters* | 2.53 ± 0.06a | 2.62 ± 0.14a | 3.11 ± 0.08a | 4.15 ± 0.46b | ||
Alcohol | ||||||
1.3-propanediol | 2.43 | - | 5.21 ± 0.21 | 5.70 ± 0.04 | 6.84 ± 0.35 | 6.30 ± 0.26 |
ethyl alcohol | 2.80 | 931 | 0.20 ± 0.00 | 0.12 ± 0.00 | 0.16 ± 0.01 | 0.89 ± 0.06 |
1-ethynylcyclohexanol | 21.53 | 1644 | 0.23 ± 0.01 | 0.22 ± 0.02 | 0.29 ± 0.01 | 0.28 ± 0.01 |
benzyl alcohol | 26.82 | 1880 | 0.07 ± 0.01 | 0.06 ± 0.01 | 0.11 ± 0.00 | 0.14 ± 0.02 |
phenylethyl alcohol | 27.57 | 1916 | 0.25 ± 0.01 | 0.22 ± 0.02 | 0.28 ± 0.01 | 0.48 ± 0.02 |
2-thiophenemethanol | 28.22 | 1947 | 0.13 ± 0.00 | 0.11 ± 0.00 | 0.16 ± 0.00 | 0.16 ± 0.04 |
4-pyridinemethanol | 31.33 | 2103 | 0.28 ± 0.00 | 0.30 ± 0.01 | 0.45 ± 0.01 | 0.35 ± 0.05 |
Total Alcohols* | 6.38 ± 0.21a | 6.73 ± 0.03a | 8.29 ± 0.35b | 8.60 ± 0.45b | ||
Hydrocarbons | ||||||
toluene | 4.45 | 1033 | 0.13 ± 0.01 | 0.13 ± 0.00 | 0.18 ± 0.01 | 0.18 ± 0.02 |
dodecane | 9.21 | 1199 | 0.18 ± 0.02 | 0.18 ± 0.02 | 0.19 ± 0.03 | 0.16 ± 0.02 |
2.7-dimethylundecane | 15.25 | 1400 | 0.12 ± 0.02 | 0.09 ± 0.01 | 0.14 ± 0.01 | 0.16 ± 0.02 |
4-isopropyl-m-xylene | 22.35 | 1679 | 0.23 ± 0.03 | 0.23 ± 0.03 | 0.29 ± 0.00 | 0.58 ± 0.07 |
decahydronaphthalene | 22.59 | 1689 | 0.22 ± 0.01 | 0.19 ± 0.02 | 0.24 ± 0.02 | 0.24 ± 0.02 |
pulegone | 22.89 | 1701 | 0.22 ± 0.02 | 0.22 ± 0.02 | 0.28 ± 0.00 | 0.36 ± 0.04 |
β-damascenone | 25.60 | 1823 | 0.27 ± 0.01 | 0.26 ± 0.02 | 0.40 ± 0.01 | 0.38 ± 0.02 |
Total Hydrocarbons* | 1.37 ± 0.19a | 1.30 ± 0.11a | 1.72 ± 0.03ab | 2.06 ± 0.21b | ||
Pyrrole | ||||||
1-ethylpyrrole | 7.23 | 1137 | 0.12 ± 0.02 | 0.09 ± 0.00 | 0.13 ± 0.01 | 0.10 ± 0.00 |
1-butyl pyrrole | 14.75 | 1382 | 0.06 ± 0.00 | 0.05 ± 0.01 | 0.04 ± 0.01 | 0.20 ± 0.01 |
pyrrole | 18.35 | 1515 | 0.51 ± 0.00 | 0.44 ± 0.05 | nd | 0.53 ± 0.09 |
1-methyl-2-pyrroleacetonitrile | 21.59 | 1647 | 0.43 ± 0.03 | 0.39 ± 0.02 | 0.54 ± 0.05 | 0.59 ± 0.04 |
1-furfurylpyrrole | 25.73 | 1829 | 1.45 ± 0.05 | 1.25 ± 0.01 | 1.92 ± 0.06 | 1.78 ± 0.21 |
2-acetylpyrrole | 28.76 | 1974 | 0.83 ± 0.05 | 0.91 ± 0.04 | 1.35 ± 0.06 | 1.06 ± 0.12 |
2-formylpyrrole | 29.81 | 2026 | 0.51 ± 0.01 | 0.49 ± 0.00 | 0.76 ± 0.01 | 0.66 ± 0.07 |
Total Pyrroles | 3.92 ± 0.06ab | 3.62 ± 0.13a | 4.74 ± 0.05ab | 4.92 ± 0.54b | ||
Pyridine | ||||||
pyridine | 8.65 | 1182 | 0.67 ± 0.06 | 1.07 ± 0.03 | 1.24 ± 0.24 | 0.92 ± 0.11 |
2.6-dimethylpyridin-4-amine | 15.39 | 1405 | 1.53 ± 0.18 | 1.36 ± 0.01 | 1.66 ± 0.10 | 2.47 ± 0.29 |
3-methoxypyridine | 20.05 | 1583 | 0.16 ± 0.01 | 0.13 ± 0.01 | 0.17 ± 0.01 | 0.32 ± 0.02 |
3-methylpyridine 1-oxide | 22.97 | 1705 | 0.08 ± 0.02 | 0.11 ± 0.01 | 0.12 ± 0.01 | 0.14 ± 0.02 |
3-(3-thienyl)pyridine | 28.09 | 1941 | 0.14 ± 0.00 | 0.13 ± 0.01 | 0.22 ± 0.00 | 0.27 ± 0.04 |
2-hydroxy-3-methylpyridine | 34.99 | 2297 | nd | nd | nd | 0.11 ± 0.02 |
4-hydroxypyridine | 37.02 | 2413 | 0.18 ± 0.01 | 0.20 ± 0.01 | 0.34 ± 0.00 | 0.22 ± 0.03 |
Total Pyridines | 2.77 ± 0.26a | 3.00 ± 0.08a | 3.75 ± 0.37ab | 4.44 ± 0.49b | ||
Pyrazine | ||||||
pyrazine | 9.54 | 1210 | 0.38 ± 0.05 | 0.27 ± 0.01 | 0.38 ± 0.01 | 0.36 ± 0.03 |
2-methylpyrazine | 11.25 | 1265 | 3.49 ± 0.30 | 3.02 ± 0.02 | 3.88 ± 0.22 | 3.80 ± 0.47 |
2.5-dimethylpyrazine | 12.98 | 1322 | 1.75 ± 0.12 | 1.69 ± 0.03 | 2.08 ± 0.16 | 2.51 ± 0.27 |
2.6-dimethylpyrazine | 13.18 | 1329 | 1.87 ± 0.12 | 1.77 ± 0.02 | 2.32 ± 0.15 | 2.63 ± 0.33 |
2-ethylpyrazine | 13.33 | 1334 | 1.30 ± 0.07 | 1.17 ± 0.01 | 1.45 ± 0.02 | 1.58 ± 0.20 |
2.3-dimethyl pyrazine | 13.69 | 1346 | 0.48 ± 0.04 | 0.43 ± 0.02 | 0.53 ± 0.04 | 0.82 ± 0.09 |
2-ethyl-6-methylpyrazine | 14.85 | 1386 | 1.77 ± 0.16 | 1.62 ± 0.01 | 2.13 ± 0.04 | 2.39 ± 0.27 |
2-ethyl-5-methylpyrazine | 15.02 | 1392 | 1.30 ± 0.11 | 1.26 ± 0.01 | 1.47 ± 0.03 | 1.86 ± 0.21 |
2.6-diethylpyrazine | 16.22 | 1436 | 0.47 ± 0.03 | 0.44 ± 0.02 | 0.55 ± 0.00 | 0.69 ± 0.08 |
3-ethyl-2.5-dimethylpyrazine | 16.54 | 1447 | 1.89 ± 0.32 | 1.59 ± 0.07 | 1.83 ± 0.02 | 2.58 ± 0.30 |
2.3-diethyl pyrazine | 16.78 | 1456 | 0.20 ± 0.01 | 0.17 ± 0.01 | 0.21 ± 0.01 | 0.23 ± 0.01 |
2-ethyl-3.5-dimethylpyrazine | 17.03 | 1466 | 2.15 ± 0.10 | 1.80 ± 0.09 | 2.35 ± 0.09 | 2.94 ± 0.34 |
2-methyl-5-propylpyrazine | 17.37 | 1478 | 0.18 ± 0.02 | 0.16 ± 0.01 | 0.22 ± 0.01 | 0.32 ± 0.02 |
2-ethenyl-6-methylpyrazine | 17.65 | 1488 | 0.28 ± 0.02 | 0.24 ± 0.01 | 0.32 ± 0.01 | 0.46 ± 0.03 |
3.5-diethyl-2-methylpyrazine | 17.85 | 1496 | 1.37 ± 0.10 | 1.21 ± 0.08 | 1.45 ± 0.05 | 1.74 ± 0.21 |
2.3.5-trimethyl-6-ethyl pyrazine | 18.43 | 1519 | 0.47 ± 0.04 | 0.43 ± 0.03 | 0.53 ± 0.04 | 0.71 ± 0.09 |
2.5-dimethyl-3-(2-methylpropyl) pyrazine | 18.70 | 1530 | 0.46 ± 0.02 | 0.34 ± 0.02 | 0.43 ± 0.01 | 0.58 ± 0.03 |
1-propenylpyrazine | 19.21 | 1550 | 0.11 ± 0.01 | 0.09 ± 0.01 | 0.12 ± 0.01 | 0.25 ± 0.02 |
isopropenyl pyrazine | 20.39 | 1596 | 0.21 ± 0.00 | 0.21 ± 0.00 | 0.29 ± 0.04 | 0.24 ± 0.01 |
2-isoamyl-6-methylpyrazine | 21.24 | 1632 | 0.14 ± 0.02 | 0.08 ± 0.01 | 0.13 ± 0.00 | 0.59 ± 0.07 |
2-methyl-6-(1-propenyl)pyrazine | 21.89 | 1659 | 0.10 ± 0.01 | 0.09 ± 0.01 | 0.14 ± 0.00 | 0.19 ± 0.04 |
pyrazinamide | 23.24 | 1717 | 0.96 ± 0.13 | 0.74 ± 0.02 | 1.13 ± 0.23 | 0.88 ± 0.08 |
2-acetyl-3.5-dimethylpyrazine | 24.79 | 1786 | 0.18 ± 0.00 | 0.16 ± 0.02 | 0.24 ± 0.00 | 0.46 ± 0.07 |
Total Pyrazines | 21.49 ± 1.54ab | 19.00 ± 0.44a | 24.17 ± 1.00ab | 28.80 ± 3.29b | ||
Phenolic | ||||||
2.3-dimethylphenol | 11.96 | 1287 | 0.15 ± 0.03 | 0.12 ± 0.01 | 0.21 ± 0.01 | 0.31 ± 0.04 |
4-amino-3-methylphenol | 20.66 | 1608 | 0.22 ± 0.06 | 0.22 ± 0.00 | 0.28 ± 0.02 | 0.37 ± 0.05 |
2-methoxyhydroquinone | 23.72 | 1738 | 0.18 ± 0.00 | 0.18 ± 0.02 | 0.24 ± 0.01 | 0.24 ± 0.02 |
4-amino-2-hydroxytoluene | 25.00 | 1795 | 0.16 ± 0.01 | 0.15 ± 0.02 | 0.26 ± 0.01 | 0.24 ± 0.03 |
guaiacol | 26.41 | 1861 | 1.38 ± 0.08 | 1.17 ± 0.00 | 1.97 ± 0.04 | 1.88 ± 0.21 |
phenol | 29.44 | 2007 | 0.66 ± 0.06 | 0.69 ± 0.04 | 1.25 ± 0.07 | 0.83 ± 0.11 |
4-ethyl-2-methoxyphenol | 29.92 | 2031 | 1.49 ± 0.05 | 1.29 ± 0.10 | 2.17 ± 0.06 | 2.33 ± 0.28 |
p-cresol | 31.10 | 2091 | 0.18 ± 0.00 | 0.19 ± 0.02 | 0.30 ± 0.02 | 0.25 ± 0.03 |
2-methoxy-4-vinylphenol | 33.06 | 2193 | 5.60 ± 0.80 | 4.09 ± 0.14 | 6.94 ± 0.78 | 5.22 ± 0.66 |
Total Phenolics* | 10.01 ± 0.52ab | 8.10 ± 0.36a | 13.61 ± 0.73c | 11.68 ± 1.43bc | ||
Thiopen | ||||||
3-ethyl thiophene | 10.09 | 1228 | 0.05 ± 0.01 | 0.04 ± 0.00 | 0.05 ± 0.00 | 0.14 ± 0.02 |
2-methylthiolan-3-one | 18.62 | 1526 | 0.17 ± 0.00 | 0.16 ± 0.00 | 0.17 ± 0.00 | 0.15 ± 0.01 |
3-acetyl-2.5-dimethylthiophene | 25.41 | 1814 | 0.28 ± 0.01 | 0.25 ± 0.03 | 0.33 ± 0.00 | 0.49 ± 0.05 |
Total Thiopens* | 0.49 ± 0.00a | 0.44 ± 0.03a | 0.55 ± 0.00a | 0.77 ± 0.08b | ||
Miscellaneous | ||||||
sec-butylamine | 1.36 | - | 0.54 ± 0.02 | 0.44 ± 0.03 | 0.59 ± 0.01 | 0.46 ± 0.05 |
1-methylpiperidine | 4.29 | 1027 | 0.07 ± 0.00 | 0.09 ± 0.00 | 0.12 ± 0.01 | 0.15 ± 0.01 |
4-methylthiazole | 11.73 | 1280 | 0.11 ± 0.01 | 0.08 ± 0.00 | 0.09 ± 0.00 | 0.19 ± 0.00 |
butyl ethyl ether | 13.52 | 1340 | 0.12 ± 0.00 | 0.11 ± 0.00 | 0.12 ± 0.01 | 0.12 ± 0.00 |
diisopropyl ether | 14.02 | 1357 | 0.11 ± 0.00 | 0.11 ± 0.01 | 0.11 ± 0.00 | 0.23 ± 0.01 |
5-amino-2-methylbenzothiazole | 19.04 | 1543 | nd | nd | nd | 0.25 ± 0.02 |
benzylhydrazine | 21.39 | 1638 | 0.14 ± 0.01 | 0.11 ± 0.01 | 0.14 ± 0.01 | 0.22 ± 0.05 |
p-aminoanisole | 21.80 | 1655 | 0.56 ± 0.00 | 0.58 ± 0.03 | 0.78 ± 0.00 | 0.71 ± 0.08 |
4-methoxyphenyl isothiocyanate | 23.54 | 1730 | 0.22 ± 0.02 | 0.20 ± 0.01 | 0.31 ± 0.01 | 0.38 ± 0.06 |
4-methoxythiophenol | 23.66 | 1736 | 0.84 ± 0.03 | 0.76 ± 0.02 | 0.89 ± 0.01 | 0.68 ± 0.09 |
2-methyl-5-methoxy-4h-pyran-4-one | 25.90 | 1837 | 0.25 ± 0.00 | nd | nd | 0.33 ± 0.05 |
6-butyltetralin | 32.02 | 2139 | 0.14 ± 0.00 | 0.12 ± 0.01 | 0.22 ± 0.00 | 0.18 ± 0.03 |
caprolactam | 32.98 | 2189 | 0.09 ± 0.00 | 0.09 ± 0.01 | 0.16 ± 0.03 | 0.08 ± 0.00 |
indole | 37.41 | 2433 | 0.15 ± 0.00 | 0.12 ± 0.02 | 0.21 ± 0.00 | 0.15 ± 0.01 |
Total Miscellaneous | 3.34 ± 0.10ab | 2.82 ± 0.15a | 3.74 ± 0.04ab | 4.14 ± 0.46b | ||
Total Volatile Compounds* | 99.91 ± 0.95ab | 92.10 ± 4.31a | 119.08 ± 0.38bc | 127.69 ± 11.08c | ||
Data were the averages ± standard deviation of two replicates. Numbers followed by different superscript letters in the same row indicate significant differences (p < 0.05) based on one-way ANOVA and Duncan test. Groups with (*) were affected by processing. RT (retention time). RI (retention index). Compounds grouping refers to pubchem.ncbi.nlm.nih.gov. |
The pyrazine compound group was the second most abundant group of compounds found in roasted coffee. In addition to furan, pyrazine is produced during the Maillard reaction by interacting with reducing sugars and amino acids. The results of this analysis suggest that differences in coffee bean processing do not significantly affect the concentration of pyrazine compounds. Several studies have reported roasting temperature leading to higher pyrazine content [31,32,33]. However, it has been shown that not only the roasting process, but also post-harvest processing can produce physical and chemical differences in the coffee bean, which significantly influence the formation of aroma compounds, including those in the pyrazine group [12,34].
The fermentation process can influence the number and type of microorganisms in coffee beans, which affect the formation of compounds responsible for the aroma and flavor of coffee, such as pyrazine compounds [35,36,37]. In this study, roasted coffee processed using the wine and full wash methods tended to contain higher levels of 2-methylpyrazine, 2,5-dimethylpyrazine, 2,6-dimethylpyrazine, 2-ethyl-6-methylpyrazine, 2-ethyl-5-methylpyrazine, 2,5-dimethyl-3-ethylpyrazine, 2-ethyl-3,5-dimethylpyrazine, and 2-methyl-3,5-diethylpyrazine compared to those processed using natural and honey processing. The different fermentation processes in full wash processing and the extended fermentation period in wine processing promote the growth of a greater variety and number of microorganisms, which can affect the formation of pyrazine compounds. Microorganisms, such as Bacillus subtilis, Pseudomonas fluorescens SBW25, and Rhodococcus erythropolis [38,39,40], produce compounds in the pyrazine group during fermentation.
Like furan and pyrazine, pyrrole compounds are formed during the roasting process. It has been widely reported that their presence is closely related to roasting conditions such as temperature and roasting intensity [32,41,42]. In this study, the processing method did not affect the relative amount of pyrrole compounds.
The processing method influenced the level of various volatile compound groups, including carboxylic acids, esters, alcohols, hydrocarbons, phenolics, thiophenes, and the total volatile compounds. Acetic acid and 4-hydroxybutyric acid, both carboxylic acid compounds, were found to be the highest in wine processing, followed by full wash processing. The level of acetic acid in wet-processed coffee is typically higher compared to that in natural processing [43]. The coffee soaking process promotes a more consistent fermentation by microorganisms, as the beans have already undergone peeling, removing the fruit flesh. In contrast, natural processing does not involve peeling, and prevents microorganisms from efficiently degrading the sugar in the fruit flesh [44].
The anaerobic fermentation and its duration also influenced the formation of the carboxylic acid. As reported by [9], the post-harvest drying process in coffee processed using the natural method may to decrease in pH, which results from the absorption of acids produced during spontaneous fermentation during. Both the post-harvest processing and the treatment of coffee beans significantly impact the type and concentration of acid compound [45]. Coffee beans from the same fruit, when subjected to different processing methods, affect the production of pectinase enzyme and lead to the formation of varying acid components [46]. Anaerobic fermentation, in particular, fosters variations in microbial communities. It has been reported by [47], microorganisms, such as Acetobacter, Leuconostoc, and Gluconobacter, are dominant during anaerobic fermentation and are also present in aerobic fermentation. The concentration of acetic acid was higher in roasted coffee subjected to fermentation processes (full wash and wine), with higher concentrations observed in roasted coffee that underwent longer fermentation (wine). These results align with those reported by [26,37], where acetic acid concentrations in roasted coffee increased with longer fermentation times.
In the ester group, the presence of ethyl acetate, which was only identified in wine processing, and ethyl salicylate in natural and wine processing had a significant effect. The analysis showed that the post-harvest processing influenced the group of ester compounds. The volatile marker compounds section will discuss the ethyl acetate and ethyl salicylate.
1.3-propanediol is the main compound in all processing that belongs to the alcohols group. It is found in higher amounts in fermented coffee, like full wash and wine. According to the yeast metabolome database, this compound is identified as being produced by Saccharomyces cerevisiae. Similar to 1.3-propanediol, ethyl alcohol can be produced by Saccharomyces cerevisiae and is most prevalent in wine-processed coffee. The high relative amount of ethyl alcohol in wine processed coffee is an essential characteristic of the anaerobic fermentation process.
Similar to the carboxylic acid and alcohol groups, the highest relative amounts of phenolic group compounds, such as 2-methoxy phenol and 4-ethyl-2-methoxyphenol were found in roasted coffee that undergoes a fermentation process before the drying process (full wash and wine). Microorganisms such as Saccharomyces cerevisiae through the metabolic process can produce 4-vinyl to 4-ethyl derivatives of free phenolic acids naturally found in fruit [48]. During the fermentation of coffee fruit involving certain microorganisms, phenolic acid derivative compounds, such as 2-methoxy phenol, 4-ethyl-2-methoxyphenol, and 2-methoxy-4-vinylphenol, can be formed.
The hydrocarbon and thiophene groups were present in the slightest relative amounts compared to other groups. Hydrocarbons and thiophene were most abundant in coffee processed with fermentation processes, specifically full wash and wine processes. The results suggest that fermentation could affect the synthesis of hydrocarbon and thiophene components. The total relative amounts of volatile compounds indicates that post-harvest processing, particularly fermentation, induces the synthesis of chemicals and elevates their concentration.
The percent contribution data were analyzed using OPLS-DA modeling. The OPLS-DA analysis successfully separated and differentiated the samples based on dominant volatile compounds and marker compounds associated with each processing method. The results indicated a total variance of 70.1%, with PC1 contributing 49.90% and PC2 contributing 20.20%. The model quality was validated using the R2X, R2Y, Q2, and CV ANOVA values. The OPLS-DA modeling produced R2X values of 0.81, R2Y 1 and Q2 0.846. Additionally, the CV ANOVA value was < 0.05 (0.04) and the regression value was > 0.4, confirming the validity of the model [49]. To further validate the statistical model, 200 permutation tests were conducted. The permutation test demonstrated the model's validity, as evidenced by a lower Q2 prediction intercept (-0.701) compared to the Q2 value obtained through analysis. These results indicated that the model can distinguish volatile compounds in second-crack roasted coffee from four different processing methods. The OPLS-DA loading plot is presented in Figure 5.
OPLS-DA analysis successfully grouped compounds based on their contribution to each processing group. Several compounds were identified in one or more processes, indicating their potential as volatile markers of second-crack roasted coffee from each processing method, as shown in Table 2. The VIP value was used as a criterion to identify compounds with the best discriminant value. According to the results, all identified compounds had the potential to serve as marker compounds for each processing method, as they exhibited VIP values greater than 0.8 [50]. These marker compounds significantly influenced the discrimination of processing differences in second-crack roasted coffee. Additionally, the percent contribution value was used to assess the importance of each marker compound. Marker compounds with a VIP value > 0.8 and a percent contribution value > 0.1% were considered potential marker compounds.
Potential markers | Percent contribution (%) | VIP | |||
Natural | Honey | Full wash | Wine | ||
ethyl acetate | nd | nd | nd | 0, 26 | 0, 98 |
5-amino-2-methylbenzothiazole | nd | nd | nd | 0, 18 | 0, 98 |
ethyl salicylate | 0, 15 | nd | nd | 0, 89 | 0, 97 |
2-methyl-5-methoxy-4H-pyran-4-one | 0, 23 | nd | nd | 0, 24 | 1, 16 |
Data were the averages of two replicates. nd (not detected). VIP (variable importance projection). |
Based on the VIP values and the percent contribution of volatile marker compounds successfully identified in second-crack roasted coffee, both natural and wine processing exhibited two potential marker compounds: ethyl salicylate and 2-Methyl-5-methoxy-4H-pyran-4-one. Ethyl salicylate an ester derivative compound with a minty aroma, was reported to be slightly affected by temperature and roasting time due to its high boiling point [51]. It was also reported by [52] that this compound is found in fresh coffee pulp. Ethyl salicylate was only detected in coffee that did not undergo a fruit skin peeling process, such as natural and wine processing, and was thought to be absent in coffee subjected to fruit skin peeling processes, like honey and full wash processing.
Ethyl acetate and 5-amino-2-methylbenzothiazole were identified as markers in second-crack roasted coffee from wine processing. Ethyl acetate, a volatile compound from the carboxylic acid class, is associated with a fruity wine aroma, characteristic of fermented products [53]. One factor contributing to the increased concentration of ethyl acetate is the duration of the fermentation process. According to [54], increasing the duration of anaerobic fermentation of coffee enhances the production of ethyl acetate. Another marker compound in wine processing was 5-amino-2-methylbenzothiazole. While limited information is available on this compound, it has not been previously reported in coffee. However, it is known to belong to the benzothiazole group, a heterocyclic component likely found in roasted coffee. In this study, honey and full wash did not exhibit any potential marker compounds.
Dry aroma refers to the aroma of ground coffee before brewing, which panelists evaluated by sniffing the roasted ground coffee. In coffee taste testing, both dry aroma and wet aroma (aroma after brewing) are used to determine the fragrance/aroma score [15]. The taste assessment conducted by the Indonesian Coffee and Cocoa Research Center indicated that robusta coffee from Sukamakmur District, Bogor, used in this study had characteristics of caramelly, chocolaty, spicy, and acidy aromas in coffee with a medium roast level [55]. The roast level greatly influences the characteristics of the coffee aroma.
This study selected 46 compounds with a percent contribution value of > 0.5% for analysis to identify the dry aroma of second-cracked roasted coffee from each processing. The OPLS-DA analysis and sensory test are displayed in the loading plot shown in Figure 6. The dry aroma sensory test results revealed that natural roasted coffee exhibited caramelly, roasted peanut, and chocolaty aromas. Honey processed coffee had caramelly, nutty, and earthy aromas. Full wash processed coffee had sweet nut, earthy, and herbal aromas with a hint of potato. Wine-roasted coffee exhibited fermented, winey, molasses, and chocolaty aromas. The OPLS-DA analysis results (Figure 6) showed that natural and honey-processed coffee were positioned on the positive PC1 plane, alongside compounds contributing to sweet, nutty, and roasted aroma types. In both natural and honey processing, the most intense aroma was caramelly, associated with compounds, such as hydroxyacetone, furfural, 5-methylfurfural, dihydro-2-methyl-3-furanone, 2-furanmethanol, and 3-Ethyl-2-hydroxy-2-cyclopentenone. The roasted peanut and chocolaty aromas in natural processed coffee were likely influenced by the presence of the 2-ethylpyrazine compound. The nutty aroma in honey processed coffee was probably due to the 2-methylpyrazine compound. Additionally, the 2-furanmethanol compound, which contributes to the caramelly aroma, was also a key contributor to the earthy aroma of honey-roasted coffee.
Full wash roasted coffee appeared on the negative PC1 plane, along with compounds that contributed to green, earthy, nutty aroma. The sweet nut aroma was likely influenced by compounds such as 2-acetylpyrrole and difurfuryl ether. The earthy aroma was thought to be influenced by the compounds like 2-formylpyrrole, phenol, and 3-methylcrotonic acid. Additionally, the aroma reminiscent of herb hint potato was attributed to the compound 1-furfurylpyrrole.
Wine-roasted coffee was positioned on the negative PC2 plane, associated with compounds contributing acidic, alcoholic, and chocolaty aroma. Acetic acid and ethyl alcohol were likely responsible for fermented, winey, and molasses aromas in wine-roasted coffee, while the chocolaty aroma was thought to be influenced by the compound 2,6-dimethylhydrazine. The description of these aroma compounds can be found in Table 3. The results indicated that the dry aroma of the second-crack roasted coffee from different processes (natural, honey, full wash, and wine) could be clearly distinguished.
Processing | Compounds | Aroma description* |
Natural and Honey | 2-ethyl pyrazine (Natural) | nutty, musty, fermented, coffee, roasted, cocoa, meaty |
hydroxyacetone (Natural) | sweet caramellic, ethereal | |
furfural | sweet, brown woody, bready, caramellic, phenolic | |
5-methylfurfural | sweet, caramellic, bready, brown, coffee-like | |
dihydro-2-methyl-3-furanone | sweet, caramel, bread | |
2-furfuryl-acetate (Honey) | fruity, roast, sweet | |
2-methyl pyrazine (Honey) | nutty, cocoa roasted, chocolate, peanut, green | |
2-furanmethanol (Honey) | alcoholic, chemical, musty, sweet caramel, bread, coffee | |
3-Ethyl-2-hydroxy-2-cyclopentenone (Honey) | maple, caramel, coffee, smoke | |
Full wash | 2-formylpyrrole | musty, beefy, coffee |
phenol | phenolic, plastic, rubbery | |
2-acetylpyrrole | musty, nutty, coumarinic | |
1-furfurylpyrrole | vegetative, cereal, bready, radish, mushroom, potato nuances | |
difurfuryl ether | coffee, nutty, earthy | |
3-methylcrotonic acid | green, phenolic, dairy | |
Wine | acetic acid | acidic, vinegar, sour, sharp |
ethyl alcohol | alcoholic, ethereal, medicinal | |
2,6-dimethylpyrazine | chocolate, nutty, roasted | |
(*) The aroma description refers to Thegoodscentcompany.com. |
The second-crack roast produced coffee at a medium-to-dark roast level across all processing methods. Out of 140 compounds identified and semiquantified, second-crack roasted coffee was dominated by furan, pyrazine, carboxylic acid, phenolic, and alcohol groups. Carboxylic acids, esters, phenolics, alcohols, hydrocarbons, and thiophenes were influenced by post-harvest processing. PCA modeling showed that natural and honey-processed coffee had similar volatile compositions, while full wash and wine-processed coffee exhibited distinct differences. Regarding the VIP value from OPLS-DA modeling and the percent contribution value, ethyl salicylate and 2-Methyl-5-methoxy-4H-pyran-4-one were identified as potential volatile marker compounds in natural and wine processing. In contrast, ethyl acetate and 5-amino-2-methylbenzothiazole were identified as potential marker compounds for wine-processed coffee. Honey and full wash processing did not present potential marker compounds. The dry aroma of natural roasted coffee was characterized by caramelly, roasted peanut, and chocolaty aromas. Honey processed coffee had caramelly, nutty, and earthy aromas. Full wash processed coffee exhibited sweet nut, earthy, herbal aromas with a hint of potato. Wine-processed coffee had fermented, winey, molasses, and chocolaty aromas. The evaluation of dry aroma through sensory analysis distinguished between natural, honey, full wash, and wine-roasted coffee.
Future research would focus on identifying types of microorganisms present in each processing method and investigating the mechanisms behind the formation of potential marker compounds in natural and wine processed coffee.
The authors state that they did not utilize Artificial Intelligence (AI) tools in creating this article.
The authors acknowledge the assistance of Sukamakmur farmers in providing green coffee bean samples. This research was financially supported by the Directorate of Research and Community Service of the Republic of Indonesia under grant code 3807/IT3.L1/PT.01.03/P/B/ 2022.
All authors declare that they have no conflict of interest.
Investigation: N.F.S.; Methodology: N.F.S and N.A.; Formal analysis: N.F.S; Project administration, N.F.S; Writing - Original draft: N.F.S.; writing—review and editing, D.N.F., D.R.A., and N.A. Supervision: D.N.F., D.R.A., and N.A. Funding acquisition: N.A. All authors have read and agreed to the published version of the manuscript.
[1] |
Suaib NF, Faridah DN, Adawiyah DR, et al. (2024) Authentication of volatile and non-volatile compounds in Robusta Java Bogor as a differentiator in post-harvest processes. BIO Web Conf 123: 1–15. http://dx.doi.org/10.1051/bioconf/202412301002 doi: 10.1051/bioconf/202412301002
![]() |
[2] | Kreicbergs V, Dimins F, Mikelsone V, et al. (2011) Biologically active compounds in roasted coffee. In: Proceedings of the 6th Baltic Conference on Food Science and Technology: Innovations for Food Science and Production, FOODBALT, Jelgava, Latvia, 110–115. |
[3] | Lambot C, Herrera JC, Bertrand B, et al. (2017) Cultivating coffee quality-terroir and agro-ecosystem. In: Folmer B (Ed.), The Craft and Science of Coffee, Elsevier, 17–49. https://doi.org/10.1016/B978-0-12-803520-7.00002-5 |
[4] |
Várady M, Tauchen J, Fraňková A, et al. (2022) Effect of method of processing specialty coffee beans (natural, washed, honey, fermentation, maceration) on bioactive and volatile compounds. LWT 172: 1–8. https://doi.org/10.1016/j.lwt.2022.114245 doi: 10.1016/j.lwt.2022.114245
![]() |
[5] |
Münchow M, Alstrup J, Steen I, et al. (2020) Roasting conditions and coffee flavor: A multi-study empirical investigation. Beverages 6: 1–14. http://dx.doi.org/10.3390/beverages6020029 doi: 10.3390/beverages6020029
![]() |
[6] | Schenker S, Rothgeb T (2017) The Roast-creating the beans' signature. In: Folmer B (Ed.), The Craft and Science of Coffee, Elsevier, 245–271. https://doi.org/10.1016/B978-0-12-803520-7.00011-6 |
[7] |
Poyraz İE, Öztürk N, Kıyan HT, et al. (2016) Volatile compounds of Coffea arabica L. green and roasted beans. Anadolu University Journal of Science and Technology C Life Sciences and Biotechnology 5: 31–35. http://dx.doi.org/10.18036/btdc.13390 doi: 10.18036/btdc.13390
![]() |
[8] |
Yergenson N, Aston DE (2020) Monitoring coffee roasting cracks and predicting with in situ near-infrared spectroscopy. J Food Process Eng 43: 1–10. https://doi.org/10.1111/jfpe.13305 doi: 10.1111/jfpe.13305
![]() |
[9] |
Cortés-Macías ET, López CF, Gentile P, et al. (2022) Impact of post-harvest treatments on physicochemical and sensory characteristics of coffee beans in Huila, Colombia. Postharvest Biol Technol 187: 1–9. https://doi.org/10.1016/j.postharvbio.2022.111852 doi: 10.1016/j.postharvbio.2022.111852
![]() |
[10] |
Hernández JA, Heyd B, Trystram G (2008) Prediction of brightness and surface area kinetics during coffee roasting. J Food Eng 89: 156–163. https://doi.org/10.1016/j.jfoodeng.2008.04.026 doi: 10.1016/j.jfoodeng.2008.04.026
![]() |
[11] |
Franca AS, Oliveira LS, Oliveira RCS, et al. (2009) A preliminary evaluation of the effect of processing temperature on coffee roasting degree assessment. J Food Eng 92: 345–352. https://doi.org/10.1016/j.jfoodeng.2008.12.012 doi: 10.1016/j.jfoodeng.2008.12.012
![]() |
[12] |
Melo Pereira GV d, Carvalho Neto DP d, Magalhães Júnior AI, et al. (2019) Exploring the impacts of postharvest processing on the aroma formation of coffee beans—A review. Food Chem 272: 441–452. https://doi.org/10.1016/j.foodchem.2018.08.061 doi: 10.1016/j.foodchem.2018.08.061
![]() |
[13] |
Gonzalez-Rios O, Suarez-Quiroz ML, Boulanger R, et al. (2007) Impact of 'ecological' post-harvest processing on coffee aroma: Ⅱ. Roasted coffee. J Food Compos Anal 20: 297–307. https://doi.org/10.1016/j.jfca.2006.12.004 doi: 10.1016/j.jfca.2006.12.004
![]() |
[14] |
Barea-Ramos JD, Cascos G, Mesías M, et al. (2022) Evaluation of the olfactory quality of roasted coffee beans using a digital nose. Sensors 22: 1–14. https://doi.org/10.3390/s22228654 doi: 10.3390/s22228654
![]() |
[15] | Hetzel A (2015) Fine robusta standards and protocols—A compilation of technical standards, evaluation procedures and reference materials for quality-differentiated robusta coffee, California, USA: Coffee Quality Institute, 1–47. |
[16] |
Ongo EA, Montevecchi G, Antonelli A, et al. (2020) Metabolomics fingerprint of philippine coffee by SPME-GC-MS for geographical and varietal classification. Food Res Int 134: 1–24. https://doi.org/10.1016/j.foodres.2020.109227 doi: 10.1016/j.foodres.2020.109227
![]() |
[17] |
Senizza A, Rocchetti G, Callegari ML, et al. (2020) Linoleic acid induces metabolic stress in the intestinal microorganism Bifidobacterium breve DSM 20213. Sci Rep 10: 1–11. https://doi.org/10.1038/s41598-020-62897-w doi: 10.1038/s41598-020-62897-w
![]() |
[18] |
Wilson PS (2014) Coffee roasting acoustics. J Acoust Soc Am 135: 265–269. https://doi.org/10.1121/1.4874355 doi: 10.1121/1.4874355
![]() |
[19] | Farah A (2012) Coffee constituents. In: Chu Y-F (Ed.), Coffee: Emerging Health Effects and Disease Prevention, England: John Wiley and Sons, 21–58. http://dx.doi.org/10.1002/9781119949893.ch2 |
[20] | Al-Shemmeri M (2023) Effect of post-harvest processing on coffee's roasting performance. Medium, 1–19. Available from: https://medium.com/@markalshemmeri/effect-of-post-harvest-processing-on-coffees-roasting-performance-130bdfe2da36. |
[21] | Dupres L (2023) How to get the perfect coffee roasting temperature every time. In: Brew coffee Daily. Available from https://www.brewcoffeedaily.com/guides/roasting/coffee-roasting-temperature/. |
[22] | Haile M, Hee Kang W (2020) The harvest and post-harvest management practices' impact on coffee quality. In: Castanheira DT (Ed.), Coffee-Production and Research, IntechOpen. 1–18. https://doi.org/10.5772/intechopen.89224 |
[23] | Gruczyńska E, Kowalska D, Kozłowska M, et al. (2018) Furan in roasted, ground and brewed coffee. Rocz Panstw Zakl Hig 69: 111–118. |
[24] |
Umano K, Hagi Y, Nakahara K, et al. (1995) Volatile chemicals formed in the headspace of a heated D-glucose/ L-cysteine maillard model system. J Agric Food Chem 43: 2212–2218. https://doi.org/10.1021/jf00056a046 doi: 10.1021/jf00056a046
![]() |
[25] |
Cannon RJ, Trinnaman L, Grainger B, et al. (2010) The key odorants of coffee from various geographical locations. ACS Symposium Series, American Chemical Society, 77–90. http://dx.doi.org/10.1021/bk-2010-1036.ch006 doi: 10.1021/bk-2010-1036.ch006
![]() |
[26] |
Afriliana A, Pratiwi D, Giyarto, et al. (2019) Volatile compounds changes in unfermented robusta coffee by re-fermentation using commercial kefir. Nutr Food Sci Int J 8: 1–6. https://doi.org/10.19080/nfsij.2019.08.555745 doi: 10.19080/nfsij.2019.08.555745
![]() |
[27] |
Liu C, Yang Q, Linforth R, et al. (2019) Modifying robusta coffee aroma by green bean chemical pre-treatment. Food Chem 272: 251–257. https://doi.org/10.1016/j.foodchem.2018.07.226 doi: 10.1016/j.foodchem.2018.07.226
![]() |
[28] |
Leino M, Lapveteläinen A, Menchero P, et al. (1991) Characterisation of stored arabica and robusta coffees by headspace-GC and sensory analysis. Food Qual Prefer 2: 115–125. https://doi.org/10.1016/0950-3293(91)90031-9 doi: 10.1016/0950-3293(91)90031-9
![]() |
[29] |
Pavesi Arisseto A, Vicente E, Soares Ueno M, et al. (2011) Furan levels in coffee as influenced by species, roast degree, and brewing procedures. J Agric Food Chem 59: 3118–3124. https://doi.org/10.1021/jf104868g doi: 10.1021/jf104868g
![]() |
[30] |
Guenther H, Hoenicke K, Biesterveld S, et al. (2010) Furan in coffee: Pilot studies on formation during roasting and losses during production steps and consumer handling. Food Addit Contam 27: 283–290. https://doi.org/10.1080/19440040903317505 doi: 10.1080/19440040903317505
![]() |
[31] |
Schenker S, Heinemann C, Huber M, et al. (2002) Impact of roasting conditions on the formation of aroma compounds in coffee beans. J Food Sci 67: 60–66. https://doi.org/10.1111/j.1365-2621.2002.tb11359.x doi: 10.1111/j.1365-2621.2002.tb11359.x
![]() |
[32] |
Moon JK, Shibamoto T (2009) Role of roasting conditions in the profile of volatile flavor chemicals formed from coffee beans. J Agric Food Chem 57: 5823–5831. https://doi.org/10.1021/jf901136e doi: 10.1021/jf901136e
![]() |
[33] |
Ku Madihah KY, Zaibunnisa AH, Norashikin S, et al. (2012) Optimization of roasting conditions for high-quality robusta coffee. APCBEE Procedia 4: 209–214. https://doi.org/10.1016/j.apcbee.2012.11.035 doi: 10.1016/j.apcbee.2012.11.035
![]() |
[34] |
Cao X, Wu H, Viejo CG, et al. (2023) Effects of postharvest processing on aroma formation in roasted coffee—A review. Int J Food Sci Technol 58: 1007–1027. http://dx.doi.org/10.1111/ijfs.16261 doi: 10.1111/ijfs.16261
![]() |
[35] |
Agnoletti Z, Dos W, Gomes S, et al. (2022) Effect of fermentation on the quality of conilon coffee (Coffea canephora): Chemical and sensory aspects rights and content. Microchem J 182: 107966. https://doi.org/10.1016/j.microc.2022.107966 doi: 10.1016/j.microc.2022.107966
![]() |
[36] |
Zofia NŁ, Aleksandra Z, Tomasz B, et al. (2020) Effect of fermentation time on antioxidant and anti-ageing properties of green coffee kombucha ferments. Molecules 25: 1–26. https://doi.org/10.3390/molecules25225394 doi: 10.3390/molecules25225394
![]() |
[37] |
Galarza G, Figueroa JG (2022) Volatile compound characterization of coffee (Coffea arabica) processed at different fermentation times using SPME–GC–MS. Molecules 27: 1–15. https://doi.org/10.3390/molecules27062004 doi: 10.3390/molecules27062004
![]() |
[38] |
Zhang L, Cao Y, Tong J, et al. (2019) An alkylpyrazine synthesis mechanism involving L-threonine-3-dehydrogenase describes the production of 2,5-dimethylpyrazine and 2, 3,5-trimethylpyrazine by Bacillus subtilis. Appl Environ Microbiol 85: 1–15. https://doi.org/10.1128/aem.01807-19 doi: 10.1128/aem.01807-19
![]() |
[39] |
Müller R, Rappert S (2010) Pyrazines: Occurrence, formation and biodegradation. Appl Microbiol Biotechnol 85: 1315–1320. https://doi.org/10.1007/s00253-009-2362-4 doi: 10.1007/s00253-009-2362-4
![]() |
[40] |
Kłosowski G, Mikulski D, Pielech-Przybylska K (2021) Pyrazines biosynthesis by Bacillus strains isolated from natto fermented soybean. Biomolecules 11: 1–12. https://doi.org/10.3390/biom11111736 doi: 10.3390/biom11111736
![]() |
[41] |
Arkadaş M (2018) Formation of volatile compounds in double roasted antakya coffee. Journal of Nutrition, Food Research and Technology 1: 19–22. http://dx.doi.org/10.30881/jnfrt.00006 doi: 10.30881/jnfrt.00006
![]() |
[42] |
Dippong T, Dan M, Kovacs MH, et al. (2022) Analysis of volatile compounds, composition, and thermal behavior of coffee beans according to variety and roasting intensity. Foods 11: 1–15. https://doi.org/10.3390/foods11193146 doi: 10.3390/foods11193146
![]() |
[43] | Pereira LL, Júnior DB, Pimenta de Sousa LHB, et al. (2021) Relationship between coffee processing and fermentation. In: Pereira LL (Ed.), Quality Determinants in Coffee Production, Switzerland: Springer, 255–301. https://doi.org/10.1007/978-3-030-54437-9_6 |
[44] |
Haile M, Kang WH (2019) The role of microbes in coffee fermentation and their impact on coffee quality. J Food Qual 2019: 1–6. https://doi.org/10.1155/2019/4836709 doi: 10.1155/2019/4836709
![]() |
[45] |
de Bruyn F, Zhang SJ, Pothakos V, et al. (2017) Exploring the impacts of postharvest processing on the microbiota and metabolite profiles during green coffee bean production. Appl Environ Microbiol 83: 1–40. https://doi.org/10.1128/AEM.02398-16 doi: 10.1128/AEM.02398-16
![]() |
[46] |
Miao Y, Zou Q, Wang Q, et al. (2022) Evaluation of the physiochemical and metabolite of different region coffee beans by using UHPLC-QE-MS untargeted-metabonomics approaches. Food Biosci 46: 1–39. https://doi.org/10.1016/j.fbio.2022.101561 doi: 10.1016/j.fbio.2022.101561
![]() |
[47] |
Lee BH, Huang CH, Liu TY, et al. (2023) Microbial diversity of anaerobic-fermented coffee and potential for inhibiting ochratoxin-produced Aspergillus niger. Foods 12: 1–17. https://doi.org/10.3390/foods12152967 doi: 10.3390/foods12152967
![]() |
[48] |
Smit A, Cordero Otero RR, Lambrechts MG, et al. (2003) Enhancing volatile phenol concentrations in wine by expressing various phenolic acid decarboxylase genes in Saccharomyces cerevisiae. J Agric Food Chem 51: 4909–4915. https://doi.org/10.1021/jf026224d doi: 10.1021/jf026224d
![]() |
[49] |
Yang Y, Wu Z, Li S, et al. (2020) Targeted blood metabolomic study on retinopathy of prematurity. Invest Ophthalmol Vis Sci 61: 1–10. https://doi.org/10.1167/iovs.61.2.12 doi: 10.1167/iovs.61.2.12
![]() |
[50] |
Rha CS, Jang EK, Hong YD, et al. (2021) Supervised statistical learning prediction of soybean varieties and cultivation sites using rapid uplc-ms separation, method validation, and targeted metabolomic analysis of 31 phenolic compounds in the leaves. Metabolites 11: 3–18. https://doi.org/10.3390/metabo11120884 doi: 10.3390/metabo11120884
![]() |
[51] |
Nebesny E, Budryn G, Kula J, et al. (2007) The effect of roasting method on headspace composition of robusta coffee bean aroma. European Food Research and Technology 225: 9–19. https://doi.org/10.1007/s00217-006-0375-0 doi: 10.1007/s00217-006-0375-0
![]() |
[52] |
Mathieu F, Malosse C, Frérot B (1998) Identification of the volatile components released by fresh coffee berries at different stages of ripeness. J Agric Food Chem 46: 1106–1110. https://doi.org/10.1021/jf970851z doi: 10.1021/jf970851z
![]() |
[53] |
Li S, Tian Y, Sun M, et al. (2022) Characterization of key aroma compounds in fermented bamboo shoots using gas chromatography-olfactometry-mass spectrometry, odor activity values, and aroma recombination experiments. Foods 11: 1–13. https://doi.org/10.3390/foods11142106 doi: 10.3390/foods11142106
![]() |
[54] |
da Silva Vale A, Balla G, Rodrigues LRS, et al. (2023) Understanding the effects of self-induced anaerobic fermentation on coffee beans quality: Microbiological, metabolic, and sensory studies. Foods 12: 1–20. https://doi.org/10.3390/foods12010037 doi: 10.3390/foods12010037
![]() |
[55] | Mawardi AD, Fadli ML, Hakim AR, et al. (2020) Karakteristik lahan dalam mendukung perkembangan kopi robusta kabupaten Bogor sebagai produk indikasi geografis. RADAR 1: 1–6. |
Compound | RT (min) | RI | Relative amount | |||
Natural | Honey | Full wash | Wine | |||
Aldehyde | ||||||
acetaldehyde | 1.59 | - | 0.23 ± 0.04 | 0.31 ± 0.00 | 0.29 ± 0.01 | 0.23 ± 0.03 |
2-methylbutanal | 2.57 | 912 | 0.49 ± 0.05 | 0.34 ± 0.01 | 0.39 ± 0.04 | 0.75 ± 0.10 |
3-methylbutanal | 2.60 | 914 | 0.39 ± 0.02 | 0.28 ± 0.01 | 0.34 ± 0.03 | 0.50 ± 0.07 |
1-methyl-1H-pyrrole-2-carboxaldehyde | 20.93 | 1619 | 0.69 ± 0.06 | 0.72 ± 0.02 | 0.95 ± 0.03 | 0.99 ± 0.13 |
2-phenyl-2-butenal | 27.88 | 1931 | 0.30 ± 0.01 | 0.26 ± 0.02 | 0.41 ± 0.00 | 0.37 ± 0.08 |
Total Aldehydes | 2.11 ± 0.06ab | 1.90 ± 0.05a | 2.38 ± 0.03ab | 2.83 ± 0.40b | ||
Furan | ||||||
furan | 1.85 | - | 0.10 ± 0.01 | 0.09 ± 0.01 | 0.12 ± 0.01 | 0.11 ± 0.01 |
2-methylfuran | 2.23 | - | 0.27 ± 0.01 | 0.22 ± 0.01 | 0.33 ± 0.01 | 0.22 ± 0.04 |
2.5-dimethylfuran | 2.99 | 946 | 0.12 ± 0.01 | 0.10 ± 0.00 | 0.15 ± 0.01 | 0.14 ± 0.02 |
2-pentylfuran | 10.41 | 1238 | 0.14 ± 0.01 | 0.15 ± 0.01 | 0.17 ± 0.00 | 0.15 ± 0.01 |
dihydro-2-methyl-3-furanone | 11.15 | 1262 | 0.51 ± 0.05 | 0.52 ± 0.01 | 0.51 ± 0.02 | 0.32 ± 0.02 |
2-methyl tetrahydrofuran | 13.93 | 1354 | 0.14 ± 0.04 | 0.15 ± 0.02 | 0.15 ± 0.00 | 0.17 ± 0.01 |
furfural | 16.92 | 1461 | 3.23 ± 0.21 | 2.53 ± 0.13 | 2.91 ± 0.24 | 2.41 ± 0.29 |
2-furfuryl methyl sulfide | 17.56 | 1485 | 0.17 ± 0.00 | 0.20 ± 0.01 | 0.24 ± 0.00 | 0.25 ± 0.02 |
2-acetylfuran | 18.02 | 1502 | 1.00 ± 0.01 | 1.08 ± 0.03 | 1.29 ± 0.01 | 1.02 ± 0.10 |
2-furfuryl-acetate | 18.92 | 1538 | 5.84 ± 0.15 | 6.53 ± 0.55 | 6.69 ± 0.10 | 8.76 ± 0.04 |
2-furanmethanamine | 19.54 | 1563 | 0.19 ± 0.02 | 0.19 ± 0.01 | 0.28 ± 0.01 | 0.30 ± 0.06 |
5-methylfurfural | 19.76 | 1571 | 4.65 ± 0.14 | 3.70 ± 0.08 | 4.43 ± 0.21 | 3.73 ± 0.47 |
2-ethyl-5-methylfuran | 19.84 | 1575 | 0.21 ± 0.00 | 0.23 ± 0.01 | 0.28 ± 0.01 | 0.16 ± 0.03 |
2.2-methylenebisfuran | 20.71 | 1610 | 0.74 ± 0.05 | 0.75 ± 0.03 | 0.93 ± 0.01 | 0.73 ± 0.08 |
2-acetyl-5-methylfuran | 20.80 | 1613 | 0.20 ± 0.01 | 0.21 ± 0.02 | 0.25 ± 0.01 | 0.19 ± 0.01 |
2-furanmethanol | 22.02 | 1666 | 12.70 ± 0.43 | 11.87 ± 0.54 | 15.15 ± 0.65 | 14.90 ± 1.93 |
2-furfuryl-5-methylfuran | 22.40 | 1681 | 0.38 ± 0.01 | 0.45 ± 0.02 | 0.68 ± 0.01 | 0.36 ± 0.05 |
2-hexyl furan | 23.12 | 1712 | 0.19 ± 0.02 | 0.12 ± 0.01 | 0.21 ± 0.01 | 0.23 ± 0.03 |
3.4-dimethylfuran-2.5-dione | 23.46 | 1727 | 0.39 ± 0.02 | 0.36 ± 0.02 | 0.47 ± 0.02 | 0.44 ± 0.03 |
3-phenyl furan | 26.24 | 1853 | 0.53 ± 0.00 | 0.44 ± 0.01 | 0.65 ± 0.03 | 0.72 ± 0.08 |
2-methyl-3-(2-furyl)propenal | 26.71 | 1875 | 0.18 ± 0.00 | 0.17 ± 0.01 | 0.25 ± 0.01 | 0.18 ± 0.05 |
5-methyl-2-furanmethanethiol | 26.93 | 1885 | 0.18 ± 0.01 | 0.17 ± 0.02 | 0.26 ± 0.01 | 0.29 ± 0.04 |
5-ethylfuran-2-carboxylic acid | 27.02 | 1890 | 0.08 ± 0.01 | 0.08 ± 0.01 | 0.10 ± 0.03 | 0.13 ± 0.04 |
difurfuryl disulfide | 27.25 | 1900 | 0.15 ± 0.01 | 0.17 ± 0.03 | 0.28 ± 0.02 | 0.20 ± 0.06 |
2-propyl furan | 27.29 | 1902 | 0.13 ± 0.02 | 0.14 ± 0.02 | 0.18 ± 0.02 | 0.12 ± 0.02 |
furfural acetone | 27.35 | 1905 | 0.15 ± 0.01 | 0.15 ± 0.00 | 0.23 ± 0.01 | 0.24 ± 0.06 |
furfuryl ether | 29.00 | 1985 | 0.47 ± 0.02 | 0.53 ± 0.04 | 0.79 ± 0.00 | 0.53 ± 0.07 |
furaneol | 30.06 | 2038 | 0.12 ± 0.00 | 0.10 ± 0.00 | 0.16 ± 0.0- | 0.11 ± 0.03 |
2-n-butyl furan | 34.03 | 2245 | 0.14 ± 0.02 | 0.16 ± 0.02 | 0.29 ± 0.02 | 0.23 ± 0.04 |
2-ethylfuran | 34.43 | 2267 | 0.10 ± 0.03 | 0.13 ± 0.02 | 0.16 ± 0.03 | 0.14 ± 0.03 |
Total Furans | 33.39 ± 0.08a | 31.71 ± 1.56a | 38.60 ± 0.16a | 37.64 ± 3.82a | ||
Ketone | ||||||
acetone | 1.94 | - | 0.43 ± 0.04 | 0.31 ± 0.01 | 0.44 ± 0.03 | 0.52 ± 0.06 |
2.3-butanedione | 3.28 | 970 | 0.16 ± 0.00 | 0.12 ± 0.01 | 0.16 ± 0.00 | 0.13 ± 0.01 |
2.3-pentanedione | 4.91 | 970 | 0.27 ± 0.02 | 0.20 ± 0.01 | 0.21 ± 0.01 | 0.18 ± 0.02 |
3-penten-2-one | 6.82 | 1123 | 0.05 ± 0.00 | 0.04 ± 0.00 | 0.05 ± 0.00 | 0.29 ± 0.04 |
hydroxyacetone | 12.22 | 1296 | 0.62 ± 0.02 | 0.51 ± 0.02 | 0.56 ± 0.01 | 0.52 ± 0.05 |
1-acetoxy-2-butanone | 18.82 | 1534 | 0.32 ± 0.01 | 0.28 ± 0.00 | 0.32 ± 0.01 | 0.27 ± 0.01 |
1-acetylcyclohexene | 19.32 | 1554 | 0.07 ± 0.01 | 0.06 ± 0.01 | 0.08 ± 0.00 | 0.15 ± 0.01 |
2-acetylpyridine | 20.49 | 1600 | 0.18 ± 0.00 | 0.18 ± 0.01 | 0.21 ± 0.02 | 0.20 ± 0.01 |
2-acetyl-4-methylpyridine | 21.15 | 1628 | 0.09 ± 0.01 | 0.12 ± 0.00 | 0.14 ± 0.01 | 0.11 ± 0.03 |
3-ethyl-2-hydroxy-2-cyclopentenone | 22.44 | 1682 | 0.82 ± 0.03 | 0.80 ± 0.00 | 0.99 ± 0.03 | 0.85 ± 0.09 |
1-(6-methyl-2-pyrazinyl)-1-ethanone | 22.66 | 1692 | 0.63 ± 0.03 | 0.56 ± 0.01 | 0.87 ± 0.04 | 0.87 ± 0.10 |
(+)-2-bornanone | 23.18 | 1714 | 0.14 ± 0.03 | 0.12 ± 0.01 | 0.19 ± 0.03 | 0.21 ± 0.03 |
Total Ketones | 3.78 ± 0.08ab | 3.30 ± 0.03a | 4.21 ± 0.12ab | 4.29 ± 0.45b | ||
Carboxylic acid | ||||||
acetic acid | 16.62 | 1450 | 3.59 ± 0.15 | 3.09 ± 0.03 | 4.21 ± 0.08 | 6.37 ± 0.34 |
4-hydroxybutyric acid | 21.05 | 1624 | 1.46 ± 0.15 | 1.31 ± 0.08 | 1.73 ± 0.14 | 2.63 ± 0.33 |
2-methyl-2-vinylmaleimide | 21.32 | 1635 | 0.20 ± 0.01 | 0.18 ± 0.00 | 0.25 ± 0.01 | 0.27 ± 0.02 |
isovaleric acid | 22.22 | 1673 | 1.26 ± 0.10 | 1.20 ± 0.06 | 1.70 ± 0.04 | 1.34 ± 0.15 |
3-methylcrotonic acid | 25.08 | 1799 | 0.53 ± 0.03 | 0.55 ± 0.03 | 0.75 ± 0.02 | 0.67 ± 0.08 |
2-furanpropionic acid | 25.24 | 1806 | 0.24 ± 0.01 | 0.25 ± 0.03 | 0.36 ± 0.00 | 0.62 ± 0.09 |
Total Carboxylic acid* | 7.27 ± 0.45a | 6.58 ± 0.23a | 9.00 ± 0.26b | 11.89 ± 0.32c | ||
Esther | ||||||
methyl acetate | 1.99 | - | 0.12 ± 0.00 | 0.11 ± 0.01 | 0.18 ± 0.01 | 0.22 ± 0.04 |
ethyl acetate | 2.34 | - | nd | nd | nd | 0.36 ± 0.05 |
ethyl formate | 2.50 | 906 | 0.12 ± 0.01 | 0.34 ± 0.00 | 0.17 ± 0.00 | 0.24 ± 0.01 |
furfuryl propionate | 20.45 | 1599 | 0.47 ± 0.02 | 0.49 ± 0.02 | 0.52 ± 0.05 | 0.38 ± 0.03 |
furfuryl isovalerate | 22.75 | 1695 | 0.33 ± 0.02 | 0.40 ± 0.02 | 0.57 ± 0.00 | 0.34 ± 0.03 |
methyl salicylate | 24.53 | 1774 | 0.47 ± 0.02 | 0.47 ± 0.03 | 0.59 ± 0.01 | 0.57 ± 0.05 |
methyl nicotinate | 24.62 | 1778 | 0.27 ± 0.02 | 0.26 ± 0.02 | 0.36 ± 0.01 | 0.28 ± 0.04 |
ethyl salicylate | 25.31 | 1810 | 0.17 ± 0.04 | nd | nd | 1.24 ± 0.15 |
methyl 3-methylfuroate | 26.52 | 1866 | 0.47 ± 0.04 | 0.45 ± 0.03 | 0.56 ± 0.02 | 0.43 ± 0.07 |
methyl palmitate | 33.50 | 2217 | 0.10 ± 0.00 | 0.10 ± 0.01 | 0.16 ± 0.00 | 0.09 ± 0.00 |
Total Esters* | 2.53 ± 0.06a | 2.62 ± 0.14a | 3.11 ± 0.08a | 4.15 ± 0.46b | ||
Alcohol | ||||||
1.3-propanediol | 2.43 | - | 5.21 ± 0.21 | 5.70 ± 0.04 | 6.84 ± 0.35 | 6.30 ± 0.26 |
ethyl alcohol | 2.80 | 931 | 0.20 ± 0.00 | 0.12 ± 0.00 | 0.16 ± 0.01 | 0.89 ± 0.06 |
1-ethynylcyclohexanol | 21.53 | 1644 | 0.23 ± 0.01 | 0.22 ± 0.02 | 0.29 ± 0.01 | 0.28 ± 0.01 |
benzyl alcohol | 26.82 | 1880 | 0.07 ± 0.01 | 0.06 ± 0.01 | 0.11 ± 0.00 | 0.14 ± 0.02 |
phenylethyl alcohol | 27.57 | 1916 | 0.25 ± 0.01 | 0.22 ± 0.02 | 0.28 ± 0.01 | 0.48 ± 0.02 |
2-thiophenemethanol | 28.22 | 1947 | 0.13 ± 0.00 | 0.11 ± 0.00 | 0.16 ± 0.00 | 0.16 ± 0.04 |
4-pyridinemethanol | 31.33 | 2103 | 0.28 ± 0.00 | 0.30 ± 0.01 | 0.45 ± 0.01 | 0.35 ± 0.05 |
Total Alcohols* | 6.38 ± 0.21a | 6.73 ± 0.03a | 8.29 ± 0.35b | 8.60 ± 0.45b | ||
Hydrocarbons | ||||||
toluene | 4.45 | 1033 | 0.13 ± 0.01 | 0.13 ± 0.00 | 0.18 ± 0.01 | 0.18 ± 0.02 |
dodecane | 9.21 | 1199 | 0.18 ± 0.02 | 0.18 ± 0.02 | 0.19 ± 0.03 | 0.16 ± 0.02 |
2.7-dimethylundecane | 15.25 | 1400 | 0.12 ± 0.02 | 0.09 ± 0.01 | 0.14 ± 0.01 | 0.16 ± 0.02 |
4-isopropyl-m-xylene | 22.35 | 1679 | 0.23 ± 0.03 | 0.23 ± 0.03 | 0.29 ± 0.00 | 0.58 ± 0.07 |
decahydronaphthalene | 22.59 | 1689 | 0.22 ± 0.01 | 0.19 ± 0.02 | 0.24 ± 0.02 | 0.24 ± 0.02 |
pulegone | 22.89 | 1701 | 0.22 ± 0.02 | 0.22 ± 0.02 | 0.28 ± 0.00 | 0.36 ± 0.04 |
β-damascenone | 25.60 | 1823 | 0.27 ± 0.01 | 0.26 ± 0.02 | 0.40 ± 0.01 | 0.38 ± 0.02 |
Total Hydrocarbons* | 1.37 ± 0.19a | 1.30 ± 0.11a | 1.72 ± 0.03ab | 2.06 ± 0.21b | ||
Pyrrole | ||||||
1-ethylpyrrole | 7.23 | 1137 | 0.12 ± 0.02 | 0.09 ± 0.00 | 0.13 ± 0.01 | 0.10 ± 0.00 |
1-butyl pyrrole | 14.75 | 1382 | 0.06 ± 0.00 | 0.05 ± 0.01 | 0.04 ± 0.01 | 0.20 ± 0.01 |
pyrrole | 18.35 | 1515 | 0.51 ± 0.00 | 0.44 ± 0.05 | nd | 0.53 ± 0.09 |
1-methyl-2-pyrroleacetonitrile | 21.59 | 1647 | 0.43 ± 0.03 | 0.39 ± 0.02 | 0.54 ± 0.05 | 0.59 ± 0.04 |
1-furfurylpyrrole | 25.73 | 1829 | 1.45 ± 0.05 | 1.25 ± 0.01 | 1.92 ± 0.06 | 1.78 ± 0.21 |
2-acetylpyrrole | 28.76 | 1974 | 0.83 ± 0.05 | 0.91 ± 0.04 | 1.35 ± 0.06 | 1.06 ± 0.12 |
2-formylpyrrole | 29.81 | 2026 | 0.51 ± 0.01 | 0.49 ± 0.00 | 0.76 ± 0.01 | 0.66 ± 0.07 |
Total Pyrroles | 3.92 ± 0.06ab | 3.62 ± 0.13a | 4.74 ± 0.05ab | 4.92 ± 0.54b | ||
Pyridine | ||||||
pyridine | 8.65 | 1182 | 0.67 ± 0.06 | 1.07 ± 0.03 | 1.24 ± 0.24 | 0.92 ± 0.11 |
2.6-dimethylpyridin-4-amine | 15.39 | 1405 | 1.53 ± 0.18 | 1.36 ± 0.01 | 1.66 ± 0.10 | 2.47 ± 0.29 |
3-methoxypyridine | 20.05 | 1583 | 0.16 ± 0.01 | 0.13 ± 0.01 | 0.17 ± 0.01 | 0.32 ± 0.02 |
3-methylpyridine 1-oxide | 22.97 | 1705 | 0.08 ± 0.02 | 0.11 ± 0.01 | 0.12 ± 0.01 | 0.14 ± 0.02 |
3-(3-thienyl)pyridine | 28.09 | 1941 | 0.14 ± 0.00 | 0.13 ± 0.01 | 0.22 ± 0.00 | 0.27 ± 0.04 |
2-hydroxy-3-methylpyridine | 34.99 | 2297 | nd | nd | nd | 0.11 ± 0.02 |
4-hydroxypyridine | 37.02 | 2413 | 0.18 ± 0.01 | 0.20 ± 0.01 | 0.34 ± 0.00 | 0.22 ± 0.03 |
Total Pyridines | 2.77 ± 0.26a | 3.00 ± 0.08a | 3.75 ± 0.37ab | 4.44 ± 0.49b | ||
Pyrazine | ||||||
pyrazine | 9.54 | 1210 | 0.38 ± 0.05 | 0.27 ± 0.01 | 0.38 ± 0.01 | 0.36 ± 0.03 |
2-methylpyrazine | 11.25 | 1265 | 3.49 ± 0.30 | 3.02 ± 0.02 | 3.88 ± 0.22 | 3.80 ± 0.47 |
2.5-dimethylpyrazine | 12.98 | 1322 | 1.75 ± 0.12 | 1.69 ± 0.03 | 2.08 ± 0.16 | 2.51 ± 0.27 |
2.6-dimethylpyrazine | 13.18 | 1329 | 1.87 ± 0.12 | 1.77 ± 0.02 | 2.32 ± 0.15 | 2.63 ± 0.33 |
2-ethylpyrazine | 13.33 | 1334 | 1.30 ± 0.07 | 1.17 ± 0.01 | 1.45 ± 0.02 | 1.58 ± 0.20 |
2.3-dimethyl pyrazine | 13.69 | 1346 | 0.48 ± 0.04 | 0.43 ± 0.02 | 0.53 ± 0.04 | 0.82 ± 0.09 |
2-ethyl-6-methylpyrazine | 14.85 | 1386 | 1.77 ± 0.16 | 1.62 ± 0.01 | 2.13 ± 0.04 | 2.39 ± 0.27 |
2-ethyl-5-methylpyrazine | 15.02 | 1392 | 1.30 ± 0.11 | 1.26 ± 0.01 | 1.47 ± 0.03 | 1.86 ± 0.21 |
2.6-diethylpyrazine | 16.22 | 1436 | 0.47 ± 0.03 | 0.44 ± 0.02 | 0.55 ± 0.00 | 0.69 ± 0.08 |
3-ethyl-2.5-dimethylpyrazine | 16.54 | 1447 | 1.89 ± 0.32 | 1.59 ± 0.07 | 1.83 ± 0.02 | 2.58 ± 0.30 |
2.3-diethyl pyrazine | 16.78 | 1456 | 0.20 ± 0.01 | 0.17 ± 0.01 | 0.21 ± 0.01 | 0.23 ± 0.01 |
2-ethyl-3.5-dimethylpyrazine | 17.03 | 1466 | 2.15 ± 0.10 | 1.80 ± 0.09 | 2.35 ± 0.09 | 2.94 ± 0.34 |
2-methyl-5-propylpyrazine | 17.37 | 1478 | 0.18 ± 0.02 | 0.16 ± 0.01 | 0.22 ± 0.01 | 0.32 ± 0.02 |
2-ethenyl-6-methylpyrazine | 17.65 | 1488 | 0.28 ± 0.02 | 0.24 ± 0.01 | 0.32 ± 0.01 | 0.46 ± 0.03 |
3.5-diethyl-2-methylpyrazine | 17.85 | 1496 | 1.37 ± 0.10 | 1.21 ± 0.08 | 1.45 ± 0.05 | 1.74 ± 0.21 |
2.3.5-trimethyl-6-ethyl pyrazine | 18.43 | 1519 | 0.47 ± 0.04 | 0.43 ± 0.03 | 0.53 ± 0.04 | 0.71 ± 0.09 |
2.5-dimethyl-3-(2-methylpropyl) pyrazine | 18.70 | 1530 | 0.46 ± 0.02 | 0.34 ± 0.02 | 0.43 ± 0.01 | 0.58 ± 0.03 |
1-propenylpyrazine | 19.21 | 1550 | 0.11 ± 0.01 | 0.09 ± 0.01 | 0.12 ± 0.01 | 0.25 ± 0.02 |
isopropenyl pyrazine | 20.39 | 1596 | 0.21 ± 0.00 | 0.21 ± 0.00 | 0.29 ± 0.04 | 0.24 ± 0.01 |
2-isoamyl-6-methylpyrazine | 21.24 | 1632 | 0.14 ± 0.02 | 0.08 ± 0.01 | 0.13 ± 0.00 | 0.59 ± 0.07 |
2-methyl-6-(1-propenyl)pyrazine | 21.89 | 1659 | 0.10 ± 0.01 | 0.09 ± 0.01 | 0.14 ± 0.00 | 0.19 ± 0.04 |
pyrazinamide | 23.24 | 1717 | 0.96 ± 0.13 | 0.74 ± 0.02 | 1.13 ± 0.23 | 0.88 ± 0.08 |
2-acetyl-3.5-dimethylpyrazine | 24.79 | 1786 | 0.18 ± 0.00 | 0.16 ± 0.02 | 0.24 ± 0.00 | 0.46 ± 0.07 |
Total Pyrazines | 21.49 ± 1.54ab | 19.00 ± 0.44a | 24.17 ± 1.00ab | 28.80 ± 3.29b | ||
Phenolic | ||||||
2.3-dimethylphenol | 11.96 | 1287 | 0.15 ± 0.03 | 0.12 ± 0.01 | 0.21 ± 0.01 | 0.31 ± 0.04 |
4-amino-3-methylphenol | 20.66 | 1608 | 0.22 ± 0.06 | 0.22 ± 0.00 | 0.28 ± 0.02 | 0.37 ± 0.05 |
2-methoxyhydroquinone | 23.72 | 1738 | 0.18 ± 0.00 | 0.18 ± 0.02 | 0.24 ± 0.01 | 0.24 ± 0.02 |
4-amino-2-hydroxytoluene | 25.00 | 1795 | 0.16 ± 0.01 | 0.15 ± 0.02 | 0.26 ± 0.01 | 0.24 ± 0.03 |
guaiacol | 26.41 | 1861 | 1.38 ± 0.08 | 1.17 ± 0.00 | 1.97 ± 0.04 | 1.88 ± 0.21 |
phenol | 29.44 | 2007 | 0.66 ± 0.06 | 0.69 ± 0.04 | 1.25 ± 0.07 | 0.83 ± 0.11 |
4-ethyl-2-methoxyphenol | 29.92 | 2031 | 1.49 ± 0.05 | 1.29 ± 0.10 | 2.17 ± 0.06 | 2.33 ± 0.28 |
p-cresol | 31.10 | 2091 | 0.18 ± 0.00 | 0.19 ± 0.02 | 0.30 ± 0.02 | 0.25 ± 0.03 |
2-methoxy-4-vinylphenol | 33.06 | 2193 | 5.60 ± 0.80 | 4.09 ± 0.14 | 6.94 ± 0.78 | 5.22 ± 0.66 |
Total Phenolics* | 10.01 ± 0.52ab | 8.10 ± 0.36a | 13.61 ± 0.73c | 11.68 ± 1.43bc | ||
Thiopen | ||||||
3-ethyl thiophene | 10.09 | 1228 | 0.05 ± 0.01 | 0.04 ± 0.00 | 0.05 ± 0.00 | 0.14 ± 0.02 |
2-methylthiolan-3-one | 18.62 | 1526 | 0.17 ± 0.00 | 0.16 ± 0.00 | 0.17 ± 0.00 | 0.15 ± 0.01 |
3-acetyl-2.5-dimethylthiophene | 25.41 | 1814 | 0.28 ± 0.01 | 0.25 ± 0.03 | 0.33 ± 0.00 | 0.49 ± 0.05 |
Total Thiopens* | 0.49 ± 0.00a | 0.44 ± 0.03a | 0.55 ± 0.00a | 0.77 ± 0.08b | ||
Miscellaneous | ||||||
sec-butylamine | 1.36 | - | 0.54 ± 0.02 | 0.44 ± 0.03 | 0.59 ± 0.01 | 0.46 ± 0.05 |
1-methylpiperidine | 4.29 | 1027 | 0.07 ± 0.00 | 0.09 ± 0.00 | 0.12 ± 0.01 | 0.15 ± 0.01 |
4-methylthiazole | 11.73 | 1280 | 0.11 ± 0.01 | 0.08 ± 0.00 | 0.09 ± 0.00 | 0.19 ± 0.00 |
butyl ethyl ether | 13.52 | 1340 | 0.12 ± 0.00 | 0.11 ± 0.00 | 0.12 ± 0.01 | 0.12 ± 0.00 |
diisopropyl ether | 14.02 | 1357 | 0.11 ± 0.00 | 0.11 ± 0.01 | 0.11 ± 0.00 | 0.23 ± 0.01 |
5-amino-2-methylbenzothiazole | 19.04 | 1543 | nd | nd | nd | 0.25 ± 0.02 |
benzylhydrazine | 21.39 | 1638 | 0.14 ± 0.01 | 0.11 ± 0.01 | 0.14 ± 0.01 | 0.22 ± 0.05 |
p-aminoanisole | 21.80 | 1655 | 0.56 ± 0.00 | 0.58 ± 0.03 | 0.78 ± 0.00 | 0.71 ± 0.08 |
4-methoxyphenyl isothiocyanate | 23.54 | 1730 | 0.22 ± 0.02 | 0.20 ± 0.01 | 0.31 ± 0.01 | 0.38 ± 0.06 |
4-methoxythiophenol | 23.66 | 1736 | 0.84 ± 0.03 | 0.76 ± 0.02 | 0.89 ± 0.01 | 0.68 ± 0.09 |
2-methyl-5-methoxy-4h-pyran-4-one | 25.90 | 1837 | 0.25 ± 0.00 | nd | nd | 0.33 ± 0.05 |
6-butyltetralin | 32.02 | 2139 | 0.14 ± 0.00 | 0.12 ± 0.01 | 0.22 ± 0.00 | 0.18 ± 0.03 |
caprolactam | 32.98 | 2189 | 0.09 ± 0.00 | 0.09 ± 0.01 | 0.16 ± 0.03 | 0.08 ± 0.00 |
indole | 37.41 | 2433 | 0.15 ± 0.00 | 0.12 ± 0.02 | 0.21 ± 0.00 | 0.15 ± 0.01 |
Total Miscellaneous | 3.34 ± 0.10ab | 2.82 ± 0.15a | 3.74 ± 0.04ab | 4.14 ± 0.46b | ||
Total Volatile Compounds* | 99.91 ± 0.95ab | 92.10 ± 4.31a | 119.08 ± 0.38bc | 127.69 ± 11.08c | ||
Data were the averages ± standard deviation of two replicates. Numbers followed by different superscript letters in the same row indicate significant differences (p < 0.05) based on one-way ANOVA and Duncan test. Groups with (*) were affected by processing. RT (retention time). RI (retention index). Compounds grouping refers to pubchem.ncbi.nlm.nih.gov. |
Potential markers | Percent contribution (%) | VIP | |||
Natural | Honey | Full wash | Wine | ||
ethyl acetate | nd | nd | nd | 0, 26 | 0, 98 |
5-amino-2-methylbenzothiazole | nd | nd | nd | 0, 18 | 0, 98 |
ethyl salicylate | 0, 15 | nd | nd | 0, 89 | 0, 97 |
2-methyl-5-methoxy-4H-pyran-4-one | 0, 23 | nd | nd | 0, 24 | 1, 16 |
Data were the averages of two replicates. nd (not detected). VIP (variable importance projection). |
Processing | Compounds | Aroma description* |
Natural and Honey | 2-ethyl pyrazine (Natural) | nutty, musty, fermented, coffee, roasted, cocoa, meaty |
hydroxyacetone (Natural) | sweet caramellic, ethereal | |
furfural | sweet, brown woody, bready, caramellic, phenolic | |
5-methylfurfural | sweet, caramellic, bready, brown, coffee-like | |
dihydro-2-methyl-3-furanone | sweet, caramel, bread | |
2-furfuryl-acetate (Honey) | fruity, roast, sweet | |
2-methyl pyrazine (Honey) | nutty, cocoa roasted, chocolate, peanut, green | |
2-furanmethanol (Honey) | alcoholic, chemical, musty, sweet caramel, bread, coffee | |
3-Ethyl-2-hydroxy-2-cyclopentenone (Honey) | maple, caramel, coffee, smoke | |
Full wash | 2-formylpyrrole | musty, beefy, coffee |
phenol | phenolic, plastic, rubbery | |
2-acetylpyrrole | musty, nutty, coumarinic | |
1-furfurylpyrrole | vegetative, cereal, bready, radish, mushroom, potato nuances | |
difurfuryl ether | coffee, nutty, earthy | |
3-methylcrotonic acid | green, phenolic, dairy | |
Wine | acetic acid | acidic, vinegar, sour, sharp |
ethyl alcohol | alcoholic, ethereal, medicinal | |
2,6-dimethylpyrazine | chocolate, nutty, roasted | |
(*) The aroma description refers to Thegoodscentcompany.com. |
Compound | RT (min) | RI | Relative amount | |||
Natural | Honey | Full wash | Wine | |||
Aldehyde | ||||||
acetaldehyde | 1.59 | - | 0.23 ± 0.04 | 0.31 ± 0.00 | 0.29 ± 0.01 | 0.23 ± 0.03 |
2-methylbutanal | 2.57 | 912 | 0.49 ± 0.05 | 0.34 ± 0.01 | 0.39 ± 0.04 | 0.75 ± 0.10 |
3-methylbutanal | 2.60 | 914 | 0.39 ± 0.02 | 0.28 ± 0.01 | 0.34 ± 0.03 | 0.50 ± 0.07 |
1-methyl-1H-pyrrole-2-carboxaldehyde | 20.93 | 1619 | 0.69 ± 0.06 | 0.72 ± 0.02 | 0.95 ± 0.03 | 0.99 ± 0.13 |
2-phenyl-2-butenal | 27.88 | 1931 | 0.30 ± 0.01 | 0.26 ± 0.02 | 0.41 ± 0.00 | 0.37 ± 0.08 |
Total Aldehydes | 2.11 ± 0.06ab | 1.90 ± 0.05a | 2.38 ± 0.03ab | 2.83 ± 0.40b | ||
Furan | ||||||
furan | 1.85 | - | 0.10 ± 0.01 | 0.09 ± 0.01 | 0.12 ± 0.01 | 0.11 ± 0.01 |
2-methylfuran | 2.23 | - | 0.27 ± 0.01 | 0.22 ± 0.01 | 0.33 ± 0.01 | 0.22 ± 0.04 |
2.5-dimethylfuran | 2.99 | 946 | 0.12 ± 0.01 | 0.10 ± 0.00 | 0.15 ± 0.01 | 0.14 ± 0.02 |
2-pentylfuran | 10.41 | 1238 | 0.14 ± 0.01 | 0.15 ± 0.01 | 0.17 ± 0.00 | 0.15 ± 0.01 |
dihydro-2-methyl-3-furanone | 11.15 | 1262 | 0.51 ± 0.05 | 0.52 ± 0.01 | 0.51 ± 0.02 | 0.32 ± 0.02 |
2-methyl tetrahydrofuran | 13.93 | 1354 | 0.14 ± 0.04 | 0.15 ± 0.02 | 0.15 ± 0.00 | 0.17 ± 0.01 |
furfural | 16.92 | 1461 | 3.23 ± 0.21 | 2.53 ± 0.13 | 2.91 ± 0.24 | 2.41 ± 0.29 |
2-furfuryl methyl sulfide | 17.56 | 1485 | 0.17 ± 0.00 | 0.20 ± 0.01 | 0.24 ± 0.00 | 0.25 ± 0.02 |
2-acetylfuran | 18.02 | 1502 | 1.00 ± 0.01 | 1.08 ± 0.03 | 1.29 ± 0.01 | 1.02 ± 0.10 |
2-furfuryl-acetate | 18.92 | 1538 | 5.84 ± 0.15 | 6.53 ± 0.55 | 6.69 ± 0.10 | 8.76 ± 0.04 |
2-furanmethanamine | 19.54 | 1563 | 0.19 ± 0.02 | 0.19 ± 0.01 | 0.28 ± 0.01 | 0.30 ± 0.06 |
5-methylfurfural | 19.76 | 1571 | 4.65 ± 0.14 | 3.70 ± 0.08 | 4.43 ± 0.21 | 3.73 ± 0.47 |
2-ethyl-5-methylfuran | 19.84 | 1575 | 0.21 ± 0.00 | 0.23 ± 0.01 | 0.28 ± 0.01 | 0.16 ± 0.03 |
2.2-methylenebisfuran | 20.71 | 1610 | 0.74 ± 0.05 | 0.75 ± 0.03 | 0.93 ± 0.01 | 0.73 ± 0.08 |
2-acetyl-5-methylfuran | 20.80 | 1613 | 0.20 ± 0.01 | 0.21 ± 0.02 | 0.25 ± 0.01 | 0.19 ± 0.01 |
2-furanmethanol | 22.02 | 1666 | 12.70 ± 0.43 | 11.87 ± 0.54 | 15.15 ± 0.65 | 14.90 ± 1.93 |
2-furfuryl-5-methylfuran | 22.40 | 1681 | 0.38 ± 0.01 | 0.45 ± 0.02 | 0.68 ± 0.01 | 0.36 ± 0.05 |
2-hexyl furan | 23.12 | 1712 | 0.19 ± 0.02 | 0.12 ± 0.01 | 0.21 ± 0.01 | 0.23 ± 0.03 |
3.4-dimethylfuran-2.5-dione | 23.46 | 1727 | 0.39 ± 0.02 | 0.36 ± 0.02 | 0.47 ± 0.02 | 0.44 ± 0.03 |
3-phenyl furan | 26.24 | 1853 | 0.53 ± 0.00 | 0.44 ± 0.01 | 0.65 ± 0.03 | 0.72 ± 0.08 |
2-methyl-3-(2-furyl)propenal | 26.71 | 1875 | 0.18 ± 0.00 | 0.17 ± 0.01 | 0.25 ± 0.01 | 0.18 ± 0.05 |
5-methyl-2-furanmethanethiol | 26.93 | 1885 | 0.18 ± 0.01 | 0.17 ± 0.02 | 0.26 ± 0.01 | 0.29 ± 0.04 |
5-ethylfuran-2-carboxylic acid | 27.02 | 1890 | 0.08 ± 0.01 | 0.08 ± 0.01 | 0.10 ± 0.03 | 0.13 ± 0.04 |
difurfuryl disulfide | 27.25 | 1900 | 0.15 ± 0.01 | 0.17 ± 0.03 | 0.28 ± 0.02 | 0.20 ± 0.06 |
2-propyl furan | 27.29 | 1902 | 0.13 ± 0.02 | 0.14 ± 0.02 | 0.18 ± 0.02 | 0.12 ± 0.02 |
furfural acetone | 27.35 | 1905 | 0.15 ± 0.01 | 0.15 ± 0.00 | 0.23 ± 0.01 | 0.24 ± 0.06 |
furfuryl ether | 29.00 | 1985 | 0.47 ± 0.02 | 0.53 ± 0.04 | 0.79 ± 0.00 | 0.53 ± 0.07 |
furaneol | 30.06 | 2038 | 0.12 ± 0.00 | 0.10 ± 0.00 | 0.16 ± 0.0- | 0.11 ± 0.03 |
2-n-butyl furan | 34.03 | 2245 | 0.14 ± 0.02 | 0.16 ± 0.02 | 0.29 ± 0.02 | 0.23 ± 0.04 |
2-ethylfuran | 34.43 | 2267 | 0.10 ± 0.03 | 0.13 ± 0.02 | 0.16 ± 0.03 | 0.14 ± 0.03 |
Total Furans | 33.39 ± 0.08a | 31.71 ± 1.56a | 38.60 ± 0.16a | 37.64 ± 3.82a | ||
Ketone | ||||||
acetone | 1.94 | - | 0.43 ± 0.04 | 0.31 ± 0.01 | 0.44 ± 0.03 | 0.52 ± 0.06 |
2.3-butanedione | 3.28 | 970 | 0.16 ± 0.00 | 0.12 ± 0.01 | 0.16 ± 0.00 | 0.13 ± 0.01 |
2.3-pentanedione | 4.91 | 970 | 0.27 ± 0.02 | 0.20 ± 0.01 | 0.21 ± 0.01 | 0.18 ± 0.02 |
3-penten-2-one | 6.82 | 1123 | 0.05 ± 0.00 | 0.04 ± 0.00 | 0.05 ± 0.00 | 0.29 ± 0.04 |
hydroxyacetone | 12.22 | 1296 | 0.62 ± 0.02 | 0.51 ± 0.02 | 0.56 ± 0.01 | 0.52 ± 0.05 |
1-acetoxy-2-butanone | 18.82 | 1534 | 0.32 ± 0.01 | 0.28 ± 0.00 | 0.32 ± 0.01 | 0.27 ± 0.01 |
1-acetylcyclohexene | 19.32 | 1554 | 0.07 ± 0.01 | 0.06 ± 0.01 | 0.08 ± 0.00 | 0.15 ± 0.01 |
2-acetylpyridine | 20.49 | 1600 | 0.18 ± 0.00 | 0.18 ± 0.01 | 0.21 ± 0.02 | 0.20 ± 0.01 |
2-acetyl-4-methylpyridine | 21.15 | 1628 | 0.09 ± 0.01 | 0.12 ± 0.00 | 0.14 ± 0.01 | 0.11 ± 0.03 |
3-ethyl-2-hydroxy-2-cyclopentenone | 22.44 | 1682 | 0.82 ± 0.03 | 0.80 ± 0.00 | 0.99 ± 0.03 | 0.85 ± 0.09 |
1-(6-methyl-2-pyrazinyl)-1-ethanone | 22.66 | 1692 | 0.63 ± 0.03 | 0.56 ± 0.01 | 0.87 ± 0.04 | 0.87 ± 0.10 |
(+)-2-bornanone | 23.18 | 1714 | 0.14 ± 0.03 | 0.12 ± 0.01 | 0.19 ± 0.03 | 0.21 ± 0.03 |
Total Ketones | 3.78 ± 0.08ab | 3.30 ± 0.03a | 4.21 ± 0.12ab | 4.29 ± 0.45b | ||
Carboxylic acid | ||||||
acetic acid | 16.62 | 1450 | 3.59 ± 0.15 | 3.09 ± 0.03 | 4.21 ± 0.08 | 6.37 ± 0.34 |
4-hydroxybutyric acid | 21.05 | 1624 | 1.46 ± 0.15 | 1.31 ± 0.08 | 1.73 ± 0.14 | 2.63 ± 0.33 |
2-methyl-2-vinylmaleimide | 21.32 | 1635 | 0.20 ± 0.01 | 0.18 ± 0.00 | 0.25 ± 0.01 | 0.27 ± 0.02 |
isovaleric acid | 22.22 | 1673 | 1.26 ± 0.10 | 1.20 ± 0.06 | 1.70 ± 0.04 | 1.34 ± 0.15 |
3-methylcrotonic acid | 25.08 | 1799 | 0.53 ± 0.03 | 0.55 ± 0.03 | 0.75 ± 0.02 | 0.67 ± 0.08 |
2-furanpropionic acid | 25.24 | 1806 | 0.24 ± 0.01 | 0.25 ± 0.03 | 0.36 ± 0.00 | 0.62 ± 0.09 |
Total Carboxylic acid* | 7.27 ± 0.45a | 6.58 ± 0.23a | 9.00 ± 0.26b | 11.89 ± 0.32c | ||
Esther | ||||||
methyl acetate | 1.99 | - | 0.12 ± 0.00 | 0.11 ± 0.01 | 0.18 ± 0.01 | 0.22 ± 0.04 |
ethyl acetate | 2.34 | - | nd | nd | nd | 0.36 ± 0.05 |
ethyl formate | 2.50 | 906 | 0.12 ± 0.01 | 0.34 ± 0.00 | 0.17 ± 0.00 | 0.24 ± 0.01 |
furfuryl propionate | 20.45 | 1599 | 0.47 ± 0.02 | 0.49 ± 0.02 | 0.52 ± 0.05 | 0.38 ± 0.03 |
furfuryl isovalerate | 22.75 | 1695 | 0.33 ± 0.02 | 0.40 ± 0.02 | 0.57 ± 0.00 | 0.34 ± 0.03 |
methyl salicylate | 24.53 | 1774 | 0.47 ± 0.02 | 0.47 ± 0.03 | 0.59 ± 0.01 | 0.57 ± 0.05 |
methyl nicotinate | 24.62 | 1778 | 0.27 ± 0.02 | 0.26 ± 0.02 | 0.36 ± 0.01 | 0.28 ± 0.04 |
ethyl salicylate | 25.31 | 1810 | 0.17 ± 0.04 | nd | nd | 1.24 ± 0.15 |
methyl 3-methylfuroate | 26.52 | 1866 | 0.47 ± 0.04 | 0.45 ± 0.03 | 0.56 ± 0.02 | 0.43 ± 0.07 |
methyl palmitate | 33.50 | 2217 | 0.10 ± 0.00 | 0.10 ± 0.01 | 0.16 ± 0.00 | 0.09 ± 0.00 |
Total Esters* | 2.53 ± 0.06a | 2.62 ± 0.14a | 3.11 ± 0.08a | 4.15 ± 0.46b | ||
Alcohol | ||||||
1.3-propanediol | 2.43 | - | 5.21 ± 0.21 | 5.70 ± 0.04 | 6.84 ± 0.35 | 6.30 ± 0.26 |
ethyl alcohol | 2.80 | 931 | 0.20 ± 0.00 | 0.12 ± 0.00 | 0.16 ± 0.01 | 0.89 ± 0.06 |
1-ethynylcyclohexanol | 21.53 | 1644 | 0.23 ± 0.01 | 0.22 ± 0.02 | 0.29 ± 0.01 | 0.28 ± 0.01 |
benzyl alcohol | 26.82 | 1880 | 0.07 ± 0.01 | 0.06 ± 0.01 | 0.11 ± 0.00 | 0.14 ± 0.02 |
phenylethyl alcohol | 27.57 | 1916 | 0.25 ± 0.01 | 0.22 ± 0.02 | 0.28 ± 0.01 | 0.48 ± 0.02 |
2-thiophenemethanol | 28.22 | 1947 | 0.13 ± 0.00 | 0.11 ± 0.00 | 0.16 ± 0.00 | 0.16 ± 0.04 |
4-pyridinemethanol | 31.33 | 2103 | 0.28 ± 0.00 | 0.30 ± 0.01 | 0.45 ± 0.01 | 0.35 ± 0.05 |
Total Alcohols* | 6.38 ± 0.21a | 6.73 ± 0.03a | 8.29 ± 0.35b | 8.60 ± 0.45b | ||
Hydrocarbons | ||||||
toluene | 4.45 | 1033 | 0.13 ± 0.01 | 0.13 ± 0.00 | 0.18 ± 0.01 | 0.18 ± 0.02 |
dodecane | 9.21 | 1199 | 0.18 ± 0.02 | 0.18 ± 0.02 | 0.19 ± 0.03 | 0.16 ± 0.02 |
2.7-dimethylundecane | 15.25 | 1400 | 0.12 ± 0.02 | 0.09 ± 0.01 | 0.14 ± 0.01 | 0.16 ± 0.02 |
4-isopropyl-m-xylene | 22.35 | 1679 | 0.23 ± 0.03 | 0.23 ± 0.03 | 0.29 ± 0.00 | 0.58 ± 0.07 |
decahydronaphthalene | 22.59 | 1689 | 0.22 ± 0.01 | 0.19 ± 0.02 | 0.24 ± 0.02 | 0.24 ± 0.02 |
pulegone | 22.89 | 1701 | 0.22 ± 0.02 | 0.22 ± 0.02 | 0.28 ± 0.00 | 0.36 ± 0.04 |
β-damascenone | 25.60 | 1823 | 0.27 ± 0.01 | 0.26 ± 0.02 | 0.40 ± 0.01 | 0.38 ± 0.02 |
Total Hydrocarbons* | 1.37 ± 0.19a | 1.30 ± 0.11a | 1.72 ± 0.03ab | 2.06 ± 0.21b | ||
Pyrrole | ||||||
1-ethylpyrrole | 7.23 | 1137 | 0.12 ± 0.02 | 0.09 ± 0.00 | 0.13 ± 0.01 | 0.10 ± 0.00 |
1-butyl pyrrole | 14.75 | 1382 | 0.06 ± 0.00 | 0.05 ± 0.01 | 0.04 ± 0.01 | 0.20 ± 0.01 |
pyrrole | 18.35 | 1515 | 0.51 ± 0.00 | 0.44 ± 0.05 | nd | 0.53 ± 0.09 |
1-methyl-2-pyrroleacetonitrile | 21.59 | 1647 | 0.43 ± 0.03 | 0.39 ± 0.02 | 0.54 ± 0.05 | 0.59 ± 0.04 |
1-furfurylpyrrole | 25.73 | 1829 | 1.45 ± 0.05 | 1.25 ± 0.01 | 1.92 ± 0.06 | 1.78 ± 0.21 |
2-acetylpyrrole | 28.76 | 1974 | 0.83 ± 0.05 | 0.91 ± 0.04 | 1.35 ± 0.06 | 1.06 ± 0.12 |
2-formylpyrrole | 29.81 | 2026 | 0.51 ± 0.01 | 0.49 ± 0.00 | 0.76 ± 0.01 | 0.66 ± 0.07 |
Total Pyrroles | 3.92 ± 0.06ab | 3.62 ± 0.13a | 4.74 ± 0.05ab | 4.92 ± 0.54b | ||
Pyridine | ||||||
pyridine | 8.65 | 1182 | 0.67 ± 0.06 | 1.07 ± 0.03 | 1.24 ± 0.24 | 0.92 ± 0.11 |
2.6-dimethylpyridin-4-amine | 15.39 | 1405 | 1.53 ± 0.18 | 1.36 ± 0.01 | 1.66 ± 0.10 | 2.47 ± 0.29 |
3-methoxypyridine | 20.05 | 1583 | 0.16 ± 0.01 | 0.13 ± 0.01 | 0.17 ± 0.01 | 0.32 ± 0.02 |
3-methylpyridine 1-oxide | 22.97 | 1705 | 0.08 ± 0.02 | 0.11 ± 0.01 | 0.12 ± 0.01 | 0.14 ± 0.02 |
3-(3-thienyl)pyridine | 28.09 | 1941 | 0.14 ± 0.00 | 0.13 ± 0.01 | 0.22 ± 0.00 | 0.27 ± 0.04 |
2-hydroxy-3-methylpyridine | 34.99 | 2297 | nd | nd | nd | 0.11 ± 0.02 |
4-hydroxypyridine | 37.02 | 2413 | 0.18 ± 0.01 | 0.20 ± 0.01 | 0.34 ± 0.00 | 0.22 ± 0.03 |
Total Pyridines | 2.77 ± 0.26a | 3.00 ± 0.08a | 3.75 ± 0.37ab | 4.44 ± 0.49b | ||
Pyrazine | ||||||
pyrazine | 9.54 | 1210 | 0.38 ± 0.05 | 0.27 ± 0.01 | 0.38 ± 0.01 | 0.36 ± 0.03 |
2-methylpyrazine | 11.25 | 1265 | 3.49 ± 0.30 | 3.02 ± 0.02 | 3.88 ± 0.22 | 3.80 ± 0.47 |
2.5-dimethylpyrazine | 12.98 | 1322 | 1.75 ± 0.12 | 1.69 ± 0.03 | 2.08 ± 0.16 | 2.51 ± 0.27 |
2.6-dimethylpyrazine | 13.18 | 1329 | 1.87 ± 0.12 | 1.77 ± 0.02 | 2.32 ± 0.15 | 2.63 ± 0.33 |
2-ethylpyrazine | 13.33 | 1334 | 1.30 ± 0.07 | 1.17 ± 0.01 | 1.45 ± 0.02 | 1.58 ± 0.20 |
2.3-dimethyl pyrazine | 13.69 | 1346 | 0.48 ± 0.04 | 0.43 ± 0.02 | 0.53 ± 0.04 | 0.82 ± 0.09 |
2-ethyl-6-methylpyrazine | 14.85 | 1386 | 1.77 ± 0.16 | 1.62 ± 0.01 | 2.13 ± 0.04 | 2.39 ± 0.27 |
2-ethyl-5-methylpyrazine | 15.02 | 1392 | 1.30 ± 0.11 | 1.26 ± 0.01 | 1.47 ± 0.03 | 1.86 ± 0.21 |
2.6-diethylpyrazine | 16.22 | 1436 | 0.47 ± 0.03 | 0.44 ± 0.02 | 0.55 ± 0.00 | 0.69 ± 0.08 |
3-ethyl-2.5-dimethylpyrazine | 16.54 | 1447 | 1.89 ± 0.32 | 1.59 ± 0.07 | 1.83 ± 0.02 | 2.58 ± 0.30 |
2.3-diethyl pyrazine | 16.78 | 1456 | 0.20 ± 0.01 | 0.17 ± 0.01 | 0.21 ± 0.01 | 0.23 ± 0.01 |
2-ethyl-3.5-dimethylpyrazine | 17.03 | 1466 | 2.15 ± 0.10 | 1.80 ± 0.09 | 2.35 ± 0.09 | 2.94 ± 0.34 |
2-methyl-5-propylpyrazine | 17.37 | 1478 | 0.18 ± 0.02 | 0.16 ± 0.01 | 0.22 ± 0.01 | 0.32 ± 0.02 |
2-ethenyl-6-methylpyrazine | 17.65 | 1488 | 0.28 ± 0.02 | 0.24 ± 0.01 | 0.32 ± 0.01 | 0.46 ± 0.03 |
3.5-diethyl-2-methylpyrazine | 17.85 | 1496 | 1.37 ± 0.10 | 1.21 ± 0.08 | 1.45 ± 0.05 | 1.74 ± 0.21 |
2.3.5-trimethyl-6-ethyl pyrazine | 18.43 | 1519 | 0.47 ± 0.04 | 0.43 ± 0.03 | 0.53 ± 0.04 | 0.71 ± 0.09 |
2.5-dimethyl-3-(2-methylpropyl) pyrazine | 18.70 | 1530 | 0.46 ± 0.02 | 0.34 ± 0.02 | 0.43 ± 0.01 | 0.58 ± 0.03 |
1-propenylpyrazine | 19.21 | 1550 | 0.11 ± 0.01 | 0.09 ± 0.01 | 0.12 ± 0.01 | 0.25 ± 0.02 |
isopropenyl pyrazine | 20.39 | 1596 | 0.21 ± 0.00 | 0.21 ± 0.00 | 0.29 ± 0.04 | 0.24 ± 0.01 |
2-isoamyl-6-methylpyrazine | 21.24 | 1632 | 0.14 ± 0.02 | 0.08 ± 0.01 | 0.13 ± 0.00 | 0.59 ± 0.07 |
2-methyl-6-(1-propenyl)pyrazine | 21.89 | 1659 | 0.10 ± 0.01 | 0.09 ± 0.01 | 0.14 ± 0.00 | 0.19 ± 0.04 |
pyrazinamide | 23.24 | 1717 | 0.96 ± 0.13 | 0.74 ± 0.02 | 1.13 ± 0.23 | 0.88 ± 0.08 |
2-acetyl-3.5-dimethylpyrazine | 24.79 | 1786 | 0.18 ± 0.00 | 0.16 ± 0.02 | 0.24 ± 0.00 | 0.46 ± 0.07 |
Total Pyrazines | 21.49 ± 1.54ab | 19.00 ± 0.44a | 24.17 ± 1.00ab | 28.80 ± 3.29b | ||
Phenolic | ||||||
2.3-dimethylphenol | 11.96 | 1287 | 0.15 ± 0.03 | 0.12 ± 0.01 | 0.21 ± 0.01 | 0.31 ± 0.04 |
4-amino-3-methylphenol | 20.66 | 1608 | 0.22 ± 0.06 | 0.22 ± 0.00 | 0.28 ± 0.02 | 0.37 ± 0.05 |
2-methoxyhydroquinone | 23.72 | 1738 | 0.18 ± 0.00 | 0.18 ± 0.02 | 0.24 ± 0.01 | 0.24 ± 0.02 |
4-amino-2-hydroxytoluene | 25.00 | 1795 | 0.16 ± 0.01 | 0.15 ± 0.02 | 0.26 ± 0.01 | 0.24 ± 0.03 |
guaiacol | 26.41 | 1861 | 1.38 ± 0.08 | 1.17 ± 0.00 | 1.97 ± 0.04 | 1.88 ± 0.21 |
phenol | 29.44 | 2007 | 0.66 ± 0.06 | 0.69 ± 0.04 | 1.25 ± 0.07 | 0.83 ± 0.11 |
4-ethyl-2-methoxyphenol | 29.92 | 2031 | 1.49 ± 0.05 | 1.29 ± 0.10 | 2.17 ± 0.06 | 2.33 ± 0.28 |
p-cresol | 31.10 | 2091 | 0.18 ± 0.00 | 0.19 ± 0.02 | 0.30 ± 0.02 | 0.25 ± 0.03 |
2-methoxy-4-vinylphenol | 33.06 | 2193 | 5.60 ± 0.80 | 4.09 ± 0.14 | 6.94 ± 0.78 | 5.22 ± 0.66 |
Total Phenolics* | 10.01 ± 0.52ab | 8.10 ± 0.36a | 13.61 ± 0.73c | 11.68 ± 1.43bc | ||
Thiopen | ||||||
3-ethyl thiophene | 10.09 | 1228 | 0.05 ± 0.01 | 0.04 ± 0.00 | 0.05 ± 0.00 | 0.14 ± 0.02 |
2-methylthiolan-3-one | 18.62 | 1526 | 0.17 ± 0.00 | 0.16 ± 0.00 | 0.17 ± 0.00 | 0.15 ± 0.01 |
3-acetyl-2.5-dimethylthiophene | 25.41 | 1814 | 0.28 ± 0.01 | 0.25 ± 0.03 | 0.33 ± 0.00 | 0.49 ± 0.05 |
Total Thiopens* | 0.49 ± 0.00a | 0.44 ± 0.03a | 0.55 ± 0.00a | 0.77 ± 0.08b | ||
Miscellaneous | ||||||
sec-butylamine | 1.36 | - | 0.54 ± 0.02 | 0.44 ± 0.03 | 0.59 ± 0.01 | 0.46 ± 0.05 |
1-methylpiperidine | 4.29 | 1027 | 0.07 ± 0.00 | 0.09 ± 0.00 | 0.12 ± 0.01 | 0.15 ± 0.01 |
4-methylthiazole | 11.73 | 1280 | 0.11 ± 0.01 | 0.08 ± 0.00 | 0.09 ± 0.00 | 0.19 ± 0.00 |
butyl ethyl ether | 13.52 | 1340 | 0.12 ± 0.00 | 0.11 ± 0.00 | 0.12 ± 0.01 | 0.12 ± 0.00 |
diisopropyl ether | 14.02 | 1357 | 0.11 ± 0.00 | 0.11 ± 0.01 | 0.11 ± 0.00 | 0.23 ± 0.01 |
5-amino-2-methylbenzothiazole | 19.04 | 1543 | nd | nd | nd | 0.25 ± 0.02 |
benzylhydrazine | 21.39 | 1638 | 0.14 ± 0.01 | 0.11 ± 0.01 | 0.14 ± 0.01 | 0.22 ± 0.05 |
p-aminoanisole | 21.80 | 1655 | 0.56 ± 0.00 | 0.58 ± 0.03 | 0.78 ± 0.00 | 0.71 ± 0.08 |
4-methoxyphenyl isothiocyanate | 23.54 | 1730 | 0.22 ± 0.02 | 0.20 ± 0.01 | 0.31 ± 0.01 | 0.38 ± 0.06 |
4-methoxythiophenol | 23.66 | 1736 | 0.84 ± 0.03 | 0.76 ± 0.02 | 0.89 ± 0.01 | 0.68 ± 0.09 |
2-methyl-5-methoxy-4h-pyran-4-one | 25.90 | 1837 | 0.25 ± 0.00 | nd | nd | 0.33 ± 0.05 |
6-butyltetralin | 32.02 | 2139 | 0.14 ± 0.00 | 0.12 ± 0.01 | 0.22 ± 0.00 | 0.18 ± 0.03 |
caprolactam | 32.98 | 2189 | 0.09 ± 0.00 | 0.09 ± 0.01 | 0.16 ± 0.03 | 0.08 ± 0.00 |
indole | 37.41 | 2433 | 0.15 ± 0.00 | 0.12 ± 0.02 | 0.21 ± 0.00 | 0.15 ± 0.01 |
Total Miscellaneous | 3.34 ± 0.10ab | 2.82 ± 0.15a | 3.74 ± 0.04ab | 4.14 ± 0.46b | ||
Total Volatile Compounds* | 99.91 ± 0.95ab | 92.10 ± 4.31a | 119.08 ± 0.38bc | 127.69 ± 11.08c | ||
Data were the averages ± standard deviation of two replicates. Numbers followed by different superscript letters in the same row indicate significant differences (p < 0.05) based on one-way ANOVA and Duncan test. Groups with (*) were affected by processing. RT (retention time). RI (retention index). Compounds grouping refers to pubchem.ncbi.nlm.nih.gov. |
Potential markers | Percent contribution (%) | VIP | |||
Natural | Honey | Full wash | Wine | ||
ethyl acetate | nd | nd | nd | 0, 26 | 0, 98 |
5-amino-2-methylbenzothiazole | nd | nd | nd | 0, 18 | 0, 98 |
ethyl salicylate | 0, 15 | nd | nd | 0, 89 | 0, 97 |
2-methyl-5-methoxy-4H-pyran-4-one | 0, 23 | nd | nd | 0, 24 | 1, 16 |
Data were the averages of two replicates. nd (not detected). VIP (variable importance projection). |
Processing | Compounds | Aroma description* |
Natural and Honey | 2-ethyl pyrazine (Natural) | nutty, musty, fermented, coffee, roasted, cocoa, meaty |
hydroxyacetone (Natural) | sweet caramellic, ethereal | |
furfural | sweet, brown woody, bready, caramellic, phenolic | |
5-methylfurfural | sweet, caramellic, bready, brown, coffee-like | |
dihydro-2-methyl-3-furanone | sweet, caramel, bread | |
2-furfuryl-acetate (Honey) | fruity, roast, sweet | |
2-methyl pyrazine (Honey) | nutty, cocoa roasted, chocolate, peanut, green | |
2-furanmethanol (Honey) | alcoholic, chemical, musty, sweet caramel, bread, coffee | |
3-Ethyl-2-hydroxy-2-cyclopentenone (Honey) | maple, caramel, coffee, smoke | |
Full wash | 2-formylpyrrole | musty, beefy, coffee |
phenol | phenolic, plastic, rubbery | |
2-acetylpyrrole | musty, nutty, coumarinic | |
1-furfurylpyrrole | vegetative, cereal, bready, radish, mushroom, potato nuances | |
difurfuryl ether | coffee, nutty, earthy | |
3-methylcrotonic acid | green, phenolic, dairy | |
Wine | acetic acid | acidic, vinegar, sour, sharp |
ethyl alcohol | alcoholic, ethereal, medicinal | |
2,6-dimethylpyrazine | chocolate, nutty, roasted | |
(*) The aroma description refers to Thegoodscentcompany.com. |