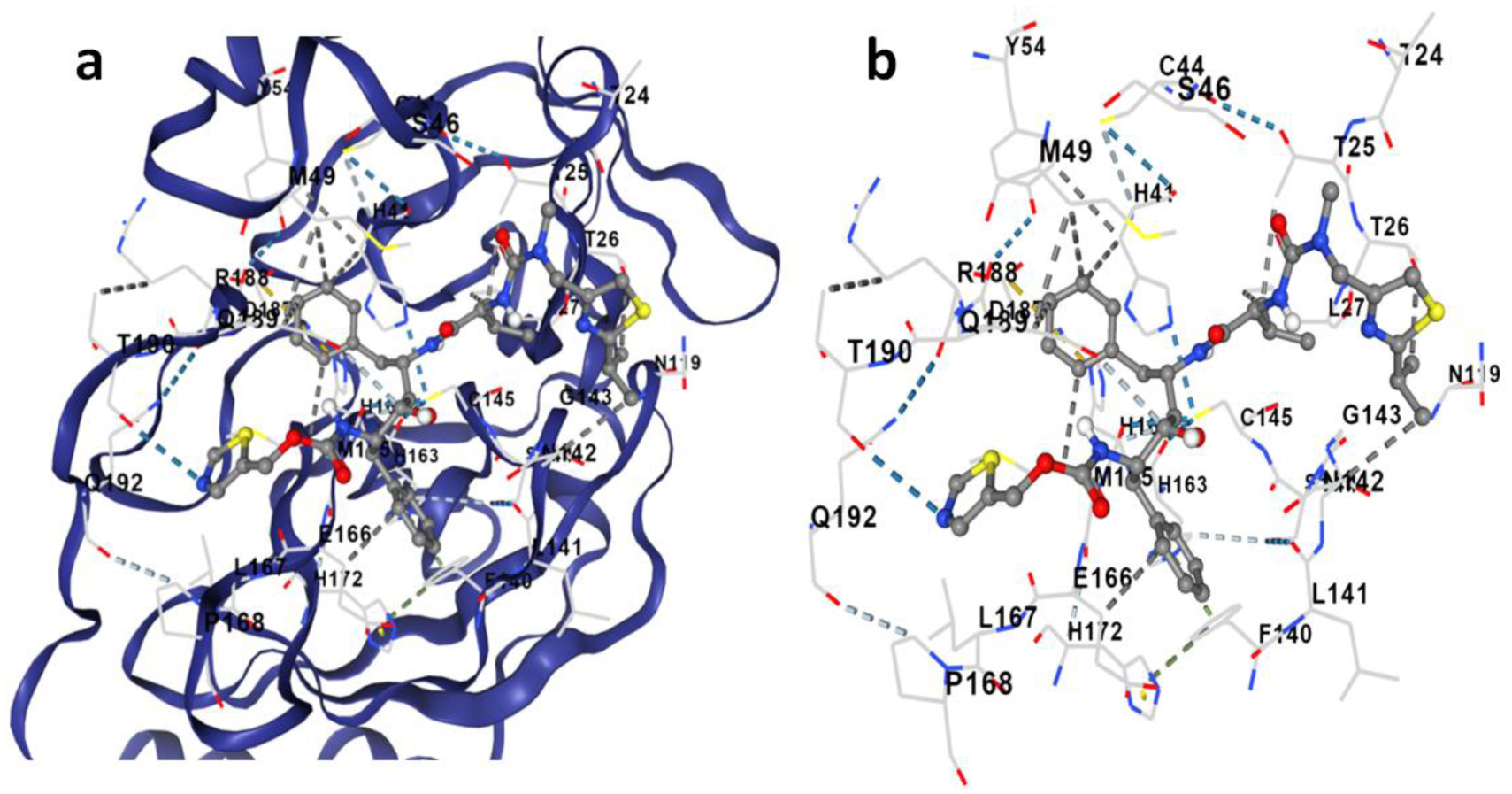
The recruitment of therapeutics and most importantly COVID-19 vaccines has seen a measurable reduction in transmission, re-infection, severity, hospitalization and mortality associated with the novel severe acute respiratory syndrome coronavirus 2 (SARS-CoV-2). The development and approval of some vaccines and therapeutics undoubtedly signaled renewed hope for public health personnel, the government, the World Health Organization (WHO) and the entire world population. At present, most countries have progressed beyond administering first and second doses to administering COVID-19 vaccine updated boosters to prevent transmission and provide protection. Notably, a bivalent COVID-19 vaccine from Pfizer–BioNTech and Moderna, also called an “updated” COVID-19 vaccine booster dose, is a formulation that houses the original virus strain and omicron BA.1, which provides broad immunity against COVID-19 including the omicron variant (BA.1) and the Paxlovid drug (Nirmatrelvir-ritonavir) authorized for use by the Food and Drug Administration (FDA) and the European Medicines Agency. This current review outlines the variant of concern (VOC), viral cell entry and pathogenesis, host immunity and viral immune evasion. In addition, we discuss the therapeutic and vaccine treatment approach, WHO and FDA authorization, vaccine storage and vaccine efficacy. In conclusion, bearing in mind the trend of continued mutations as observed on the spike (S) glycoprotein and receptor binding domain (RBD) of SARS-CoV-2, which lead to more immune-evasive strains such as BQ.1, BQ.1.1, BF.7, XBB and XBB.1, researcher and clinician attention should be tailored toward the design and development of variant-specific vaccines for future interventions.
Citation: Daniel Danladi Gaiya, Jonathan Danladi Gaiya, Richard Auta, Aliyu Muhammad, Bege Jonathan, Stella Kuyet Udu, Ekpa Emmanuel, Amina Shehu Bature. The journey so far with SARS-CoV-2 variants: Pathogenesis, immunity and treatments[J]. AIMS Allergy and Immunology, 2023, 7(4): 222-250. doi: 10.3934/Allergy.2023016
[1] | Lia Tsverava, Nazibrola Chitadze, Gvantsa Chanturia, Merab Kekelidze, David Dzneladze, Paata Imnadze, Amiran Gamkrelidze, Vincenzo Lagani, Zaza Khuchua, Revaz Solomonia . Antibody profiling reveals gender differences in response to SARS-COVID-2 infection. AIMS Allergy and Immunology, 2022, 6(1): 6-13. doi: 10.3934/Allergy.2022002 |
[2] | Yu. A. Desheva, A. S. Mamontov, P. G. Nazarov . Contribution of antibody-dependent enhancement to the pathogenesis of coronavirus infections. AIMS Allergy and Immunology, 2020, 4(3): 50-59. doi: 10.3934/Allergy.2020005 |
[3] | Darrell O. Ricke . Antibodies and infected monocytes and macrophages in COVID-19 patients. AIMS Allergy and Immunology, 2022, 6(2): 64-70. doi: 10.3934/Allergy.2022007 |
[4] | DharmaSaranya Gurusamy, Vasuki Selvamurugesan, Swaminathan Kalyanasundaram, Shantaraman Kalyanaraman . Is COVID 19, a beginning of new entity called chronic viral systemic inflammatory syndrome?. AIMS Allergy and Immunology, 2021, 5(2): 127-134. doi: 10.3934/Allergy.2021010 |
[5] | Kamyar Nasiri, Zohreh Mortezania, Sanaz Oftadehbalani, Mohammad Khosousi Sani, Amin Daemi, Seyyedeh Touran Hosseini, Yusuf Döğüş, Zafer Yönden . Parasitology and Covid-19: epidemiological, immunological, pathological, and therapeutic aspects. AIMS Allergy and Immunology, 2023, 7(1): 92-103. doi: 10.3934/Allergy.2023007 |
[6] | Mansur Aliyu, Ali Akbar Saboor-Yaraghi, Sadegh Khodavaisy, Behrouz Robat-Jazi, Muhammad Ibrahim Getso . Glucuronoxylomannan: the salient polysaccharide in cryptococcal immunity. AIMS Allergy and Immunology, 2022, 6(2): 71-89. doi: 10.3934/Allergy.2022008 |
[7] | Mansur Aliyu, Sayed-Hamidreza Mozhgani, Omid Kohandel Gargari, Mustapha Ahmed Yusuf, Ali Akbar Saboor-Yaraghi . The host immune responses to SARS-CoV-2 and therapeutic strategies in the treatment of COVID-19 cytokine storm. AIMS Allergy and Immunology, 2021, 5(4): 240-258. doi: 10.3934/Allergy.2021018 |
[8] | Darrell O. Ricke . Etiology model for many vaccination adverse reactions, including SARS-CoV-2 spike vaccines. AIMS Allergy and Immunology, 2022, 6(4): 200-215. doi: 10.3934/Allergy.2022015 |
[9] | Zinia Pervin, Anika Tasnim, Hasib Ahamed, Md Al Hasibuzzaman . Epigenetic regulation of the COVID-19 pathogenesis: its impact on the host immune response and disease progression. AIMS Allergy and Immunology, 2023, 7(1): 60-81. doi: 10.3934/Allergy.2023005 |
[10] | Y. Desheva, T. Shvedova, A. Lerner, P. Kudar, O. Kopteva, D. Petrachkova, I. Koroleva, G. Leontieva, S. Ponkratov, A. Suvorov . Exploring immune response and lab markers across COVID-19 severity levels. AIMS Allergy and Immunology, 2024, 8(4): 216-231. doi: 10.3934/Allergy.2024013 |
The recruitment of therapeutics and most importantly COVID-19 vaccines has seen a measurable reduction in transmission, re-infection, severity, hospitalization and mortality associated with the novel severe acute respiratory syndrome coronavirus 2 (SARS-CoV-2). The development and approval of some vaccines and therapeutics undoubtedly signaled renewed hope for public health personnel, the government, the World Health Organization (WHO) and the entire world population. At present, most countries have progressed beyond administering first and second doses to administering COVID-19 vaccine updated boosters to prevent transmission and provide protection. Notably, a bivalent COVID-19 vaccine from Pfizer–BioNTech and Moderna, also called an “updated” COVID-19 vaccine booster dose, is a formulation that houses the original virus strain and omicron BA.1, which provides broad immunity against COVID-19 including the omicron variant (BA.1) and the Paxlovid drug (Nirmatrelvir-ritonavir) authorized for use by the Food and Drug Administration (FDA) and the European Medicines Agency. This current review outlines the variant of concern (VOC), viral cell entry and pathogenesis, host immunity and viral immune evasion. In addition, we discuss the therapeutic and vaccine treatment approach, WHO and FDA authorization, vaccine storage and vaccine efficacy. In conclusion, bearing in mind the trend of continued mutations as observed on the spike (S) glycoprotein and receptor binding domain (RBD) of SARS-CoV-2, which lead to more immune-evasive strains such as BQ.1, BQ.1.1, BF.7, XBB and XBB.1, researcher and clinician attention should be tailored toward the design and development of variant-specific vaccines for future interventions.
Genetic mutations among viruses, especially SARS-CoV-2, occur frequently due to high levels of viral replication mechanism, re-infection and transmissibility capacity [1]. Severe acute respiratory syndrome coronavirus 2 (SARS-CoV-2) is a virulent strain member of the Coronaviridae family that has had a significant impact on human health, the world economy, food and animal productivity [2]. SARS-CoV-2 was first reported to cause severe pneumonia in December 2019, in a seafood wholesale market in Wuhan, Hubei province, China. As of 28 February 2020, over 80000 confirmed cases had been reported worldwide, which made the World Health Organization on 30 January 2020 declare that the SARS-CoV-2 outbreak constituted a Public Health Emergency of International Concern [1]. The ongoing coronavirus disease 2019 (COVID-19) pandemic has resulted in over 5.2 million deaths globally; however, concerted efforts in understanding the mechanism and mode of transmission, incubation and genetic mutations of the virus paved the way for the development of effective vaccines [2]. SARS-CoV-2 is capable of causing mild to severe respiratory symptoms which highlight the need for continued testing, active monitoring, surveillance, control and most importantly vaccination.
Severe acute respiratory syndrome coronavirus 2 (SARS-CoV-2) viruses have continuously evolved over the years now, with many variants simultaneously emerging across the world [2]. Presently, the growing public burden caused by the continued infection and re-infection of people whose immune systems had produced antibodies as a result of previous infection has made researchers judge SARS-CoV-2 variants as variants of concern (VOC) and variants under monitoring (VUM) to ensure effective global surveillance and efficient investigations of the ongoing global response to the COVID-19 pandemic [3],[4]. Several SARS-CoV-2 variants have emerged across different countries with Alpha, Beta, Delta, Gamma and Omicron being the most recent variants of concern as approved by WHO Virus Evolution Working Group [4]. This classification was done based on genetic changes known to affect the virus's features, such as transmissibility, prevalence and virulence, which led many countries to impose travel restrictions and border control measures. These variants of concern, also known as variants of interest, have been reported to have the ability to re-infect previously infected and recovered persons because they can evade immune responses, thus making them less susceptible to monoclonal antibodies, which impact the effectiveness of mRNA vaccines [4],[5]. According to reports, the spike (S) protein of SARS-CoV-2 has the ability to evade neutralizing antibodies, and D614G mutations within the receptor binding domain (RBD) of the spike (S) protein result in increased viral load and promote adhesion of the virus spike protein to the angiotensin converting enzyme 2 (ACE2) receptor [6]. Therefore, the biochemical mechanism of spike (S) glycoprotein and ACE2 as an entry receptor for SARS-CoV-2, in no doubt, consequently increases infectivity [7]. Today, these variants of concern have necessitated the need for effective and continued vaccination, sandwiched with non-pharmaceutical protocols and other public health prevention measures [3].
The scourge of SARS-CoV-2 has left an indelible mark that has revolutionized the world's perspective in responding to pandemics and emerging infectious diseases [8]. The pharmaceutical industries were not left behind, because their swift and proactive approach to producing prophylaxis and therapeutics and re-profiling some novel drugs to mitigate the spread of COVID-19 disease was top notch [9]. At the moment, anti-SARS-CoV-2 vaccines are the game changers, due to their ability to reduce morbidity and mortality [10],[11]. Vaccination targeting the S glycoprotein (including the E, M and N proteins) is one of the most effective tools to halt the spread of COVID-19 disease [10]. Vaccines have been proven to stimulate a strong humoral as well as cellular response, which is determined by the strength of the immune responses generated after vaccination [11]. These responses are aimed at stimulating the production of SARS-CoV-2 neutralizing antibodies as well as memory cells [12]. Cascades of immunomechanistic processes involving activated T cells and stimulated B cells differentiate into plasma cells to produce neutralizing antibodies [12],[13]. Some of the B cells become quiescent and later on differentiate into memory B cells, which elicit immunological memory against SARS-CoV-2 [13],[14]. It was also reported that primed CD4+ memory cells and CD8+ T cells are capable of proliferating in the human system to modulate the elimination of infected cells and clearance of SARS-CoV-2 [15]. The present study reviewed the SARS-CoV-2 variants of concern, viral entry and host immunity, viral immune evasion and treatment approach with the intention of providing current knowledge on SARS-CoV-2 variants of concern, vaccine efficacy on these variants and updated booster shots.
As it stands today, SARS-CoV-2 is one of the most lethal infectious diseases of public health concern because of its high transmissibility, mortality scale and genetic mutations [16]. The current pipeline of research on SARS-CoV-2 has attracted huge financial commitment in understanding the SARS-CoV-2 pathogenesis, virus etiology, genetic mutations, chemotherapy and immunogenicity, which has significantly accelerated clinical treatments [17]. However, there are still knowledge gaps that need to be unraveled for effective prophylaxis design and development [4]. Evolution is a gradual process that leads to the emergence of young, complex organisms from simpler, older organisms (WHO, 2021). SARS-CoV-2 has been reported to evolve strains characterized by genetic mutations in the spike (S) protein, N-terminal domain (NTD) and receptor binding domain (RBD), which enhance its entry into the target host cell receptors and could also compromise the neutralizing antibodies [18],[19]. Genetic mutations are rapid in RNA viruses due to the RNA-dependent RNA polymerase and cofactors that possess proofreading activity [19]. The World Health Organization (WHO) SARS-CoV-2 Evolution Working Group was established to monitor SARS-CoV-2 variants, virulence, genetic changes and their impact on COVID-19 countermeasures and develop and implement a global risk monitoring framework for SARS-CoV-2 variants [18],[20]. SARS-CoV-2 has continuously undergone genetic mutations leading to the emergence of different viral strains of public health concern categorized as variants of concern as approved by the WHO Technical Advisory Group on Virus Evolution (TAG-VE) [21]. Five VOCs had been classified and designated on March 26, 2022, to be currently in circulation to reflect evolutionary changes in their epidemiology over time [21].
The SARS-CoV-2 lineage B.1.17 was the first most dominant circulating variant reported in the United Kingdom (UK) on 14 December 2020 [4],[16]. This alpha variant is characterized by high transmissibility of about 40–50%, 43–90% fecundity, hospitalization, disease severity and deaths compared with the original virus [4]. The virulence attributed to the SARS-CoV-2 lineage B.1.17 resulted from the genetic mutations observed in the spike (S) protein, with about eight mutations: Δ69–70 deletion, Δ144 deletion, N501Y, A570D, P681H, T716I, S982A and D1118H [22]. The N501Y within the receptor binding domain (RBD) of the spike (S) protein plays a vital role in the binding affinity to the host angiotensin converting enzyme 2 (ACE2) receptor, leading to a high viral load [23]. Furthermore, immune escape in immunocompromised patients was linked to Δ69–70 and Δ144 deletions in the spike protein [24],[25]. Based on clinical reports, the Pfizer (mRNA vaccine), Moderna (mRNA vaccine), AstraZeneca, Novavax (adjuvant recombinant protein vaccine) and Janssen (adenoviral vector vaccine) vaccines were effective in preventing the severity of the disease and hospitalizations when working against the alpha variant [26],[27]. Pfizer and AstraZeneca vaccines were evaluated and proven to elicit effective immune responses of about 90% after first and second doses among individuals whose sera displayed excellent reduced neutralizing effects against the alpha variant [28],[29]. Additionally, Novavax was reported to have 89.3% efficacy against the alpha variant and reduce viral transmission [30],[31]. Moderna and Janssen vaccines were also reported to significantly neutralize alpha variants, with the Moderna vaccine being approved by the FDA for children aged six months to five years with certain immunocompromising conditions [32].
The beta variant was first identified in the UK in May 2020, probably connected to travelers from South Africa [23]. According to Kim et al. [16], the beta variant's genetic changes resulted in increased transmissibility and hospitalizations compared to the alpha variant. It was found to have genetic mutations (L18F, D80A, D215G, R246I, K417N, E484K, N501Y, D614G and A701V) in the spike (S) protein, with the K417N, E484K and N501Y mutations located on the receptor binding domain (RBD) [33],[34]. These alterations enhance spike (S) protein infusion to the ACE2 receptor of the host cells, thus leading to an increased transmission rate [35],[36]. Studies have shown that the beta variant is highly resistant to neutralizing antibodies from vaccinated individuals because of the E484K mutation [16],[23]. However, the disease severity of the beta variant in immunocompromised patients is moderate compared with the delta and omicron variants [37],[38]. Multiple studies have shown that Pfizer and Moderna vaccines with efficacy of 96.4% significantly neutralize beta variants after first and second doses; likewise, Novavax was 60% effective against the beta variant [4],[31],[39],[40]. Also, a significant reduction of the beta variant was observed when bamlanivimab and etesevimab monoclonal antibody treatments were co-administered [41],[42].
The P.1 lineage (gamma variant) was the major cause of many hospitalizations and deaths in Manaus, Brazil. It was first identified by the Institute of Infectious Diseases, Japan, in Amazonas state returnees from Brazil in January 2021 [23],[43]. The molecular characterization of the genome sequence of lineage P.1 found that the gamma variant contains about 21 genetic mutations with 10 alterations (L18F, T20N, P26S, D138Y, R190S, H655Y, T1027I, K417T, E484K, and N501Y) in the spike (S) protein [4],[44]–[46]. Additionally, three genetic mutations identified on the receptor binding domain mirror those of the alpha and beta variants, which are capable of accelerating the binding affinity of the spike (S) protein to the ACE2 host receptor cells and are associated with increased virus transmissibility, immune escape potential, re-infections and viral fecundity [4],[45],[47]. Gamma variant re-infection cases have not been well established at a population level across the globe but have been widely reported with clinical proof [48]. Based on the reports of Collier et al. [49], the gamma variant has been shown to be relatively resistant to neutralizing antibodies from vaccine sera after vaccination with Moderna and Pfizer vaccines. However, according to a study in France, the first and second doses of vaccination with Moderna and Pfizer vaccines were reported to have 77–88% efficacy against the previous variants of concern [50],[51]. The gamma variants have been implicated as being susceptible to the therapeutic effects of casirivimab and imdevimab [50].
According to the Ministry of Health and Family Welfare's report on March 24, 2021, the delta version of lineage B.1.617 SARS-CoV-2, which caused the second wave of the COVID-19 pandemic in India, has three sub-lineages (B.1.617.1, B.1.617.2 and B.1.617.3). Due to the sub-lineage B.1.617.2's high transmissibility, quick incubation period, severe illness and probable immunological escape, WHO named it a variant of concern (VOC) [52]. According to studies, the delta variant is 50% more contagious than the alpha variant due to genetic mutations in the spike (S) protein (19R, G142D, 156del, 157del, R158G, L452R, T478K, D614G, P681R and D950N), with P681R being linked to promoting SARS-CoV-2 infusion into host receptor cells and increased morbidity [23]. According to studies, L452R and T478K mutations can increase the spike (S) protein's affinity for the ACE2 receptor on host cells, increasing viral load and transmissibility. These mutations have also been linked to immune escape from vaccine recipients [53]–[56]. Furthermore, compared to the alpha, beta and gamma versions, the WHO assessment revealed that the delta variation was the most widespread and virulent SARS-CoV-2, suggesting that it was the most dominant form to be concerned about [52].
Additionally, studies from Ontario, Canada, found that delta variant virulence, disease severity and transmissibility rate were remarkably higher compared with other variants, accompanied by hospitalizations, intensive care unit admissions and deaths [57],[58]. The evolution of the original lineage of the delta variant due to mutations in the spike (S) protein was found to give rise to lineages B.1.617.2.1 (delta AY.1 and AY.4.1) with mutations (V70F, A222V, W258L and K417) in the spike (S) protein [59],[60]. The K417 mutation mirrors that which is present in the beta variants identified to contribute to immune evasion and reduction of the spike protein interaction with neutralizing antibodies from host cells [61]. Treatment with monoclonal antibodies, convalescent plasma and vaccines has shown a positive neutralization effect [62]. The Pfizer and AstraZeneca vaccines have shown 72–88% efficacy against the delta and alpha variants from studies carried out in the UK and Scotland after two doses [63]. A study showed that Pfizer and AstraZeneca vaccines are effective in reducing the transmissibility of COVID-19 and hospitalization cases in individuals with confirmed delta variant infection [64].
The omicron variant with sub-lineages BA.1, BA.2, BA.3, BA.4 and BA.5 was first detected by WHO on 11 November 2021 in Botswana, on 14 November 2021 in South Africa and on 1 December 2021 in California, United States [23],[65]. The omicron variant (BA.1.1.529) was designated as a variant of concern due to a potential increase in transmissibility, resistance to therapeutics and immune escape [52]. Additionally, instances of the omicron variants have been reported in England, Switzerland, Portugal, New Zealand, Nigeria, Mauritius and the Democratic Republic of the Congo [66]. The sub-variant BA.2, with 59 genetic mutations, of which 29 are in the spike (S) protein, has a higher replication potential of about 1.4 fold that of BA.1. However, the clinical symptoms of BA.2 infection, such as sore throat and pharyngitis, are similar to those of BA.1 [67],[68]. The evolution of the omicron sub-variant BA.2 generated the 12th lineage BA.2.12.1, which accounts for about 26% of the SARS-CoV-2 genomes, with additional spike mutations S704L and L452Q critically responsible for virus infusion and binding of the spike protein to the ACE2 receptor of the host cell [62]. It is the most dominant strain in the United States, whereas BA.4 and BA.5 are dominant variants in South Africa and Europe [69].
Furthermore, BA.2.12.1 has the ability to infect individuals already recovered from or immune to BA.1 [67],[70]. The risk of transmission, mutation, immune evasion and proliferation associated with the omicron variants remains exceptionally high [67]. This fact makes the omicron variant the most mutated, contagious even among vaccinated people and easily spread variant of concern, with recent evidence presenting the omicron variants with a higher transmissibility potential than other variants [66]. The omicron variant was responsible for the fourth wave of SARS-CoV-2 infections that ravaged South Africa, leading to increased hospitalizations, disease severity, straining of health facilities, community and global spread and deaths [65]. As of January 10, 2022, the US had reported about 1.35 million cases of SARS-CoV-2 infections, and in May 2022, the figures increased beyond the normal as a result of increased home testing [71],[72]. According to research conducted in Denmark, the transmission potential of the omicron variant among people, especially household members, was 2.6–3.7 fold greater than the delta variant among vaccinated and boosted individuals, while among unvaccinated individuals, the omicron variant was only 1.17 fold more infectious than the delta variant [73]. The sequence genome of the omicron variant found more than 30 amino acid mutations and deletions (K417N, N440K, G446S, S477N, T478K, E484A, Q493K, G496S, Q498R, N501Y, Y505H and P681H) on the spike (S) protein capable of binding to ACE2 of the host cell, thus leading to high transmission of SARS-CoV-2 and immune evasion [66]. The mutation N501Y has been implicated in playing a very pivotal role in virus binding to ACE2 host receptor cells, which mirrors that of the alpha variant [74].
Based on the vaccination reports, three doses of Pfizer and Moderna vaccine have been proven to neutralize the omicron variants; however, reported cases of omicron variant transmission among some fully vaccinated individuals were observed [75]. This finding is in accord with the report of Hoffmann et al. [76], which showed the omicron variant capable of resisting vaccine elicited neutralizing antibodies and sera of convalescent patients. The mutations present in the omicron variants were linked to increased transmissibility and virus infusion into host cells [54]. Given the surge in infection and reinfection with omicron and its subvariants, booster doses were recommended for vaccinated and immunocompromised individuals [67].
Severe acute respiratory syndrome coronavirus 2 (SARS-CoV-2) is the cause of coronavirus disease 2019 (COVID-19). It is a single-stranded RNA-positive (+ssRNA) sarbecovirus subgenus beta-coronavirus with structures and genetic composition closely related to severe acute respiratory syndrome coronavirus (SARS-CoV) and Middle East respiratory syndrome coronavirus (MERS-CoV) [55]. The SARS-CoV-2 protein structure is made up of the spike (S) protein, nucleocapsid (N) protein containing RNA as the genetic material, envelope (E) glycoprotein, membrane (M) protein and multiple open reading frames (ORF3a, ORF3b, ORF6, ORF7a, ORF7b, ORF8, ORF9, and ORF10) [77]. The virus gains entry into the host cell with the help of the spike (S) glycoprotein located on the outer surface of the virion, which mediates the infection process, leading to the cleavage of the virus spike (S) protein by human transmembrane proteases 2 (TMPRSS2) into an amino (N) terminal S1 subunit and a carboxyl (C) terminal S2 subunit containing a fusion peptide to enhance the infusion of the virus into the host cell [55]. The amino (N) terminal S1 subunit is composed of a receptor binding domain (RBD) and an N-terminal domain (NTD), which facilitate spike (S) glycoprotein binding to human angiotensin converting enzyme 2 (ACE2) receptors (virus-cell membrane fusion), thus enhancing viral entry into the host cell [78],[79]. Scientific findings have shown that the spike glycoprotein has the ability to preferentially influence the interaction, recognition, receptor binding and virus fusion with the ACE2 receptor of host cells [79]. The respiratory and circulatory systems are preferentially targeted by SARS-CoV-2 upon entry into the host cell, leading to pneumonia with the lungs and other tissues as the primary targets [55],[80]. Other organs such as myocardial cells, the upper esophagus, enterocytes from the ileum, proximal tubular cells of the kidney and urothelial cells of the bladder also express ACE2 receptors in addition to the respiratory epithelial cells of the human lungs [55],[81]. Human transmembrane proteases 2 begin to cleave the spike (S) protein as a result of the spike (S) glycoprotein's receptor binding domain interacting with ACE2 receptors on type 2 alveolar epithelial cells in the human lungs. This enhances viral cell membrane fusion and replication, which is crucial for viral entry into the host cell and results in severe damage to the gastrointestinal tract (GI) and hepatobiliary, cardiovascular, renal and central nervous systems [82]. Furthermore, research has demonstrated that the highly immunogenic phosphoproteins known as nucleocapsid (N) proteins interact with viral genomic RNA to release a spiral nucleocapsid that promotes viral RNA replication and cell signaling, thereby making it easier for the virus to infect host cells [79],[83],[84]. Additionally, it has been suggested that the membrane protein (M) and envelope (E) glycoprotein work together to block the immunological responses and activation brought on by gamma interferon (IFN) [79],[85].
The human immune system is endowed with multiple signaling cascades of biochemical mechanisms designed to confer immunity and defend the human system against pathogens. These cascades of immunophysiological processes ultimately lead to the activation of antiviral innate immunity (with interferons, cytokines, chemokines, Toll-like receptors, natural killer cells, macrophages, monocytes, granulocytes, mast cells and dendritic cells) and adaptive immunity (with T and B lymphocytes) [86],[87]. The innate immunity is the first defense against invading SARS-CoV-2, resulting in a nonspecific response leading to the production of antiviral and proinflammatory molecules aimed at interrupting critical steps of the viral cycle, thus clearing infection and also preventing reinfection by SARS-CoV-2 [86],[88]. During viral infection, the SARS-CoV-2 spike (S) receptor binding domain interacts with the host ACE2 to generate new viral copies, which are then released into the host [86]. This viral invasion prompts a rapid immune response (immune defense-based protective phase) through the recruitment of interferons, cytokines, chemokines, Toll-like receptors, natural killer cells, macrophages, monocytes, granulocytes, mast cells and a host of other immune mediators to detect and restrict different stages of the viral life cycle in order to prevent the spread of SARS-CoV-2 [89],[90]. Previous studies have shown that activation of TLR2 triggers an innate immune response to viral clearance in order to prevent tissue damage and also suppress excessive inflammation, thus maintaining the integrity of local epithelial cells [90]. The innate immune system uses immune cells such as Toll-like receptors 3, 7 and 8, retinoic acid inducible gene I (RIG-I) and melanoma differentiation-associated protein 5 (MDA5) to recognize pathogen-associated molecular patterns (PAMPs) or host molecules associated with disturbances of homeostasis (danger-associated molecular patterns (DAMPs)) through the specific pattern recognition receptors (PRRs), which then activate the antiviral signaling cascades to release interferons (IFN), chemokines and proinflammatory cytokines [91],[92]. These soluble factors are locally mobilized to the infected tissues and organs for a rapid cellular response, which is dependent on the concentration and time of secretion of these soluble mediators [93],[94].
A cytokine storm (cytokine-releasing syndrome) is caused by dysregulated production or overactivation by inducing a massive release of cytokines and chemokines, which can cause organ damage and ultimately result in organ failure and death in critically ill patients [95],[96]. This multisystemic immune dysregulation is what causes viral sepsis in these patients. By spreading methodically, the localized inflammatory response brought on by viral infection draws immune cells to the particular infected organs. Necrosis factor alpha (TNF-α) and gamma interferon levels were concurrently elevated, according to Karki et al. [97], which was the cause of the inflammatory cell death. Patients with severe MERS-CoV and SARS-CoV infections were also shown to have cytokine-releasing syndrome. Controlled cytokine release is crucial in preventing SARS-CoV-2 infection, but Leisman et al. [98] found that dysregulated synthesis of antiviral and proinflammatory mediators is the main factor in multiorgan failure and acute respiratory distress syndrome. Increased levels of IL-1, IL-1 receptor antagonist (IL1RA), IL-2, IL-6, IL-7, IL-10, tumor necrosis factor (TNF-α), IFN-gamma, granulocyte-macrophage colony-stimulating factor (G-CSF), fibroblast growth factor, platelet-derived growth factor (PDGF) and vascular endothelial growth factor were found in COVID-19 patients [98]. According to clinical studies, the severity of COVID-19 was found to significantly correlate with serum levels of IL-1, IL-2, IL-6, IL-7, IL-10, TNF-α, G-CSF, CCL2, CCL3, CXCL8 and CXCL10 [99]. Additionally, to support earlier research, a significant plasma rise in IL-6, IL-10 and TNF-α in COVID-19 patients compared to those with mild symptoms revealed a negative correlation between the recovery stage and plasma cytokine concentration in the COVID-19 patients [100],[101]. IL-6, IL-1β and other chemokines were seen upregulated in a different study of bronchoalveolar lavage fluid (BALF) in COVID-19 patients with severe and mild SARS-CoV-2, confirming the recruitment of these cytokines and chemokines to the inflamed lung and their presence in the profiled blood of SARS-CoV-2 patients [101],[103]. Since there is a link between the plasma levels of patients with severe COVID-19 and the level of pulmonary inflammation and viral load, these immune cells can be used as helpful biomarkers for testing and monitoring SARS-CoV-2 illness development [86]. It is important to note that the COVID-19 cytokine releasing syndrome is caused by coordinated actions of immune cells including macrophages, dendritic cells, neutrophils, monocytes, B cells, T cells, NK cells and tissue-resident cells like epithelial and endothelial cells with cytokines and chemokines [100].
In order to prevent viral clearance and postpone the emergence of adaptive immunity, the SARS-CoV-2 virus and other coronaviruses employ a variety of evasion techniques. To disrupt the interferon (IFN) signaling system, they accomplish this by encoding a diverse variety of viral structural proteins and non-structural proteins (nsp). The SARS-CoV-2 virus and other coronaviruses are susceptible to cascades of interferon-mediated antiviral responses that are triggered by the interferon (IFN) signaling pathway [104]. A protein complex (retinoic inducible gene I (RIG-I), adapter protein mitochondrial antiviral signaling protein (MAVS) and type 1 IFN induction turn on Janus kinase 1 (JAK1), tyrosine kinase 2 (Tyk2) and interferon regulatory factor 3 (IRF3)), which transduces signals downstream of the IFN-receptor to bind interferon-stimulated response elements (ISREs). In order to decrease the type 1 IFN pathway and evade the host immune response, the SARS-CoV-2 inhibits STAT phosphorylation, nuclear translocation and activation of interferon regulatory factor 3 (IRF3) [105]. SARS-CoV-2 encodes non-structural proteins like nsp1, nsp6, nsp8, nsp9, nsp13 and nsp16 that play integral roles in inhibiting host transcription, translation and protein transport [79],[86],[106]. Studies have shown that nsp6 and nsp13 interact with TANK binding kinase 1 (TBK1) to antagonize IFN signaling in order to prevent IRF3 phosphorylation [107]. The SARS-CoV-2 ORF6 was demonstrated to efficiently bind importin karyopherin α2 (KPNA2) to prevent IRF3 nuclear translocation [79],[108],[109]. In addition, open reading frame 8 (ORF8) and N glycoprotein were reported to inhibit IFN signaling by suppressing phosphorylation and nuclear translocation of STAT1 and STAT2, while ORF8 and nsp1 impair the expression of IFN-stimulated response elements (ISRE)-driven transcription of interferon stimulated genes (ISGs) and production of IFN in epithelial cells of tissues [90].
Viral proteases such as the papain-like protease initiate proteolytic cleavage and processing of viral polyproteins such as the ubiquitin-like molecule ISG15 found in positive-strand RNA viruses [110]. Zhang et al. [90] reported two viral proteases, PLpro and 3Clpro or main protease (Mpro), encoded in the genome of SARS-CoV-2 that play pivotal roles in viral polyprotein cleavage during viral replication. The essential modulators of IFN signaling, STAT2 and nuclear factor Kappa B essential modulator (NEMO), are cleaved by the main protease, a 3C-like protease (3Clpro), while a papain-like protease (PLpro) cleaves the ubiquitin-like molecule ISG15 with the aim to attenuate type 1 IFN responses [111],[112].
The SARS-CoV-2 virus has also developed another strategy to shut off host gene expression by exploring the host translational machinery to degrade host mRNA [113],[114]. Small ribosomal 40S subunits favorably bind no-structural protein 1 (nsp1) to interrupt mRNA translation at different stages during initiation to suppress the synthesis of antiviral genes [115],[116]. In addition, non-structural proteins such as nsp10 and nsp14 have been implicated in shutting off the synthesis of antiviral genes by exerting translational inhibition activity geared toward stalling the transcription of interferon stimulate genes (ISGs) to release type 1 IFN by forming the nsp10-nsp14 complex [117].
Studies have shown that SARS-CoV-2 can trigger multiple complement cascades through three major pathways, which are the lactic pathway, the classical pathway and the alternative pathway of the complement system [114],[118]. The complement system coordinates highly ordered interactions involving more than 50 proteins present on cell surfaces and in the plasma [114]. During viral infection, complement cascade hyperactivation makes the S glycoprotein interact with lectin pathway recognition molecules to activate the alternative pathway while the N glycoprotein interact with lectin pathway effector enzyme mannose-binding lectin-associated serine protease 2 (MASP-2) to activate the lectin pathway, which leads to severe inflammatory lung damage [119],[120]. In addition, the deleterious effect of the complement system in eliminating MASP-2 or blocking the N glycoprotein–MASP-2 interaction has been implicated as a major cause of N protein-induced complement hyperactivation and lung injury, which can consequently lead to lung failure [121],[122].
At the early stage of the pandemic, clinical scientists had limited scientific information about the pathogenesis and mode of transmission of SARS-CoV-2, which made treatment and prevention very difficult. This created the urgency for clinical researchers to explore viable means of mitigating the surge of reported cases of infection and increased deaths. Some of the therapeutic strategies used for treatment and prevention of COVID-19 spread include drug repurposing, experimental therapies, and vaccination.
Molnupiravir is a broad-spectrum oral antiviral medicine that works by acting on the RdRp enzyme of SARS-CoV-2 to inhibit viral replication and prevent severe symptoms [123]. It is usually used to treat early SARS-CoV-2 infection after neutralizing monoclonal antibodies (nMAbs). Early treatment with molnupiravir from a phase 3 double-blind, randomized, placebo-controlled trial has shown credible results in terms of reduced viral load, risk of hospitalization and mortality in at-risk unvaccinated individuals [124]. Molnupiravir is characterized by common side effects such as feeling dizzy and headaches and is not recommended for pregnant women [125]. In addition, the potential effect of molnupiravir on bone and cartilage of patients younger than 18 years has been reported by Gandh et al. [125]; thus, it should not be prescribed for patients below 18 years.
Nirmatrelvir-ritonavir (Paxlovid) is a SARS-CoV-2 protease inhibitor composed of two antiviral agents (oral pill) used to treat COVID-19 patients with underlying conditions that increase the risk of severe COVID-19 symptoms [123]. Nirmatrelvir-ritonavir has been reported to inhibit viral replication as well as reduce viral load in COVID-19 patients with mild to moderate symptoms and a risk of hospitalization [123]. It is more effective than molnupiravir and is also considered for individuals aged 12 or older or 65 or older [126],[127]. Nirmatrelvir-ritonavir is authorized for use within 5 days of COVID-19 symptom onset in nonhospitalized patients, but it is devoid of common side effects such as nausea, diarrhea, and dizziness [125]. Molecular docking approach was used to predict the binding affinity of ritonavir (a component of Nirmatrelvir-ritonavir ) with SARS-CoV-2 main protease complex structure through Cavity-detection guided Blind Docking (CB-Dock2) computation method (Figure 1a). Ritonavir binds to the SARS-CoV-2 main protease active site and prevents cleavage of the viral polyproteins thereby forming immature non-infectious viral particles. The molecular interactions between ritonavir and SARS-CoV-2 main protease (Mpro) showed high binding affinity with binding energy of −7.8 kcal/mol. The molecular interactions produced eight hydrophobic interactions with MET165, MET49, ASN142, THR25, THR26, LEU27, GLU166, HIS41and one hydrogen bond with THR190 whereas three weak hydrogen bond with GLN189 and MET165 (Figure 1b). In addition, nirmatrelvir-ritonavir is not recommended for patients with severe kidney or liver disease and should not be prescribed to pregnant patients or patients attempting to become pregnant [125].
Fluvoxamine is an antidepressant medication of the selective serotonin reuptake inhibitor (SSRI) and sigma-1 receptor agonist (S1R) class. It is approved by the Food and Drug Administration (FDA) to treat patients with obsessive compulsive disorder (OCD); however, in the course of repurposing drugs for COVID-19 treatment, fluvoxamine was used for clinical trials [128]. Fluvoxamine interacts with the sigma-1 receptor on immune cells to downregulate the expression of inflammatory genes. Previous studies by Calusic et al. [129] showed fluvoxamine to be effective and safe for the treatment of COVID-19 intensive care unit (ICU) patients, but due to insufficient evidence from clinical trials to support this claim, the FDA did not approved fluvoxamine for the treatment of COVID-19.
Remdesivir is an antiviral medication known to inhibit viral RNA-dependent RNA polymerase in vitro against SARS-CoV-2 [130]. The Food and Drug Administration (FDA) approved remdesivir for treatment of hospitalized COVID-19 patients, especially adults and pediatrics (12 years of age or older and weighing at least 40 kg) [131],[132]. Clinical evidence from a randomized double-blind placebo-controlled trial showed 87% lower risk of hospitalization than placebo when at-risk non-hospitalized patients with COVID-19 were treated with a 3-day course of remdesivir; however, several trials found no significant effect of remdesivir on patient outcomes [133],[134].
Sotrovimab is an anti-SARS-CoV-2 neutralizing antibody medicine known to be very effective in vitro against alpha, beta, gamma and delta SARS-CoV-2 variants. It is a neutralizing monoclonal antibody (nMAb). Neutralizing monoclonal antibodies (nMAbs) are synthetic proteins that act like human antibodies in the immune system [135]. They are made by cloning an antibody that can stick to the spike protein of the virus and neutralize it before migrating into the lungs, hence preventing transmission and reinfection [136]. It is administered to non-hospitalized patients as an infusion.
The COVID-19 pandemic outbreak in 2020 overstretched health facilities and world economies and caused unprecedented deaths across the world [137]. These key indices, out of several others, made governments, the World Health Organization (WHO), health institutions, pharmaceutical companies, universities, research institutions and other partners invest substantial capital and human resources in sequencing the SARS-CoV-2 genome for the purpose of developing vaccines against COVID-19 [137],[138]. The approach of drug repurposing to stem the spread of SARS-CoV-2 did not yield the expected results; thus, vaccines were the available antidotes [139]. Vaccines are biologics that provide active, acquired immunity through the production and release of antibodies against diseases. This is achieved through a process called vaccination, which is simple, safe and effective and is therefore rightly considered one of the most economical healthcare interventions [137]. Vaccines could serve as prophylaxis administered to healthy individuals to prevent infection with a disease-causing organism or therapeutics that are administered to fight an already existing illness in an individual by persuading the immune system to fight harder [29]. The spike S glycoprotein of SARS-CoV-2 has been the prime target for most research groups in developing vaccines, explored for its antigenic and immunogenic value [30]. There are different types of SARS-CoV-2 vaccine platforms, which are inactivated vaccines, live virus vaccines, viral vector-based vaccines, nucleic acid vaccines and protein-based vaccines.
Inactivated vaccines are traditionally produced by inactivating in vitro-cultured viruses (whole viral particles) using heat or formaldehyde while maintaining the integrity of the virus particles to substantially stimulate the immune response. This is one of the oldest procedures for vaccine design and development, as it is noninfectious, safer, more stable and easy to produce and has successfully been deployed in the production of the Hepatitis A vaccine and the polio vaccine [139]. The concept of designing inactivated vaccines is to utilize the whole virus in killed form to develop vaccines that can elicit a robust immune response without host cell interference [139]. These vaccines have a relatively short development cycle, thus guaranteeing fast production of safe and effective vaccines with no side effects. This could be attested to when about half of the COVID-19 inactivated vaccines progressed into phase III and IV clinical trials, with some of them being approved for distribution. Notable vaccines that fall into this platform include Covaxin, BBIBP-CorV, Sinopharm (Wuhan), CoronaVac, and CoviVac [140]–[150] (Table 1). Inactivated vaccines have antigen neutralizing efficacy on SARS-CoV-2 variants of concern, including delta and omicron variants. Decreased immunogenicity was probably observed against mutant strains; therefore, repeated doses are needed to sustain immune responses [151],[152]. These vaccines have been reported to confer 50–90% protection against SARS-CoV-2 variants, are noninfectious in immunosuppressed individuals and are suitable for long-term preservation (freeze-drying) and transportation [153]–[155]. Since the cultured viruses have been inactivated and are therefore nonreplicating, it is worthy of noting that inactivated vaccines require adjuvants such as aluminum salt (alum) to increase their immunogenicity.
Vaccine name | Platform | Target | Efficacy* | Efficacy against variant strain (reduction%) | Formulation of booster dose | Storage | Reference |
**Pfizer BioNTech (BNT162b2) | mRNA-based vaccine (mRNA expressing a trimeric RBD) and BNT162b2 (mRNA expressing spike protein) | Full-length S protein with proline substitutions | 95% | Alpha B.1.1.7 (93%), Beta B.1351 (75%), Gamma (P1) (88%), Delta B1.617.2 (88%) and Omicron variants (55.9%) | Bivalent | −70 °C (shipping) and 2–8 °C (6 months) | [141],[142] |
**Moderna (mRNA-1273) | mRNA-based vaccine (mRNA expressing spike protein) | Full-length S-2P protein | 95% | Alpha B.1.1.7 (88%), Beta B.1351 (73%), Gamma (P1) (63%), Delta B.1617.2 (77.8%) and Omicron variants (55.9%) | Bivalent | −20 °C (shipping) and 2–8 °C (6 months) | [141],[142] |
Johnson and Johnson (Ad26.CoV2) | Non-replicated viral vector (adenovirus Ad26) | Recombinant replication in competent adenovirus serotype 26 (Ad26) vector encoding full-length S protein | 66–85% | Alpha B.1.1.7 (73.9%), Beta B.1351 (31%), Gamma (P1) and (P2) (62.7%), Delta B.1617.2 (65%) and Omicron variants (55.9%) | Monovalent | 2-8 °C (3 month) and −20 °C (2 years) | [143] |
Oxford/Astrazeneca (ChAdOx1) | Non-replicated viral vector (ChAdOx1-S-(AZD1222)) | Chimpanzee adenovirus vectored vaccine (ChAdOx1) expressing S protein | 70% | Alpha B.1.1.7 (73.9%), Beta B.1351 (31%), Gamma (P1) and (P2) (62.7%), Delta B.1617.2 (65%) and Omicron variants (55.9%) | Monovalent | 2–8 °C (6 months) | [144],[145] |
Novavax (NVX CoV2373) | Recombinant protein vaccine (spike protein + matrix-M adjuvant) | S protein with matrix-M adjuvant | 90% | Alpha B.1.1.7 (89.7%), Beta B.1351 (51.1%), Gamma P1 (91.8%), Delta B.1617.2 (78.7%) and Omicron variants (55.9%) | Monovalent, saponin | 2–8 °C (6 months) | [144],[143] |
Sinovac–coronaVac vaccine | Inactivated vaccine (inactivated virion + aluminium hydroxide) | Whole inactivated SARS-CoV-2 | 50–84% | Alpha B.1.1.7 (71–91%), Beta B1.351 variants (70%), delta B.1617.2 (52%), Gamma (P1) (50%), Omicron BA.2 (62.65%) | Monovalent, aluminum salts | 2–8 °C (6 months) | [146],[147] |
Sputnik V (Gam-Covid-Vac) | Non-replicated viral vector (adenovirus Ad5 and Ad26) | Recombinant Ad26 and recombinant Ad5 encoding fulllength S protein | 92% | Alpha B.1.1.7 (85.7%), Beta B.1351 (81.17%), Delta B.1617.2 (65.35%) and Omicron variants (56.9%) | Monovalent | 2–8 °C (6 months) | [148],[149] |
*: Efficacy against original strain and protection from severe infections; **: Bivalent COVID-19 vaccine approved by the Food and Drug Administration (FDA).
Live virus vaccines, also called live attenuated viral vaccines, are designed using virulence gene knockout and codon pair deoptimization concepts by passing the weakened viruses through cell cultures to produce non-pathogenic vaccines with the inherent ability to induce lifelong innate immunity [156]. The codon pair deoptimization-based method was used by Trimpert et al. [157] to mutate the S protein by knocking out an amino acid at position 283 on the spike protein to genetically modify the SARS-CoV-2 genes and thus attenuate the virulence of the virus [158]. Codon pair deoptimization, also known as synthetic attenuated virus engineering, is a novel technique that utilizes suboptimal or underrepresented codon pairs to achieve attenuation of recorded SARS-CoV-2 [156]. The strategy involves synthetic recording of viral genomes that alters the positions of synonymous codons, thereby increasing the number of suboptimal codon pairs and CpG dinucleotides in recorded genomes [159]. However, the retained viral amino acid sequences induce innate, mucosal, cellular and humoral immunity against viral structural and nonstructural proteins and also protect the upper respiratory tract through nasal inhalation [2]. Live attenuated viral vaccines are very reactive, although there are potential safety concerns for individuals with weakened immune systems due to the reverse genetics of virulent strains. At the moment, no live attenuated viral vaccine has been approved for use by the WHO. However, COVI-VAC and MV-014-212 vaccines were approved for clinical trials [159].
The viral vector vaccines are engineered viruses with replication attenuated (Adenovirus shell) carrying DNA encoding a SARS-CoV-2 viral protein capable of evoking strong Th1 immune responses. Non-replicating viral vector-based vaccinations convey genetic material of a specific antigen to the host cell in order to stimulate immunization against the targeted antigen [160],[161]. Vaccinations based on viral vectors are not new: “(a) high-efficiency gene transduction; (b) highly selective delivery of genes to target cells; and (c) generation of powerful immune responses and improved cellular immunity” are the benefits of viral vectors. There are seven viral vector-based vaccinations in use as of June 2021 [159]. Two of them are for the Ebola virus, and five are for the COVID-19 virus. The viral vector-based vaccines approved by WHO for emergencies are AZD1222 (AstraZeneca-University of Oxford) and Ad26.COV-2-S (Johnson & Johnson) [162] (Table 1).
The SARS-CoV-2 viral-like particle vaccines are empty virus shell without infectious viral genome. They are non-infectious assemblies of viral structural proteins with very good adjuvant potential to induce strong immune response [156]. Virus-like particle vaccines induce both humoral and cellular immune response through action of binding unto the ACE2 on the S protein [163]. The biology and safety of virus-like particle vaccines is well established, as is the technology process [156]. A very good example of a SARS-CoV-2 viral-like particle vaccine produced by Premas Biotech is Triple-Antigen vaccine [159].
The concept of an mRNA-based vaccine dates back to years of designing therapeutics to address cancer and other infectious diseases. This technology involves the in vitro synthesis of antigen-encoding mRNA capable of stimulating the production of specific proteins to induce strong humoral and cellular immune responses [164],[165]. BNT162b2 by Pfizer-BioNTech and mRNA-1273 by Moderna Biotechnologies Inc. are the most effective mRNA vaccines authorized by the FDA and WHO for emergency use against SARS-CoV-2 [154],[166] (Table 1). The choice of BNT162b2 and mRNA-1273 is predicated on the strong Th1 and GC B cell immune responses exerted by the nucleoside-modified mRNA encoding the full-length S protein encapsulated in lipid nanoparticles, vector, viral protein or polypeptide [167]. COVID-19 vaccines can only convey the genetic information of the antigen by synthesizing the corresponding DNA or RNA of the viral protein [168]. Messenger RNA vaccines are stored at low temperatures to avoid degradation (temperatures of −80 to −60 °C for BNT162b2 and −25 to −15 °C for mRNA-1273).
DNA vaccines are produced from genetically engineered plasmids that contain viral antigens. This vaccine platform containing the viral antigens uses a vector to deliver itself into the host cell in order to initiate the production of viral proteins by transcription in the nucleus and translation in the cytoplasm to induce strong immunogenicity. The prospects of DNA vaccine research on HIV, malaria and influenza have shown remarkable progress over the years [169]. The most common COVID-19 DNA vaccines in clinical trials include INO-4800 COVID-19 vaccine, AG0301-COVID-19 vaccine, and nCov vaccine; however, no DNA vaccine has been approved for emergency use by the WHO [170]. Furthermore, studies have shown that DNA vaccines are safe, are more stable than mRNA vaccines, have a low production risk and can be stored for a long duration either in refrigeration or at room temperature, but they have been shown to induce slow immune responses [171],[172].
Protein subunit vaccines are primarily recombinant proteins engineered from antigenic proteins of the full-length SARS-CoV-2 spike glycoprotein using an established cell expression system such as the baculovirus-Spodoptera frugiperda (Sf9) insect cell expression system, yeasts, bacteria and human embryonic kidney cells [173]. The process involves the selection of a gene that encodes the spike glycoprotein or receptor binding domain (RBD), followed by fusion of the encoded gene with an adenovirus [159]. Reports have shown protein subunit vaccines capable of eliciting Th1 cell immunogenicity. At the present, NVX-CoV2373 (Novavax) is the only protein subunit vaccine approved by the WHO for emergency use [173] (Table 1). Protein subunit vaccines are easy to produce, safe, robust and more effective.
The evolution of the omicron variant (BA.1), whose target spike (S) glycoprotein and receptor binding domain contain a staggering number of mutations, makes it highly transmissible with high records of hospitalization and reinfection. Considering the concerns, the attention of public health personnel on the threats posed by the omicron variant (BA.1) to the efficacy and effectiveness of the COVID-19 vaccines as a matter of emergency led to the creation of bivalent COVID-19 vaccines [166]. A bivalent COVID-19 vaccine, also called an “updated” COVID-19 vaccine booster dose, is a formulation that houses the original virus strain, which provides broad immunity against COVID-19, and the omicron variant (BA.1), which provides better protection against COVID-19 caused by the omicron variant (BA.1) [174]. The first of this kind approved by the FDA were produced by Pfizer-BioNtech (containing 15 µg of mRNA directed against the ancestral strain of SARS-CoV-2 and 15 µg directed against BA.1) and Moderna (containing 15 µg of mRNA directed against the ancestral strain of SARS-CoV-2 and 15 µg directed against BA.1) [141] (Table 1).
The FDA's Vaccines and Related Biological Products Advisory Committee report shows that the research findings of Pfizer–BioNTech and Moderna revealed not much significant difference (1.5 to 1.75 times as high) in titer values of the bivalent COVID-19 vaccine compared with the monovalent boosters [141]. The gradual replacement of the omicron BA.1 strain by the more immune-evasive and contagious omicron sub-strains BA.4 and BA.5 caused the FDA to authorize the immediate use of the bivalent COVID-19 vaccine as agreed by the US Government led by the Biden administration [174]. However, research findings from David Ho and colleagues examining the levels of neutralizing antibodies against BA.4 and BA.5 strains in individuals after the monovalent and bivalent COVID-19 vaccines were administered depict no significant difference in neutralization of the SARS-CoV-2 variant, including the antibodies against BA.4 and BA.5 strains [136]. Similarly, report findings from Barouch and colleagues also revealed no clinical difference in the CD4+ or CD8+ T-cell responses between participants in the monovalent booster group and those in the bivalent booster group [141]. This outcome could be attributed to the primed memory response mechanism already initiated by the immune cells of previously vaccinated individuals against the ancestral SARS-CoV-2 on epitopes shared between BA.4 and BA.5 and the ancestral strain [142].
A more robust strategy is in place to develop BA.4 and BA.5 mRNA vaccines with a greater quantity of BA.4 and BA.5 mRNA to target specific epitopes peculiar to the BA.1 strain and sub-variants (of BA.4 and BA.5). This was evidently proven through BioNTech's published data on its bivalent vaccine containing the BA.1 strain, which showed significant BA.1 specific neutralizing antibody responses compared to individuals vaccinated with the monovalent vaccine containing 30 or 60 µg of BA.1 mRNA or a bivalent vaccine containing 30 µg of BA.1 mRNA and 30 µg of ancestral strain mRNA [141]. From the findings so far, it is obvious that the bivalent COVID-19 vaccine elicits similar immune responses as a booster vaccine with the monovalent vaccines [141],[142]. Considering the trend of continued mutations as observed on the spike (S) glycoprotein and receptor binding domain (RBD) of SARS-CoV-2, which led to more immune-evasive strains such as BQ.1, BQ.1.1, BF.7, XBB and XBB.1, the attention of researchers and clinicians might be tailored toward the design and development of variant-specific vaccines for future interventions [141].
The authorization of therapeutics and vaccines by the WHO and other regulatory bodies has gone a long way toward conferring immunity and protection against SARS-CoV-2. However, the continued mutations as observed on the spike (S) glycoprotein and receptor binding domain (RBD) which may likely lead to the emergence of new unfamiliar strains is a genuine cause of concern. Therefore, constant and steady research is required to improve present knowledge on viral pathogenesis and also to design and develop variant-specific vaccines for future interventions.
The authors declare they have not used Artificial Intelligence (AI) tools in the creation of this article.
[1] |
Lauer SA, Grantz KH, Bi Q, et al. (2020) The incubation period of coronavirus disease 2019 (COVID-19) from publicly reported confirmed cases: Estimation and application. Ann Intern Med 172: 577-582. https://doi.org/10.7326/M20-0504 ![]() |
[2] |
Dai L, Gao GF (2021) Viral targets for vaccines against COVID-19. Nat Rev Immunol 21: 73-82. https://doi.org/10.1038/s41577-020-00480-0 ![]() |
[3] |
Townsend JP, Hassler HB, Wang Z, et al. (2021) The durability of immunity against reinfection by SARS-CoV-2: a comparative evolutionary study. Lancet Microbe 2: e666-e675. https://doi.org/10.1016/S2666-5247(21)00219-6 ![]() |
[4] |
Choi JY, Smith DM (2021) SARS-CoV-2 variants of concern. Yonsei Med J 62: 961-968. https://doi.org/10.3349/ymj.2021.62.11.961 ![]() |
[5] |
Petersen E, Ntoumi F, Hui DS, et al. (2022) Emergence of new SARS-CoV-2 variant of concern omicron (B.1.1.529)—highlights Africa's research capabilities, but exposes major knowledge gaps, inequities of vaccine distribution, inadequacies in global COVID-19 response and control efforts. Int J Infect Dis 114: 268-272. https://doi.org/10.1016/j.ijid.2021.11.040 ![]() |
[6] |
Alsaadi E, Jones IM (2019) Membrane binding proteins of coronaviruses. Future Virol 14: 275-286. https://doi.org/10.2217/fvl-2018-0144 ![]() |
[7] |
Walls AC, Park Y, Tortorici MA, et al. (2020) Structure, function and antigenicity of the SAR-CoV-2 spike glycoprotein. Cell 180: 281-292. https://doi.org/10.1016/j.cell.2020.02.058 ![]() |
[8] |
Chukwudozie OS, Gray CM, Fagbayi TA, et al. (2021) Immuno-informatics design of a multimeric epitope peptide based vaccine targeting SARS-CoV-2 spike glycoprotein. PloS One 16: e0248061. https://doi.org/10.1371/journal.pone.0248061 ![]() |
[9] |
Amanat F, Krammer F (2020) SARS-CoV-2 vaccines: Status report. Immunity 52: 583-589. https://doi.org/10.1016/j.immuni.2020.03.007 ![]() |
[10] |
Tosta E (2021) The protective immunity induced by SARS-CoV-2 infection and vaccination: a critical appraisal. Explor Immunol 1: 199-225. https://doi.org/10.37349/ei.2021.00014 ![]() |
[11] |
Sumon TA, Hussain MA, Hasan MT, et al. (2021) A revisit to the research updates of drugs, vaccines, and bioinformatics approaches in combating COVID-19 pandemic. Front Mol Biosci 7: 585899. https://doi.org/10.3389/fmolb.2020.585899 ![]() |
[12] |
Garg M, Maralakunte M, Kumar Y, et al. (2021) Vaccine-induced immune responses against SARS-CoV-2 infections. Explor Immunol 1: 356-373. https://doi.org/10.37349/ei.2021.00024 ![]() |
[13] |
Sonani B, Aslam F, Goyal A, et al. (2021) COVID-19 vaccination in immunocompromised patients. Clin Rheumatol 40: 797-798. https://doi.org/10.1007/s10067-020-05547-w ![]() |
[14] |
Pollard AJ, Bijker EM (2021) Publisher correction: A guide to vaccinology: from basic principles to new developments. Nat Rev Immunol 21: 129. https://doi.org/10.1038/s41577-020-00497-5 ![]() |
[15] |
Peng Y, Mentzer AJ, Liu G, et al. (2020) Broad and strong memory CD4+ and CD8+ T cells induced by SARS-CoV-2 in UK convalescent individuals following COVID-19. Nat Immunol 21: 1336-1345. https://doi.org/10.1038/s41590-020-0782-6 ![]() |
[16] |
Kim YI, Casel MAB, Choi YK (2022) Transmissibility and pathogenicity of SARS-CoV-2 variants in animal models. J Microbiol 60: 255-267. https://doi.org/10.1007/s12275-022-2033-z ![]() |
[17] |
Chemaitelly H, Yassine HM, Benslimane FM, et al. (2021) mRNA-1273 COVID-19 vaccine effectiveness against the B.1.1.7 and B.1.351 variants and severe COVID-19 disease in Qatar. Nat Med 27: 1614-1621. https://doi.org/10.1038/s41591-021-01446-y ![]() |
[18] | (2021) WHOTracking SARS-CoV-2 variants.World Health Organization. Available from: https://www.who.int/en/activities/tracking-SARS-CoV-2-variants/ |
[19] |
Chaillon A, Smith DM, et al. (2021) Phylogenetic analyses of severe acute respiratory syndrome Coronavirus 2 (SARS-CoV-2) B.1.1.7 lineage suggest a single origin followed by multiple exportation events versus convergent evolution. Clin Infect Dis 73: 2314-2317. https://doi.org/10.1093/cid/ciab265 ![]() |
[20] |
Davies NG, Abbott S, Barnard RC, et al. (2021) Estimated transmissibility and impact of SARS-CoV-2 lineage B.1.1.7 in England. Science 372: eabg3055. https://doi.org/10.1126/science.abg3055 ![]() |
[21] |
Subissi L, von Gottberg A, Thukral L, et al. (2022) An early warning system for emerging SARS-CoV-2 variants. Nat Med 28: 1110-1115. https://doi.org/10.1126/science.abg3055 ![]() |
[22] |
Graham MS, Sudre CH, May A, et al. (2021) Changes in symptomatology, reinfection, and transmissibility associated with the SARS-CoV-2 variant B.1.1.7: an ecological study. Lancet Public Health 6: e335-e345. https://doi.org/10.1016/s2468-2667(21)00055-4 ![]() |
[23] |
Chavda VP, Patel AB, Vaghasiya DD, et al. (2022) SARS-CoV-2 variants and vulnerability at the global level. J Med Virol 94: 2986-3005. https://doi.org/10.1002/jmv.27717 ![]() |
[24] |
Meng B, Kemp SA, Papa G, et al. (2021) Recurrent emergence of SARS-CoV-2 spike deletion H69/V70 and its role in the Alpha variant B.1.1.7. Cell Rep 35: 109292. https://doi.org/10.1016/j.celrep.2021.109292 ![]() |
[25] |
Starr TN, Greaney AJ, Hilton SK, et al. (2020) Deep mutational scanning of SARS-CoV-2 receptor binding domain reveals constraints on folding and ACE2 binding. Cell 182: 1295-1310. https://doi.org/10.1016/j.cell.2020.08.012 ![]() |
[26] |
Collier DA, De Marco A, Ferreira IA, et al. (2022) Author correction: Sensitivity of SARS-CoV-2 B.1.1.7 to mRNA vaccine-elicited antibodies. Nature 608: E24. https://doi.org/10.1038/s41586-022-05103-3 ![]() |
[27] |
Dagan N, Barda N, Kepten E, et al. (2021) BNT162b2 mRNA COVID-19 vaccine in a nationwide mass vaccination setting. N Engl J Med 384: 1412-1423. https://doi.org/10.1056/nejmoa2101765 ![]() |
[28] |
Barbra AD, Gerlovin H, Madenci AL, et al. (2022) Comparative effectiveness of BNT162b2 and mRNA-1273 vaccines in U.S. veterans. N Engl J Med 386: 105-115. https://doi.org/10.1056/nejmoa2115463 ![]() |
[29] |
McNamara LA, Wiegand RE, Burke RM, et al. (2022) Estimating the early impact of the US COVID-19 vaccination programme on COVID-19 cases, emergency department visits, hospital admissions, and deaths among adults aged 65 years and older: an ecological analysis of national surveillance data. Lancet 399: 152-160. https://doi.org/10.1016/s0140-6736(21)02226-1 ![]() |
[30] |
George NI, Locke ER, O'Hare AM, et al. (2022) COVID-19 vaccination effectiveness against infection or death in a national US health care system: A target trial emulation study. Ann Intern Med 175: 352-361. https://doi.org/10.7326/m21-3256 ![]() |
[31] |
Mahase E (2021) Covid-19: Novavax vaccine efficacy is 86% against UK variant and 60% against South African variant. BMJ 372: n296. https://doi.org/10.1136/bmj.n296 ![]() |
[32] |
Mark WT, Self WH, Gaglani M, et al. (2022) Effectiveness of mRNA vaccination in preventing COVID-19-associated invasive mechanical ventilation and death—United States, March 2021–January 2022. MMWR Morb Mortal Wkly Rep 71: 459-465. https://doi.org/10.15585/mmwr.mm7112e1 ![]() |
[33] |
Khan A, Zia T, Suleman M, et al. (2021) Higher infectivity of the SARS-CoV-2 new variants is associated with K417N/T, E484K, and N501Y mutants: An insight from structural data. J Cell Physiol 236: 7045-7057. https://doi.org/10.1002/jcp.30367 ![]() |
[34] |
William NW, Ip S, Cooper JA, et al. (2022) Association of COVID-19 vaccines ChAdOx1 and BNT162b2 with major venous, arterial, or thrombocytopenic events: A population-based cohort study of 46 million adults in England. PLoS Med 19: e1003926. https://doi.org/10.1371/journal.pmed.1003926 ![]() |
[35] |
Andeweg SP, Vennema H, Veldhuijzen I, et al. (2023) Elevated risk of infection with SARS-CoV-2 Beta, Gamma, and Delta variants compared with Alpha variant in vaccinated individuals. Sci Transl Med 15: eabn4338. https://doi.org/10.1126/scitranslmed.abn4338 ![]() |
[36] |
Boehm E, Kronig I, Neher RA, et al. (2021) Novel SARS-CoV-2 variants: the pandemics within the pandemic. Clin Microbiol Infect 27: 1109-1117. https://doi.org/10.1016%2Fj.cmi.2021.05.022 ![]() |
[37] |
Kustin T, Harel N, Finkel U, et al. (2021) Evidence for increased breakthrough rates of SARS-CoV-2 variants of concern in BNT162b2-mRNA-vaccinated individuals. Nat Med 27: 1379-1384. https://doi.org/10.1038/s41591-021-01413-7 ![]() |
[38] |
Liu J, Liu Y, Xia H, et al. (2021) BNT162b2-elicited neutralization of B.1.617 and other SARS-CoV-2 variants. Nature 596: 273-275. https://doi.org/10.1038/s41586-021-03693-y ![]() |
[39] |
Xie X, Liu Y, Liu J, et al. (2021) Neutralization of SARS-CoV-2 spike 69/70 deletion, E484K and N501Y variants by BNT162b2 vaccine-elicited sera. Nat Med 27: 620-621. https://doi.org/10.1038/s41591-021-01270-4 ![]() |
[40] |
Singh H, Dahiya N, Yadav M, et al. (2022) Emergence of SARS-CoV-2 new variants and their clinical significance. Can J Infect Dis Med Microbiol 2022: 7336309. https://doi.org/10.1155/2022%2F7336309 ![]() |
[41] | (2021) FDAFact sheet for health care providers emergency use authorization (EUA) of bamlanivimab and etesevimab.US Food and Drug Administration. Available from: https://www.fda.gov/media/145802/download |
[42] |
Wang Z, Schmidt F, Weisblum Y, et al. (2021) mRNA vaccine-elicited antibodies to SARS-CoV-2 and circulating variants. Nature 592: 616-622. https://doi.org/10.1038/s41586-021-03324-6 ![]() |
[43] |
Prete CA, Buss LF, Buccheri R, et al. (2022) Reinfection by the SARS-CoV-2 Gamma variant in blood donors in Manaus, Brazil. BMC Infect Dis 22: 127. https://doi.org/10.1186/s12879-022-07094-y ![]() |
[44] |
Banho CA, Sacchetto L, Campos GRF, et al. (2022) Impact of SARS-CoV-2 Gamma lineage introduction and COVID-19 vaccination on the epidemiological landscape of a Brazilian city. Commun Med 2: 41. https://doi.org/10.1038/s43856-022-00108-5 ![]() |
[45] |
Buss LF, Prete CA, Abrahim CMM, et al. (2021) Three-quarters attack rate of SARS-CoV-2 in the Brazilian Amazon during a largely unmitigated epidemic. Science 371: 288-292. https://doi.org/10.1126/science.abe9728 ![]() |
[46] |
Sabino EC, Buss LF, Carvalho MPS, et al. (2021) Resurgence of COVID-19 in Manaus, Brazil, despite high seroprevalence. Lancet 397: 452-455. https://doi.org/10.1016/s0140-6736(21)00183-5 ![]() |
[47] |
Volz E, Mishra S, Chand M, et al. (2021) Assessing transmissibility of SARS-CoV-2 lineage B.1.1.7 in England. Nature 593: 266-269. https://doi.org/10.1038/s41586-021-03470-x ![]() |
[48] |
Lucas C, Vogels CBF, Yildirim I, et al. (2021) Impact of circulating SARS-CoV-2 variants on mRNA vaccine-induced immunity. Nature 600: 523-529. https://doi.org/10.1038/s41586-021-04085-y ![]() |
[49] |
Collier DA, Ferreira IATM, Kotagiri P, et al. (2021) Age-related immune response heterogeneity to SARS-CoV-2 vaccine BNT162b2. Nature 596: 417-422. https://doi.org/10.1038/s41586-021-03739-1 ![]() |
[50] |
Wang P, Casner RG, Nair MS, et al. (2021) Increased resistance of SARS-CoV-2 variant P.1 to antibody neutralization. Cell Host Microbe 29: 747-751. https://doi.org/10.1101/2021.03.01.433466 ![]() |
[51] |
Firouzabadi N, Ghasemiyeh P, Moradishooli F, et al. (2023) Update on the effectiveness of COVID-19 vaccines on different variants of SARS-CoV-2. Int Immunopharmacol 117: 109968. https://doi.org/10.1016/j.intimp.2023.109968 ![]() |
[52] | (2021) WHOClassification of omicron (B.1.1.529): SARS-CoV-2 variant of concern.World Health Organization. Available from: https://www.who.int/news/item/26-11-2021-classification-of-omicron-(b.1.1.529)-sars-cov-2-variant-of-concern |
[53] |
Lauring AS, Malani PN (2021) Variants of SARS-CoV-2. JAMA 326: 14181. https://doi.org/10.1001/jama.2021.14181 ![]() |
[54] |
Alkhatib M, Svicher V, Salpini R, et al. (2021) SARS-CoV-2 variants and their relevant mutational profiles: Update summer 2021. Microbiol Spectr 9: e0109621. https://doi.org/10.1128/spectrum.01096-21 ![]() |
[55] | Cascella M, Rajnik M, Aleem A, et al. (2023) Features, evaluation, and treatment of coronavirus (COVID-19). StatPearls. Tampa: StatPearls Publishing. |
[56] |
Harvey WT, Carabelli AM, Jackson B, et al. (2021) SARS-CoV-2 variants, spike mutations and immune escape. Nat Rev Microbiol 19: 409-424. https://doi.org/10.1038/s41579-021-00573-0 ![]() |
[57] |
Fisman DN, Tuite AR (2021) Progressive increase in virulence of novel SARS-CoV-2 variants in Ontario, Canada. CMAJ 193: E1619-E1625. https://doi.org/10.1503/cmaj.211248 ![]() |
[58] |
King KL, Wilson S, Napolitano JM, et al. (2022) SARS-CoV-2 variants of concern Alpha and Delta show increased viral load in saliva. PloS One 17: e0267750. http://dx.doi.org/10.1371/journal.pone.0267750 ![]() |
[59] | (2021) CDC, SARS-CoV-2 variant classifications and definitions.Centers for Disease Control and Prevention. Available from: https://www.cdc.gov/coronavirus/2019-ncov/variants/variant-classifications.html |
[60] |
Kannan SR, Spratt AN, Cohen AR, et al. (2021) Evolutionary analysis of the Delta and Delta Plus variants of the SARS-CoV-2 viruses. J Autoimmun 124: 102715. https://doi.org/10.1016/j.jaut.2021.102715 ![]() |
[61] |
Planas D, Veyer D, Baidaliuk A, et al. (2021) Reduced sensitivity of SARS-CoV-2 variant delta to antibody neutralization. Nature 596: 276-280. https://doi.org/10.1038/s41586-021-03777-9 ![]() |
[62] |
Tchesnokova V, Kulasekara H, Larson L, et al. (2021) Acquisition of the L452R mutation in the ACE2-binding interface of spike protein triggers recent massive expansion of SARS-CoV-2 variants. J Clin Microbiol 59: e0092121. https://doi.org/10.1128/jcm.00921-21 ![]() |
[63] |
Sheikh A, Mcmenamin J, Taylor B, et al. (2021) SARS-CoV-2 Delta VOC in Scotland: demographics, risk of hospital admission, and vaccine effectiveness. Lancet 397: 2461-2462. https://doi.org/10.1016/s0140-6736(21)01358-1 ![]() |
[64] |
Bernal JL, Andrews N, Gower C, et al. (2021) Effectiveness of COVID-19 vaccines against the B.1.617.2 (delta) variant. N Engl J Med 385: 585-594. https://doi.org/10.1056/nejmoa2108891 ![]() |
[65] |
Mohsin M, Mahmud S (2022) Omicron SARS-CoV-2 variant of concern: A review on its transmissibility, immune evasion, reinfection, and severity. Medicine 101: e29165. https://doi.org/10.1097/md.0000000000029165 ![]() |
[66] |
Ntoumi FPE, Hui DS, Abubakar A, et al. (2022) Emergence of new SARS-CoV-2 variant of concern omicron (B.1.1.529)—highlights Africa's research capabilities, but exposes major knowledge gaps, inequities of vaccine distribution, inadequacies in global COVID-19 response and control efforts. Int J Infect Dis 114: 268-272. https://doi.org/10.1016/j.ijid.2021.11.040 ![]() |
[67] |
Rio C, Malani PN (2022) COVID-19 in 2022—The beginning of the end or the end of the beginning?. JAMA 327: 2389-2390. https://doi.org/10.1001/jama.2022.9655 ![]() |
[68] |
Yamasoba D, Kimura I, Nasser H, et al. (2022) Virological characteristics of the SARS-CoV-2 Omicron BA.2 spike. Cell 185: 2103-2115. https://doi.org/10.1016/j.cell.2022.04.035 ![]() |
[69] |
Lassaunière R, Polacek C, Frische A, et al. (2022) Neutralizing antibodies against the SARS-CoV-2 omicron variant (BA.1) 1 to 18 weeks after the second and third doses of the BNT162b2 mRNA vaccine. JAMA Network Open 5: e2212073. https://doi.org/10.1001/jamanetworkopen.2022.12073 ![]() |
[70] |
Cao Y, Yisimayi A, Jian F, et al. (2022) BA.2.12.1, BA.4 and BA.5 escape antibodies elicited by Omicron infection. Nature 608: 593-602. https://doi.org/10.1038/s41586-022-04980-y ![]() |
[71] | Centers for Disease Control and Prevention.SARS-CoV-2 B.1.1.529 (Omicron) Variant United States. Morb Mortal Wkly Rep (2021) 70: 1731-1734. http://dx.doi.org/10.15585/mmwr.mm7050e1 |
[72] |
Wolter N, Jassat W, Walaza S, et al. (2021) Early assessment of the clinical severity of the SARS-CoV-2 omicron variant in South Africa. Lancet 399: 437-446. https://doi.org/10.1016/s0140-6736(22)00017-4 ![]() |
[73] |
Tegally H, Moir M, Everatt J, et al. (2022) Emergence of SARS-CoV-2 omicron lineages BA.4 and BA.5 in South Africa. Nat Med 28: 1785-1790. https://doi.org/10.1038/s41591-022-01911-2 ![]() |
[74] |
Callaway E (2021) Heavily mutated omicron variant puts scientists on alert. Nature 600: 21. https://doi.org/10.1038/d41586-021-03552-w ![]() |
[75] |
Liu L, Iketani S, Guo Y, et al. (2022) Striking antibody evasion manifested by the omicron variant of SARS-CoV-2. Nature 602: 676-681. https://doi.org/10.1038/s41586-021-04388-0 ![]() |
[76] |
Hoffmann M, Krüger N, Schulz S, et al. (2022) The Omicron variant is highly resistant against antibody-mediated neutralization: Implications for control of the COVID-19 pandemic. Cell 185: 447-456.e11. https://doi.org/10.1016/j.cell.2021.12.032 ![]() |
[77] |
Jiang S, Hillyer C, Du L, et al. (2020) Neutralizing antibodies against SARS-CoV-2 and other human coronaviruses. Trends Immunol 41: 355-359. https://doi.org/10.1016/j.it.2020.03.007 ![]() |
[78] |
Watanabe Y, Allen JD, Wrapp D, et al. (2020) Site-specific glycan analysis of the SARS-CoV-2 spike. Science 369: 330-333. https://doi.org/10.1126/science.abb9983 ![]() |
[79] |
Zhou H, Ni WJ, Huang W, et al. (2022) Advances in pathogenesis, progression, potential targets and targeted therapeutic strategies in SARS-CoV-2-induced COVID-19. Front Immunol 13: 834942. https://doi.org/10.3389/fimmu.2022.834942 ![]() |
[80] |
De Abajo FJ, Rodríguez-Martín S, Lerma V, et al. (2020) Use of renin-angiotensin-aldosterone system inhibitors and risk of COVID-19requiring admission to hospital: a case-population study. Lancet 395: 1705-1714. https://doi.org/10.1016/s0140-6736(20)31030-8 ![]() |
[81] |
Hoffmann M, Kleine-Weber H, Schroeder S, et al. (2020) SARS-CoV-2 cell entry depends on ACE2 and TMPRSS2 and is blocked by a clinically proven protease inhibitor. Cell 181: 271-280. https://doi.org/10.1016/j.cell.2020.02.052 ![]() |
[82] |
Xu H, Zhong L, Deng J, et al. (2020) High expression of ACE2 receptor of 2019-nCoV on the epithelial cells of oral mucosa. Int J Oral Sci 12: 8. https://doi.org/10.1038/s41368-020-0074-x ![]() |
[83] |
Lu S, Ye Q, Singh D, et al. (2021) The SARS-CoV-2 nucleocapsid phosphoprotein forms mutually exclusive condensates with RNA and the membrane-associated M protein. Nat Commun 12: 502. https://doi.org/10.1038/s41467-020-20768-y ![]() |
[84] |
Mandala VS, McKay MJ, Shcherbakov AA, et al. (2020) Structure and drug binding of the SARS-CoV-2 envelope protein transmembrane domain in lipid bilayers. Nat Struct Mol Biol 27: 1202-1208. https://doi.org/10.1038/s41594-020-00536-8 ![]() |
[85] |
Peng Y, Du N, Lei Y, et al. (2020) Structures of the SARS-CoV-2 nucleocapsid and their perspectives for drug design. EMBO J 39: e105938. https://doi.org/10.15252/embj.2020105938 ![]() |
[86] |
Ricci D, Etna MP, Rizzo F, et al. (2021) Innate immune response to SARS-CoV-2 infection: From cells to soluble mediators. Int J Mol Sci 22: 7017. https://doi.org/10.3390/ijms22137017 ![]() |
[87] |
Schultze JL, Aschenbrenner AC (2021) COVID-19 and the human innate immune system. Cell 184: 1671-1692. https://doi.org/10.1016/j.cell.2021.02.029 ![]() |
[88] |
Gu W, Gan H, Ma Y, et al. (2022) The molecular mechanism of SARS-CoV-2 evading host antiviral innate immunity. Virol J 19: 49. https://doi.org/10.1186/s12985-022-01783-5 ![]() |
[89] |
Wauters E, Van Mol P, Garg AD, et al. (2021) Discriminating mild from critical COVID-19 by innate and adaptive immune single-cell profiling of bronchoalveolar lavages. Cell Res 31: 272-290. https://doi.org/10.1038/s41422-020-00455-9 ![]() |
[90] |
Zhang S, Wang L, Cheng G, et al. (2022) The battle between host and SARS-CoV-2: Innate immunity and viral evasion strategies. Mol Ther 30: 1869-1884. https://doi.org/10.1016/j.ymthe.2022.02.014 ![]() |
[91] |
Paludan SR, Mogensen TH (2022) Innate immunological pathways in COVID-19 pathogenesis. Sci Immunol 7: eabm5505. https://doi.org/10.1126/sciimmunol.abm5505 ![]() |
[92] |
Thorne LG, Reuschl AK, Zuliani-Alvarez L, et al. (2021) SARS-CoV-2 sensing by RIG-I and MDA5 links epithelial infection to macrophage inflammation. EMBO J 40: e107826. https://doi.org/10.15252/embj.2021107826 ![]() |
[93] |
Cheemarla NR, Watkins TA, Mihaylova VT, et al. (2021) Dynamic innate immune response determines susceptibility to SARS-CoV-2 infection and early replication kinetics. J Exp Med 218: e20210583. https://doi.org/10.1084/jem.20210583 ![]() |
[94] |
Shah VK, Firmal P, Alam A, et al. (2020) Overview of immune response during SARS-CoV-2 infection: Lessons from the past. Front Immunol 11: 1949. https://doi.org/10.3389/fimmu.2020.01949 ![]() |
[95] |
Li H, Liu L, Zhang D, et al. (2020) SARS-CoV-2 and viral sepsis: observations and hypotheses. Lancet 395: 1517-1520. https://doi.org/10.1016/S0140-6736(20)30920-X ![]() |
[96] |
Mehta P, McAuley DF, Brown M, et al. (2020) COVID-19: consider cytokine storm syndromes and immunosuppression. Lancet 395: 1033-1034. https://doi.org/10.1016/S0140-6736(20)30628-0 ![]() |
[97] |
Karki R, Sharma BR, Tuladhar S, et al. (2021) Synergism of TNF-α and IFN-g triggers inflammatory cell death, tissue damage, and mortality in SARS-CoV-2 infection and cytokine shock syndromes. Cell 184: 149-168. https://doi.org/10.1016/j.cell.2020.11.025 ![]() |
[98] |
Leisman DE, Ronner L, Pinotti R, et al. (2020) Cytokine elevation in severe and critical COVID-19: a rapid systematic review, meta-analysis, and comparison with other inflammatory syndromes. Lancet Respir Med 8: 1233-1244. https://doi.org/10.1016/s2213-2600(20)30404-5 ![]() |
[99] |
Contoli M, Papi A, Tomassetti L, et al. (2021) Blood interferon-α levels and severity, outcomes, and inflammatory profiles in hospitalized COVID-19 patients. Front Immunol 12: 648004. https://doi.org/10.3389/fimmu.2021.648004 ![]() |
[100] |
Chen G, Wu D, Guo W, et al. (2020) Clinical and immunological features of severe and moderate coronavirus disease 2019. J Clin Invest 130: 2620-2629. https://doi.org/10.1172/jci137244 ![]() |
[101] |
Lau SKP, Lau CCY, Chan KH, et al. (2013) Delayed induction of proinflammatory cytokines and suppression of innate antiviral response by the novel Middle East respiratory syndrome coronavirus: implications for pathogenesis and treatment. J Gen Virol 94: 2679-2690. https://doi.org/10.1099/vir.0.055533-0 ![]() |
[102] |
Pereda R, González D, Rivero HB, et al. (2020) Therapeutic effectiveness of interferon alpha 2b treatment for COVID-19 patient recovery. J Interferon Cytokine Res 40: 578-588. https://doi.org/10.1089/jir.2020.0188 ![]() |
[103] |
Yang Y, Shen C, Li J, et al. (2020) Plasma IP-10 and MCP-3 levels are highly associated with disease severity and predict the progression of COVID-19. J Allergy Clin Immunol 146: 119-127. https://doi.org/10.1016/j.jaci.2020.04.027 ![]() |
[104] |
Schreiber G (2020) The role of type I interferons in the pathogenesis and treatment of COVID-19. Front Immunol 11: 595739. https://doi.org/10.3389/fimmu.2020.595739 ![]() |
[105] |
Sui L, Zhao Y, Wang W, et al. (2021) SARS-CoV-2 membrane protein inhibits type I interferon production through ubiquitin-mediated degradation of TBK1. Front Immunol 12: 662989. https://doi.org/10.3389/fimmu.2021.662989 ![]() |
[106] |
Li A, Zhao K, Zhang B, Hua R, et al. (2021) SARS-CoV-2 NSP12 protein is not an interferon-β antagonist. J Virol 95: e0074721. https://doi.org/10.1128/jvi.00747-21 ![]() |
[107] |
Konno Y, Kimura I, Uriu K, et al. (2020) SARS-CoV-2 ORF3b is a potent interferon antagonist whose activity is increased by a naturally occurring elongation variant. Cell Rep 32: 108185. https://doi.org/10.1016/j.celrep.2020.108185 ![]() |
[108] |
Geng H, Subramanian S, Wu L, et al. (2021) SARS-CoV-2 ORF8 forms intracellular aggregates and inhibits IFNγ-induced antiviral gene expression in human lung epithelial cells. Front Immunol 12: 679482. https://doi.org/10.3389/fimmu.2021.679482 ![]() |
[109] |
Wang W, Zhou Z, Xiao X, et al. (2021) SARS-CoV-2 nsp12 attenuates type I interferon production by inhibiting IRF3 nuclear translocation. Cell Mol Immunol 18: 945-953. https://doi.org/10.1038/s41423-020-00619-y ![]() |
[110] |
Lei J, Hilgenfeld R (2017) RNA-virus proteases counteracting host innate immunity. FEBS Lett 591: 3190-3210. https://doi.org/10.1002/1873-3468.12827 ![]() |
[111] |
Prescott L (2022) SARS-CoV-2 3CLpro whole human proteome cleavage prediction and enrichment/depletion analysis. Comput Biol Chem 98: 107671. https://doi.org/10.1016/j.compbiolchem.2022.107671 ![]() |
[112] |
Wang D, Fang L, Shi Y (2016) Porcine epidemic diarrhea virus 3C-like protease regulates its interferon antagonism by cleaving NEMO. J Virol 90: 2090-2101. https://doi.org/10.1128/jvi.02514-15 ![]() |
[113] |
Gaglia MM, Covarrubias S, Wong W, et al. (2012) A common strategy for host RNA degradation by divergent viruses. J Virol 86: 9527-9530. https://doi.org/10.1128/jvi.01230-12 ![]() |
[114] |
Zhang K, Miorin L, Makio T, et al. (2021) Nsp1 protein of SARS-CoV-2 disrupts the mRNA export machinery to inhibit host gene expression. Sci Adv 7: eabe7386. https://doi.org/10.1126/sciadv.abe7386 ![]() |
[115] |
Finkel Y, Gluck A, Nachshon A, et al. (2021) SARS-CoV-2 uses a multipronged strategy to impede host protein synthesis. Nature 594: 240-245. https://doi.org/10.1038/s41586-021-03610-3 ![]() |
[116] |
Lo MW, Woodruff TM (2020) Complement: Bridging the innate and adaptive immune systems in sterile inflammation. J Leukoc Biol 108: 339-351. https://doi.org/10.1002/JLB.3MIR0220-270R ![]() |
[117] |
Hsu JCC, Laurent-Rolle M, Pawlak JB, et al. (2021) Translational shutdown and evasion of the innate immune response by SARS-CoV-2 NSP14 protein. Proc Natl Acad Sci USA 118: e2101161118. https://doi.org/10.1073/pnas.2101161118 ![]() |
[118] |
Noris M, Remuzzi G (2013) Overview of complement activation and regulation. Sem Nephr 33: 479-492. https://doi.org/10.1016/j.semnephrol.2013.08.001 ![]() |
[119] |
Ali YM, Ferrari M, Lynch NJ, et al. (2021) Lectin pathway mediates complement activation by SARS-CoV-2 proteins. Front Immunol 12: 714511. https://doi.org/10.3389/fimmu.2021.714511 ![]() |
[120] |
Holter JC, Pischke SE, de Boer E, et al. (2020) Systemic complement activation isassociated with respiratory failure in COVID-19 hospitalized patients. Proc Natl Acad Sci USA 117: 25018-25025. https://doi.org/10.1073/pnas.2010540117 ![]() |
[121] | Gao T, Hu M, Zhang X, et al. (2020) Highly pathogenic coronavirus N protein aggravates lung injury by MASP-2-mediated complement over-activation. medRxiv . Preprint. http://dx.doi.org/10.1101/2020.03.29.20041962 |
[122] |
Yu J, Yuan X, Chen H, et al. (2020) Direct activation of the alternative complement pathway by SARS-CoV-2 spike proteins is blocked by factor D inhibition. Blood 136: 2080-2089. https://doi.org/10.1182/blood.2020008248 ![]() |
[123] |
Wen W, Chen C, Tang J, et al. (2022b) Efficacy and safety of three new oral antiviral treatment (molnupiravir, fluvoxamine and Paxlovid) for COVID-19: a meta-analysis. Ann Med 54: 516-523. https://doi.org/10.1080/07853890.2022.2034936 ![]() |
[124] |
Bernal AJ, da Silva MMG, Musungaie DB, et al. (2022) Molnupiravir for oral treatment of Covid-19 in nonhospitalized patients. N Engl J Med 386: 509-520. https://doi.org/10.1056/nejmoa2116044 ![]() |
[125] |
Gandhi RT, Malani PN, Del Rio C (2022) COVID-19 therapeutics for nonhospitalized patients. JAMA 327: 617-618. https://doi.org/10.1001/jama.2022.0335 ![]() |
[126] | (2021) Pfizer Inc.Pfizer's novel COVID-19 oral antiviral treatment candidate reduced risk of hospitalization or death by 89% in interim analysis of phase 2/3 EPIC-HR study.Pfizer Inc.. Available from: https://www.pfizer.com/news/press-release/press-release-detail/pfizers-novel-covid-19-oral-antiviral-treatment-candidate |
[127] |
Mahase E (2021b) Covid-19: Pfizer's paxlovid is 89% effective in patients at risk of serious illness, company reports. BMJ 375: n2713. https://doi.org/10.1136/bmj.n2713 ![]() |
[128] |
Seftel D, Boulware DR (2021) Prospective cohort of fluvoxamine for early treatment of Coronavirus disease 19. Open Forum Infect Dis 8: ofab050. https://doi.org/10.1093/ofid/ofab050 ![]() |
[129] |
Calusic M, Marcec R, Luksa L, et al. (2022) Safety and efficacy of fluvoxamine in COVID-19 ICU patients: An open label, prospective cohort trial with matched controls. Br J Clin Pharmacol 88: 2065-2073. https://doi.org/10.1111/bcp.15126 ![]() |
[130] |
Gottlieb RL, Vaca CE, Paredes R, et al. (2022) Early Remdesivir to Prevent Progression to Severe Covid-19 in Outpatients. N Engl J Med 386: 305-315. https://doi.org/10.1056/nejmoa2116846 ![]() |
[131] |
Jason DG, Lye DCB, Hui DS, et al. (2020) Remdesivir for 5 or 10 days in patients with severe COVID-19. N Engl J Med 383: 1827-1837. https://doi.org/10.1056/nejmoa2015301 ![]() |
[132] |
Spinner CD, Gottlieb RL, Criner GJ, et al. (2020) Effect of remdesivir vs standard care on clinical status at 11 days in patients with moderate COVID-19: A randomized clinical trial. JAMA 324: 1048-1057. https://doi.org/10.1001/jama.2020.16349 ![]() |
[133] |
Vegivinti CTR, Evanson KW, Lyons H, et al. (2022) Efficacy of antiviral therapies for COVID-19: a systematic review of randomized controlled trials. BMC Infect Dis 22: 107. https://doi.org/10.1186/s12879-022-07068-0 ![]() |
[134] | Wang L, Zhou T, Zhang Y, et al. (2021) Antibodies with potent and broad neutralizing activity against antigenically diverse and highly transmissible SARS-CoV-2 variants. BioRxiv . Preprint. https://doi.org/10.1101/2021.02.25.432969 |
[135] |
Wang Q, Bowen A, Valdez R, et al. (2023) Antibody response to omicron BA.4-BA.5 bivalent booster. N Engl J Med 388: 567-569. https://doi.org/10.1056/NEJMc2213907 ![]() |
[136] |
Eric JH, Angulo FJ, McLaughlin JM, et al. (2021) Impact and effectiveness of mRNA BNT162b2 vaccine against SARS-CoV-2 infections and COVID-19 cases, hospitalisations, and deaths following a nationwide vaccination campaign in Israel: an observational study using national surveillance data. Lancet 397: 1819-1829. https://doi.org/10.1016/s0140-6736(21)00947-8 ![]() |
[137] |
Thompson MG, Stenehjem E, Grannis S, et al. (2021) Effectiveness of Covid-19 vaccines in ambulatory and inpatient care settings. N Engl J Med 385: 1355-1371. https://doi.org/10.1056/NEJMoa2110362 ![]() |
[138] |
Vasileiou E, Simpson CR, Shi T, et al. (2021) Interim findings from first-dose mass COVID-19 vaccination roll-out and COVID-19 hospital admissions in Scotland: a national prospective cohort study. Lancet 397: 1646-1657. https://doi.org/10.1016/s0140-6736(21)00677-2 ![]() |
[139] |
Al Kaabi N, Zhang Y, Xia S, et al. (2021) Effect of 2 inactivated SARS-CoV-2 vaccines on symptomatic COVID-19 infection in adults: A randomized clinical trial: A randomized clinical trial. JAMA 326: 35-45. https://doi.org/10.1001/jama.2021.8565 ![]() |
[140] |
Offit PA (2023) Bivalent COVID-19 vaccines—A cautionary tale. N Engl J Med 388: 481-483. https://doi.org/10.1056/nejmp2215780 ![]() |
[141] |
Link-Gelles R, Ciesla AA, Fleming-Dutra KE, et al. (2022) Effectiveness of bivalent mRNA vaccines in preventing symptomatic SARS-CoV-2 infection—Increasing Community Access to testing program, United States, September-November 2022. MMWR Morb Mortal Wkly Rep 71: 1526-1530. http://dx.doi.org/10.15585/mmwr.mm7148e1 ![]() |
[142] |
Zeng B, Gao L, Zhou Q, et al. (2022) Effectiveness of COVID-19 vaccines against SARS-CoV-2 variants of concern: a systematic review and meta-analysis. BMC Med 20: 200. https://doi.org/10.1186/s12916-022-02397-y ![]() |
[143] |
Fiolet T, Kherabi Y, MacDonald CJ, et al. (2022) Comparing COVID-19 vaccines fortheir characteristics, efficacy and effectiveness against SARS-CoV-2 and variants of concern: a narrative review. Clin Microbiol Infect 28: 202-221. https://doi.org/10.1016/j.cmi.2021.10.005 ![]() |
[144] | Graña C, Ghosn L, Evrenoglou T, et al. (2022) Efficacy and safety of COVID-19 vaccines. Cochrane Database Syst Rev 12: CD015477. https://doi.org/10.1002/14651858.CD015477 |
[145] |
Scovino AM, Dahab EC, Vieira GF, et al. (2022) SARS-CoV-2's variants of concern: A brief characterization. Front Immunol 13: 834098. https://doi.org/10.3389/fimmu.2022.834098 ![]() |
[146] |
Xu S, Sun M (2022) COVID-19 vaccine effectiveness during Omicron BA.2 pandemic in Shanghai: A cross-sectional study based on EMR. Medicine 101: e31763. https://doi.org/10.1097/MD.0000000000031763 ![]() |
[147] |
Shkoda AS, Gushchin VA, Ogarkova DA, et al. (2022) Sputnik V effectiveness against hospitalization with COVID-19 during Omicron dominance. Vaccines 10: 938. https://doi.org/10.3390/vaccines10060938 ![]() |
[148] | Zheng C, Shao W, Chen X, et al. (2022) Effectiveness of COVID-19 vaccines against SARS-CoV-2 variants of concern in real-world: a literature review and meta-analysis. Int J Infect Dis 11: 2383-2392. https://doi.org/10.1016/j.ijid.2021.11.009 |
[149] |
Cerqueira-Silva T, Andrews JR, Boaventura VS, et al. (2022) Effectiveness of CoronaVac, ChAdOx1 nCoV-19, BNT162b2, and Ad26.COV2.S among individuals with previous SARS-CoV-2 infection in Brazil: a test-negative, case-control study. Lancet Infect Dis 22: 791-801. https://doi.org/10.1016/S1473-3099(22)00140-2 ![]() |
[150] |
Negahdaripour M, Shafiekhani M, Moezzi SMI, et al. (2021) Administration of COVID-19 vaccines in immunocompromised patients. Int Immunopharmacol 99: 108021. https://doi.org/10.1016/j.intimp.2021.108021 ![]() |
[151] |
Zhao J, Zhao S, Ou J, et al. (2020) COVID-19: Coronavirus vaccine development updates. Front Immunol 11: 602256. https://doi.org/10.3389/fimmu.2020.602256 ![]() |
[152] |
Chen Y, Shen H, Huang R, et al. (2021) Serum neutralising activity against SARS-CoV-2 variants elicited by CoronaVac. Lancet Infect Dis 21: 1071-1072. https://doi.org/10.1016/S1473-3099(21)00287-5 ![]() |
[153] |
Olu-Abiodun O, Abiodun O, Okafor N, et al. (2022) COVID-19 vaccination in Nigeria: A rapid review of vaccine acceptance rate and the associated factors. PloS One 17: e0267691. https://doi.org/10.1371/journal.pone.0267691 ![]() |
[154] |
Wu Z, Hu Y, Xu M, et al. (2021) Safety, tolerability, and immunogenicity of an inactivated SARS-CoV-2 vaccine (CoronaVac) in healthy adults aged 60 years and older: a randomised, double-blind, placebo-controlled, phase 1/2 clinical trial. Lancet Infect Dis 21: 803-812. https://doi.org/10.1016/s1473-3099(20)30987-7 ![]() |
[155] |
Jafari A, Pouya FD, Niknam Z, et al. (2022) Current advances and challenges in COVID-19 vaccine development: from conventional vaccines to next-generation vaccine platforms. Mol Biol Rep 49: 4943-4957. https://doi.org/10.1007/s11033-022-07132-7 ![]() |
[156] |
Trimpert J, Dietert K, Firsching TC, et al. (2021) Development of safe and highly protective live-attenuated SARS-CoV-2 vaccine candidates by genome recoding. Cell Rep 36: 109493. https://doi.org/10.1016/j.celrep.2021.109493 ![]() |
[157] |
Jeyanathan M, Afkhami S, Smaill F, et al. (2020) Immunological considerations for COVID-19 vaccine strategies. Nat Rev Immunol 20: 615-632. https://doi.org/10.1038/s41577-020-00434-6 ![]() |
[158] |
Li M, Wang H, Tian L, et al. (2022) COVID-19 vaccine development: milestones, lessons and prospects. Signal Transduct Target Ther 7: 146. https://doi.org/10.1038/s41392-022-00996-y ![]() |
[159] |
Folegatti PM, Ewer KJ, Aley PK, et al. (2020) Safety and immunogenicity of the ChAdOx1 nCoV-19 vaccine against SARS-CoV-2: a preliminary report of a phase 1/2, single-blind, randomised controlled trial. Lancet 396: 467-478. https://doi.org/10.1016/s0140-6736(20)31604-4 ![]() |
[160] |
Zhang Z, Shen Q, Chang H, et al. (2022) Vaccines for COVID-19: A systematic review of immunogenicity, current development, and future prospects. Front Immunol 13: 843928. https://doi.org/10.3389/fimmu.2022.843928 ![]() |
[161] |
Sah R, Shrestha S, Mehta R (2021) AZD1222 (Covishield) vaccination for COVID-19: Experiences, challenges, and solutions in Nepal. Travel Med Infect Dis 40: 101989. https://doi.org/10.1016/j.tmaid.2021.101989 ![]() |
[162] |
Naskalska A, Dabrowska A, Nowak P, et al. (2018) Novel coronavirus-like particles targeting cells lining the respiratory tract. PloS One 13: e0203489. https://doi.org/10.1371/journal.pone.0203489 ![]() |
[163] |
Ward BJ, Gobeil P, Séguin A, et al. (2021) Phase 1 randomized trial of a plant-derived virus-like particle vaccine for COVID-19. Nat Med 27: 1071-1078. https://doi.org/10.1038/s41591-021-01370-1 ![]() |
[164] |
Fang E, Liu X, Li M, et al. (2022) Advances in COVID-19 mRNA vaccinedevelopment. Signal Transduct Target Ther 7: 94. https://doi.org/10.1038/s41392-022-00950-y ![]() |
[165] |
Collier ARY, Miller J, Hachmann NP, et al. (2023) Immunogenicity of the BA.5 bivalent mRNA vaccine boosters. New Engl J Med 388: 565-567. https://doi.org/10.1056/NEJMc2213948 ![]() |
[166] |
Vogel AB, Kanevsky I, Che Y, et al. (2021) BNT162b vaccines protect rhesus macaques from SARS-CoV-2. Nature 592: 283-289. https://doi.org/10.1038/s41586-021-03275-y ![]() |
[167] |
Li M, Li Y, Li S, et al. (2022) The nano delivery systems and applications of mRNA. Eur J Med Chem 227: 113910. https://doi.org/10.1016/j.ejmech.2021.113910 ![]() |
[168] |
Gooch KE, Smith TRF, Salguero FJ, et al. (2021) One or two dose regimen of the SARS-CoV-2 synthetic DNA vaccine INO-4800 protects against respiratory tract disease burden in nonhuman primate challenge model. Vaccine 39: 4885-4894. https://doi.org/10.1016/j.vaccine.2021.06.057 ![]() |
[169] |
Kaur SP, Gupta V (2020) COVID-19 vaccine: a comprehensive status report. Virus Res 288: 198114. https://doi.org/10.1016/j.virusres.2020.198114 ![]() |
[170] |
Tebas P, Yang S, Boyer JD, et al. (2021) Safety and immunogenicity of INO-4800 DNAvaccine against SARS-CoV-2: A preliminary report of an open-label, Phase 1 clinical trial. EClinical Medicine 31: 100689. https://doi.org/10.1016/j.eclinm.2020.100689 ![]() |
[171] |
Brocato RL, Kwilas SA, Kim RK, et al. (2021) Protective efficacy of a SARS-CoV-2 DNA vaccine in wild-type and immunosuppressed Syrian hamsters. NPJ Vaccines 6: 16. https://doi.org/10.1038/s41541-020-00279-z ![]() |
[172] |
Mallory RM, Formica N, Pfeiffer S, et al. (2022) Safety and immunogenicity following a homologous booster dose of a SARS-CoV-2 recombinant spike protein vaccine (NVX-CoV2373): a secondary analysis of a randomised, placebo-controlled, phase 2 trial. Lancet Infect Dis 22: 1565-1576. https://doi.org/10.1016/s1473-3099(22)00420-0 ![]() |
[173] |
Krammer F (2020) SARS-CoV-2 vaccines in development. Nature 586: 516-527. https://doi.org/10.1038/s41586-020-2798-3 ![]() |
[174] |
Sinclair AH, Taylor MK, Weitz JS, et al. (2023) Reasons for receiving or not receiving bivalent COVID-19 booster vaccinations among adults—United States, November 1–December 10, 2022. MMWR Morb Mortal Wkly Rep 72: 73-75. https://doi.org/10.15585/mmwr.mm7203a5 ![]() |
Vaccine name | Platform | Target | Efficacy* | Efficacy against variant strain (reduction%) | Formulation of booster dose | Storage | Reference |
**Pfizer BioNTech (BNT162b2) | mRNA-based vaccine (mRNA expressing a trimeric RBD) and BNT162b2 (mRNA expressing spike protein) | Full-length S protein with proline substitutions | 95% | Alpha B.1.1.7 (93%), Beta B.1351 (75%), Gamma (P1) (88%), Delta B1.617.2 (88%) and Omicron variants (55.9%) | Bivalent | −70 °C (shipping) and 2–8 °C (6 months) | [141],[142] |
**Moderna (mRNA-1273) | mRNA-based vaccine (mRNA expressing spike protein) | Full-length S-2P protein | 95% | Alpha B.1.1.7 (88%), Beta B.1351 (73%), Gamma (P1) (63%), Delta B.1617.2 (77.8%) and Omicron variants (55.9%) | Bivalent | −20 °C (shipping) and 2–8 °C (6 months) | [141],[142] |
Johnson and Johnson (Ad26.CoV2) | Non-replicated viral vector (adenovirus Ad26) | Recombinant replication in competent adenovirus serotype 26 (Ad26) vector encoding full-length S protein | 66–85% | Alpha B.1.1.7 (73.9%), Beta B.1351 (31%), Gamma (P1) and (P2) (62.7%), Delta B.1617.2 (65%) and Omicron variants (55.9%) | Monovalent | 2-8 °C (3 month) and −20 °C (2 years) | [143] |
Oxford/Astrazeneca (ChAdOx1) | Non-replicated viral vector (ChAdOx1-S-(AZD1222)) | Chimpanzee adenovirus vectored vaccine (ChAdOx1) expressing S protein | 70% | Alpha B.1.1.7 (73.9%), Beta B.1351 (31%), Gamma (P1) and (P2) (62.7%), Delta B.1617.2 (65%) and Omicron variants (55.9%) | Monovalent | 2–8 °C (6 months) | [144],[145] |
Novavax (NVX CoV2373) | Recombinant protein vaccine (spike protein + matrix-M adjuvant) | S protein with matrix-M adjuvant | 90% | Alpha B.1.1.7 (89.7%), Beta B.1351 (51.1%), Gamma P1 (91.8%), Delta B.1617.2 (78.7%) and Omicron variants (55.9%) | Monovalent, saponin | 2–8 °C (6 months) | [144],[143] |
Sinovac–coronaVac vaccine | Inactivated vaccine (inactivated virion + aluminium hydroxide) | Whole inactivated SARS-CoV-2 | 50–84% | Alpha B.1.1.7 (71–91%), Beta B1.351 variants (70%), delta B.1617.2 (52%), Gamma (P1) (50%), Omicron BA.2 (62.65%) | Monovalent, aluminum salts | 2–8 °C (6 months) | [146],[147] |
Sputnik V (Gam-Covid-Vac) | Non-replicated viral vector (adenovirus Ad5 and Ad26) | Recombinant Ad26 and recombinant Ad5 encoding fulllength S protein | 92% | Alpha B.1.1.7 (85.7%), Beta B.1351 (81.17%), Delta B.1617.2 (65.35%) and Omicron variants (56.9%) | Monovalent | 2–8 °C (6 months) | [148],[149] |
*: Efficacy against original strain and protection from severe infections; **: Bivalent COVID-19 vaccine approved by the Food and Drug Administration (FDA).
Vaccine name | Platform | Target | Efficacy* | Efficacy against variant strain (reduction%) | Formulation of booster dose | Storage | Reference |
**Pfizer BioNTech (BNT162b2) | mRNA-based vaccine (mRNA expressing a trimeric RBD) and BNT162b2 (mRNA expressing spike protein) | Full-length S protein with proline substitutions | 95% | Alpha B.1.1.7 (93%), Beta B.1351 (75%), Gamma (P1) (88%), Delta B1.617.2 (88%) and Omicron variants (55.9%) | Bivalent | −70 °C (shipping) and 2–8 °C (6 months) | [141],[142] |
**Moderna (mRNA-1273) | mRNA-based vaccine (mRNA expressing spike protein) | Full-length S-2P protein | 95% | Alpha B.1.1.7 (88%), Beta B.1351 (73%), Gamma (P1) (63%), Delta B.1617.2 (77.8%) and Omicron variants (55.9%) | Bivalent | −20 °C (shipping) and 2–8 °C (6 months) | [141],[142] |
Johnson and Johnson (Ad26.CoV2) | Non-replicated viral vector (adenovirus Ad26) | Recombinant replication in competent adenovirus serotype 26 (Ad26) vector encoding full-length S protein | 66–85% | Alpha B.1.1.7 (73.9%), Beta B.1351 (31%), Gamma (P1) and (P2) (62.7%), Delta B.1617.2 (65%) and Omicron variants (55.9%) | Monovalent | 2-8 °C (3 month) and −20 °C (2 years) | [143] |
Oxford/Astrazeneca (ChAdOx1) | Non-replicated viral vector (ChAdOx1-S-(AZD1222)) | Chimpanzee adenovirus vectored vaccine (ChAdOx1) expressing S protein | 70% | Alpha B.1.1.7 (73.9%), Beta B.1351 (31%), Gamma (P1) and (P2) (62.7%), Delta B.1617.2 (65%) and Omicron variants (55.9%) | Monovalent | 2–8 °C (6 months) | [144],[145] |
Novavax (NVX CoV2373) | Recombinant protein vaccine (spike protein + matrix-M adjuvant) | S protein with matrix-M adjuvant | 90% | Alpha B.1.1.7 (89.7%), Beta B.1351 (51.1%), Gamma P1 (91.8%), Delta B.1617.2 (78.7%) and Omicron variants (55.9%) | Monovalent, saponin | 2–8 °C (6 months) | [144],[143] |
Sinovac–coronaVac vaccine | Inactivated vaccine (inactivated virion + aluminium hydroxide) | Whole inactivated SARS-CoV-2 | 50–84% | Alpha B.1.1.7 (71–91%), Beta B1.351 variants (70%), delta B.1617.2 (52%), Gamma (P1) (50%), Omicron BA.2 (62.65%) | Monovalent, aluminum salts | 2–8 °C (6 months) | [146],[147] |
Sputnik V (Gam-Covid-Vac) | Non-replicated viral vector (adenovirus Ad5 and Ad26) | Recombinant Ad26 and recombinant Ad5 encoding fulllength S protein | 92% | Alpha B.1.1.7 (85.7%), Beta B.1351 (81.17%), Delta B.1617.2 (65.35%) and Omicron variants (56.9%) | Monovalent | 2–8 °C (6 months) | [148],[149] |